- Department of Solar Materials, Helmholtz-Centre for Environmental Research–UFZ, Leipzig, Germany
6-hydroxyhexanoic acid (6HA) represents a polymer building block for the biodegradable polymer polycaprolactone. Alternatively to energy- and emission-intensive multistep chemical synthesis, it can be synthesized directly from cyclohexane in one step by recombinant Pseudomonas taiwanensis harboring a 4-step enzymatic cascade without the accumulation of any intermediate. In the present work, we performed a physiological characterization of this strain in different growth media and evaluated the resulting whole-cell activities. RB and M9* media led to reduced gluconate accumulation from glucose compared to M9 medium and allowed specific activities up to 37.5 ± 0.4 U gCDW−1 for 6HA synthesis. However, 50% of the specific activity was lost within 1 h in metabolically active resting cells, specifying growing cells, or induced resting cells as favored options for long-term biotransformation. Furthermore, the whole-cell biocatalyst was evaluated in a stirred-tank bioreactor setup with a continuous cyclohexane supply via the gas phase. At cyclohexane feed rates of 0.276 and 1.626 mmol min−1 L−1, whole-cell biotransformation occurred at first-order and zero-order rates, respectively. A final 6HA concentration of 25 mM (3.3 g L−1) and a specific product yield of 0.4 g gCDW−1 were achieved with the higher feed rate. Product inhibition and substrate toxification were identified as critical factors limiting biocatalytic performance. Future research efforts on these factors and the precise adjustment of the cyclohexane feed combined with an in situ product removal strategy are discussed as promising strategies to enhance biocatalyst durability and product titer and thus to enable the development of a sustainable multistep whole-cell process.
Introduction
Biodegradable polymers such as polycaprolactone (PCL), polyhydroxyalkanoates, and polylactic acid are gaining interest due to the high ecological footprint of nondegradable plastics. PCL polymerization typically is accomplished either via ring-opening polymerization of ε-caprolactone (ε-CL) or via polycondensation of 6-hydroxyhexanoic acid (6HA) (Labet and Thielemans, 2009). However, the conventional monomer production processes starting from cyclohexane are associated with multiple reaction steps involving the isolation of intermediates, high temperature and pressure variations, and usage of toxic and explosive chemicals (Wittcoff et al., 2012). Monomer production in “one pot” under mild conditions, for example, in a bioprocess, would relieve such critical aspects. In this context, the production of ε-CL and 6HA from cyclohexanol with recombinant E. coli has been reported (Srinivasamurthy et al., 2019; Srinivasamurthy et al., 2020). In our previous work, a 4-step enzymatic cascade for the conversion of cyclohexane via cyclohexanol, cyclohexanone, and ε-caprolactone to 6-hydroxyhexanoic acid has been rationally engineered in P. taiwanensis VLB120 (Schäfer et al., 2020a). A high overall specific activity of 44 U gCDW−1 and complete conversion of 5 mM cyclohexane to 6-hydroxyhexanoic acid have been achieved (Schäfer et al., 2020b). This study now reports on the evaluation of this promising strain regarding its performance under process conditions, including respective biochemical engineering.
The catalytic performance of microorganisms depends on the production of functional enzymes and relies on the cellular machinery fueling enzyme synthesis and the reaction itself with energy and reduction equivalents. Specifically, heterologous pathways involving oxidoreductases typically are connected to cellular metabolism via co-substrates in the form of redox cofactors. The metabolic activity necessary to provide energy and redox equivalents is closely linked to the physiological state of cells and thus cultivation conditions. The use of growing cells, on the one hand, enables efficient (re)-synthesis of enzymes and energy carriers and therefore can convey superior biocatalyst stability but, on the other hand, can suffer from a trade-off between demands for biomass synthesis and redox biocatalysis (Peralta-Yahya et al., 2012). The use of metabolically active but nongrowing (resting) cells, a condition induced by the lack or depletion of, for example, nitrogen or magnesium sources in the medium (Harder and Dijkhuizen, 1983), has resulted in superior whole-cell activities for redox biocatalysis compared to growing cells (Bühler et al., 2008; Jelling et al., 2012). Depending on the reaction(s) to be catalyzed, resting cells can be easier to handle and reusable but often suffer from poor stability (Julsing et al., 2012; Zhang et al., 2012). Recently, mixed-species concepts have been used to produce the monomer adipic acid, 6-aminohexanoic acid, or 1,6-hexanediol from cyclohexane (Wang et al., 2020; Zhang et al., 2020; Bretschneider et al., 2021).
A major challenge regarding the biocatalytic cyclohexane conversion is related to the physiochemical properties of the substrate. With its high vapor pressure (77 mm Hg) and low water solubility (55 mg L−1, 650 µM), cyclohexane constitutes a highly volatile compound under standard conditions (Henry’s law constant Kair/water = 0.15 atm m3 mol−1) (ChemSpider, 2020). Such toxicity issues can be avoided via substrate feeding strategies. A non–water-miscible second organic phase can act as a substrate reservoir, continuously supplying the substrate to appropriate aqueous concentrations (Lilly, 1982; Van Sonsbeek et al., 1993). This two-liquid phase concept has been successfully applied for styrene epoxidation (Volmer et al., 2019), toluene hydroxylation (Collins et al., 1995; Willrodt et al., 2015), and multistep pseudocomene oxidation (Bühler et al., 2003). Alternatively, the substrate can be fed in liquid form via a pump (Srinivasamurthy et al., 2019) or via the gas phase (Karande et al., 2016). Moreover, some bacterial strains are known to feature adaptive solvent tolerance mechanisms involving, for example, solvent efflux systems and/or the alteration of membrane fluidity and surface properties, which qualify them as promising hosts for applications involving toxic reactants and products (Inoue and Horikoshi, 1989; Heipieper et al., 2007). P. taiwanensis VLB120 exhibits such features, primarily encoded on its megaplasmid (Köhler et al., 2013).
The goal of this study was to evaluate the bioconversion of cyclohexane to 6-hydroxyhexanoic acid on a bioreactor scale, with recombinant P. taiwanensis_6HA as the biocatalyst (Schäfer et al., 2020a). Its biocatalytic performance was investigated in growing and resting cell formats (Figure 1). Furthermore, the catalytic potential of resting cells was evaluated in bioreactor setups with cyclohexane being fed via the gas phase.
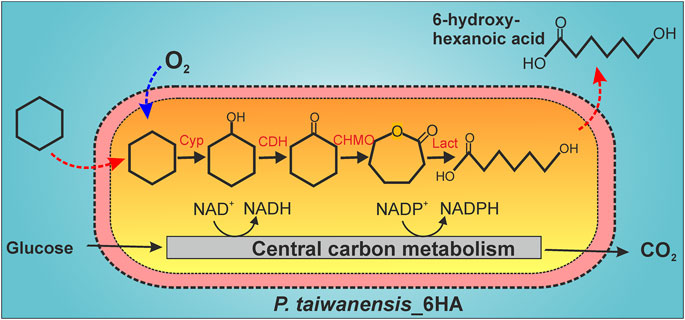
FIGURE 1. Biocatalytic cascade for 6-hydroxyhexanoic acid (6HA) synthesis from cyclohexane with P. taiwanensis_6HA. Cyclohexane is successively converted to cyclohexanol, cyclohexanone, ε-caprolactone, and 6HA by a cytochrome P450 monooxygenase (Cyp), a cyclohexanol dehydrogenase (CDH), a cyclohexanone monooxygenase (CHMO), and a lactonase (Lact), respectively. Cyclohexane is a small (84 Da) and hydrophobic molecule and can rapidly pass the outer and inner membranes of P. taiwanensis (Chen, 2007; Nikaido, 2003). The mode of 6HA export is unknown but possibly involves active transport due to its charged character at pH 7.2.
Materials and Methods
Bacterial Strains, Plasmids, Media, and Chemicals
The microbial strains and plasmids used in this work are listed in Supplementary Table S1. Cells were grown in lysogeny broth (LB), M9 (Sambrook and Russell, 2001), M9* (Panke et al., 1999), or RB medium (Riesenberg et al., 1991; Volmer et al., 2019) at a pH of 7.2 (adjusted with 10 M NaOH) and supplemented with 0.5% (w/v) glucose as the sole carbon and energy source. Kanamycin (50 μg mL−1) was applied for selection when necessary. Unless stated otherwise, all chemicals were purchased from Sigma-Aldrich (Steinheim, Germany) or Carl Roth (Karlsruhe, Germany) in the highest purity available and used without further purification. 6HA was acquired from abcr (Karlsruhe, Germany). Molecular biology methods and plasmid construction are explained in detail in Supplementary Materials.
Growth of Bacterial Cultures
Cultivations were carried out at 30°C and 200 rpm in a Multitron shaker (Infors, Bottmingen, Switzerland). Pre-cultivation generally was started with an LB culture (∼20 h), from which a minimal medium pre-culture (1% v/v) was inoculated and incubated for another 20 h. The latter culture was used to inoculate the main culture to an optical density at 450 nm (OD450) of 0.2. Heterologous gene expression was induced with 1 mM isopropyl β-d-1-thiogalactopyranoside (IPTG) when the cultures reached an OD450 of ∼0.5. Incubation was continued for maximally 7.5 h.
Evaluation of Induction Kinetics
Main cultures were harvested after induction in time intervals of 90 min by centrifugation (RT, 5,000 g, 10 min) and resuspended in 10 mL potassium phosphate buffer containing 1% (w/v) glucose (Kpi-g) to a final cell concentration of 0.5 gCDW L−1. The cell suspension was transferred to 100 mL baffled screw-capped shake flasks and equilibrated for 10 min at 30°C and 250 rpm. Biotransformations were started by adding 5.46 µL pure cyclohexane (it corresponds to 5 mM with respect to the aqueous phase volume) to the flask, which was then tightly closed. The assay was stopped after 10 min by pipetting 1 mL of the sample either into 1 M HCl (100 µL) to reach a pH of 3, followed by centrifugation and storage at −20°C for HPLC analysis, or into 0.5 mL ice-cold diethyl ether containing 0.2 mM n-decane as an internal standard. The latter sample was extracted via 2 min of vortexing. After short centrifugation, the organic phase was dried over water-free Na2SO4 and transferred to a GC vial for analysis.
Long-Term Biotransformations on Shake Flask Scale
Cells were harvested via centrifugation (10 min, RT, 5,000 g) after 6 h of induction in RB- or M9*–shake flask cultures and resuspended to a final biomass concentration of 0.5 gCDW L−1 in 20 ml of either Kpi-g, Kpi-g containing 1 mM IPTG (Kpi-g-I), or the respective growth medium containing 1 mM IPTG. After equilibration in 100 ml baffled and screw-capped shake flasks, 10.9 µL cyclohexane (5 mM referring to aqueous phase volume) was added to start the reaction. Sampling of ca. 2.1 mL liquid volume was carried out by using a syringe through a silicone/PTFE septum after 10, 60, 110, and 160 min reaction time. One mL each was extracted with 0.5 mL diethyl ether or diluted with 1 M HCL (100 µL for Kpi and RB medium and 200 µL for M9* medium) for GC and HPLC analysis, respectively, as described above. Additionally, OD450 was determined, and 100 µL gas samples were taken with a gas-tight glass syringe (Hamilton, Reno, NV).
Toxicity Assay
Cyclohexane toxicity was evaluated based on its influence on the growth rate of P. taiwanensis VLB120. Cells were precultivated as described above and inoculated in M9* main cultures to an OD450 of 0.1. Different cyclohexane amounts were added after 4 h of cultivation, and flasks were closed airtight. Sampling was performed with a syringe through a septum in the lid. Growth was followed via OD450 measurement for 7 h. Aqueous cyclohexane concentrations were estimated via the Henry coefficient and verified via GC.
Product Inhibition Assay
P. taiwanensis_6HA was cultivated as described above and harvested after 4 h of induction. Cells were resuspended in 6 mL Kpi-g buffer containing defined 6HA concentrations (pH readjusted to 7.4) to a biomass concentration of 1.5 gCDW L−1 and transferred into 100 mL screw-capped Erlenmeyer flasks. Afterward, 1 mL was sampled, and the cells were equilibrated for 10 min at 30°C. Biotransformations were started by adding 5.5 µL pure cyclohexane and stopped after 1 h for HPLC and GC analysis as described before.
Inhibition of Single Enzymes by 6HA
P. taiwanensis VLB120 containing plasmids pSEVA_Cyp, pSEVA_CDH, pSEVA_CHMO, or pSEVA_Lact was cultivated in M9* medium as described above and harvested after 6 h of induction. Cells were resuspended to a biomass concentration of 0.25 gCDW L−1 in Kpi-g buffer containing defined 6HA concentrations (pH readjusted to 7.4). Then, 10 mL cell suspension was transferred into 100 mL screw-capped Erlenmeyer flasks (P. taiwanensis VLB120 with pSEVA_Cyp/pSEVA_Lact) or 1 mL cell suspension into 2 mL reaction tubes (P. taiwanensis VLB120 with pSEVA_CDH/pSEVA_CHMO). The cells were equilibrated for 10 min at 30°C, and biotransformations were started by adding 5 mM substrate with respect to the aqueous phase volume (cyclohexane for pSEVA_Cyp, cyclohexanol for pSEVA_CDH, and cyclohexanone for pSEVA_CHMO) or 10 mM ε-CL for pSEVA_Lact. The reactions were stopped after 5 or 10 min of reaction time and prepared for GC and HPLC analysis as described above.
Bioreactor Experiments
Bioreactor experiments were conducted in a 3.6 L stirred-tank Labfors 5 bioreactor controlled via Iris software (Infors AG, Bottmingen, Switzerland). Precultivations were performed in LB and RB media as described above. RB precultures were used to inoculate bioreactors containing 1 L RB-medium (10 g L−1 glucose, pH 7.2, 1.2 vvm aeration, 30°C, 1,500 rpm stirrer speed). The pH was automatically controlled via the addition of 25% (v/v) ammonia and 30% (w/v) phosphoric acid. Batch cultivation was carried out until glucose depletion (cell concentration ∼3 gCDW L−1) and followed by exponential feeding of a solution containing 730 g L−1 glucose and 19.6 g L−1 MgSO4.7 H2O to maintain a specific growth rate µ of 0.1 h−1 until a biomass concentration of 13 gCDW L−1 was reached. For the last 4–6 h, cells were induced with 1 mM IPTG. Then, cells were harvested by centrifugation (3,124 g, RT, 30 min) and resuspended in 1 L Kpi-g-I buffer to a final cell concentration of 10 gCDW L−1. The pH was set to 7.4, and it was automatically controlled via the addition of 10 M NaOH and 30% (w/v) phosphoric acid. After 10 min of equilibration, the aeration was switched to a mix of cyclohexane-saturated air (0.051 or 0.3 L min−1) and pressurized air (1.2 L min−1). Glucose solution (730 g L−1 glucose and 19.6 g L−1 MgSO4.7 H2O) was fed into the reactor as given in the legends of Figures 5, 6. A 5 mL sample was taken at each sampling point and used for GC sample preparation as described above, OD450 measurement, and CO difference spectrum analysis. The rest of the sample was centrifuged (17,000 g, 4°C, 10 min). The pellet (for SDS-PAGE) and supernatant (for HPLC analysis) were stored at −20°C.
Analytical Methods
Suspended biomass concentrations were determined by measuring OD450 using a Libra S11 spectrophotometer (Biochrom, Cambridge, UK). For P. taiwanensis, one OD450 unit corresponds to 0.186 gCDW L−1 (Halan et al., 2010). Recombinant gene expression was analyzed via SDS-PAGE according to Laemmli (Laemmli, 1970), loading 30 μg of total protein per lane, and via CO difference spectra to quantify active Cyp performed as described before (Schäfer et al., 2020b).
Cyclohexane, cyclohexanol, cyclohexanone, and ε-CL concentrations were determined by GC, and 6-hydroxyhexanoic acid and adipic acid concentrations were determined via HPLC as described before (Schäfer et al., 2020a).
Glucose, gluconic acid, and α-ketoglutarate were quantified by HPLC using a Dionex Ultimate 300 separation module (Thermo Fisher Scientific, Waltham, MA) equipped with a ligand exchange column (Hi-Plex H, 30 cm length, 7.7 mm diameter, 8 μm particle size, Agilent, Santa Clara, CA, United States). Glucose was quantified by using a refractive index detector, keeping the column oven temperature constant at 15°C and using MilliQ deionized water as a mobile phase at a flow rate of 0.4 mL min−1. For gluconic acid and α-ketoglutarate quantification, a variable wavelength detector was operated at 210 nm, the column oven temperature was controlled at 40°C, and 5.5 mM H2SO4 in deionized water was applied as the mobile phase at a flow rate of 0.4 mL min−1.
O2 was quantified using a Trace 1310 gas chromatograph (Thermo Fisher Scientific) equipped with a TG-BOND Msieve 5A capillary column (30 m, I.D: 0.32 mm, film thickness: 30 μm, Thermo Fisher Scientific) and a thermal conductivity detector operating at 100°C with a filament temperature of 300°C and a reference gas flow rate of 2 mL min−1. Argon gas was applied as the carrier gas at a constant flow rate of 5 mL min−1. The injection temperature was set to 50°C, and a split ratio of 2 was applied. The oven temperature was kept constant at 35°C for 5 min. N2 was used as the internal standard.
The kinetic parameters Vmax, KS, and KI were calculated in MATLAB 6.1 and fitted to the following equation using the method of least squares:
V0, initial reaction velocity given in U gCDW−1; Vmax, maximal reaction velocity; S, aqueous cyclohexane concentration; KS, apparent substrate uptake constant (cyclohexane concentration, at which reaction velocity is half-maximal); and KI, inhibition constant.
Results
In our previous work, P. taiwanensis_6HA (P. taiwanensis VLB120 containing the plasmid pSEVA_6HA_2) has been developed, enabling cyclohexane conversion to 6HA as the exclusive product (Schäfer et al., 2020a). In this study, we evaluate how and to what extent the catalytic performance of this strain can be exploited under process conditions. For this purpose, the physiology and catalytic performance of P. taiwanensis_6HA were characterized in different media and physiological states with the objective to find suitable conditions for stirred-tank bioreactor (STR) setups.
The Cultivation Medium Influences P. taiwanensis_6HA Physiology and Specific Activity
In the first step, P. taiwanensis_6HA growth physiology and cascade activity were investigated in different standard media, that is, M9, RB, and M9*. Compared to M9, M9* contains 3-fold higher phosphate salt concentrations for a higher buffering capacity (Sambrook and Russell, 2001), whereas RB medium contains more ammonium than M9 and M9* media and intermediate phosphate concentrations (Volmer, 2016). Previous studies have demonstrated that low phosphate and ammonia concentrations lead to gluconate accumulation and higher growth rates of P. aeruginosa and P. taiwanensis VLB120 (Buch et al., 2008; Volmer, 2016). The impact of these factors on biocatalytic performance, however, has not been studied. Here, growth, glucose consumption, gluconate accumulation, and specific 6HA formation activities were investigated utilizing these standard media (Supplementary Figures S1, S2).
The P. taiwanensis_6HA growth physiology differed significantly in the three media (Supplementary Figure S1). The growth rate was highest in M9 medium and was only slightly lower in RB medium, but it was 30% lower in M9* medium (Table 1). Also, significant differences in gluconate accumulation were observed with negligible accumulation in M9* medium, resulting in a relatively stable pH over the cultivation period (Supplementary Figure S1). In M9 medium, however, gluconate transiently accumulated up to 4 g L−1, resulting in a significant pH decrease, whereas cultivation in RB medium resulted in an intermediate gluconate accumulation. The transient gluconate accumulation did not affect the overall biomass yield, which was similar in all three media tested (Table 1).

TABLE 1. Selected physiological parameters for P. taiwanensis_6HA growing in M9, RB, or M9* media with glucose (glu) as carbon source
To investigate whether these apparent physiological differences influence the catalytic performance of P. taiwanensis_6HA, short-term activity assays were conducted with cells sampled during the cultivations (Figure 2). In general, similar induction kinetics (slightly faster in RB medium) were observed in all three media with the maximum specific activity reached after 6 h. The maximum activities reached in RB and M9* media were, however, 2-fold higher than in M9 medium, where some leakiness was also observed. This indicates that the cultivation medium significantly influenced the cell physiology and the heterologous enzymes’ catalytic performance and/or expression. As SDS-PAGE analysis proved inappropriate to detect expression differences among the untagged cascade enzymes, we investigated the medium effect on the expression of tagged CHMO (Supplementary Figure S2). Indeed, we observed a lower CHMO expression level in M9 than in M9* medium, correlating with a lower whole-cell CHMO activity, indicating a negative effect of gluconate accumulation and/or the accordingly fluctuating pH (Supplementary Figure S1) on recombinant gene expression.
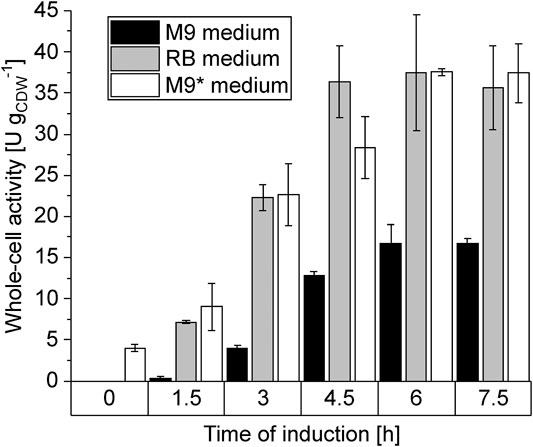
FIGURE 2. Induction studies with P. taiwanensis_6HA. Cells were cultivated in M9 (black), RB (gray), or M9* (white) medium with 0.5% (w/v) glucose, induced by IPTG, and harvested in time intervals of 1.5 h. After resuspension in Kpi buffer containing 1% (w/v) glucose to a biomass concentration of 0.5 gCDW L−1, 10 ml liquid volume was transferred to a 100 mL screw-capped flask and equilibrated for 10 min at 30°C. Reactions were started by adding 5.4 µL pure cyclohexane (corresponding to 5 mM referring to the aqueous phase volume) and stopped after 10 min. Graphs represent average values and standard deviations of two independent biological replicates. The average experimental error over all activity measurements is 15.8%.
Physiological State Affects the P. taiwanensis_6HA Catalytic Activity
For styrene monooxygenase–based in vivo catalysis, metabolically active resting E. coli cells showed a higher specific activity for styrene epoxidation than growing cells (Julsing et al., 2012), whereas the opposite was observed with P. taiwanensis VLB120ΔC (Kuhn et al., 2012a; Kuhn et al., 2012b). To investigate whether P. taiwanensis_6HA is subject to such differences, its biocatalytic performance was investigated for growing or resting cells over an extended time range. Growing cells (GCA), nitrogen-limited resting cells (RCA), and resting cells with an inducer (RCA + IPTG) grown either in RB or M9* medium were applied (Figure 3). M9 medium was not tested as only low specific activities have been obtained in the short-term assays (Figure 3). In the resting cell format, cells grown in M9* and RB media showed a high initial activity of ∼43 U gCDW−1, which, however, dropped by more than 50% within the first hour of the reaction and further to ∼10.5 U gCDW−1 after 2 h, leading to final 6HA titers of 1.1 ± 0.1 and 1.4 ± 0.1 mM, respectively (Figures 3A,D).
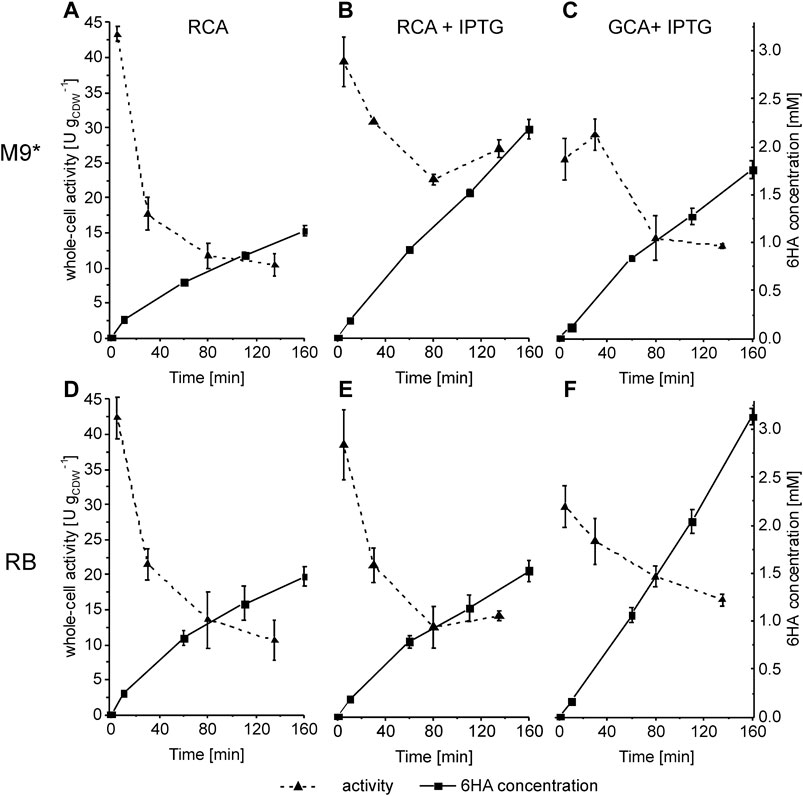
FIGURE 3. Influence of cellular physiology on specific cascade activity of P. taiwanensis_6HA. Cells were cultivated in M9* (A, Band C) or RB (D, E and F) medium with 0.5% (w/v) glucose, induced by IPTG, and harvested 6 h after induction. Cells were resuspended in Kpi buffer containing 1% (w/v) glucose (A, D, RCA), Kpi buffer containing 1 mM IPTG and 1% (w/v) glucose (B, E, RCA + IPTG), or fresh growth medium containing 1 mM IPTG (C, F, GCA) to a biomass concentration of 0.5 gCDW L−1. 20 mL liquid volume was transferred to 100 mL screw-capped flasks and equilibrated for 10 min at 30°C. Reactions were started by adding 10.9 µL pure cyclohexane (corresponding to 5 mM referring to the aqueous phase volume). Sampling was conducted after 10, 60, 100, and 160 min. Graphs represent average values and standard deviations of two independent biological replicates. The average experimental errors over all measurements for the activities and 6HA concentrations are 15.2 and 6.4%, respectively.
To investigate if the whole-cell activity was maintained by continued induction in the resting cell format, we added IPTG to the resting cell suspensions and analyzed the course of specific activities (Figures 3B,E). Again, cells grown in either medium reached initial activities of 40 U gCDW−1. RB-grown cells showed a similar activity decrease as in the absence of IPTG, reaching a final activity of 14.4 ± 0.7 U gCDW−1 and a 6HA titer of 1.5 ± 0.1 mM, indicating no prominent effect of the inducer. However, M9*-grown cells showed less activity drop to 22.6 ± 0.8 U gCDW−1 after 2 h producing 2.2 ± 0.1 mM 6HA, two-fold more than in the absence of IPTG.
The initial activity of growing cells (induced with IPTG) in both media was 30% lower than that of resting cells and dropped to 13.2 ± 0.4 U gCDW−1 in M9* medium and 16.7 ± 0.8 U gCDW−1 in RB medium after 2 h of incubation (Figures 3C,F). The higher growth rate in RB medium resulted in a higher final 6HA concentration of 3.1 ± 0.1 as compared to 1.8 ± 0.1 mM in M9* medium. The gas-phase O2 concentration (headspace) reached 5% of saturation after 160 min growth in RB medium, indicating O2 limitation as a possible reason for the activity decrease. In all other experiments shown in Figure 3, the gas-phase O2 concentration remained above 15% saturation, indicating that O2 was not a major limiting factor. Cyclohexane limitation could be the possible reason for the activity decrease as its concentration in the aqueous phase (extracellular concentration) dropped to 50 µM. Thus, whole-cell kinetic analyses were the next step, also being crucial to optimize cyclohexane feeding for maximal 6HA production.
Whole-Cell Kinetics for Cyclohexane Biotransformation Is Subject to Substrate Inhibition or Toxification
To understand the dependency of the whole-cell activity on the cyclohexane concentration (Karande et al., 2016), the whole-cell kinetics was analyzed in resting cell assays. A substrate inhibition type of kinetics was found, for which parameters are given in Figure 4. Indeed, the obtained substrate uptake constant KS (169 µM) indicates that substrate limitation occurs at the substrate concentrations present (and decreasing) in the experiments shown in Figure 3. Combined with substrate inhibition, this allowed for maximal activities ≥40 U gCDW−1 to be achieved in an aqueous cyclohexane concentration range of ∼100–500 μM, which just includes the initial aqueous substrate concentration of 125 µM in biotransformation assays. Besides, the biomass concentration in resting cell assays dropped from 0.54 ± 0.01 g L−1 to 0.21 ± 0.01 g L−1 (ca. 60%) during 10 min of assay time at 1 mM aqueous cyclohexane concentration. This indicates cell toxification, which can be expected to impair metabolic and catalytic activity at high cyclohexane concentrations. In an earlier study, 70% of the cells were reported to be permeabilized when exposed to an aqueous cyclohexane concentration of 1.1 mM (Karande et al., 2016). Substrate limitation on the one hand and the strong inhibition or toxification of P. taiwanensis_6HA by cyclohexane on the other hand demand a setup with a well-controlled cyclohexane supply to maximally exploit the biocatalytic capacity of the cells.
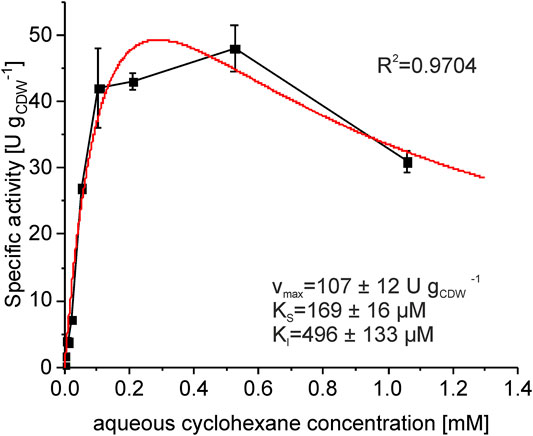
FIGURE 4. Specific 6HA formation rates as a function of the aqueous cyclohexane concentration. Cells were cultivated in M9* medium with 0.5% (w/v) glucose, induced by IPTG, and harvested after 4 h. After resuspension in Kpi buffer containing 1% (w/v) glucose to a biomass concentration of 0.5 gCDW L−1, 10 mL liquid volume was transferred to 100 mL screw-capped Erlenmeyer flasks and equilibrated for 10 min at 30°C. Reactions were started by adding different volumes of pure cyclohexane and stopped after 10 min. The graph represents average values and standard deviations of two independent biological replicates for each substrate concentration. The average experimental error over all measurements for the activities is 7.6%. Kinetic parameters and the coefficient of determination are depicted for fitting the data according to Michaelis–Menten kinetics with substrate inhibition (red line) yielding apparent values for the maximal activity Vmax, the substrate uptake constant KS, and the inhibition constant KI.
Continuous Cyclohexane Feeding in a Stirred-Tank Bioreactor Allows 6HA Production
For establishing such a controlled cyclohexane supply in a stirred-tank bioreactor under process conditions, the application of a second organic phase acting as the substrate reservoir has been tested but was not satisfactory as even low aeration rates led to substantial cyclohexane stripping and thus to a low yield on cyclohexane and substrate limitation (Hoschek et al., 2019b). Direct cyclohexane feeding may reduce stripping but may involve a concentration gradient and cell toxification. Instead, cyclohexane feeding via the gas phase was evaluated. For this purpose, the aeration stream was saturated with cyclohexane in a separate container, leading to a gas phase concentration of 5.42 ± 0.04 mM. This cyclohexane-saturated air was then mixed with pure air to create an aeration gas flow providing the desired cyclohexane amount (Supplementary Figure S3). Thereby, a variation of the cyclohexane-saturated air to pure air flow rate ratio allowed for the tuning of the gaseous cyclohexane concentration in the reactor. For the first set of experiments, we selected a cyclohexane feed rate of 0.276 mmol min−1 L−1, corresponding to an aqueous phase concentration of 30 µM upon equilibration with the gas phase. Thereby, the system was operated under substrate limitation to minimize cyclohexane toxicity effects.
Cells were first grown in batch mode in RB medium until glucose depletion, and they were further grown in fed-batch mode with an exponential feed enabling µ = 0.1 h−1. During fed-batch cultivation, cells were induced for heterologous cascade gene expression, followed by harvesting and resuspension in glucose and IPTG-containing Kpi buffer. Within the first 2 h, the cell concentration increased slightly from 9.2 to 11.4 gCDW L−1, possibly due to remaining nutrients from the cultivation medium, and then remained constant for the next 4 h (Figure 5A). O2 limitation was avoided by maintaining the dissolved oxygen concentration (pO2) between 20 and 50% of saturation. Gluconate accumulated to a final concentration of 8.5 g L−1 after 6 h of biotransformation. Within the first 3 h, the aqueous cyclohexane concentration remained low (Figure 5B), whereas the cyclohexane-limited specific activity slightly decreased from ∼8 to 6 U gCDW−1, roughly correlating to the cell density increase expected to lead to more severe substrate limitation (Figure 5C). During this time period, ∼50 mmol cyclohexane was fed via the gas phase and 13.3 ± 0.3 mmol product accumulated (about 25% conversion). After 3 h, the cyclohexane concentration increased to and stabilized at ∼18 μM, whereas the specific activity decreased to ∼4 U gCDW−1. This is lower than the 10.3 U gCDW−1 expected at 18 µM cyclohexane according to the kinetic parameters (Figure 4 and Eq. 1). Considering that no pathway intermediates accumulated, this may be due to a loss in Cyp activity (first reaction step) or a change in cell physiology (mass transfer over cellular membranes and metabolic activity). As assessed via CO difference spectra (Figure 5C), the amount of active Cyp dropped by 30% within the first 3 h of reaction and then remained stable, which cannot completely explain the activity course. A final product concentration of 22.8 ± 1.0 mM was reached (Figure 5B), which includes the main product 6HA and the overoxidation product adipic acid (AA) (1.2% of the total product). Overall, a product yield on biomass of 0.28 g gCDW−1 and an average productivity of 0.50 g L−1 h−1 were obtained (Table 2).
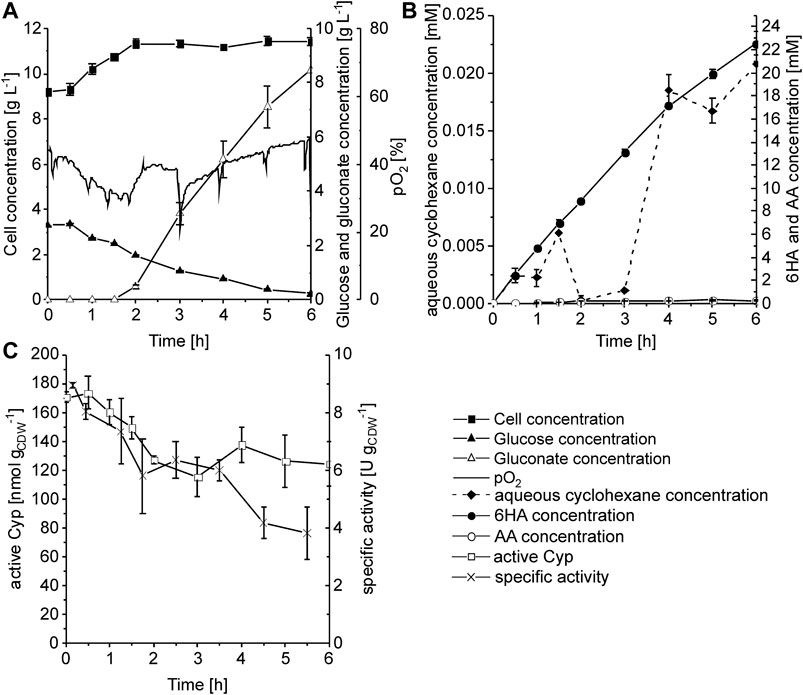
FIGURE 5. Biotransformation of cyclohexane to 6HA in a stirred-tank bioreactor with a cyclohexane feed of 0.276 mmol min−1 L−1. P. taiwanensis_6HA was cultivated in batch mode in 1 L RB medium, followed by glucose-limited fed-batch cultivation with exponential feeding for µ = 0.1 h−1. Cells were induced after 6 h of fed-batch cultivation and cultivated for another 6 h until they were harvested. Afterward, the biotransformation was started with 10 g L−1 biomass concentration in 1 L Kpi buffer containing 1% glucose (w/v) and 1 mM IPTG by applying a cyclohexane feed rate of 0.276 mmol min−1 L−1 and a glucose feed rate of 7.4 g h−1 (started after 1.5 h). (A) Time courses of cell, glucose, gluconate, and dissolved oxygen (pO2) concentrations. (B) Time courses of aqueous cyclohexane, 6HA, and AA concentrations. (C) Time courses of active Cyp amount determined via CO difference spectra and specific activity based on 6HA and AA accumulation. Graphs represent average values and standard deviations of two technical replicates.
Higher Cyclohexane Concentrations Result in Enhanced Initial Activities but Poor Biocatalyst Stability
To evaluate how far substrate limitation can be relieved without harming biocatalyst performance, the substrate feed rate via the gas phase was increased 6-fold, corresponding to an aqueous cyclohexane concentration of 147 µM (see Materials and Methods for details). The cell concentration increased slightly during the first 4 h from 8 to 9 gCDW L−1 but then dropped to 7 gCDW L−1 after 8 h (Figure 6A). Together with the continuous pO2 increase, this indicates inhibition/toxification of cell metabolism, which led to a pronounced accumulation of glucose and also gluconate (up to 50 and 20 g L−1, respectively). Furthermore, minor α-ketoglutarate formation (1.6 g L−1) was observed. The aqueous cyclohexane concentration continuously increased from an initial value of 0.1 mM to 0.4 mM after 8 h of biotransformation (Figure 6B), indicating an increase in cyclohexane solubility due to the biology- and biotransformation-related changes in aqueous phase composition. This has also been observed before in a nonaerated STR for cyanobacteria-based cyclohexane hydroxylation in the presence of a second organic phase (Hoschek et al., 2019b). It has been shown that some microbial species, including pseudomonads, produce surfactants to increase substrate solubility and thus availability (Margaritis et al., 1979; Mukherjee et al., 2006). Also, the addition of hydrophobic compounds to E. coli cultures in an aerated STR was found to cause the appearance of a cell fraction with hydrophobic surface properties (Collins et al., 2015) – another possible cause for such a solubility increase. The feed rate increase led to a 2.5-fold higher initial whole-cell activity of 22.8 ± 2.2 U gCDW−1, which was followed by a more drastic decrease to 4.7 ± 1.9 U gCDW−1 within the first 3 h of the reaction (Figure 6C), and thus did not lead to an improvement of overall biotransformation performance (Figures 5, 6; Table 2). Interestingly, active Cyp amounts remained stable over the reaction time and no pathway intermediates accumulated, indicating a limitation caused by changes in cell physiology/metabolism and not by intracellular enzyme amounts.
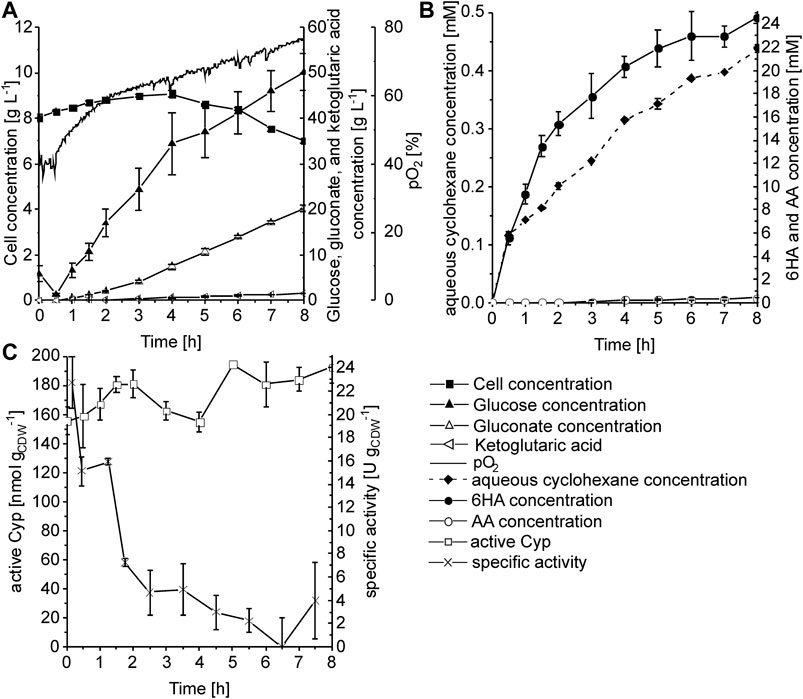
FIGURE 6. Biotransformation of cyclohexane to 6HA in a stirred-tank bioreactor with a cyclohexane feed of 1.626 mmol min−1 L−1. P. taiwanensis_6HA was cultivated in batch mode in 1 L RB medium, followed by fed-batch cultivation with exponential feeding for µ = 0.1 h−1. Cells were induced after 10 h of fed-batch cultivation and cultivated for another 3.5 h until they were harvested. The biotransformation was started with 10 g L−1 biomass concentration in 1 L Kpi buffer containing 1% glucose (w/v) and 1 mM IPTG by applying a cyclohexane feed rate of 1.626 mmol min−1 L−1 and a glucose feed rate of 14.8 g h−1 (started after 0.5 h). (A) Time courses of cell, glucose, gluconate, and dissolved oxygen (pO2) concentrations. (B) Time courses of aqueous cyclohexane, 6HA, and AA concentrations. (C) Time courses of active Cyp amount determined via CO difference spectra and specific activity based on 6HA and AA accumulation. Graphs represent average values and standard deviations of two technical replicates.
To identify possible reasons for the observed activity decrease, we evaluated product (6HA) inhibition and cell toxification by cyclohexane. Indeed, increasing 6HA concentrations led to decreasing specific activities (Figure 7A) and, therefore, might have contributed to the activity loss in both bioreactors. The mode of microbial 6HA export is unknown but possibly involves active transport due to its charged character at pH 7.2. Strikingly, only Cyp and lactonase were inhibited by 6HA when cells containing a single cascade enzyme were tested (Supplementary Figure S4). On the other hand, cyclohexane inhibited the growth of P. taiwanensis VLB120 with a half-maximal growth rate observed at an aqueous cyclohexane concentration of ∼450 µM (Figure 7B). Cyclohexane concentrations in the first reactor (Figure 5B) were far lower, but they reached 0.4 mM in the second reactor (Figure 6B). Overall, both cyclohexane toxicity and product inhibition are factors affecting biocatalyst stability under process conditions.
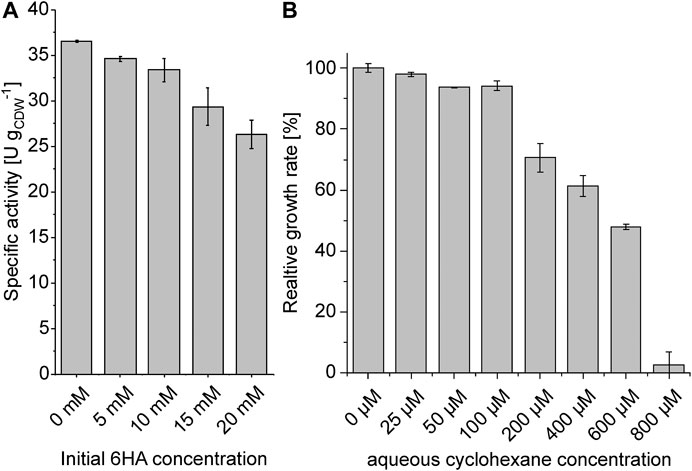
FIGURE 7. Impact of 6HA on the specific activity of P. taiwanensis_6HA (A) and effect of cyclohexane on growth of P. taiwanensis VLB120 (B). (A) Cells were cultivated in M9*-containing shake flasks, induced for 4 h, harvested, and resuspended in Kpi buffer (1% glucose (w/v), varying 6HA concentrations, pH 7.4) to a biomass concentration of 1.5 gCDW L−1. Biotransformations were conducted in 5 mL liquid volume within 100 mL screw-capped Erlenmeyer flasks, started by adding 5.5 µL pure cyclohexane (corresponding to 10 mM referring to the aqueous phase volume), and terminated after 1 h. (B) Relative growth rate in the presence of varying amounts of cyclohexane in M9* medium (a growth rate of 0.461 ± 0.006 h−1 represents 100%). Graphs represent average values and standard deviations of two independent biological replicates. The average experimental errors over all measurements for the specific activities and relative growth rates (except the values at 800 µM indicating complete growth inhibition) are 3.6 and 2.5%, respectively.
Discussion
Polycaprolactone (PCL) belongs to the group of biodegradable polymers originating from fossil resources. PCL is often used in blends and has applications in the medicinal (Serrano et al., 2010), packaging (Gross and Kalra, 2002), and electronics sectors (Labet and Thielemans, 2009; Gao et al., 2017). Its ester bonds are mainly responsible for its hydrolysis-based biodegradability, which depends on its molecular weight and degree of crystallinity (Gross and Kalra, 2002). At least 39 species of the bacterial classes Firmicutes and Proteobacteria have been identified to degrade PCL within several days (Tokiwa et al., 2009). Recently, we have developed recombinant P. taiwanensis VLB120 strains that synthesize polycaprolactone monomers from cyclohexane with 100% conversion and turnover numbers of 45,000 mol monomer per mol of Cyp, the first and rate-limiting enzyme of the engineered in vivo cascade (Schäfer et al., 2020a). In this work, we studied the impact of cultivation conditions on P. taiwanensis_6HA growth and physiology and, in turn, on specific activity in order to evaluate how and to what extent its catalytic potential can be exploited in bioreactor setups for cyclohexane conversion to 6HA.
Cultivation Medium and Conditions Influence Gluconate Accumulation and Whole-Cell Activity
P. taiwanensis_6HA has been characterized in different media and whole-cell biocatalyst formats. M9, RB, and M9* media are standard minimal media used to cultivate heterotrophic organisms (Riesenberg et al., 1991; Sambrook and Russell, 2001). Their composition can influence cellular physiology and catalytic performance, as confirmed in this study for P. taiwanensis_6HA. Cultivation of P. aeruginosa, Aspergillus niger, and P. taiwanensis VLB120 at low phosphate and ammonium concentrations has been reported to involve gluconate accumulation (Buch et al., 2008; Müller, 1986; Volmer, 2016). We observed that gluconate accumulation could be prevented by increasing the phosphate content 3-fold in M9* compared to M9 medium, which, however, involved a reduction in the specific growth rate (Figure 2; Table 1). With an intermediate phosphate level, RB medium leads to 40% less gluconate accumulation than M9 medium but does not significantly affect the growth rate. Gluconate is formed by periplasmatic glucose dehydrogenase (Gcd) and diffuses via pores into the medium, where it is known to function as a phosphate solubilizer (Buch et al., 2008; Del Castillo et al., 2007). It has been reported that excessive gluconate formation hampered polyhydroxyalkanoate synthesis (Poblete-Castro et al., 2013) and isobutyric acid production (Lang et al., 2014). Although gluconate was formed in RB medium, no difference in the specific activity of P. taiwanensis_6HA was observed compared to M9* medium (Figure 2), indicating that gluconate formation in RB medium does not affect biocatalyst performance. P. taiwanensis_6HA, however, showed lower specific activity when grown in M9 medium (Figure 2). More pronounced gluconate formation and an unstable pH in M9 medium constitute possible reasons for such differences (Figure 2; Table 1). Another study revealed that high growth rates observed for the fungus Trichoderma reesei in M9 medium resulted in lower heterologous protein synthesis (Pakula et al., 2005). Accordingly, a decrease in heterologous protein synthesis appeared to cause the lower activity of P. taiwanensis_6HA grown in an M9 medium (Supplementary Figure S1). Overall, the M9 medium can be considered not suitable for 6HA production by P. taiwanensis_6HA due to gluconate accumulation and lower specific activities. A previous study reported that metabolically active resting E. coli cells have a higher styrene epoxidation capacity than growing cells cultivated in RB medium (Julsing et al., 2012). Conversely, the more stable activity of growing cells made them more suitable for process setups (Kuhn et al., 2012b). These results indicate that the cellular growth state significantly influences whole-cell activity, especially during long-term cultivation. Resting cells of P. taiwanensis_6HA exhibited a fast drop in specific activity by more than 50% within the first hour of the reaction (Figures 3A,D) The addition of the inducer IPTG to resting cells resulted in some activity stabilization (Figures 3B,E), possibly due to protein resynthesis based on amino acids derived from proteolysis of denatured and obsolete proteins (Konovalova et al., 2014). Whereas growing cells showed lower initial activities than resting cells, their activity loss was less pronounced (Figures 3C,F) as it can be expected from their more constant and high metabolic and protein synthesis activity. However, substrate limitation, product inhibition, and/or toxification by cyclohexane cannot be excluded as additional factors leading to the observed decrease in whole-cell activities, although 6HA and aqueous cyclohexane remained low (below 3.5 mM and 125 μM, respectively). With growing cells, RB medium appeared to be more promising than M9*. Thereby, the constant volumetric product accumulation rate and low O2 levels in the gas phase indicate that the slow decrease in specific activity may have been caused by an increasing O2 limitation at the increasing cell concentration in shake flasks.
Substrate Supply and Product Removal as Critical Aspects in Reaction Engineering
Cyclohexane is a small (84 Da) and hydrophobic molecule and can rapidly pass the outer and inner membranes of P. taiwanensis (Chen, 2007; Nikaido, 2003). In addition, its hydrophobic character (logPoctanol/water = 3.4) favors its accumulation in the cellular membrane, making it extremely toxic for microorganisms (membrane disintegration at 600 µM) (Sikkema et al., 1994). Thus, cyclohexane supply in a stirred-tank bioreactor is the most critical step to produce monomers. Previous studies either performed shake-flask experiments (Wang et al., 2020; Zhang et al., 2020) or started from the less toxic and less volatile substrate cyclohexanol to produce monomers via cascade reactions (Srinivasamurthy et al., 2019). To exploit the catalytic performance of P. taiwanensis_6HA in a controlled environment enabling high O2 transfer, a continuous aeration-mediated gaseous cyclohexane feed was established for bioreactor-based biotransformations (Supplementary Figure S3). Considering the resulting aqueous substrate concentrations (Figures 5B, 6B), the two different feed rates applied can be expected to involve first- and zero-order kinetics (Figure 4). Whereas the initial activity was indeed 2.5-fold higher with the higher feed rate (Figures 5C, 6C), the specific activities obtained in shake flasks were not reached, and the higher feed rate involved a relatively fast activity decrease. The stable Cyp amount during the entire non–cyclohexane-limited biotransformation (Figure 6C) indicates that product inhibition and/or an impaired metabolic activity and thus NADH regeneration possibly due to cyclohexane toxicity hampered biocatalyst activity. Cyclohexane toxicity was indeed found to be a critical factor, with the growth rate reduced by half at ∼450 µM (Figure 7B), roughly corresponding to its concentration at the end of the non-cyclohexane-limited biotransformation (Figure 6B). Such a possible toxicity effect is supported by the much less prominent activity decrease in the cyclohexane-limited biotransformation (Figure 5). Metabolic activity–related toxicity effects have also been observed for other low logP-compounds such as styrene oxide, even at apparently subtoxic levels (Kuhn et al., 2013; Kadisch et al., 2017a; Kadisch et al., 2017b). Furthermore, the contribution of product inhibition/toxicity cannot be excluded (Schäfer et al., 2020a), although 6HA concentrations in the time period of the most prominent activity decrease only had a minor effect on resting cell activities (Figure 7A). In the substrate-limited biotransformation, the cyclohexanone concentration remained below 25 µM and thus was not in the toxic range (Figure 5B). Nevertheless, a moderate activity decrease was also observed here, which, despite the lower initial activity, still enabled a comparable final product concentration as obtained with the higher feed rate (Table 2). In this case, product inhibition, especially toward the end of the biotransformation, constitutes the most plausible reason for the moderate activity decrease (Figure 7A).
Srinivasamurthy et al. (2020) developed a whole-cell process with E. coli and obtained 185 mM ε-caprolactone from the more expensive but less volatile and less toxic substrate cyclohexanol. They applied a continuous substrate feed via a syringe pump and a purified lipase to obtain 6HA as the main product (Srinivasmurthy et al., 2020). The resulting process was stable for 70 h, resulting in 7-fold higher final product titers than our whole-cell process with cyclohexane as substrate (Table 2). While the approach presented in this study makes use of a cheaper substrate and does not involve the addition of an expensive enzyme, process performance needs to be further improved to reach industrial relevance. Gaseous cyclohexane feeding does not allow high substrate conversion (Table 2) and thus requires cyclohexane recycling via condensation, which, however, can be considered standard technology. The optimal cyclohexane feed concentration may lie between the two tested cyclohexane concentrations but cannot be expected to completely avoid the toxicity and inhibitory effects of the substrate and product. Additionally, the impact of prolonged cyclohexane exposure on whole-cell activity needs to be evaluated to fine-tune aqueous substrate concentrations. In situ product removal appears mandatory, which is challenging for 6HA due to its hydrophilic nature. It has been shown that solid-phase extraction could enhance the product titer up to 2000-fold (Phillips et al., 2013). Additionally, the use of supercritical fluid extraction constitutes an option (Khosravi-Darani and Vasheghani-Farahani, 2005). It has been shown that in situ polymerization to PCL with a lipase is possible, which might be combined with product extraction into a second organic or supercritical phase (Schmidt et al., 2015; Scherkus et al., 2016). This second phase may also be used as a cyclohexane reservoir to alleviate its toxic effects (Hoschek et al., 2019b). The use of cyclohexane as organic phase and VLB120 cells with a solvent-tolerant phenotype may constitute another option (Volmer et al., 2014). However, the high energy demand for solvent tolerance (Blank et al., 2008; Kuhn et al., 2012a) and the volatility and explosivity of cyclohexane in an aerated system are drawbacks of this approach.
Although growing cells have a high energy demand for growth (Bühler et al., 2008) and showed lower initial activities (Figure 3), it is worth testing them for cascade reactions in a bioreactor setup as they have been found to show less severe product inhibition/toxification (Julsing et al., 2012). This may involve an energy-dependent tolerance mechanism and a more efficient protein resynthesis, thereby enhancing process stability. Such a higher stability may also be realized by employing continuous biofilm capillary reactors as an alternative to STRs (Heuschkel et al., 2019). Such a system has been shown to allow cyclohexane oxidation rates of 0.4 g L−1 h−1 for several days (Karande et al., 2016). A combination of heterotrophic P. taiwanensis with the autotrophic Synechocystis sp. enabled even higher stabilities and 100% conversion of cyclohexane (Hoschek et al., 2019a).
Conclusion and Outlook
This study reports on the successful transfer of a 4-step cascade harbored by recombinant P. taiwanensis VLB120 into a bioreactor format for the conversion of cyclohexane to the polymer building block 6HA and discusses encountered challenges. The cultivation medium composition was found to be a critical factor for the achievement of high whole-cell activities. The standard M9 medium was not suitable due to excessive gluconate formation from glucose, which is associated with lower catalytic activities. RB and M9* media were both suitable, with RB medium enabling faster growth to higher cell densities. Metabolically active resting cells exhibited a rather fast activity decrease under reaction conditions, whereas growing cells showed more stable but lower initial activities. The addition of an inducer could stabilize resting cells, which were applied in a bioreactor setup involving a gaseous cyclohexane feed. This approach enabled a product titer of 24 mM. Further analyses showed that toxification by the substrate cyclohexane constituted a critical factor, resulting in a delicate trade-off between substrate toxicity and substrate limitation. Also, product inhibition was found to become critical at high 6HA levels. Optimization of the cyclohexane feed, gas recycling, in situ product removal, and continuous reaction formats constitute strategies to further increase yield and productivity.
Data Availability Statement
The raw data supporting the conclusion of this article will be made available by the authors, without undue reservation.
Author Contributions
LB, IH, KB, RK, and BB were involved in conception and design of the study and data interpretation. LB, IH, MW, and ML collected data and were involved in data analysis. LB wrote the original draft of the manuscript. KB, RK, and BB contributed in terms of article structuring and editing. All authors were involved in final editing and approved the submitted version.
Funding
LS, IH, and ML were funded by the ERA-IB Project PolyBugs (ID:16006) and the Sächsisches Ministerium für Wissenschaft und Kunst (SMWK) (Project ID: 100318259).
Conflict of Interest
The authors declare that the research was conducted in the absence of any commercial or financial relationships that could be construed as a potential conflict of interest.
Acknowledgments
We acknowledge the use of the facilities of the Center for Biocatalysis (MiKat) at the Helmholtz Center for Environmental Research, which is supported by the European Regional Development Funds (EFRE, Europe funds Saxony) and the Helmholtz Association. The authors would like to thank Andreas Schmid for helpful discussions.
Supplementary Material
The Supplementary Material for this article can be found online at: https://www.frontiersin.org/articles/10.3389/fctls.2021.683248/full#supplementary-material
References
Blank, L. M., Ionidis, G., Ebert, B. E., Bühler, B., and Schmid, A. (2008). Metabolic Response of Pseudomonas Putida during Redox Biocatalysis in the Presence of a Second Octanol Phase. FEBS J. 275, 5173–5190. doi:10.1111/j.1742-4658.2008.06648.x
Bretschneider, L., Wegner, M., Bühler, K., Bühler, B., and Karande, R. (2021). One-pot Synthesis of 6-aminohexanoic Acid from Cyclohexane Using Mixed-Species Cultures. Microb. Biotechnol 14 (3), 1011–1025. doi:10.1002/bit.27791
Buch, A., Archana, G., and Naresh Kumar, G. (2008). Metabolic Channeling of Glucose towards Gluconate in Phosphate-Solubilizing Pseudomonas aeruginosa P4 under Phosphorus Deficiency. Res. Microbiol. 159, 635–642. doi:10.1016/j.resmic.2008.09.012
Bühler, B., Park, J. B., Blank, L. M., and Schmid, A. (2008). NADH Availability Limits Asymmetric Biocatalytic Epoxidation in a Growing Recombinant Escherichia coli Strain. Appl. Environ. Microbiol. 74, 1436–1446. doi:10.1128/AEM.02234-07
Bühler, B., Bollhalder, I., Hauer, B., Witholt, B., and Schmid, A. (2003). Use of the Two-Liquid Phase Concept to Exploit Kinetically Controlled Multistep Biocatalysis. Biotechnol. Bioeng. 81, 683–694. doi:10.1002/bit.10512
ChemSpider (2020). Cyclohexane. Available at: http://www.chemspider.com/Chemical-Structure.7787.html. Accessed October 14, 2020.
Chen, R. R. (2007). Permeability Issues in Whole-Cell Bioprocesses and Cellular Membrane Engineering. Appl. Microbiol. Biotechnol. 74, 730–738. doi:10.1007/s00253-006-0811-x
Collins, A. M., Woodley, J. M., and Liddell, J. M. (1995). Determination of Reactor Operation for the Microbial Hydroxylation of Toluene in a Two-Liquid Phase Process. J. Ind. Microbiol. 14, 382–388. doi:10.1007/bf01569955
Collins, J., Grund, M., Brandenbusch, C., Sadowski, G., Schmid, A., and Bühler, B. (2015). The Dynamic Influence of Cells on the Formation of Stable Emulsions in Organic-Aqueous Biotransformations. J. Ind. Microbiol. Biotechnol. 42, 1011–1026. doi:10.1007/s10295-015-1621-x
Del Castillo, T., Ramos, J. L., Rodríguez-Herva, J. J., Fuhrer, T., Sauer, U., and Duque, E. (2007). Convergent Peripheral Pathways Catalyze Initial Glucose Catabolism in Pseudomonas Putida: Genomic and Flux Analysis. J Bacteriol. 189, 5142–5152. doi:10.1128/jb.00203-07
Gao, Y., Sim, K., Yan, X., Jiang, J., Xie, J., and Yu, C. (2017). Thermally Triggered Mechanically Destructive Electronics Based on Electrospun Poly (ε-Caprolactone) Nanofibrous Polymer Films. Sci. Rep. 7, 947. doi:10.1038/s41598-017-01026-6
Gross, R. A., and Kalra, B. (2002). Biodegradable Polymers for the Environment. Science 297, 803–807. doi:10.1126/science.297.5582.803
Halan, B., Schmid, A., and Buehler, K. (2010). Maximizing the Productivity of Catalytic Biofilms on Solid Supports in Membrane Aerated Reactors. Biotechnol. Bioeng. 106, 516–527. doi:10.1002/bit.22732
Harder, W., and Dijkhuizen, L. (1983). Physiological Responses to Nutrient Limitation. Annu. Rev. Microbiol. 37, 1–23. doi:10.1146/annurev.mi.37.100183.000245
Heipieper, H. J., Neumann, G., Cornelissen, S., and Meinhardt, F. (2007). Solvent-tolerant Bacteria for Biotransformations in Two-phase Fermentation Systems. Appl. Microbiol. Biotechnol. 74, 961–973. doi:10.1007/s00253-006-0833-4
Heuschkel, I., Hoschek, A., Schmid, A., Bühler, B., Karande, R., and Bühler, K. (2019). Mixed-trophies Biofilm Cultivation in Capillary Reactors. MethodsX 6, 1822–1831. doi:10.1016/j.mex.2019.07.021
Hoschek, A., Heuschkel, I., Schmid, A., Bühler, B., Karande, R., and Bühler, K. (2019a). Mixed-species Biofilms for High-Cell-Density Application of Synechocystis Sp. PCC 6803 in Capillary Reactors for Continuous Cyclohexane Oxidation to Cyclohexanol. Bioresour. Tech. 282, 171–178. doi:10.1016/j.biortech.2019.02.093
Hoschek, A., Toepel, J., Hochkeppel, A., Karande, R., Bühler, B., and Schmid, A. (2019b). Light‐Dependent and Aeration‐Independent Gram‐Scale Hydroxylation of Cyclohexane to Cyclohexanol by CYP450 Harboring Synechocystis Sp. PCC 6803. Biotechnol. J. 14, 1800724. doi:10.1002/biot.201800724
Inoue, A., and Horikoshi, K. (1989). A Pseudomonas Thrives in High Concentrations of Toluene. Nature 338, 264–266. doi:10.1038/338264a0
Julsing, M. K., Kuhn, D., Schmid, A., and Bühler, B. (2012). Resting Cells of Recombinant E. coli Show High Epoxidation Yields on Energy Source and High Sensitivity to Product Inhibition. Biotechnol. Bioeng. 109, 1109–1119. doi:10.1002/bit.24404
Kadisch, M., Julsing, M. K., Schrewe, M., Jehmlich, N., Scheer, B., von Bergen, M., et al. (2017a). Maximization of Cell Viability rather Than Biocatalyst Activity Improves Whole-Cell ω-oxyfunctionalization Performance. Biotechnol. Bioeng. 114, 874–884. doi:10.1002/bit.26213
Kadisch, M., Willrodt, C., Hillen, M., Bühler, B., and Schmid, A. (2017b). Maximizing the Stability of Metabolic Engineering-Derived Whole-Cell Biocatalysts. Biotechnol. J. 12, 1600170. doi:10.1002/biot.201600170
Karande, R., Debor, L., Salamanca, D., Bogdahn, F., Engesser, K.-H., Buehler, K., et al. (2016). Continuous Cyclohexane Oxidation to Cyclohexanol Using a Novel Cytochrome P450 Monooxygenase from Acidovorax sp. CHX100 in recombinant P. taiwanensis VLB120 Biofilms. Biotechnol. Bioeng. 113, 52–61. doi:10.1002/bit.25696
Khosravi-Darani, K., and Vasheghani-Farahani, E. (2005). Application of Supercritical Fluid Extraction in Biotechnology. Crit. Rev. Biotechnol. 25, 231–242. doi:10.1080/07388550500354841
Köhler, K. A. K., Rückert, C., Schatschneider, S., Vorhölter, F.-J., Szczepanowski, R., Blank, L. M., et al. (2013). Complete Genome Sequence of Pseudomonas Sp. Strain VLB120 a Solvent Tolerant, Styrene Degrading Bacterium, Isolated from Forest Soil. J. Biotechnol. 168, 729–730. doi:10.1016/j.jbiotec.2013.10.016
Konovalova, A., Søgaard-Andersen, L., and Kroos, L. (2014). Regulated Proteolysis in Bacterial Development. FEMS Microbiol. Rev. 38, 493–522. doi:10.1111/1574-6976.12050
Kuhn, D., Bühler, B., and Schmid, A. (2012a). Production Host Selection for Asymmetric Styrene Epoxidation: Escherichia coli vs. Solvent-Tolerant Pseudomonas. J. Ind. Microbiol. Biotechnol. 39, 1125–1133. doi:10.1007/s10295-012-1126-9
Kuhn, D., Fritzsch, F. S. O., Zhang, X., Wendisch, V. F., Blank, L. M., Bühler, B., et al. (2013). Subtoxic Product Levels Limit the Epoxidation Capacity of Recombinant E. coli by Increasing Microbial Energy Demands. J. Biotechnol. 163, 194–203. doi:10.1016/j.jbiotec.2012.07.194
Kuhn, D., Julsing, M. K., Heinzle, E., and Bühler, B. (2012b). Systematic Optimization of a Biocatalytic Two-Liquid Phase Oxyfunctionalization Process Guided by Ecological and Economic Assessment. Green. Chem. 14, 645–653. doi:10.1039/c2gc15985f
Labet, M., and Thielemans, W. (2009). Synthesis of Polycaprolactone: a Review. Chem. Soc. Rev. 38, 3484–3504. doi:10.1039/b820162p
Laemmli, U. K. (1970). Cleavage of Structural Proteins during the Assembly of the Head of Bacteriophage T4. Nature 227, 680–685. doi:10.1038/227680a0
Lang, K., Zierow, J., Buehler, K., and Schmid, A. (2014). Metabolic Engineering of Pseudomonas Sp. Strain VLB120 as Platform Biocatalyst for the Production of Isobutyric Acid and Other Secondary Metabolites. Microb. Cel Fact. 13, 2. doi:10.1186/1475-2859-13-2
Lilly, M. (1982). Two‐liquid‐phase Biocatalytic Reactions. J. Chem. Technol. Biotechnol. 32, 162–169.
Margaritis, A., Zajic, J. E., and Gerson, D. F. (1979). Production and Surface-Active Properties of Microbial Surfactants. Biotechnol. Bioeng. 21, 1151–1162. doi:10.1002/bit.260210706
Mukherjee, S., Das, P., and Sen, R. (2006). Towards Commercial Production of Microbial Surfactants. Trends Biotechnol. 24, 509–515. doi:10.1016/j.tibtech.2006.09.005
Müller, H.-M. (1986). Gluconate Accumulation and Enzyme Activities with Extremely Nitrogen-Limited Surface Cultures of Aspergillus niger. Arch. Microbiol. 144, 151–157. doi:10.1007/BF00414726
Nikaido, H. (2003). Molecular Basis of Bacterial Outer Membrane Permeability Revisited. Microbiol. Mol. Biol. Rev. 67, 593–656. doi:10.1128/mmbr.67.4.593-656.2003
Pakula, T. M., Salonen, K., Uusitalo, J., and Penttilä, M. (2005). The Effect of Specific Growth Rate on Protein Synthesis and Secretion in the Filamentous Fungus Trichoderma Reesei. Microbiology 151, 135–143. doi:10.1099/mic.0.27458-0
Panke, S., Meyer, A., Huber, C. M., Witholt, B., and Wubbolts, M. G. (1999). An Alkane-Responsive Expression System for the Production of Fine Chemicals. Appl. Environ. Microbiol. 65, 2324–2332. doi:10.1128/aem.65.6.2324-2332.1999
Peralta-Yahya, P. P., Zhang, F., Del Cardayre, S. B., and Keasling, J. D. (2012). Microbial Engineering for the Production of Advanced Biofuels. Nature 488, 320–328. doi:10.1038/nature11478
Phillips, T., Chase, M., Wagner, S., Renzi, C., Powell, M., DeAngelo, J., et al. (2013). Use of In Situ Solid-phase Adsorption in Microbial Natural Product Fermentation Development. J. Ind. Microbiol. Biotechnol. 40, 411–425. doi:10.1007/s10295-013-1247-9
Poblete-Castro, I., Binger, D., Rodrigues, A., Becker, J., Martins dos Santos, V. A. P., and Wittmann, C. (2013). In-silico-driven Metabolic Engineering of Pseudomonas Putida for Enhanced Production of Poly-Hydroxyalkanoates. Metab. Eng. 15, 113–123. doi:10.1016/j.ymben.2012.10.004
Riesenberg, D., Schulz, V., Knorre, W. A., Pohl, H.-D., Korz, D., Sanders, E. A., et al. (1991). High Cell Density Cultivation of Escherichia coli at Controlled Specific Growth Rate. J. Biotechnol. 20, 17–27. doi:10.1016/0168-1656(91)90032-q
Sambrook, J., and Russell, D. W. (2001). Molecular Cloning: A Laboratory Manual. New York, NY: Cold Spring Harbor Laboratory, Cold Spring Harbor.
Schäfer, L., Bühler, K., Karande, R., and Bühler, B. (2020a). Rational Engineering of a Multi‐Step Biocatalytic Cascade for the Conversion of Cyclohexane to Polycaprolactone Monomers in Pseudomonas Taiwanensis. Biotechnol. J. 15, 2000091. doi:10.1002/biot.202000091
Schäfer, L., Karande, R., and Bühler, B. (2020b). Maximizing Biocatalytic Cyclohexane Hydroxylation by Modulating Cytochrome P450 Monooxygenase Expression in P. Taiwanensis VLB120. Front. Bioeng. Biotechnol. 8, 140. doi:10.3389/fbioe.2020.00140
Scherkus, C., Schmidt, S., Bornscheuer, U. T., Gröger, H., Kara, S., and Liese, A. (2016). A Fed-Batch Synthetic Strategy for a Three-step Enzymatic Synthesis of Poly-ϵ-Caprolactone. ChemCatChem 8, 3446–3452. doi:10.1002/cctc.201600806
Schmidt, S., Scherkus, C., Muschiol, J., Menyes, U., Winkler, T., Hummel, W., et al. (2015). An Enzyme Cascade Synthesis of ε-Caprolactone and its Oligomers. Angew. Chem. Int. Ed. 54, 2784–2787. doi:10.1002/anie.201410633
Serrano, M. C., Chung, E. J., and Ameer, G. A. (2010). Advances and Applications of Biodegradable Elastomers in Regenerative Medicine. Adv. Funct. Mater. 20, 192–208. doi:10.1002/adfm.200901040
Sikkema, J., De Bont, J., and Poolman, B. (1994). Interactions of Cyclic Hydrocarbons with Biological Membranes. J. Biol. Chem. 269, 8022–8028.
Srinivasamurthy, V. S. T., Böttcher, D., and Bornscheuer, U. T. (2019). A Multi-Enzyme Cascade Reaction for the Production of 6-hydroxyhexanoic Acid. Z. Naturforsch. C Bio. Sci. 74, 71–76. doi:10.1515/znc-2018-0216
Srinivasamurthy, V. S. T., Böttcher, D., Engel, J., Kara, S., and Bornscheuer, U. T. (2020). A Whole-Cell Process for the Production of ε-caprolactone in Aqueous Media. Process Biochem. 88, 22–30. doi:10.1016/j.procbio.2019.10.009
Tokiwa, Y., Calabia, B., Ugwu, C., and Aiba, S. (2009). Biodegradability of Plastics. Int J Mol.Sci. 10, 3722–3742. doi:10.3390/ijms10093722
Van Sonsbeek, H. M., Beeftink, H. H., and Tramper, J. (1993). Two-liquid-phase Bioreactors. Enzyme Microb. Tech. 15, 722–729. doi:10.1016/0141-0229(93)90001-i
Volmer, J., Lindmeyer, M., Seipp, J., Schmid, A., and Bühler, B. (2019). Constitutively solvent‐tolerantPseudomonas taiwanensisVLB120∆C∆ttgVsupports Particularly High‐styrene Epoxidation Activities when Grown under Glucose Excess Conditions. Biotechnol. Bioeng. 116, 1089–1101. doi:10.1002/bit.26924
Volmer, J., Neumann, C., Bühler, B., and Schmid, A. (2014). Engineering of Pseudomonas Taiwanensis VLB120 for Constitutive Solvent Tolerance and Increased Specific Styrene Epoxidation Activity. Appl. Environ. Microbiol. 80, 6539–6548. doi:10.1128/aem.01940-14
Volmer, J. (2016). Strain and Process Engineering to Exploit Solvent Tolerance Mechanisms of Pseudomonas Taiwanensis VLB120 for Asymmetric Styrene Epoxidation. Aachen, Germany: Shaker Verlag.
Wang, F., Zhao, J., Li, Q., Yang, J., Li, R., Min, J., et al. (2020). One-pot Biocatalytic Route from Cycloalkanes to α, ω‐dicarboxylic Acids by Designed Escherichia coli Consortia. Nat. Commun. 11, 5035. doi:10.1038/s41467-020-18833-7
Willrodt, C., Karande, R., Schmid, A., and Julsing, M. K. (2015). Guiding Efficient Microbial Synthesis of Non-natural Chemicals by Physicochemical Properties of Reactants. Curr. Opin. Biotechnol. 35, 52–62. doi:10.1016/j.copbio.2015.03.010
Wittcoff, H. A., Reuben, B. G., and Plotkin, J. S. (2012). Industrial Organic Chemicals. Hoboken, NJ: John Wiley & Sons. doi:10.1002/9781118229996
Zhang, Y., Song, L., Gao, Q., Yu, S. M., Li, L., and Gao, N. F. (2012). The Two-step Biotransformation of Monosodium Glutamate to GABA by Lactobacillus Brevis Growing and Resting Cells. Appl. Microbiol. Biotechnol. 94, 1619–1627. doi:10.1007/s00253-012-3868-8
Keywords: biotransformation, whole-cell biocatalysis, cascade reaction, cyclohexane, reaction engineering, biodegradable plastics, PCL
Citation: Bretschneider L, Heuschkel I, Wegner M, Lindmeyer M, Bühler K, Karande R and Bühler B (2021) Conversion of Cyclohexane to 6-Hydroxyhexanoic Acid Using Recombinant Pseudomonas taiwanensis in a Stirred-Tank Bioreactor. Front. Catal. 1:683248. doi: 10.3389/fctls.2021.683248
Received: 20 March 2021; Accepted: 29 April 2021;
Published: 21 May 2021.
Edited by:
Wuyuan Zhang, Tianjin Institute of Industrial Biotechnology (CAS), ChinaReviewed by:
Gazi Sakir Hossain, National University of Singapore, SingaporeAitao Li, Hubei University, China
Changzhu Wu, University of Southern Denmark, Denmark
Copyright © 2021 Bretschneider, Heuschkel, Wegner, Lindmeyer, Bühler, Karande and Bühler. This is an open-access article distributed under the terms of the Creative Commons Attribution License (CC BY). The use, distribution or reproduction in other forums is permitted, provided the original author(s) and the copyright owner(s) are credited and that the original publication in this journal is cited, in accordance with accepted academic practice. No use, distribution or reproduction is permitted which does not comply with these terms.
*Correspondence: Rohan Karande, cm9oYW4ua2FyYW5kZUB1ZnouZGU=