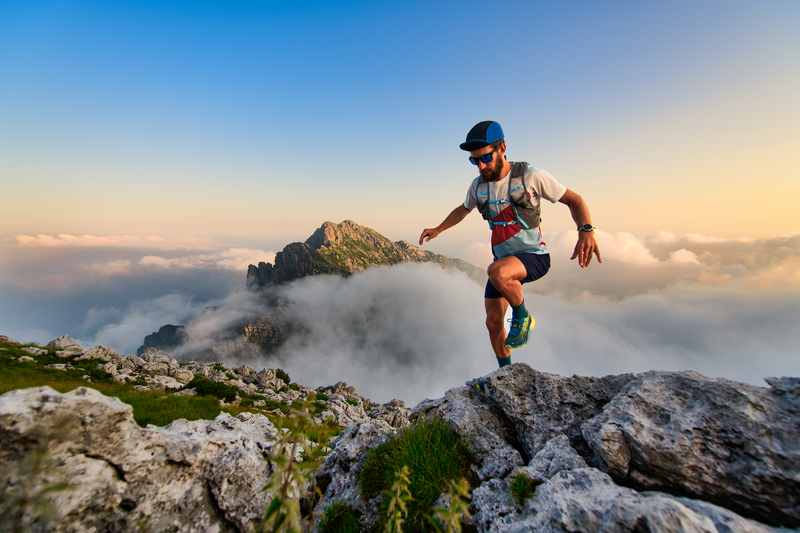
94% of researchers rate our articles as excellent or good
Learn more about the work of our research integrity team to safeguard the quality of each article we publish.
Find out more
REVIEW article
Front. Cell Dev. Biol. , 13 October 2014
Sec. Cell Death and Survival
Volume 2 - 2014 | https://doi.org/10.3389/fcell.2014.00058
This article is part of the Research Topic Promiscuous functions of the prion protein gene family View all 13 articles
The deletion of the cellular form of the prion protein (PrPC) in mouse, goat, and cattle has no drastic phenotypic consequence. This stands in apparent contradiction with PrPC quasi-ubiquitous expression and conserved primary and tertiary structures in mammals, and its pivotal role in neurodegenerative diseases such as prion and Alzheimer's diseases. In zebrafish embryos, depletion of PrP ortholog leads to a severe loss-of-function phenotype. This raises the question of a potential role of PrPC in the development of all vertebrates. This view is further supported by the early expression of the PrPC encoding gene (Prnp) in many tissues of the mouse embryo, the transient disruption of a broad number of cellular pathways in early Prnp−/− mouse embryos, and a growing body of evidence for PrPC involvement in the regulation of cell proliferation and differentiation in various types of mammalian stem cells and progenitors. Finally, several studies in both zebrafish embryos and in mammalian cells and tissues in formation support a role for PrPC in cell adhesion, extra-cellular matrix interactions and cytoskeleton. In this review, we summarize and compare the different models used to decipher PrPC functions at early developmental stages during embryo- and organo-genesis and discuss their relevance.
Prion diseases are a group of fatal and transmissible neurodegenerative diseases affecting a broad range of mammals including humans. The causative agent (the prion) is primarily composed of abnormally folded and aggregated forms of a host-encoded protein, the cellular prion protein (PrPC). PrPC is a glycosyl-phosphatidyl-inositol anchored cell surface sialoglycoprotein associated with lipid rafts (Taylor et al., 2009). PrP primary sequence is highly conserved among mammals (Wopfner et al., 1999) and PrP putative functional domains are structurally conserved between mammals, avians, and fish (Wopfner et al., 1999; Rivera-Milla et al., 2006). PrPC is widely expressed in nearly all the organism, albeit at highest levels in the adult nervous system (Bendheim et al., 1992). Together, these data lend support for an essential role of PrPC in mammals and possibly in vertebrates in general. However, the production of mice, goat, and cattle lacking PrP did not lead to any obvious phenotype (Bueler et al., 1992; Manson et al., 1994a; Richt et al., 2007; Yu et al., 2009) except, for mice, a resistance to experimental prion diseases (Bueler et al., 1993; Prusiner et al., 1993; Manson et al., 1994b; Mallucci et al., 2003). Additionally, goats, naturally devoid of PrPC due to a nonsense mutation, do not seem to present any abnormal phenotype (Benestad et al., 2012). Subtle behavioral and oxidative stress-related alterations have been then reported in adult mice devoid of PrPC (see Table 1) (Tobler et al., 1996; Wong et al., 2001; Roucou et al., 2004; Meotti et al., 2007; Sanchez-Alavez et al., 2008; Le Pichon et al., 2009; Gadotti et al., 2012) although some of them are debated (Steele et al., 2007) and some may be related to Prnp-flanking genes rather than to PrPC absence itself (Nuvolone et al., 2013). However, none of them seems, at first glance, so important to justify the conserved structure and broad expression of PrPC. The physiological role of PrPC still remains highly uncertain despite more than two decades of research and numerous proposed functions (Nicolas et al., 2009; Martins et al., 2010). To conciliate these discrepant data, it has been hypothesized that PrPC function is either dispensable or redundant with that of other proteins. Yet, recent advances, notably in the developmental biology, shed a new light on PrPC functions and suggest that, perhaps, the quest for PrPC functions has been made at the wrong place and/or at the wrong period of time.
The apparent lack of a phenotype in mice invalidated for PrPC sounds at odds with the very early and quasi-ubiquitous expression pattern of the protein at embryonic and postnatal stages, respectively. Expression of the gene encoding PrP—Prnp—is detected in post-implantation embryo from embryonic day (E) 6.5 in extra-embryonic regions (Manson et al., 1992) and from E7.5 (late allantoic bud stage) in cardiac mesoderm (Hidaka et al., 2010). Prnp expression is then observed in the developing central nervous system and heart around E8 before extending rapidly almost to the entire embryo (Tremblay et al., 2007). Prnp may even be expressed earlier on as PrP mRNAs have been found maternally expressed in the zygotes of Xenopus laevis and zebrafish (Danio rerio) (van Rosmalen et al., 2006; Malaga-Trillo et al., 2009).
Two PrP homologs, PrP-1, PrP-2 and a more divergent form, PrP-3, have been identified in zebrafish (Cotto et al., 2005; Rivera-Milla et al., 2006). The expression patterns of the corresponding genes (PrP-1. PrP-2, and PrP-3, respectively) in zebrafish embryos are partially conflicting (Cotto et al., 2005; Malaga-Trillo et al., 2009) although both studies agree on their spatio-temporal complementarity. Both studies describe early PrP gene expression from the mid-blastula stage although it is PrP-2 according to the Cotto et al. study and PrP-1 according to Malaga-Trillo et al. At pharyngula stage (24–48 h postfertilization), both studies show a strong and broad expression of PrP-2 in the developing central nervous system. Additionally, the Cotto et al. study reports the expression of PrP-1 in the floor plate, an essential organizing center of the central nervous system and in the peripheral nervous system around the same developmental period. The expression of PrP-2 and PrP-3 in the developing neuromasts (sensory organs) is also described at the larval stage. PrP-encoding genes are also expressed in developing organs or tissues outside the nervous system such as the heart (PrP-2 and PrP-3), the kidney (PrP-2), the pectoral fins (PrP-2 and PrP-3) and the intestinal epithelium (PrP-2) (Cotto et al., 2005).
In sharp contrast with the situation in mammals, knockdown (KD) of PrP-1 or PrP-2 by morpholino injection led to severe biological phenotypes in zebrafish (see Table 1): gastrulation arrest for PrP-1 KD (Malaga-Trillo et al., 2009) and malformations of the brain and the eyes associated to a reduced number of peripheral neurons for PrP-2 KD (Malaga-Trillo et al., 2009; Nourizadeh-Lillabadi et al., 2010). However, PrP-2 KD-induced phenotype is subject to caution as PrP-2 knockout (KO) by zinc finger nuclease-induced targeted mutation did not lead to any obvious developmental phenotype (Fleisch et al., 2013). Additional and aspecific effects due to morpholino injection may have occurred. There is less concern about PrP-1 KD phenotype as partial rescue was obtained by the injection of PrP-1 mRNA, PrP-2 mRNA and, remarkably, mouse Prnp mRNA (Malaga-Trillo et al., 2009), strongly suggesting that zebrafish and mammalian PrPs may share conserved functions.
PrP inactivation studies in zebrafish allow investigating PrPC functions. They also favor a more critical role of PrPC in early development rather than during postnatal stages (see Table 1). Accordingly, transcriptomic analyses (by RNAseq) of Prnp−/− versus wild-type mouse embryos identified numerous genes differentially expressed (73 and 263 genes at E6.5 and E7.5, respectively) (Khalife et al., 2011), while only 1 gene was found differentially expressed in adult brains (Chadi et al., 2010). Proteomic analysis also failed to identify major alterations (Crecelius et al., 2008) although many cellular stress markers such as protein oxidation, protein ubiquitination, and lipid peroxidation were reportedly activated in the brains of Prnp−/− versus wild-type mice (Wong et al., 2001). This suggests either that PrPC is quite dispensable in the adult brain or that, in a Prnp−/− context, alternative mechanisms are activated during the brain development to compensate the absence of PrPC. Supporting the second hypothesis, the number of genes differentially expressed in the adult Prnp−/− mouse brain was substantially higher upon postnatal depletion in neurons than embryonically (Chadi et al., 2010), albeit to a less extent than in the embryo. The genes differentially expressed in the embryo covered various cell functions including adhesion, apoptosis and proliferation and are related to heart formation and blood vessel development, vascular diseases, immune response, nervous system development, and prion diseases (Khalife et al., 2011). Interestingly and in line with the idea of evolutionary conserved functions of PrPs, similar biological functions were impacted by PrP-2 KD in zebrafish, as revealed by transcriptomic analyses: development including that of the cardiovascular and nervous systems specifically, cell death, cellular assembly, cell cycle, immunological, and neurological diseases (Nourizadeh-Lillabadi et al., 2010).
Finally, the KD of the Sprn gene encoding a PrP-related protein, Shadoo resulted in embryonic lethality between E8 and E11 specifically in Prnp−/− embryos, supporting a role for PrPC and Shadoo in mouse embryogenesis, notably cell adhesion and placenta development, and a possible functional redundancy between the two proteins (Young et al., 2009; Passet et al., 2012). However, the recent generation of viable Prnp−/−; Sprn−/− animals is challenging this view (Daude et al., 2012). These apparently conflicting observations are discussed in Makhzami et al. (2014).
Thus, studying the consequences of PrP inactivation during developmental stages rather than in adult animals could be a more efficient, although not easier, strategy to decipher PrPC functions. Additionally, studying PrPC implications in regenerative processes and in renewing tissues could also be an informative approach (see Table 1).
As PrPC is mediating neuronal dysfunction and degeneration in prion diseases and reportedly in Alzheimer's disease (Lauren et al., 2009; Klyubin et al., 2014), it has been logically suspected to play a role in neuronal homeostasis and during neural development. As PrPC functions in mature neurons are not under the scope of the present review, we will not discuss them and only focus on developmental aspects. In vivo direct evidence supporting a role of PrPC in neural development is quite elusive and PrPC expression pattern remains one of the strongest pro arguments. Indeed, PrPC expression increases as neurons mature, although it is not detected in mitotically active neural progenitor cells (Steele et al., 2006; Peralta et al., 2011). Other experimental evidence was obtained from the comparative study of WT and Prnp−/− and/or Prnp overexpressing animals (see Table 1). The levels of PrPC were found to positively regulate the proliferation in neurogenic regions in adult mice (Steele et al., 2006; Bribian et al., 2012) and the maturation of astrocytes in the developing brain (Arantes et al., 2009; Hartmann et al., 2013). Conversely, the absence of PrPC was associated with an increased proliferation in oligodendrocyte precursor cells both in embryos and adults (Bribian et al., 2012). However, no brain morphology abnormalities or myelin defects could be observed in Prnp−/− animals, casting doubt on a crucial role of PrPC in cell proliferation and maturation during brain development or, at least, suggesting the occurrence of compensatory mechanisms. Yet, electrophysiological recordings on hippocampus from Prnp−/− mice (Collinge et al., 1994) and on cerebellum from juvenile Prnp−/− mice (Prestori et al., 2008) revealed functional alterations. However, no hippocampus-related behavioral or learning alterations were observed in Prnp−/− mice (Bueler et al., 1992) suggesting the hippocampus functional alterations are minor or at least compensated. Conversely, cerebellum functional alterations were found associated to a failure of juvenile Prnp−/− mice in motor control test and to protracted cell proliferation in the cerebellum granular layer at the third week of postnatal development although all these abnormalities vanished in older animals (Prestori et al., 2008). Taken together, these arguments suggest a delay in the maturation of the cerebellar granule cells in Prnp−/− mice.
Further dissecting the underlying mechanisms responsible for the subtle phenotypes in Prnp−/− mice is a particularly arduous task in vivo. For example, determining whether the effects are cell-autonomous would require the use of several transgenic lines or genetic chimeras to limit the expression of PrPC to a subset of cells. And this question is far from being irrelevant as PrPC is a cell surface protein. Such topology could elicit specific cell responses in neighboring cells. Additionally, PrPC may act at distance since secreted forms of have been described both in vitro and in vivo (Borchelt et al., 1993; Harris et al., 1993).
The use of in vitro or ex vivo models has generated a wealth of data with regard to the involvement of PrPC in neural development and, importantly, has comforted the putative functions suggested by the in vivo approaches. Moreover they often allow pinpointing more precisely the cellular processes and signaling pathways involving PrPC. Collectively, these experimental data argue that PrPC positively regulates (1) the differentiation of pluripotent progenitors cells toward the neural lineage (Peralta et al., 2011), (2) the proliferation of neural progenitor cells (Prodromidou et al., 2014) (3) the self-renewal of neural progenitor cells (Santos et al., 2011; Prodromidou et al., 2014) (4) the neuronal differentiation in terms of choice of cell fate (Steele et al., 2006) and (5) acquisition of neuronal features (Graner et al., 2000). However, how PrPC fulfills these different actions is only partially understood. The current idea is that PrPC is part of multiprotein signaling complexes, able to bind various partners, and participate to signal transduction events along different cellular pathways depending on the cellular context (Caughey and Baron, 2006; Watts et al., 2009; Stuermer, 2011; Hirsch et al., 2014). Non-exhaustively, the interaction between PrPC and one of its identified ligand, Stress inducible protein 1 (STI1), has been shown to promote the self-renewal of the neural progenitor cells (Santos et al., 2011). Besides, interaction with the neural cell adhesion molecule (NCAM) can induce their neuronal differentiation (Prodromidou et al., 2014). In cultured neurons, STI1 binding to PrPC increases protein synthesis (Roffe et al., 2010), STI1 and Laminin-γ1 binding promotes intracellular Ca2+ increase and axonogenesis (Santos et al., 2013), and vitronectin binding, axonal growth (Hajj et al., 2007). Experimental evidence also favors a role of PrPC in glia development since the lack of PrPC promotes the proliferation of oligodendrocyte precursor cells and delays their differentiation in culture (Bribian et al., 2012). Studies in astrocyte primary cultures revealed that the involvement of PrPC in astrocytes maturation is dependent upon its interaction with STI1 and the downstream activation of ERK1/2 (Arantes et al., 2009). Of note, PrPC action does not necessarily rely on PrPC expression solely in the cell population considered. For instance, proper neuritogenesis has been shown to require PrPC expression in both neurons and surrounding astrocytes in co-cultures experiments (Lima et al., 2007). Beyond a role in the interactions between both populations, PrPC serves as a neuronal receptor for laminin (Graner et al., 2000) and is also involved in the organization of the extracellular matrix by the astrocytes, especially through laminin deposition (Lima et al., 2007).
It should also be stressed that PrPC partners and the cellular context could tightly determine the final consequences of PrPC stimulation. For example PrPC interaction with contactin-associated protein (Caspr) protects Caspr from proteolysis allowing it to inhibit more efficiently neurite outgrowth (Devanathan et al., 2010). On the opposite, PrPC and NCAM interactions allow stabilization of NCAM in lipid rafts allowing Fyn kinase activation and promoting neurite outgrowth (Santuccione et al., 2005).
In vitro, PrPC is thereby found involved in all the major steps of neural development. Since it notably regulates the self-renewal and differentiation processes in neural progenitor cells, the question arises as to whether this role can be extended to other cell types.
The role of PrPC is far from being restricted to neural development and a growing body of evidence support its involvement in stem cell biology see also Martin-Lannerée et al. (2014). Whether PrPC is expressed in embryonic stem cells (ESC) at basal state remains unclear (Lee and Baskakov, 2010; Miranda et al., 2011), possibly due to limitations in the sensitivity of the detection methods, choice of the target (i.e., the gene or the protein itself) and methods of production and culture of the cells. Nevertheless, there is a consensus that PrPC expression progressively increases during stem cell differentiation (Miranda et al., 2011; Lee and Baskakov, 2013). To understand the role played by PrPC during early embryogenesis, the consequences of Prnp KD or overexpression were studied in human ESC (Lee and Baskakov, 2013). Forcing PrPC expression under self-renewal conditions was found to alter cell cycle regulation and to promote ESC differentiation. Blocking PrPC expression in differentiating ESC also impacts on cell cycle regulation and inhibits the differentiation toward ectodermal lineages, in line with PrPC functions in neural differentiation (Peralta et al., 2011) and the reduced expression of Nestin, an ectodermal marker, observed in ESC in the absence of PrPC (Miranda et al., 2011; Peralta et al., 2011). Mesodermal and endodermal differentiations were, however, not affected. Finally, overexpressing PrPC in differentiating ESC promotes proliferation and inhibits differentiation toward all the lineages (Lee and Baskakov, 2013).
Further supporting PrPC function in stem cell biology, PrPC is expressed in hematopoietic stem cells and promotes their self-renewal (Zhang et al., 2006). PrPC is therefore involved in the self-renewal and differentiation of stem cells although its role and requirement seem to vary along the differentiation process and the cell lineage considered. Identifying the molecular mechanisms at play is a particularly challenging task since self-renewal, differentiation and proliferation are highly intertwined processes impacting each other.
Looking outside the central nervous system in newborn to full-grown animals allowed pinpointing subtle phenotypes in the absence of PrPC (see Table 1), in perfect consistency with PrPC widespread expression. PrPC is expressed in developing human and murine teeth and study of dental cell cultures and teeth from Prnp−/− animals revealed multiple alterations during tooth formation (Schneider et al., 2007; Zhang et al., 2011). In vitro, embryonic dental mesenchymal cells from Prnp−/− embryos proliferate more rapidly and differentiate earlier and coherently, Prnp−/− newborn mice exhibit earlier formation of mesenchymally- and epithelially-derived dentin and enamel, respectively (Zhang et al., 2011).
PrPC is also abundantly expressed in muscles and, although no abnormalities could be observed in a physiological context, it is involved in the regeneration of skeletal muscles in adult mice. Regeneration of locally damaged muscle fibers occurs at slower pace in Prnp−/− animals. Yet total recovery is finally achieved (Stella et al., 2010). This is associated with a longer phase of proliferation and a delayed maturation of muscle precursors, likely due to a reduced level of released myogenic factors (Stella et al., 2010). However, the identity of the releasing cells is difficult to establish, showing again the limits of the in vivo paradigm.
Another extra-neural tissue where PrPC depletion induces alterations is the intestinal epithelium, a constantly renewing tissue. PrPC is expressed by human enterocytes (Morel et al., 2004) and Prnp−/− animals exhibit shorter intestinal villi associated to an increased number of mitotic cells (Morel et al., 2008).
Collectively, these observations support a role of PrPC in the regulation of cell cycle and cell differentiation extraneurally. But how can these functions be exerted?
PrP-1 KD-induced gastrulation arrest in zebrafish embryos is due to impaired morphogenetic cell movements. This defect is, at least partially, caused by the disruption of cellular adhesion (Malaga-Trillo et al., 2009). The study revealed that PrP-1 normally accumulates at cell-cell contacts at early embryonic stages and regulates cell adhesion, including E-cadherin processing and/or storage, in a cell-autonomous way. Such functions may be conserved in mammals as injection of mouse mRNA Prnp partially rescued the PrP-1 KD-induced phenotype (Malaga-Trillo et al., 2009). Consistent with this idea, a number of experimental evidence have linked PrPC to cell adhesion and intimately associated processes such as cytoskeleton remodeling and interactions with extra-cellular matrix (Petit et al., 2013): (i) the expression pattern of PrPC. PrPC is expressed at the cell-to-cell contacts, growth cone, and other extending process tips in cultured neural progenitors and neurons (Santuccione et al., 2005; Devanathan et al., 2010; Miyazawa et al., 2010), cell-to-cell junctions in human intestinal epithelium villi (Morel et al., 2004), cell-to-cell contacts in primary cultured endothelial cells (Viegas et al., 2006), focal adhesions in HeLa cell lines (Schrock et al., 2009) and the apical face of ameloblasts of developing teeth (Zhang et al., 2011); (ii) relevant PrPC partners and ligands, such as cell junction proteins including integrins, the cytoskeleton protein actin and components of the extra-cellular matrix (Graner et al., 2000; Schmitt-Ulms et al., 2001; Morel et al., 2004, 2008; Nieznanski et al., 2005; Hajj et al., 2007; Watts et al., 2009); (iii) alterations in cell adhesion, cytoskeleton dynamics, and extra-cellular matrix interactions upon ectopic expression or deletion of PrPC in different cell systems. Prnp KD leads to alterations in the actin cytoskeleton, remodeling of focal adhesions and over-production of fibronectin in neuronal progenitors (Loubet et al., 2012), to disruption of adherens junctions in carcinoma cells (Solis et al., 2012) and to miss-localization of cell junction-related proteins in enterocytes (Morel et al., 2008). As for Prnp overexpression, it promotes aggregation and filopodia formation as well as alterations of the focal adhesion dynamics in neuroblastoma N2a cells (Mange et al., 2002; Schrock et al., 2009). Subtle changes were even noticed in vivo such as a decrease in the length of desmosomes in the intestinal epithelium of Prnp−/− mice (Morel et al., 2008).
Interestingly, the alterations induced by the deletion of PrPC can be partially rescued by neutralizing antibodies to specific integrins: use of β 1 and β 5 integrins was found to restore neuritogenesis in differentiating PrP-deficient neuronal progenitors (Loubet et al., 2012) and Nanog expression in differentiating PrP-deficient ESC (Miranda et al., 2011), respectively. Moreover, modulation of integrin activity was suspected to be a spontaneous compensatory mechanism occurring in the absence of PrPC (Hajj et al., 2007). This last observation supports an involvement of PrPC in extra-cellular matrix interactions and suggests a possible adaptive mechanism to the absence of PrPC, which may account for the lack of drastic phenotypes in Prnp−/− animals.
Taken together, these observations demonstrate a clear involvement of PrPC in the regulation of cell adhesion, the interactions with the extra-cellular matrix and the cytoskeleton dynamics. The links between these functions and the role of PrPC in the regulation of cell proliferation and differentiation have been the focus of few studies (Miranda et al., 2011; Loubet et al., 2012). Are they under-estimated? And are these functions still ongoing in adult organism?
Little attention has been devoted to the potential functions of PrPC during aging (see Table 1 and also Gasperini and Legname, 2014). It is commonly accepted that the cellular machinery deteriorates or, at least, evolves with aging. As the potential development of adaptive mechanisms to the absence of PrPC is assumed to complicate the study of PrPC functions in Prnp−/− mice, the study of aged animals could be a way to bypass such difficulty: because of the age-related alterations accumulation, the tolerance to PrPC depletion could partially break. In line with this scenario, behavioral differences between WT and Prnp−/− mice seem more pronounced when performed on aged animals (Rial et al., 2009; Massimino et al., 2013). Age-related physiological traits can also be found amplified in absence of PrPC. Myelin abnormalities in the peripheral nervous system accumulate with aging (Verdu et al., 2000) and sciatic nerves from moderately aged mice devoid of PrPC (in selected or all cell populations) showed highly precocious and increased demyelination in the absence of neuronal PrPC (Bremer et al., 2010). If the axons-glia interactions are known to play a key role in myelination during development (Sherman and Brophy, 2005), their role in myelin maintenance in adulthood is less well-understood. However, myelin maintenance is clearly established as an active process (Bremer et al., 2011). The early demyelination observed in the absence of neuronal PrPC suggests axonal PrPC participates in myelin maintenance and is likely required, directly or indirectly, in axons-Schwann cells communication.
Thus, aging may perhaps be the ultimate stage to unravel the mystery of PrPC function(s). However, it has to be considered that aging could also impact on PrPC and/or its function(s). For instance, the age-related change in the cholesterol/sphingolipids ratio in lipid rafts is suspected to alter PrPC compartmentalization (Agostini et al., 2013), which could ultimately impact on its functions. A functional study of PrPC during aging could therefore be difficult to analyse due to the numerous changes occurring at the same time.
A major function of PrPC, consistent with its cell surface expression consists in the modulation of signaling pathways in response to various cues. The panel of putative PrPC ligands is quite large, including soluble ligands, extra-cellular matrix components, and adhesion molecules. Their modes of interaction with PrPC are variable, including cooperation (Santos et al., 2013) and both cis- and trans-interactions for a same ligand (Santuccione et al., 2005). The cellular context can strongly influence the composition of the PrPC-multiprotein complexes and the sub-cellular localization of PrPC is highly dynamic: it can cycle rapidly from the plasma membrane to internal membranes (Griffiths et al., 2007) possibly in response to a given stimulus (Lee et al., 2001; Brown and Harris, 2003; Caetano et al., 2008). All these elements taken together may allow a specific response to a given signal with regard to the cell type and differentiation state. This may explain why PrPC appears to be involved in a multitude of functions. Regarding development, this view perfectly fits the requirement of different stem and progenitor cells by eliciting appropriate cell responses to adapt efficiently to different microenvironments. But how PrPC precisely impacts on stemness maintenance and differentiation processes remains unresolved. The potential implication of PrPC in cell polarization and cytoskeleton remodeling in differentiating stem cells is a very attractive hypothesis and should obviously deserve further investigation. Cell polarity and cytoskeleton dynamics are life-long important cell processes, notably during cell fate determination. They have been studied more particularly during drosophilia neurogenesis and in mammalian neuroepithelial cells (Fietz and Huttner, 2011), but they are fundamental processes for all sort of stem and progenitor cells such as hematopoietic progenitor cells (Bullock et al., 2007). Our unpublished data (Halliez et al., in preparation) reveal a polarized expression of PrPC in neural and cardiovascular progenitor cells of the developing mouse embryo and therefore are compatible with such idea.
If PrPC is involved in so many cell lineages, understanding the lack of drastic phenotype in PrPC-depleted animals remains an ongoing issue. Yet, a modulation of the cell maturation phase (generally delayed but also shortened) is consistently observed in different models (Prnp KO but also KD and overexpression) and may therefore be the general price to pay for the loss of PrPC (see Table 1). This may have a more selective impact on free-range than on laboratory animals (for example acquisition of perfect motor control) and justify the high degree of PrP conservation through evolution. Moreover, building an organism is a highly complex task requiring spatial and temporal fine-tuning of a broad number of biological processes via multiple regulatory networks. Cells, tissues and organs do not develop separately along each other but rely on multiple interactions to develop in a concerted, interconnected, and time-scheduled manner. Partial redundancies into and between signaling pathways ensure robustness to the system. That two or more proteins may exert overlapping functions during development and therefore allow tolerance to the loss of one of them is not unprecedented (Yoon et al., 2005; Santamaria and Ortega, 2006; Nicolae et al., 2007). Moreover, it is not unheard-of, notably in plants where environment adaptation is largely studied, that redundant genes in a regular context allow survival under environmental modifications and stress (Wu et al., 2002). Interestingly, PrP KO animals seem to react differentially than WT animals to stressful situations (Nico et al., 2005) and to alcohol (Rial et al., 2014) and show increased susceptibility to convulsant agents (Walz et al., 1999; Fleisch et al., 2013) (see Table 1). The general function of PrPC could be to facilitate responses to external stimulus/factors at the cell- and at the organism-scale. Yet, laboratory animals are raised in standardized and protective environment and are often homogenous genetically. In such conditions, the time window to apprehend the cell specific functions of PrPC may be restricted to the developmental and perinatal phases, with so much information provided and so much transformation occurring at the same time.
The authors declare that the research was conducted in the absence of any commercial or financial relationships that could be construed as a potential conflict of interest.
Agostini, F., Dotti, C. G., Perez-Canamas, A., Ledesma, M. D., Benetti, F., and Legname, G. (2013). Prion protein accumulation in lipid rafts of mouse aging brain. PLoS ONE 8:e74244. doi: 10.1371/journal.pone.0074244
Pubmed Abstract | Pubmed Full Text | CrossRef Full Text | Google Scholar
Arantes, C., Nomizo, R., Lopes, M. H., Hajj, G. N., Lima, F. R., and Martins, V. R. (2009). Prion protein and its ligand stress inducible protein 1 regulate astrocyte development. Glia 57, 1439–1449. doi: 10.1002/glia.20861
Pubmed Abstract | Pubmed Full Text | CrossRef Full Text | Google Scholar
Bendheim, P. E., Brown, H. R., Rudelli, R. D., Scala, L. J., Goller, N. L., Wen, G. Y., et al. (1992). Nearly ubiquitous tissue distribution of the scrapie agent precursor protein. Neurology 42, 149–156. doi: 10.1212/WNL.42.1.149
Pubmed Abstract | Pubmed Full Text | CrossRef Full Text | Google Scholar
Benestad, S. L., Austbo, L., Tranulis, M. A., Espenes, A., and Olsaker, I. (2012). Healthy goats naturally devoid of prion protein. Vet. Res. 43, 87. doi: 10.1186/1297-9716-43-87
Pubmed Abstract | Pubmed Full Text | CrossRef Full Text | Google Scholar
Borchelt, D. R., Rogers, M., Stahl, N., Telling, G., and Prusiner, S. B. (1993). Release of the cellular prion protein from cultured cells after loss of its glycoinositol phospholipid anchor. Glycobiology 3, 319–329. doi: 10.1093/glycob/3.4.319
Pubmed Abstract | Pubmed Full Text | CrossRef Full Text | Google Scholar
Bremer, J., Baumann, F., Tiberi, C., Wessig, C., Fischer, H., Schwarz, P., et al. (2010). Axonal prion protein is required for peripheral myelin maintenance. Nat. Neurosci. 13, 310–318. doi: 10.1038/nn.2483
Pubmed Abstract | Pubmed Full Text | CrossRef Full Text | Google Scholar
Bremer, M., Frob, F., Kichko, T., Reeh, P., Tamm, E. R., Suter, U., et al. (2011). Sox10 is required for Schwann-cell homeostasis and myelin maintenance in the adult peripheral nerve. Glia 59, 1022–1032. doi: 10.1002/glia.21173
Pubmed Abstract | Pubmed Full Text | CrossRef Full Text | Google Scholar
Bribian, A., Fontana, X., Llorens, F., Gavin, R., Reina, M., Garcia-Verdugo, J. M., et al. (2012). Role of the cellular prion protein in oligodendrocyte precursor cell proliferation and differentiation in the developing and adult mouse CNS. PLoS ONE 7:e33872. doi: 10.1371/journal.pone.0033872
Pubmed Abstract | Pubmed Full Text | CrossRef Full Text | Google Scholar
Brown, L. R., and Harris, D. A. (2003). Copper and zinc cause delivery of the prion protein from the plasma membrane to a subset of early endosomes and the Golgi. J. Neurochem. 87, 353–363. doi: 10.1046/j.1471-4159.2003.01996.x
Pubmed Abstract | Pubmed Full Text | CrossRef Full Text | Google Scholar
Bueler, H., Aguzzi, A., Sailer, A., Greiner, R. A., Autenried, P., Aguet, M., et al. (1993). Mice devoid of PrP are resistant to scrapie. Cell 73, 1339–1347. doi: 10.1016/0092-8674(93)90360-3
Bueler, H., Fischer, M., Lang, Y., Bluethmann, H., Lipp, H. P., DeArmond, S. J., et al. (1992). Normal development and behaviour of mice lacking the neuronal cell-surface PrP protein. Nature 356, 577–582. doi: 10.1038/356577a0
Pubmed Abstract | Pubmed Full Text | CrossRef Full Text | Google Scholar
Bullock, T. E., Wen, B., Marley, S. B., and Gordon, M. Y. (2007). Potential of CD34 in the regulation of symmetrical and asymmetrical divisions by hematopoietic progenitor cells. Stem Cells 25, 844–851. doi: 10.1634/stemcells.2006-0346
Pubmed Abstract | Pubmed Full Text | CrossRef Full Text | Google Scholar
Caetano, F. A., Lopes, M. H., Hajj, G. N., Machado, C. F., Pinto Arantes, C., Magalhaes, A. C., et al. (2008). Endocytosis of prion protein is required for ERK1/2 signaling induced by stress-inducible protein 1. J. Neurosci. 28, 6691–6702. doi: 10.1523/JNEUROSCI.1701-08.2008
Pubmed Abstract | Pubmed Full Text | CrossRef Full Text | Google Scholar
Caughey, B., and Baron, G. S. (2006). Prions and their partners in crime. Nature 443, 803–810. doi: 10.1038/nature05294
Pubmed Abstract | Pubmed Full Text | CrossRef Full Text | Google Scholar
Chadi, S., Young, R., Le Guillou, S., Tilly, G., Bitton, F., Martin-Magniette, M. L., et al. (2010). Brain transcriptional stability upon prion protein-encoding gene invalidation in zygotic or adult mouse. BMC Genomics 11:448. doi: 10.1186/1471-2164-11-448
Pubmed Abstract | Pubmed Full Text | CrossRef Full Text | Google Scholar
Collinge, J., Whittington, M. A., Sidle, K. C., Smith, C. J., Palmer, M. S., Clarke, A. R., et al. (1994). Prion protein is necessary for normal synaptic function. Nature 370, 295–297. doi: 10.1038/370295a0
Pubmed Abstract | Pubmed Full Text | CrossRef Full Text | Google Scholar
Cotto, E., Andre, M., Forgue, J., Fleury, H. J., and Babin, P. J. (2005). Molecular characterization, phylogenetic relationships, and developmental expression patterns of prion genes in zebrafish (Danio rerio). FEBS J. 272, 500–513. doi: 10.1111/j.1742-4658.2004.04492.x
Pubmed Abstract | Pubmed Full Text | CrossRef Full Text | Google Scholar
Crecelius, A. C., Helmstetter, D., Strangmann, J., Mitteregger, G., Frohlich, T., Arnold, G. J., et al. (2008). The brain proteome profile is highly conserved between Prnp−/− and Prnp+/+ mice. Neuroreport 19, 1027–1031. doi: 10.1097/WNR.0b013e3283046157
Pubmed Abstract | Pubmed Full Text | CrossRef Full Text | Google Scholar
Daude, N., Wohlgemuth, S., Brown, R., Pitstick, R., Gapeshina, H., Yang, J., et al. (2012). Knockout of the prion protein (PrP)-like Sprn gene does not produce embryonic lethality in combination with PrP(C)-deficiency. Proc. Natl. Acad. Sci. U.S.A. 109, 9035–9040. doi: 10.1073/pnas.1202130109
Pubmed Abstract | Pubmed Full Text | CrossRef Full Text | Google Scholar
Devanathan, V., Jakovcevski, I., Santuccione, A., Li, S., Lee, H. J., Peles, E., et al. (2010). Cellular form of prion protein inhibits Reelin-mediated shedding of Caspr from the neuronal cell surface to potentiate Caspr-mediated inhibition of neurite outgrowth. J. Neurosci. 30, 9292–9305. doi: 10.1523/JNEUROSCI.5657-09.2010
Pubmed Abstract | Pubmed Full Text | CrossRef Full Text | Google Scholar
Fietz, S. A., and Huttner, W. B. (2011). Cortical progenitor expansion, self-renewal and neurogenesis-a polarized perspective. Curr. Opin. Neurobiol. 21, 23–35. doi: 10.1016/j.conb.2010.10.002
Pubmed Abstract | Pubmed Full Text | CrossRef Full Text | Google Scholar
Fleisch, V. C., Leighton, P. L., Wang, H., Pillay, L. M., Ritzel, R. G., Bhinder, G., et al. (2013). Targeted mutation of the gene encoding prion protein in zebrafish reveals a conserved role in neuron excitability. Neurobiol. Dis. 55, 11–25. doi: 10.1016/j.nbd.2013.03.007
Pubmed Abstract | Pubmed Full Text | CrossRef Full Text | Google Scholar
Gadotti, V. M., Bonfield, S. P., and Zamponi, G. W. (2012). Depressive-like behaviour of mice lacking cellular prion protein. Behav. Brain Res. 227, 319–323. doi: 10.1016/j.bbr.2011.03.012
Pubmed Abstract | Pubmed Full Text | CrossRef Full Text | Google Scholar
Gasperini, L., and Legname, G. (2014). Prion protein and aging. Front. Cell Dev. Biol. 2:44. doi: 10.3389/fcell.2014.00044
Graner, E., Mercadante, A. F., Zanata, S. M., Forlenza, O. V., Cabral, A. L., Veiga, S. S., et al. (2000). Cellular prion protein binds laminin and mediates neuritogenesis. Brain Res. Mol. Brain Res. 76, 85–92. doi: 10.1016/S0169-328X(99)00334-4
Pubmed Abstract | Pubmed Full Text | CrossRef Full Text | Google Scholar
Griffiths, R. E., Heesom, K. J., and Anstee, D. J. (2007). Normal prion protein trafficking in cultured human erythroblasts. Blood 110, 4518–4525. doi: 10.1182/blood-2007-04-085183
Pubmed Abstract | Pubmed Full Text | CrossRef Full Text | Google Scholar
Hajj, G. N., Lopes, M. H., Mercadante, A. F., Veiga, S. S., da Silveira, R. B., Santos, T. G., et al. (2007). Cellular prion protein interaction with vitronectin supports axonal growth and is compensated by integrins. J. Cell Sci. 120, 1915–1926. doi: 10.1242/jcs.03459
Pubmed Abstract | Pubmed Full Text | CrossRef Full Text | Google Scholar
Harris, D. A., Huber, M. T., van Dijken, P., Shyng, S. L., Chait, B. T., and Wang, R. (1993). Processing of a cellular prion protein: identification of N- and C-terminal cleavage sites. Biochemistry 32, 1009–1016. doi: 10.1021/bi00055a003
Pubmed Abstract | Pubmed Full Text | CrossRef Full Text | Google Scholar
Hartmann, C. A., Martins, V. R., and Lima, F. R. (2013). High levels of cellular prion protein improve astrocyte development. FEBS Lett. 587, 238–244. doi: 10.1016/j.febslet.2012.11.032
Pubmed Abstract | Pubmed Full Text | CrossRef Full Text | Google Scholar
Hidaka, K., Shirai, M., Lee, J. K., Wakayama, T., Kodama, I., Schneider, M. D., et al. (2010). The cellular prion protein identifies bipotential cardiomyogenic progenitors. Circ. Res. 106, 111–119. doi: 10.1161/CIRCRESAHA.109.209478
Pubmed Abstract | Pubmed Full Text | CrossRef Full Text | Google Scholar
Hirsch, T. Z., Hernandez-Rapp, J., Martin-Lannerée, S., Launay, J. M., and Mouillet-Richard, S. (2014). PrP signalling in neurons: from basics to clinical challenges. Biochimie. 104, 2–11. doi: 10.1016/j.biochi.2014.06.009.
Pubmed Abstract | Pubmed Full Text | CrossRef Full Text | Google Scholar
Khalife, M., Young, R., Passet, B., Halliez, S., Vilotte, M., Jaffrezic, F., et al. (2011). Transcriptomic analysis brings new insight into the biological role of the prion protein during mouse embryogenesis. PLoS ONE 6:e23253. doi: 10.1371/journal.pone.0023253
Pubmed Abstract | Pubmed Full Text | CrossRef Full Text | Google Scholar
Klyubin, I., Nicoll, A. J., Khalili-Shirazi, A., Farmer, M., Canning, S., Mably, A., et al. (2014). Peripheral administration of a humanized anti-PrP antibody blocks Alzheimer's disease abeta synaptotoxicity. J. Neurosci. 34, 6140–6145. doi: 10.1523/JNEUROSCI.3526-13.2014
Pubmed Abstract | Pubmed Full Text | CrossRef Full Text | Google Scholar
Lauren, J., Gimbel, D. A., Nygaard, H. B., Gilbert, J. W., and Strittmatter, S. M. (2009). Cellular prion protein mediates impairment of synaptic plasticity by amyloid-beta oligomers. Nature 457, 1128–1132. doi: 10.1038/nature07761
Pubmed Abstract | Pubmed Full Text | CrossRef Full Text | Google Scholar
Lee, K. S., Magalhaes, A. C., Zanata, S. M., Brentani, R. R., Martins, V. R., and Prado, M. A. (2001). Internalization of mammalian fluorescent cellular prion protein and N-terminal deletion mutants in living cells. J. Neurochem. 79, 79–87. doi: 10.1046/j.1471-4159.2001.00529.x
Pubmed Abstract | Pubmed Full Text | CrossRef Full Text | Google Scholar
Lee, Y. J., and Baskakov, I. V. (2010). Treatment with normal prion protein delays differentiation and helps to maintain high proliferation activity in human embryonic stem cells. J. Neurochem. 114, 362–373. doi: 10.1111/j.1471-4159.2010.06601.x
Pubmed Abstract | Pubmed Full Text | CrossRef Full Text | Google Scholar
Lee, Y. J., and Baskakov, I. V. (2013). The cellular form of the prion protein is involved in controlling cell cycle dynamics, self-renewal, and the fate of human embryonic stem cell differentiation. J. Neurochem. 124, 310–322. doi: 10.1111/j.1471-4159.2012.07913.x
Pubmed Abstract | Pubmed Full Text | CrossRef Full Text | Google Scholar
Le Pichon, C. E., Valley, M. T., Polymenidou, M., Chesler, A. T., Sagdullaev, B. T., Aguzzi, A., et al. (2009). Olfactory behavior and physiology are disrupted in prion protein knockout mice. Nat. Neurosci. 12, 60–69. doi: 10.1038/nn.2238
Pubmed Abstract | Pubmed Full Text | CrossRef Full Text | Google Scholar
Lima, F. R., Arantes, C. P., Muras, A. G., Nomizo, R., Brentani, R. R., and Martins, V. R. (2007). Cellular prion protein expression in astrocytes modulates neuronal survival and differentiation. J. Neurochem. 103, 2164–2176. doi: 10.1111/j.1471-4159.2007.04904.x
Pubmed Abstract | Pubmed Full Text | CrossRef Full Text | Google Scholar
Loubet, D., Dakowski, C., Pietri, M., Pradines, E., Bernard, S., Callebert, J., et al. (2012). Neuritogenesis: the prion protein controls beta1 integrin signaling activity. FASEB J. 26, 678–690. doi: 10.1096/fj.11-185579
Pubmed Abstract | Pubmed Full Text | CrossRef Full Text | Google Scholar
Makhzami, S., Passet, B., Halliez, S., Castille, J., Moazami-Goudarzi, K., Duchesne, A., et al. (2014). The prion protein family: a view from the placenta. Front. Cell Dev. Biol. 2:35. doi: 10.3389/fcell.2014.00035
Malaga-Trillo, E., Solis, G. P., Schrock, Y., Geiss, C., Luncz, L., Thomanetz, V., et al. (2009). Regulation of embryonic cell adhesion by the prion protein. PLoS Biol. 7:e55. doi: 10.1371/journal.pbio.1000055
Pubmed Abstract | Pubmed Full Text | CrossRef Full Text | Google Scholar
Mallucci, G., Dickinson, A., Linehan, J., Klohn, P. C., Brandner, S., and Collinge, J. (2003). Depleting neuronal PrP in prion infection prevents disease and reverses spongiosis. Science 302, 871–874. doi: 10.1126/science.1090187
Pubmed Abstract | Pubmed Full Text | CrossRef Full Text | Google Scholar
Mange, A., Milhavet, O., Umlauf, D., Harris, D., and Lehmann, S. (2002). PrP-dependent cell adhesion in N2a neuroblastoma cells. FEBS Lett. 514, 159–162. doi: 10.1016/S0014-5793(02)02338-4
Pubmed Abstract | Pubmed Full Text | CrossRef Full Text | Google Scholar
Manson, J. C., Clarke, A. R., Hooper, M. L., Aitchison, L., McConnell, I., and Hope, J. (1994a). 129/Ola mice carrying a null mutation in PrP that abolishes mRNA production are developmentally normal. Mol. Neurobiol. 8, 121–127. doi: 10.1007/BF02780662
Pubmed Abstract | Pubmed Full Text | CrossRef Full Text | Google Scholar
Manson, J. C., Clarke, A. R., McBride, P. A., McConnell, I., and Hope, J. (1994b). PrP gene dosage determines the timing but not the final intensity or distribution of lesions in scrapie pathology. Neurodegeneration 3, 331–340.
Manson, J., West, J. D., Thomson, V., McBride, P., Kaufman, M. H., and Hope, J. (1992). The prion protein gene: a role in mouse embryogenesis? Development 115, 117–122.
Martin-Lannerée, S., Hirsch, T. Z., Hernandez-Rapp, J., Halliez, S., Vilotte, J.-L., Launay, J.-M., et al. (2014). PrPC from stem cells to cancer. Front. Cell Dev. Biol. 2:55. doi: 10.3389/fcell.2014.00055
Martins, V. R., Beraldo, F. H., Hajj, G. N., Lopes, M. H., Lee, K. S., Prado, M. A., et al. (2010). Prion protein: orchestrating neurotrophic activities. Curr. Issues Mol. Biol. 12, 63–86.
Massimino, M. L., Redaelli, M., Bertoli, A., Sorgato, M. C., and Mucignat-Caretta, C. (2013). Altered behavioral aspects of aged mice lacking the cellular prion protein. Physiol. Behav. 119, 86–91. doi: 10.1016/j.physbeh.2013.06.006
Pubmed Abstract | Pubmed Full Text | CrossRef Full Text | Google Scholar
Meotti, F. C., Carqueja, C. L., Gadotti Vde, M., Tasca, C. I., Walz, R., and Santos, A. R. (2007). Involvement of cellular prion protein in the nociceptive response in mice. Brain Res. 1151, 84–90. doi: 10.1016/j.brainres.2007.03.024
Pubmed Abstract | Pubmed Full Text | CrossRef Full Text | Google Scholar
Miranda, A., Pericuesta, E., Ramirez, M. A., and Gutierrez-Adan, A. (2011). Prion protein expression regulates embryonic stem cell pluripotency and differentiation. PLoS ONE 6:e18422. doi: 10.1371/journal.pone.0018422
Pubmed Abstract | Pubmed Full Text | CrossRef Full Text | Google Scholar
Miyazawa, K., Emmerling, K., and Manuelidis, L. (2010). Proliferative arrest of neural cells induces prion protein synthesis, nanotube formation, and cell-to-cell contacts. J. Cell. Biochem. 111, 239–247. doi: 10.1002/jcb.22723
Pubmed Abstract | Pubmed Full Text | CrossRef Full Text | Google Scholar
Morel, E., Fouquet, S., Chateau, D., Yvernault, L., Frobert, Y., Pincon-Raymond, M., et al. (2004). The cellular prion protein PrPc is expressed in human enterocytes in cell-cell junctional domains. J. Biol. Chem. 279, 1499–1505. doi: 10.1074/jbc.M308578200
Pubmed Abstract | Pubmed Full Text | CrossRef Full Text | Google Scholar
Morel, E., Fouquet, S., Strup-Perrot, C., Pichol Thievend, C., Petit, C., Loew, D., et al. (2008). The cellular prion protein PrP(c) is involved in the proliferation of epithelial cells and in the distribution of junction-associated proteins. PLoS ONE 3:e3000. doi: 10.1371/journal.pone.0003000
Pubmed Abstract | Pubmed Full Text | CrossRef Full Text | Google Scholar
Nico, P. B., de-Paris, F., Vinade, E. R., Amaral, O. B., Rockenbach, I., Soares, B. L., et al. (2005). Altered behavioural response to acute stress in mice lacking cellular prion protein. Behav. Brain Res. 162, 173–181. doi: 10.1016/j.bbr.2005.02.003
Pubmed Abstract | Pubmed Full Text | CrossRef Full Text | Google Scholar
Nicolae, C., Ko, Y. P., Miosge, N., Niehoff, A., Studer, D., Enggist, L., et al. (2007). Abnormal collagen fibrils in cartilage of matrilin-1/matrilin-3-deficient mice. J. Biol. Chem. 282, 22163–22175. doi: 10.1074/jbc.M610994200
Pubmed Abstract | Pubmed Full Text | CrossRef Full Text | Google Scholar
Nicolas, O., Gavin, R., and del Rio, J. A. (2009). New insights into cellular prion protein (PrPc) functions: the “ying and yang” of a relevant protein. Brain Res. Rev. 61, 170–184. doi: 10.1016/j.brainresrev.2009.06.002
Pubmed Abstract | Pubmed Full Text | CrossRef Full Text | Google Scholar
Nieznanski, K., Nieznanska, H., Skowronek, K. J., Osiecka, K. M., and Stepkowski, D. (2005). Direct interaction between prion protein and tubulin. Biochem. Biophys. Res. Commun. 334, 403–411. doi: 10.1016/j.bbrc.2005.06.092
Pubmed Abstract | Pubmed Full Text | CrossRef Full Text | Google Scholar
Nourizadeh-Lillabadi, R., Seilo Torgersen, J., Vestrheim, O., Konig, M., Alestrom, P., and Syed, M. (2010). Early embryonic gene expression profiling of zebrafish prion protein (Prp2) morphants. PLoS ONE 5:e13573. doi: 10.1371/journal.pone.0013573
Pubmed Abstract | Pubmed Full Text | CrossRef Full Text | Google Scholar
Nuvolone, M., Kana, V., Hutter, G., Sakata, D., Mortin-Toth, S. M., Russo, G., et al. (2013). SIRPalpha polymorphisms, but not the prion protein, control phagocytosis of apoptotic cells. J. Exp. Med. 210, 2539–2552. doi: 10.1084/jem.20131274
Pubmed Abstract | Pubmed Full Text | CrossRef Full Text | Google Scholar
Passet, B., Young, R., Makhzami, S., Vilotte, M., Jaffrezic, F., Halliez, S., et al. (2012). Prion protein and Shadoo are involved in overlapping embryonic pathways and trophoblastic development. PLoS ONE 7:e41959. doi: 10.1371/journal.pone.0041959
Pubmed Abstract | Pubmed Full Text | CrossRef Full Text | Google Scholar
Peralta, O. A., Huckle, W. R., and Eyestone, W. H. (2011). Expression and knockdown of cellular prion protein (PrPC) in differentiating mouse embryonic stem cells. Differentiation 81, 68–77. doi: 10.1016/j.diff.2010.09.181
Pubmed Abstract | Pubmed Full Text | CrossRef Full Text | Google Scholar
Petit, C. S., Besnier, L., Morel, E., Rousset, M., and Thenet, S. (2013). Roles of the cellular prion protein in the regulation of cell-cell junctions and barrier function. Tissue Barriers 1:e24377. doi: 10.4161/tisb.24377
Pubmed Abstract | Pubmed Full Text | CrossRef Full Text | Google Scholar
Prestori, F., Rossi, P., Bearzatto, B., Laine, J., Necchi, D., Diwakar, S., et al. (2008). Altered neuron excitability and synaptic plasticity in the cerebellar granular layer of juvenile prion protein knock-out mice with impaired motor control. J. Neurosci. 28, 7091–7103. doi: 10.1523/JNEUROSCI.0409-08.2008
Pubmed Abstract | Pubmed Full Text | CrossRef Full Text | Google Scholar
Prodromidou, K., Papastefanaki, F., Sklaviadis, T., and Matsas, R. (2014). Functional cross-talk between the cellular prion protein and the neural cell adhesion molecule is critical for neuronal differentiation of neural stem/precursor cells. Stem Cells 32, 1674–1687. doi: 10.1002/stem.1663
Pubmed Abstract | Pubmed Full Text | CrossRef Full Text | Google Scholar
Prusiner, S. B., Groth, D., Serban, A., Koehler, R., Foster, D., Torchia, M., et al. (1993). Ablation of the prion protein (PrP) gene in mice prevents scrapie and facilitates production of anti-PrP antibodies. Proc. Natl. Acad. Sci. U.S.A. 90, 10608–10612. doi: 10.1073/pnas.90.22.10608
Pubmed Abstract | Pubmed Full Text | CrossRef Full Text | Google Scholar
Rial, D., Duarte, F. S., Xikota, J. C., Schmitz, A. E., Dafre, A. L., Figueiredo, C. P., et al. (2009). Cellular prion protein modulates age-related behavioral and neurochemical alterations in mice. Neuroscience 164, 896–907. doi: 10.1016/j.neuroscience.2009.09.005
Pubmed Abstract | Pubmed Full Text | CrossRef Full Text | Google Scholar
Rial, D., Pandolfo, P., Bitencourt, R. M., Pamplona, F. A., Moreira, K. M., Hipolide, D., et al. (2014). Cellular prion protein (PrP(C)) modulates ethanol-induced behavioral adaptive changes in mice. Behav. Brain Res. 271, 325–332. doi: 10.1016/j.bbr.2014.05.067
Pubmed Abstract | Pubmed Full Text | CrossRef Full Text | Google Scholar
Richt, J. A., Kasinathan, P., Hamir, A. N., Castilla, J., Sathiyaseelan, T., Vargas, F., et al. (2007). Production of cattle lacking prion protein. Nat. Biotechnol. 25, 132–138. doi: 10.1038/nbt1271
Pubmed Abstract | Pubmed Full Text | CrossRef Full Text | Google Scholar
Rivera-Milla, E., Oidtmann, B., Panagiotidis, C. H., Baier, M., Sklaviadis, T., Hoffmann, R., et al. (2006). Disparate evolution of prion protein domains and the distinct origin of Doppel- and prion-related loci revealed by fish-to-mammal comparisons. FASEB J. 20, 317–319. doi: 10.1096/fj.05-4279fje
Pubmed Abstract | Pubmed Full Text | CrossRef Full Text | Google Scholar
Roffe, M., Beraldo, F. H., Bester, R., Nunziante, M., Bach, C., Mancini, G., et al. (2010). Prion protein interaction with stress-inducible protein 1 enhances neuronal protein synthesis via mTOR. Proc. Natl. Acad. Sci. U.S.A. 107, 13147–13152. doi: 10.1073/pnas.1000784107
Pubmed Abstract | Pubmed Full Text | CrossRef Full Text | Google Scholar
Roucou, X., Gains, M., and LeBlanc, A. C. (2004). Neuroprotective functions of prion protein. J. Neurosci. Res. 75, 153–161. doi: 10.1002/jnr.10864
Pubmed Abstract | Pubmed Full Text | CrossRef Full Text | Google Scholar
Sanchez-Alavez, M., Criado, J. R., Klein, I., Moroncini, G., and Conti, B. (2008). Hypothalamic-pituitary-adrenal axis disregulation in PrPC-null mice. Neuroreport 19, 1473–1477. doi: 10.1097/WNR.0b013e32830f1e90
Pubmed Abstract | Pubmed Full Text | CrossRef Full Text | Google Scholar
Santamaria, D., and Ortega, S. (2006). Cyclins and CDKS in development and cancer: lessons from genetically modified mice. Front. Biosci. 11, 1164–1188. doi: 10.2741/1871
Pubmed Abstract | Pubmed Full Text | CrossRef Full Text | Google Scholar
Santos, T. G., Beraldo, F. H., Hajj, G. N., Lopes, M. H., Roffe, M., Lupinacci, F. C., et al. (2013). Laminin-gamma1 chain and stress inducible protein 1 synergistically mediate PrPC-dependent axonal growth via Ca2+ mobilization in dorsal root ganglia neurons. J. Neurochem. 124, 210–223. doi: 10.1111/jnc.12091
Pubmed Abstract | Pubmed Full Text | CrossRef Full Text | Google Scholar
Santos, T. G., Silva, I. R., Costa-Silva, B., Lepique, A. P., Martins, V. R., and Lopes, M. H. (2011). Enhanced neural progenitor/stem cells self-renewal via the interaction of stress-inducible protein 1 with the prion protein. Stem Cells 29, 1126–1136. doi: 10.1002/stem.664
Pubmed Abstract | Pubmed Full Text | CrossRef Full Text | Google Scholar
Santuccione, A., Sytnyk, V., Leshchyns'ka, I., and Schachner, M. (2005). Prion protein recruits its neuronal receptor NCAM to lipid rafts to activate p59fyn and to enhance neurite outgrowth. J. Cell Biol. 169, 341–354. doi: 10.1083/jcb.200409127
Pubmed Abstract | Pubmed Full Text | CrossRef Full Text | Google Scholar
Schmitt-Ulms, G., Legname, G., Baldwin, M. A., Ball, H. L., Bradon, N., Bosque, P. J., et al. (2001). Binding of neural cell adhesion molecules (N-CAMs) to the cellular prion protein. J. Mol. Biol. 314, 1209–1225. doi: 10.1006/jmbi.2000.5183
Pubmed Abstract | Pubmed Full Text | CrossRef Full Text | Google Scholar
Schneider, K., Korkmaz, Y., Addicks, K., Lang, H., and Raab, W. H. (2007). Prion protein (PrP) in human teeth: an unprecedented pointer to PrP's function. J. Endod. 33, 110–113. doi: 10.1016/j.joen.2006.11.010
Pubmed Abstract | Pubmed Full Text | CrossRef Full Text | Google Scholar
Schrock, Y., Solis, G. P., and Stuermer, C. A. (2009). Regulation of focal adhesion formation and filopodia extension by the cellular prion protein (PrPC). FEBS Lett. 583, 389–393. doi: 10.1016/j.febslet.2008.12.038
Pubmed Abstract | Pubmed Full Text | CrossRef Full Text | Google Scholar
Sherman, D. L., and Brophy, P. J. (2005). Mechanisms of axon ensheathment and myelin growth. Nat. Rev. Neurosci. 6, 683–690. doi: 10.1038/nrn1743
Pubmed Abstract | Pubmed Full Text | CrossRef Full Text | Google Scholar
Solis, G. P., Schrock, Y., Hulsbusch, N., Wiechers, M., Plattner, H., and Stuermer, C. A. (2012). Reggies/flotillins regulate E-cadherin-mediated cell contact formation by affecting EGFR trafficking. Mol. Biol. Cell 23, 1812–1825. doi: 10.1091/mbc.E11-12-1006
Pubmed Abstract | Pubmed Full Text | CrossRef Full Text | Google Scholar
Steele, A. D., Emsley, J. G., Ozdinler, P. H., Lindquist, S., and Macklis, J. D. (2006). Prion protein (PrPc) positively regulates neural precursor proliferation during developmental and adult mammalian neurogenesis. Proc. Natl. Acad. Sci. U.S.A. 103, 3416–3421. doi: 10.1073/pnas.0511290103
Pubmed Abstract | Pubmed Full Text | CrossRef Full Text | Google Scholar
Steele, A. D., Lindquist, S., and Aguzzi, A. (2007). The prion protein knockout mouse: a phenotype under challenge. Prion 1, 83–93. doi: 10.4161/pri.1.2.4346
Pubmed Abstract | Pubmed Full Text | CrossRef Full Text | Google Scholar
Stella, R., Massimino, M. L., Sandri, M., Sorgato, M. C., and Bertoli, A. (2010). Cellular prion protein promotes regeneration of adult muscle tissue. Mol. Cell. Biol. 30, 4864–4876. doi: 10.1128/MCB.01040-09
Pubmed Abstract | Pubmed Full Text | CrossRef Full Text | Google Scholar
Stuermer, C. A. (2011). Reggie/flotillin and the targeted delivery of cargo. J. Neurochem. 116, 708–713. doi: 10.1111/j.1471-4159.2010.07007.x
Pubmed Abstract | Pubmed Full Text | CrossRef Full Text | Google Scholar
Taylor, D. R., Whitehouse, I. J., and Hooper, N. M. (2009). Glypican-1 mediates both prion protein lipid raft association and disease isoform formation. PLoS Pathog. 5:e1000666. doi: 10.1371/journal.ppat.1000666
Pubmed Abstract | Pubmed Full Text | CrossRef Full Text | Google Scholar
Tobler, I., Gaus, S. E., Deboer, T., Achermann, P., Fischer, M., Rulicke, T., et al. (1996). Altered circadian activity rhythms and sleep in mice devoid of prion protein. Nature 380, 639–642. doi: 10.1038/380639a0
Pubmed Abstract | Pubmed Full Text | CrossRef Full Text | Google Scholar
Tremblay, P., Bouzamondo-Bernstein, E., Heinrich, C., Prusiner, S. B., and DeArmond, S. J. (2007). Developmental expression of PrP in the post-implantation embryo. Brain Res. 1139, 60–67. doi: 10.1016/j.brainres.2006.12.055
Pubmed Abstract | Pubmed Full Text | CrossRef Full Text | Google Scholar
van Rosmalen, J. W., Born, J. M., and Martens, G. J. (2006). Prion protein mRNA expression in Xenopus laevis: no induction during melanotrope cell activation. Brain Res. 1075, 20–25. doi: 10.1016/j.brainres.2005.12.105
Pubmed Abstract | Pubmed Full Text | CrossRef Full Text | Google Scholar
Verdu, E., Ceballos, D., Vilches, J. J., and Navarro, X. (2000). Influence of aging on peripheral nerve function and regeneration. J. Peripher. Nerv. Syst. 5, 191–208. doi: 10.1046/j.1529-8027.2000.00026.x
Pubmed Abstract | Pubmed Full Text | CrossRef Full Text | Google Scholar
Viegas, P., Chaverot, N., Enslen, H., Perriere, N., Couraud, P. O., and Cazaubon, S. (2006). Junctional expression of the prion protein PrPC by brain endothelial cells: a role in trans-endothelial migration of human monocytes. J. Cell Sci. 119, 4634–4643. doi: 10.1242/jcs.03222
Pubmed Abstract | Pubmed Full Text | CrossRef Full Text | Google Scholar
Walz, R., Amaral, O. B., Rockenbach, I. C., Roesler, R., Izquierdo, I., Cavalheiro, E. A., et al. (1999). Increased sensitivity to seizures in mice lacking cellular prion protein. Epilepsia 40, 1679–1682. doi: 10.1111/j.1528-1157.1999.tb01583.x
Pubmed Abstract | Pubmed Full Text | CrossRef Full Text | Google Scholar
Watts, J. C., Huo, H., Bai, Y., Ehsani, S., Jeon, A. H., Shi, T., et al. (2009). Interactome analyses identify ties of PrP and its mammalian paralogs to oligomannosidic N-glycans and endoplasmic reticulum-derived chaperones. PLoS Pathog. 5:e1000608. doi: 10.1371/annotation/9eb11869-6acb-49b0-978e-abedc3cc545a
Pubmed Abstract | Pubmed Full Text | CrossRef Full Text | Google Scholar
Wong, B. S., Liu, T., Li, R., Pan, T., Petersen, R. B., Smith, M. A., et al. (2001). Increased levels of oxidative stress markers detected in the brains of mice devoid of prion protein. J. Neurochem. 76, 565–572. doi: 10.1046/j.1471-4159.2001.00028.x
Pubmed Abstract | Pubmed Full Text | CrossRef Full Text | Google Scholar
Wopfner, F., Weidenhofer, G., Schneider, R., von Brunn, A., Gilch, S., Schwarz, T. F., et al. (1999). Analysis of 27 mammalian and 9 avian PrPs reveals high conservation of flexible regions of the prion protein. J. Mol. Biol. 289, 1163–1178. doi: 10.1006/jmbi.1999.2831
Pubmed Abstract | Pubmed Full Text | CrossRef Full Text | Google Scholar
Wu, Z., Liang, F., Hong, B., Young, J. C., Sussman, M. R., Harper, J. F., et al. (2002). An endoplasmic reticulum-bound Ca(2+)/Mn(2+) pump, ECA1, supports plant growth and confers tolerance to Mn(2+) stress. Plant Physiol. 130, 128–137. doi: 10.1104/pp.004440
Pubmed Abstract | Pubmed Full Text | CrossRef Full Text | Google Scholar
Yoon, B. S., Ovchinnikov, D. A., Yoshii, I., Mishina, Y., Behringer, R. R., and Lyons, K. M. (2005). Bmpr1a and Bmpr1b have overlapping functions and are essential for chondrogenesis in vivo. Proc. Natl. Acad. Sci. U.S.A. 102, 5062–5067. doi: 10.1073/pnas.0500031102
Pubmed Abstract | Pubmed Full Text | CrossRef Full Text | Google Scholar
Young, R., Passet, B., Vilotte, M., Cribiu, E. P., Béringue, V., Le Provost, F., et al. (2009). The prion or the related Shadoo protein is required for early mouse embryogenesis. FEBS Lett. 583, 3296–3300. doi: 10.1016/j.febslet.2009.09.027
Pubmed Abstract | Pubmed Full Text | CrossRef Full Text | Google Scholar
Yu, G., Chen, J., Xu, Y., Zhu, C., Yu, H., Liu, S., et al. (2009). Generation of goats lacking prion protein. Mol. Reprod. Dev. 76, 3. doi: 10.1002/mrd.20960
Pubmed Abstract | Pubmed Full Text | CrossRef Full Text | Google Scholar
Zhang, C. C., Steele, A. D., Lindquist, S., and Lodish, H. F. (2006). Prion protein is expressed on long-term repopulating hematopoietic stem cells and is important for their self-renewal. Proc. Natl. Acad. Sci. U.S.A. 103, 2184–2189. doi: 10.1073/pnas.0510577103
Pubmed Abstract | Pubmed Full Text | CrossRef Full Text | Google Scholar
Zhang, Y., Kim, S. O., Opsahl-Vital, S., Ho, S. P., Souron, J. B., Kim, C., et al. (2011). Multiple effects of the cellular prion protein on tooth development. Int. J. Dev. Biol. 55, 953–960. doi: 10.1387/ijdb.113348yz
Pubmed Abstract | Pubmed Full Text | CrossRef Full Text | Google Scholar
Keywords: prion protein, development, neural development, stem cells, cell adhesion, extra-cellular matrix, cytoskeleton
Citation: Halliez S, Passet B, Martin-Lannerée S, Hernandez-Rapp J, Laude H, Mouillet-Richard S, Vilotte J-L and Béringue V (2014) To develop with or without the prion protein. Front. Cell Dev. Biol. 2:58. doi: 10.3389/fcell.2014.00058
Received: 28 July 2014; Accepted: 22 September 2014;
Published online: 13 October 2014.
Edited by:
Kim Newton, Genentech, Inc., USAReviewed by:
Peter Christian Kloehn, University College London, UKCopyright © 2014 Halliez, Passet, Martin-Lannerée, Hernandez-Rapp, Laude, Mouillet-Richard, Vilotte and Béringue. This is an open-access article distributed under the terms of the Creative Commons Attribution License (CC BY). The use, distribution or reproduction in other forums is permitted, provided the original author(s) or licensor are credited and that the original publication in this journal is cited, in accordance with accepted academic practice. No use, distribution or reproduction is permitted which does not comply with these terms.
*Correspondence: Sophie Halliez and Vincent Béringue, Institut National de la Recherche Agronomique, U892 Virologie et Immunologie Moléculaires, Equipe MAP2, Bâtiment 440, 78350 Jouy-en-Josas, France e-mail:c2hhbGxpZXpAam91eS5pbnJhLmZy;dmluY2VudC5iZXJpbmd1ZUBqb3V5LmlucmEuZnI=
Disclaimer: All claims expressed in this article are solely those of the authors and do not necessarily represent those of their affiliated organizations, or those of the publisher, the editors and the reviewers. Any product that may be evaluated in this article or claim that may be made by its manufacturer is not guaranteed or endorsed by the publisher.
Research integrity at Frontiers
Learn more about the work of our research integrity team to safeguard the quality of each article we publish.