- Department of Molecular Biotechnology, Faculty of Biology, TU Dresden, Dresden, Germany
Considering the importance of biocatalysis in chemical synthesis, technologies allowing full exploitation of its potential are urgently wanted. Eleven years ago, our team proposed Pickering emulsions as a concept to overcome the severe restrictions set by the general requirement for the presence of water. In this brief perspective, we demonstrate that the insights into bioactive Pickering emulsions gathered meanwhile strongly designate it a key technology to non-aqueous and multi-step biocatalysis. Mainly, this relates to the extensive compatibility of this system with different solvents, materials, biocatalysts, reactions and demands on productive use. We here give a brief overview of the most relevant details, including recent results from our own research.
Introduction
Within the last 20 years, biocatalysis has become an important tool for chemical synthesis accomplishing production of intermediates, specialty and fine chemicals, pharmaceutics and even commodities (Woodley, 2022). Its full potential, however, is still far from exploitation, inter alia because of the prevalent requirement for aqueous media that disagrees with the hydrophobicity of most organic compounds or the water-sensitivity of many compounds and reactions (Hollmann and Fernandez-Lafuente, 2021). Consequently, implementation of biocatalysis in multi-step synthetic pathways is also hardly possible as these mostly employ organic solvents to improve solubility, miscibility or stability of reagents, act as thermal buffers for exothermic reactions, or condition (chemo-)catalyst efficiency and concentration (Pera-Titus et al., 2015). The obvious solution to overcome these difficulties is the introduction of biphasic systems, in which biocatalysis takes place in an aqueous surrounding, while reaction educts and products are concentrated in a non-miscible solvent. Accordingly, various technical concepts for practical realization of biphasic biocatalysis emerged over the years, including free, mechanically mixed phases, gel-stabilized water phases suspended in liquid solvent, and surfactant-stabilized reverse micelles or micro-emulsions. However, negative effects on biocatalyst stability, mass transfer or, in case of micro-emulsions and micelles, downstream processing prevent broad application (Ansorge-Schumacher, 2008; Bago Rodriguez and Binks, 2020).
Eleven years ago, our group first introduced the use of Pickering emulsions (PE) for multi-phase biocatalysis demonstrating considerable improvement of the catalytic activity of two lipases, CalA and CalB, from Pseudozyma aphidis (formerly Candida antarctica) and benzaldehyde lyase (BAL) from Pseudomonas fluorescence Biovar I (Wu et al., 2011). The latter was particularly remarkable since BAL is a very vulnerable enzyme that had never before exhibited satisfying activity in the presence of organic solvents. Meanwhile, an increasing number of researchers worldwide have reported successful biphasic biocatalysis with PE; in parallel, PE also have been employed for stoichiometric chemical synthesis and chemo-catalysis (Pera-Titus et al., 2015; Bago Rodriguez and Binks, 2020). On this background and in view of our own advancing insights into the system, we have come to the perspective that PE provide the future key technology to non-aqueous biocatalysis and its implementation in multi-step reactions.
Biocatalytic active pickering emulsions
PE are liquid biphasic systems, in which solid or colloidal micro- or nanoparticles stabilize the dispersed phase in small, stable droplets (Figure 1). Thus, they continuously provide broad contact between phases for a fast exchange of reactants rendering vigorous stirring or solidification of the dispersed phase unnecessary. At the same time, they keep away vulnerable compounds from a detrimental surrounding. Distinct from emulsifying surfactants, stabilizing particles do not spontaneously detach from the interface due to an exceedingly large energy barrier (Binks, 2002; Aveyard et al., 2003) and thus can obviate product spoiling with free stabilizers as recurrent with surfactant-induced emulsions (Palmer and Hartley, 2018).
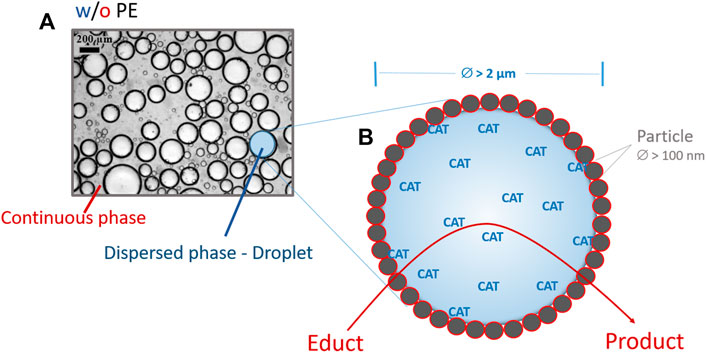
FIGURE 1. Pickering emulsion (PE) of the water-in-oil type (w/o). (A) Microscopic picture of a buffered aqueous phase (50 mmol⋅L−1 KPi, pH 7.0) in cyclopentyl methyl ether at a volume phase ratio of 1:10 containing 3% (weight per volume of dispersed phase) silanized silica particles. (B) Schematic illustration of an aqueous droplet stabilized by solid particles and harboring a biocatalyst (CAT). Particle and droplet sizes denote typical values (Chevalier and Bolzinger, 2013).
PE used for biocatalysis (BioPE) normally are of the water-in-oil type (w/o), i.e. the aqueous phase forms the dispersed phase; nevertheless, oil-in-water (o/w) PE can also be employed (Yu et al., 2019). The biocatalysts are located in the aqueous phase or at the water-in-oil interface. Whether a system forms a w/o PE or an o/w PE mainly depends on the partial wettability of the stabilizing particles by water and oil, and thus on the hydrophilicity/hydrophobicity of their surfaces (Binks and Lumsdon, 2000b). Generally, w/o PE form with more hydrophobic, o/w PE with more hydrophilic particles. The particle surfaces together with the phase composition also influence the emulsion stability over time in terms of resistance against coalescence of the dispersed droplets. The droplet diameter predominantly determines the actual performance of a biocatalytic active w/o PE (Pera-Titus et al., 2015; Bago Rodriguez and Binks, 2020). Small droplet diameters ensure a large interface and thus high reaction efficiency due to appropriate mass exchange between phases. The initial droplet size depends on the hydrodynamic conditions during PE formation, and overall on the PE composition, in particular the solvent ratios and the particle size, type and amount (Tcholakova et al., 2008). Small droplet diameters arise from high dispersion energy (Binks and Lumsdon, 2000a; Kempin et al., 2020), a small volume fraction of the dispersed phase (Binks and Lumsdon, 2000a), and a high content of stabilizing particles with small sizes and rough surfaces (Li et al., 2020).
Continuous and dispersed phases
In principal, almost any liquid fluid with low water miscibility is suitable for the formation of BioPE. We were able to demonstrate this in a small study on the performance of 18 organic solvents from various structural classes and with designated importance to biocatalyzed reactions (Supplementary Figure S1, Supplementary Material). In a standard mixture of 10% (v/v) buffered aqueous phase (50 mmol⋅L−1 KPi, pH 7.0) in solvent and 3% (weight per volume of dispersed phase; w/vdp) of surface-modified silica particles, formation of w/o emulsions was clearly visible under the microscope with almost all solvents. An exception was only diethyl ether, which evaporated too fast under the microscope to verify emulsion formation. The initial sizes of stabilized droplets and their size distributions varied with the solvent. Most of the obtained PE were reasonably stable over more than 10 days, i.e. droplets coalesced only slightly. The best results were obtained with toluene, 2-methyltetrahydrofuran (2-MeTHF) and cyclopentyl methyl ether (CPME). Noteworthy, the latter two represent environmentally more benign “green” solvents, and of late have been used increasingly for biocatalysis exhibiting broad compatibility with both biocatalysts and reactants (Alcantára and Dominguez de María, 2018; Petrenz et al., 2015; Röllig et al., 2019).
Dispersed phases for w/o BioPE usually comprise salt-buffered aqueous solutions. To our current knowledge, the type of the employed buffer salt or pH hardly influence emulsion formation or stability. A high salt concentration, however, might influence the surface tension and thus particle binding at the interface (Binks and Lumsdon, 2000b). For better handling or restriction of diffusion within the droplets, jellification of the dispersed phase, e.g. with agarose is possible (Wu et al., 2011). On the other hand, almost water-free PE can be obtained from the use of solvent-immiscible ionic liquids as the dispersed phase accommodating the biocatalyst (Zhang et al., 2017). Our own research recently showed that environmentally more benign and easy-to-prepare deep eutectic solvents (DES) could serve the same purpose. Tentatively, we obtained stable BioPE with typical droplet sizes using 5% (v/v) choline chloride:glycerine and choline chloride:urea, respectively, as the dispersed and toluene as continuous phase (Supplementary Figure S2, Supplementary Material).
Particle choice and biocatalyst location
Currently, stabilization of BioPE mostly employs solid or porous silica-based particles, which are surface-modified to obtain the necessary hydrophobicity for the stabilization of w/o emulsions (Bago Rodriguez and Binks, 2020). Suitable solid particles in different sizes are commercially available or easily produced via Stöber synthesis (Stöber et al., 1968). Covalent grafting with an appropriately hydrophobic organosilane such as trimethoxy (octadecyl)silane (TMODS) (Wu et al., 2011), dichlorodimethylsilane or hexamethyldisilazane (Chevalier and Bolzinger, 2013) adjusts the surface hydrophobicity to the desired value. However, hydrophobic colloidal silica particles (Heyse et al., 2019) or other hydrophobic solid materials such as zeolites (Zapata et al., 2012), polymerosomes based on amphiphilic block co-polymers (Wang et al., 2012) or even soft gel particles (Wiese et al., 2013) also can successfully stabilize BioPE, provided that an appropriate, stable contact with the interface can be made (Binks and Lumsdon, 2000b). In our lab, we have invented a method for the partial coating of micron-sized particles with silicone that allows employment of a broad variety of inorganic or organic materials for PE stabilization (Ansorge-Schumacher and Plikat, 2017). Here, the choice of building blocks varies hydrophobicity enabling adaption to both phase composition and material. Applying this method, we even were able to employ whole cells of Escherichia coli efficiently as stabilizing particles (Röllig et al., 2019).
In principal, biocatalysts in PE are either dispersed in the aqueous phase or bound to the stabilizing particles, thus conducting Pickering Assisted Catalysis (PAC) or Pickering Interfacial Catalysis (PIC), respectively (Pera-Titus et al., 2015). At present, dispersion is most common (Bago Rodriguez and Binks, 2020), but our recent studies strongly point at particle binding being the preferable strategy (Plikat et al., 2022). We observed that lipases dissolved in the aqueous phase had severe effects on both droplet size and emulsion stability, which we accounted to the almost quantitative intercalation of the enzymes between particles at the interface. With increasing enzyme concentration, the droplet sizes, but also the emulsion stability decreased considerably. The exact extent of the effects depended on the individual enzymes, but coalescence always became critical already at rather low protein loading. Thus, the scope to optimize the productivity in BioPE with dissolved enzymes appears rather limited. Putting the enzymes on - or rather in - the stabilizing particles will probably overcome the obstacle and in addition, benefit from the general advantages of performing PIC, such as even smaller mass transfer restrictions (Ni et al., 2022). Results from BioPE using lipase in polymerosomes or adsorbed to the silica particles support this view (Pera-Titus et al., 2015; Meng et al., 2019; Wang et al., 2019; Sun et al., 2020). However, considering the usually strong impact of supporting materials on enzyme activity (Ansorge-Schumacher, 2008), the approach would considerably profit from extending the choice of particles currently in use.
Biocatalysts and reactions
The choice of enzymes employed for biocatalysis in PE presently focuses on lipases [EC 3.1.1.x] as versatile and robust biocatalysts for ester hydrolysis, esterification and transesterification reactions (Pera-Titus et al., 2015; Bago Rodriguez and Binks, 2020). Only few reports involve other hydrolases (Xue et al., 2017) or enzymes from other classes (Bago Rodriguez et al., 2020), including our own work on the application of BAL [EC 4.1.2.38] for stereo-selective carboligations (Wu et al., 2011; Röllig et al., 2019). However, we recently extended the application to alcohol dehydrogenases [ADH; EC 1.1.1.x] and aminotransferases [ATA; EC 2.1.6.x] successfully and for the first time demonstrated enzyme-mediated multi-step catalysis with PE in one-pot and in a cascade (Figure 2).
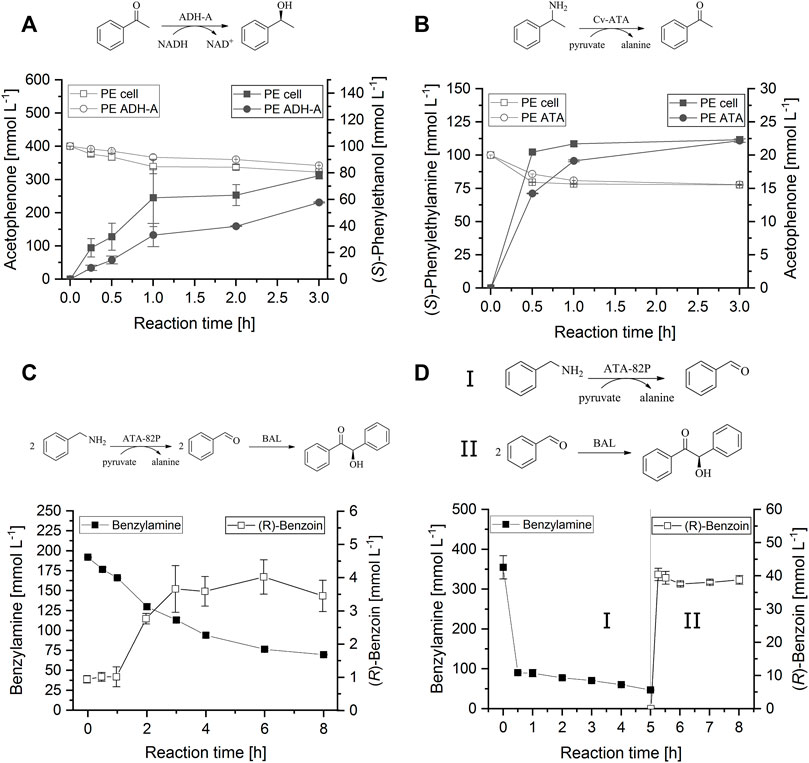
FIGURE 2. Single- and multi-step reactions in w/o PE catalyzed by ADH-A from R. rhodocrous (A), Cv-ATA from C. violaceum (B) and a combination of commercial ATA-82P (c-Lecta, Germany) and BAL from Pseudomonas fluorescens Biovar I (C,D). (A) PE consisting of HEPES buffer in CPME at a volume phase ratio of 1:5 containing 6% (w/vdp) silica particles. Reduction of acetophenone (empty symbols) to (S)-1-phenylethanol (filled symbols) with NADH and iso-propanol. (B) PE consisting of HEPES buffer in CPME at a volume phase ratio of 1:5 containing 6% (w/vdp) silica particles. Deamination of rac-1-phenylethylamine (empty symbols) to acetophenone (filled symbols) using pyruvate as co-substrate. (C) PE consisting of TEA buffer in CPME at a volume phase ratio of 1:3 containing 9% (w/vdp) silica particles. Deamination of benzylamine with pyruvate as co-substrate, and carboligation of benzaldehyde to (R)-benzoin in one-pot over time. (D) PE consisting of TEA buffer in CPME at a volume phase ratio of 1:2 with ATA-82P and 1:5 with BAL containing 36% (ATA-82P) or 9% (BAL) (w/vdp) silica particles. Deamination of benzylamine with pyruvate as co-substrate (I), and carboligation of benzaldehyde to (R)-benzoin (II) in separate vessels. Between the enzyme-catalyzed steps, N-benzyl-1-phenyl-methaneimine (NBPMI) was hydrolyzed with HCl. All experiments were performed in triplicate. Detailed experimental information is given in the Supplementary.
In our experiments, both the ADH-A from Rhodococcus ruber and the ω-transaminase from Chromobacterium violaceum (Cv-ATA) showed notable catalytic activity for ketone reduction and deamination, respectively, either as isolated enzymes or within lyophilized whole cells of recombinant E. coli in the dispersed phase of BioPE (Figures 2A,B, respectively). Likewise, a set of commercial ADH-preparations with a lower solvent-stability than ADH-A were active (Supplementary Table S1, Supplementary Material). All ADHs accomplished regeneration of the co-compartmentalized cofactor nicotinamide adenine dinucleotide (NADH) effectively within the BioPE using iso-propanol as co-substrate. Without extensive optimization of the reaction systems, isolated ADH-A and ADH-A in whole cells yielded about 2.4 g⋅L−1⋅h−1 and 3.2 g⋅L−1⋅h−1 (S)-phenylethanol, respectively, over a reaction time of 3 h. The corresponding space-time-yields for acetophenone production with isolated Cv-ATA or Cv-ATA in whole cells were 0.9 g⋅L−1⋅h−1 each.
Combination of the commercial transaminase ATA-82P (c-LEcta, Germany) and BAL in the aqueous phase of a BioPE successfully converted benzylamine to (R)-benzoin in one-pot (Figure 2C). Both enzymes showed good activities over a reaction time of at least 8 h. Unfortunately, an unexpectedly strong autocatalytic formation of N-benzyl-1-phenylmethanimine (NBPMI) from benzylamine and the intermediate benzaldehyde occurred simultaneously, which limited the availability of benzaldehyde as a substrate for BAL to a maximum of 9.6 mmol⋅L−1. Thus, the overall yield of (R)-benzoin in this system was only 0.8 g⋅L−1⋅h−1. However, we were able to increase the space-time-yield to 2.7 g⋅L−1⋅h−1 (R)-benzoin by transferring the reaction into a multi-pot cascade consisting of two separate, slightly optimized BioPE with ATA-82P and BAL, respectively, and an intermediate acid-catalyzed hydrolysis of NBPMI into benzaldehyde and benzylamine (Figure 2D).
Reaction control
Most reactions in BioPE are performed in a batch or repeated batch mode (Bago Rodriguez and Binks, 2020), and in the beginning, the tedious and potentially damaging steps for breaking the emulsion between reaction cycles (e.g., centrifugation) were regarded as a major obstacle to enzyme recycling and thus, economic synthetic use. Meanwhile, however, the development of PE, where particles desorb on demand upon an external stimulus like pH or temperature (Bago Rodriguez and Binks, 2020), and in particular, the introduction of continuous flow systems provide promising options for productive application. Owing to the high droplet stability, PE can form a dense packed-bed in a column reactor (Zhang et al., 2016), where reactants and products can be fed or extracted, respectively, with the continuous phase at a constant flow. With standard PE, the hydraulic residence times in these systems are overall high, because the small droplet sizes incur high pressure losses while at the same time the droplet vulnerability to compaction and coalescence allows only small pressure. However, cross-linking of particles at the interface has been described to considerably improve the performance (Zhang et al., 2019). In parallel, in collaboration with partners at HTW Berlin (Germany), we demonstrated membrane reactors for continuous ultrafiltration as a promising alternative (Heyse et al., 2018). These use a stirred vessel as the reaction chamber and can achieve industrially relevant residence times without modification of the PE. Thus, a technical basis for the use of BioPE and its application for multiple consecutive reactions, as tentatively examined in the previous section, is at hand.
Conclusion and outlook
Our condensed overview of the state-of-the-art and latest developments in the investigation of PE for biocatalytic use illustrates the enormous versatility of this system in terms of compatibility with different solvents, materials, biocatalysts, reactions and demands on productive application. In principal, a strategic fit to almost any synthetic problem seems plausible, while the advantages over alternative biphasic systems are immanent. The possible implementation of green solvents or hydrophobic liquid substrates as alternative organic phases and the general efficiency of small continuous systems also provide promising credentials for sustainable green chemistry. All this strongly designates BioPE a key technology to non-aqueous and multi-step biocatalysis and thus, a key to better exploit the potential of biocatalysis. Of course, with the many interacting solid and liquid, organic and inorganic, biological, and chemical components involved, considerable further research and development will be required to fully understand the system and achieve industrial implementation. Major efforts will probably lie with the design of materials to match both technical requirements and requirements of the individual biocatalysts, design of the biocatalysts to remain active and selective, design of the phase system to distribute reactants appropriately, and design of reaction control to cope with the special features of PE such as particle release in the presence of proteins (Heyse et al., 2018). Since all the aspects strongly interfere, approaches should at best be interdisciplinary, involving molecular biologists, chemists, and engineers. In our perspective, this will be very much worthwhile.
Data availability statement
The raw data supporting the conclusion of this article will be made available by the authors, without undue reservation.
Author contributions
All authors listed have made a substantial, direct, and intellectual contribution to the work and approved it for publication.
Acknowledgments
MA-S and CP acknowledge funding from the German Federal Ministry of Education and Research (BMBF) within project BioPick (grant number 031A163B). MAS also acknowledges funding from the German Research Foundation (DFG) within project BioPOW (project number 469188416).
Conflict of interest
The authors declare that the research was conducted in the absence of any commercial or financial relationships that could be construed as a potential conflict of interest.
Publisher’s note
All claims expressed in this article are solely those of the authors and do not necessarily represent those of their affiliated organizations, or those of the publisher, the editors and the reviewers. Any product that may be evaluated in this article, or claim that may be made by its manufacturer, is not guaranteed or endorsed by the publisher.
Supplementary material
The Supplementary Material for this article can be found online at: https://www.frontiersin.org/articles/10.3389/fctls.2022.1032088/full#supplementary-material
References
Alcantara, A. R., and de Maria, P. (2018). Recent advances on the use of 2-methyltetrahydrofuran (2-MeTHF) in biotransformations. Cgc 5, 86–103. doi:10.2174/2213346105666180727100924
Ansorge-Schumacher, M. B. (2008). “Immobilisation of biological catalysts,”. Editors K Ertl, and W Schüth (Wiley VCH Weinheim), China 1, 644–655. 978-3-527-31241-2.Handb. Heterogeneous Catal.
Aveyard, R., Binks, B. P., and Clint, J. H. (2003). Emulsions stabilised solely by colloidal particles. Adv. Colloid Interface Sci. 100-102, 503–546. doi:10.1016/S0001-8686(02)00069-6
Bago Rodriguez, A. M., Schober, L., Hinzmann, A., Gröger, H., and Binks, B. P. (2020). Effect of particle wettability and particle concentration on the enzymatic dehydration of n ‐octanaloxime in pickering emulsions. Angew. Chem. Int. Ed. 60, 1450–1457. doi:10.1002/anie.202013171
Binks, B. P., and Lumsdon, S. O. (2000a). Catastrophic phase inversion of water-in-oil emulsions stabilized by hydrophobic silica. Langmuir 16, 2539–2547. doi:10.1021/la991081j
Binks, B. P., and Lumsdon, S. O. (2000b). Influence of particle wettability on the type and stability of surfactant-free emulsions. Langmuir 16, 8622–8631. doi:10.1021/la000189s
Binks, B. P. (2002). Particles as surfactants-similarities and differences. Curr. Opin. Colloid & Interface Sci. 7, 21–41. doi:10.1016/S1359-0294(02)00008-0
Chevalier, Y., and Bolzinger, M.-A. (2013). Emulsions stabilized with solid nanoparticles: Pickering emulsions. Colloids Surfaces A Physicochem. Eng. Aspects 439, 23–34. doi:10.1016/j.colsurfa.2013.02.054
Heyse, A., Plikat, C., Grün, M., Delaval, S., Ansorge-Schumacher, M. B., and Drews, A. (2018). Impact of enzyme properties on drop size distribution and filtration of water-in-oil Pickering emulsions for application in continuous biocatalysis. Process Biochem. 72, 86–95. doi:10.1016/j.procbio.2018.06.018
Hollmann, F., and Fernandez‐Lafuente, R. (2021). Grand challenges in biocatalysis. Front. Catal. 1, 633893. doi:10.3389/fctls.2021.633893
Kempin, M. V., Kraume, M., and Drews, A. (2020). W/O Pickering emulsion preparation using a batch rotor-stator mixer - influence on rheology, drop size distribution and filtration behavior. J. Colloid Interface Sci. 573, 135–149. doi:10.1016/j.jcis.2020.03.103
Li, Y., Zhao, G., Hong, B., Zhao, S., Han, X., and Pera-Titus, M. (2020). Unraveling particle size and roughness effects on the interfacial catalytic properties of pickering emulsions. Colloids Surfaces A Physicochem. Eng. Aspects 599, 124800. doi:10.1016/j.colsurfa.2020.124800
Meng, T., Bai, R., Wang, W., Yang, X., Guo, T., and Wang, Y. (2019). Enzyme-loaded mesoporous silica particles with tuning wettability as a Pickering catalyst for enhancing biocatalysis. Catalysts 9, 78. doi:10.3390/catal9010078
Ni, L., Yu, C., Wei, Q., Liu, D., and Qiu, J. (2022). Pickering emulsion catalysis: Interfacial chemistry, catalyst design, challenges, and perspectives. Angew. Chem. Int. Ed. 61, e202115885. doi:10.1002/anie.202115885
Palmer, M., and Hatley, H. (2018). The role of surfactants in wastewater treatment: Impact, removal and future techniques: A critical review. Water Res. 147, 60–72. doi:10.1016/j.watres.2018.09.039
Pera-Titus, M., Leclercq, L., Clacens, J.-M., De Campo, F., and Nardello-Rataj, V. (2015). Pickering interfacial catalysis for biphasic systems: From emulsion design to green reactions. Angew. Chem. Int. Ed. 54, 2006–2021. doi:10.1002/anie.201402069
Petrenz, A., María, P., Ramanathan, A., Hanefeld, U., Ansorge-Schumacher, M. B., and Kara, S. (2015). Medium and reaction engineering for the establishment of a chemo-enzymatic dynamic kinetic resolution of rac-benzoin in batch and continuous mode. J. Mol. Catal. B Enzym. 114, 42–49. doi:10.1016/j.molcatb.2014.10.011
Rodriguez, A. M., and Binks, B. P. (2020). Catalysis in pickering emulsions. Soft Matter 16, 10221–10243. doi:10.1039/d0sm01636e
Röllig, R., Plikat, C., and Ansorge‐Schumacher, M. B. (2019). Efficient and selective carboligation with whole‐cell biocatalysts in pickering emulsion. Angew. Chem. Int. Ed. 58, 12960–12963. doi:10.1002/anie.201907209
Stöber, W., Fink, A., and Bohn, E. (1968). Controlled growth of monodisperse silica spheres in the micron size range. J. Colloid Interface Sci. 26, 62–69. doi:10.1016/0021-9797(68)90272-5
Sun, Z., Cai, M., Hübner, R., Ansorge‐Schumacher, M. B., and Wu, C. (2020). Tailoring particle‐enzyme nanoconjugates for biocatalysis at the organic‐organic interface. ChemSusChem 13, 6523–6527. doi:10.1002/cssc.202002121
Tcholakova, S., Denkov, N. D., and Lips, A. (2008). Comparison of solid particles, globular proteins and surfactants as emulsifiers. Phys. Chem. Chem. Phys. 10, 1608–1627. doi:10.1039/B715933C
Wang, L., Liu, X., Jiang, Y., Liu, P., Zhou, L., Ma, L., et al. (2019). Silica nanoflowers-stabilized Pickering emulsion as a robust biocatalysis platform for enzymatic production of biodiesel. Catalysts 9, 1026. doi:10.3390/catal9121026
Wang, Z., van Oers, M. C. M., Rutjes, F. P. J. T., and van Hest, J. C. M. (2012). Polymersome colloidosomes for enzyme catalysis in a biphasic system. Angew. Chem. Int. Ed. 51, 10746–10750. doi:10.1002/anie.201206555
Wiese, S., Spiess, A. C., and Richtering, W. (2013). Microgel-stabilized smart emulsions for biocatalysis. Angew. Chem. Int. Ed. 52, 576–579. doi:10.1002/anie.201206931
Wu, C., Bai, S., Ansorge-Schumacher, M. B., and Wang, D. (2011). Nanoparticle cages for enzyme catalysis in organic media. Adv. Mat. 23, 5694–5699. doi:10.1002/adma20110269310.1002/adma.201102693
Xue, L.-H., Xie, C.-Y., Meng, S.-X., Bai, R.-X., Yang, X., Wang, Y., et al. (2017). Polymer-protein conjugate particles with biocatalytic activity for stabilization of water-in-water emulsions. ACS Macro Lett. 6, 679–683. doi:10.1021/acsmacrolett.7b00294
Yu, S., Zhang, D., Jiang, J., Cui, Z., Xia, W., Binks, B. P., et al. (2019). Biphasic biocatalysis using a CO2-switchable Pickering emulsion. Green Chem. 21, 4062–4068. doi:10.1039/c8gc03879a
Zapata, P. A., Faria, J., Ruiz, m. P., Jentoft, R. E., and Resasco, D. E. (2012). Hydrophobic zeolites for biofuel upgrading reactions at the liquid-liquid interface in water/oil emulsions. J. Am. Chem. Soc. 134, 8570–8578. doi:10.1021/ja3015082
Zhang, M., Ettelaie, R., Yan, T., Zhang, S., Cheng, F., Binks, B. P., et al. (2017). Ionic liquid droplet microreactor for catalysis reactions not at equilibrium. J. Am. Chem. Soc. 139, 17387–17396. doi:10.1021/jacs.7b07731
Zhang, M., Hou, Y., Ettelaie, R., Guan, R., Zhang, M., Zhang, Y., et al. (2019). Pickering emulsion-derived liquid-solid hybrid catalyst for bridging homogeneous and heterogeneous catalysis. J. Am. Chem. Soc. 141, 5220–5230. doi:10.1021/jacs.8b11860
Keywords: multi-phase biocatalysis, multi-step biocatalysis, Pickering emulsion, phase composition, materials, particle choice, enzyme, reaction control
Citation: Ansorge-Schumacher MB and Plikat C (2022) Key technology to non-aqueous and multi-step biocatalysis: Pickering emulsions. Front. Catal. 2:1032088. doi: 10.3389/fctls.2022.1032088
Received: 30 August 2022; Accepted: 27 September 2022;
Published: 14 October 2022.
Edited by:
Andrés R. Alcántara, Complutense University of Madrid, SpainReviewed by:
Davide Tessaro, Politecnico di Milano, ItalyCopyright © 2022 Ansorge-Schumacher and Plikat. This is an open-access article distributed under the terms of the Creative Commons Attribution License (CC BY). The use, distribution or reproduction in other forums is permitted, provided the original author(s) and the copyright owner(s) are credited and that the original publication in this journal is cited, in accordance with accepted academic practice. No use, distribution or reproduction is permitted which does not comply with these terms.
*Correspondence: Marion B. Ansorge-Schumacher, marion.ansorge@tu-dresden.de