- 1Department of Cardiovascular Rehabilitation, The Third Affiliated Hospital of Gansu University of Traditional Chinese Medicine, Baiyin, China
- 2Department of Endocrinology, The Third Affiliated Hospital of Gansu University of Traditional Chinese Medicine, Baiyin, China
- 3Cardiovascular Department, The Third Affiliated Hospital of Gansu University of Traditional Chinese Medicine, Baiyin, China
RNA therapeutics are emerging as a promising approach for cardiovascular diseases (CVDs) management, offering targeted gene regulation through modalities like mRNA, siRNA, and miRNA. In recent years, researchers have conducted a lot of research on the application of RNA therapeutics technology in the treatment of CVDs. Despite hurdles in off-target effects and immune responses, the clinical trial outcomes are encouraging. This review synthesizes the current progress in RNA therapeutics for CVDs, examining their mechanisms, advantages, and challenges in delivery and safety. We highlight the potential of personalized medicine, combination artificial intelligence (AI) and bioinformatics in advancing RNA therapeutics. The future of RNA therapeutics in CVDs is poised for significant impact, necessitating continued research and interdisciplinary collaboration to optimize these treatments and ensure patient safety and efficacy.
1 Introduction
Cardiovascular diseases (CVDs) encompass a range of disorders affecting the heart and blood vessels, including coronary artery disease, stroke, heart failure, and hypertension (1). According to the World Health Organization, CVDs are the leading cause of death globally, claiming approximately 17.9 million lives each year, which accounts for 32% of all global deaths (2). Notably, ischemic heart disease and stroke represent the most prevalent forms of CVDs, with the Global Burden of Disease Study reporting that ischemic heart disease alone accounted for 8.9 million deaths in 2019 (3). The prevalence of CVDs is expected to rise, particularly in low- and middle-income countries due to aging populations, lifestyle changes, and increasing rates of obesity and diabetes (4).
The global burden of CVDs goes beyond just mortality; it also has a considerable impact on healthcare systems and economies around the world (5). According to the American Heart Association, direct medical costs and indirect productivity losses attributed to CVDs exceeded $200 billion annually in the United States alone (6). Disparities in CVDs outcomes are evident, with variations in incidence and mortality rates among different populations attributed to factors such as access to healthcare, socioeconomic status, and lifestyle choices (7). For instance, studies demonstrate that individuals with low socioeconomic status are at a heightened risk for CVDs, with mortality rates up to three times higher compared to their higher-income counterparts (8). This variability highlights the need for targeted interventions and public health strategies to effectively manage and mitigate CVDs risk, particularly in disadvantaged populations.
RNA therapeutics have emerged as a groundbreaking approach in modern medicine, leveraging the potential of RNA molecules to modulate gene expression and protein synthesis (9). These therapeutics encompass a variety of modalities, including small interfering RNA (siRNA), messenger RNA (mRNA), microRNA (miRNA), and antisense oligonucleotides (ASOs) (9). The success of mRNA vaccines against COVID-19, such as those developed by Pfizer-BioNTech and Moderna, has demonstrated the power of RNA technology to elicit robust immune responses and has ushered in a new era of rapid vaccine development (10). Furthermore, RNA therapeutics can be designed to target specific pathways involved in various diseases, providing a tailored treatment method that offers advantages over traditional small-molecule drugs, particularly in terms of specificity and reduced off-target effects (11).
In the context of CVDs, preclinical trials have shown promising results. For instance, siRNA targeting PCSK9 has been effective in reducing LDL cholesterol levels in animal models (12). Similarly, mRNA encoding for vascular endothelial growth factor (VEGF) has demonstrated potential in promoting angiogenesis and improving myocardial perfusion in preclinical studies (13, 14). The therapeutic use of miRNA involves either inhibiting the activity of pathologically upregulated miRNAs or replacing downregulated protective miRNAs (15). For example, let-7 family inhibition has demonstrated beneficial effects on cardiac remodeling following injury (16). ASOs have also shown potential in preclinical trials, with studies demonstrating their ability to target specific genes involved in lipid metabolism and inflammatory pathways (17).
The objective of this review is to comprehensively summarize the current state of RNA-based therapies in the management of CVDs, highlighting recent advancements, ongoing clinical trials, and emerging strategies. We aim to critically evaluate the efficacy and safety of these therapies, discussing variability in study outcomes and the underlying factors that contribute to these differences. By synthesizing and contextualizing these findings, this review will provide valuable insights into the potential of RNA therapeutics as transformative approaches in CVDs management, paving the way for future research and clinical application.
2 RNA therapeutics
In recent years, RNA therapeutics have emerged as a promising modality in the treatment of CVDs (18). With the capability to directly modulate gene expression and cellular processes, RNA-based approaches provide novel avenues for intervention in the complex cellular networks that underlie cardiovascular pathologies (19). The main types of RNA therapeutics include mRNA (20), siRNA (21), microRNA (miRNA) (15), and RNA aptamers (22) (Figure 1). They act on diseases through mechanisms of action such as gene silencing (23), gene replacement (24) and modulation of gene expression (25), and have advantages in the treatment of CVDs (26).
2.1 Types of RNA therapeutics
mRNA serves as a template for protein synthesis and has garnered significant attention in the development of RNA therapeutics (20). The advent of lipid nanoparticles (LNPs) technology has enabled efficient delivery of mRNA vaccines, as witnessed during the COVID-19 pandemic (20). For CVDs, mRNA therapy holds the potential for gene replacement strategies, where mRNA encoding for cardioprotective factors, such as VEGF or angiopoietins, can be introduced to enhance myocardial repair after ischemic injury (27). However, the challenge of ensuring sufficient mRNA stability and avoiding unwanted immune responses remains a critical consideration (28). Future research must critically assess the long-term efficacy and safety profiles of mRNA therapies in diverse CVDs contexts.
siRNA mediates gene silencing through the RNA interference (RNAi) pathway. siRNA can specifically target and degrade mRNA transcripts of genes implicated in CVDs, such as those involved in lipid metabolism or inflammatory pathways (21). Notably, the siRNA drug, Patisiran, which targets transthyretin mRNA, has shown success in treating hereditary transthyretin amyloidosis (29). While the precision of siRNA offers significant therapeutic prospects, challenges such as off-target effects, delivery methods, and the potential for immune responses need careful scrutiny (30). Comprehensive studies comparing the effectiveness of siRNA therapies across various CVDs models will elucidate their true therapeutic potential.
miRNA are short, non-coding RNA molecules that play a critical role in post-transcriptional regulation of gene expression (31). Dysregulation of specific miRNAs has been implicated in numerous cardiovascular disorders, including heart failure and atherosclerosis (32). The therapeutic use of miRNAs involves either inhibiting the activity of pathologically upregulated miRNAs or replacing downregulated protective miRNAs (15). For instance, let-7 family inhibition has demonstrated beneficial effects on cardiac remodeling following injury (16). Despite their potential, the use of miRNAs as therapeutics necessitates rigorous evaluation (33). The interplay of different miRNAs within cellular networks can lead to complex outcomes that warrant careful interpretation of preclinical findings, ensuring translational relevance to human pathology (33).
RNA aptamers are short, single-stranded RNA molecules that can bind to specific target proteins with high affinity and specificity (22). Their unique ability to inhibit protein functions offers therapeutic promise for diseases characterized by dysregulated protein interactions (22). For example, RNA aptamers targeting platelet-derived growth factor are being investigated for their role in inhibiting vascular smooth muscle cell proliferation, a key event in atherosclerosis (34). However, the clinical application of RNA aptamers is still in its infancy, requiring comprehensive evaluation of their pharmacodynamics, stability, and delivery mechanisms (35). Effective strategies to enhance their systemic availability and reduce renal clearance are pivotal to realizing their therapeutic potential (35).
2.2 Mechanisms of action
RNA therapeutics have emerged as a promising frontier in the treatment of CVDs. They offer precise and potent interventions by targeting specific molecular pathways involved in the pathogenesis of CVDs. RNA therapeutics exert their effects through distinct mechanisms depending on their type, providing targeted interventions for CVDs.
2.2.1 siRNA mechanism
siRNA operates via the RNA interference (RNAi) pathway, a conserved biological process for sequence-specific gene silencing (30). Mechanistically, exogenous double-stranded siRNA is processed by the cytoplasmic enzyme Dicer into 21–23 nucleotide duplexes (21). One strand (the guide strand) is incorporated into the RNA-induced silencing complex (RISC), where it directs the complex to complementary mRNA sequences (30). Upon binding, the Argonaute 2 (AGO2) component of RISC cleaves the target mRNA, leading to its degradation and subsequent suppression of protein synthesis (25). For example, siRNA targeting PCSK9 binds to its mRNA with perfect complementarity, enabling RISC-mediated cleavage. This prevents PCSK9 protein synthesis, stabilizing hepatic LDL receptors and enhancing cholesterol clearance (36). The specificity of siRNA hinges on base-pairing fidelity, though off-target binding to partially complementary mRNAs remains a concern, necessitating rigorous bioinformatic design.
2.2.2 mRNA mechanism
mRNA therapeutics function through a gene replacement strategy. Synthetic mRNA, engineered with modified nucleotides (e.g., pseudouridine) to evade immune detection, is delivered into the cytoplasm via LNPs (37). The mRNA bypasses nuclear entry and directly engages ribosomes for translation into functional proteins. For instance, VEGF-A mRNA is translated into vascular endothelial growth factor, which binds to endothelial cell receptors (VEGFR1/2), activating the PI3K-Akt and MAPK-ERK pathways to promote angiogenesis (38). Key challenges include optimizing codon usage for enhanced translational efficiency and incorporating untranslated regions to regulate stability (39). Recent advances in nucleoside modifications (e.g., 5-methoxyuridine) further prolong mRNA half-life by reducing RNase recognition (40).
2.2.3 miRNA mechanism
miRNAs are endogenous, single-stranded RNAs that regulate gene expression post-transcriptionally (41). Therapeutic strategies involve either inhibiting overexpressed miRNAs or supplementing deficient miRNAs. miRNAs bind to partially complementary sequences in the 3′ untranslated region of target mRNAs via the miRNA-induced silencing complex (41). This interaction typically represses translation or recruits deadenylases and decapping enzymes to degrade the mRNA. For example, inhibition of the let-7 family prevents its binding to pro-fibrotic transcripts (e.g., TGF-βR1), thereby attenuating cardiac fibrosis (42). Conversely, miR-21 mimics can suppress apoptotic pathways by targeting PDCD4 and PTEN (43). The pleiotropic nature of miRNAs—each regulating hundreds of transcripts—requires careful validation to avoid unintended network-wide effects (41).
2.2.4 RNA aptamer mechanism
RNA aptamers are structured, single-stranded RNAs that bind to specific proteins with high affinity through conformational complementarity (44). Their mechanism involves blocking active sites, disrupting protein-protein interactions, or altering protein conformation. For instance, aptamers targeting platelet-derived growth factor (PDGF) adopt a G-quadruplex structure that sterically hinders PDGF binding to its receptor, inhibiting vascular smooth muscle cell proliferation (45). Aptamers can also be engineered with chemical modifications (e.g., 2′-fluoro pyrimidines) to enhance nuclease resistance. Unlike antibodies, aptamers lack immunogenicity and can be thermally renatured, offering advantages in storage and delivery (46).
The diverse mechanisms of RNA therapeutics highlight their great potential in combating CVDs. As research progresses, these therapies are expected to bring more tailored and effective treatment options for patients, opening up new horizons in the field of cardiovascular medicine.
2.3 Advantages of RNA therapeutics in CVDs
RNA therapeutics represent a groundbreaking advancement in the treatment of CVDs, offering a variety of mechanisms to address the complexities associated with these conditions (19). One prominent advantage lies in their capacity for precise gene regulation (Table 1). Techniques such as siRNA and ASOs enable targeted silencing of specific genes implicated in the pathology of CVDs (47, 48). For example, studies have shown that targeting angiotensinogen via siRNA reduces blood pressure and mitigates cardiac hypertrophy in animal models, highlighting the potential of RNA-based approaches to manage hypertension (49). This selective targeting minimizes off-target effects and maximizes therapeutic efficiency, a significant improvement over conventional therapies, which often exhibit broader systemic effects and subsequent side effects (50).
Another advantage of RNA therapeutics is their flexibility in design and rapid development. Using advances in RNA synthesis and delivery systems, researchers can quickly adapt RNA-based therapies in response to emerging disease mechanisms or evolving patient profiles (Table 1) (51). Recent research has illustrated this adaptability; for instance, mRNA vaccines have been rapidly repurposed for addressing viral infections, showcasing a paradigm that can be applied to CVDs for delivering therapeutic factors, such as endothelial nitric oxide synthase (eNOS) mRNA to enhance endothelial function (52). The rapid translation of these technologies from laboratory research to clinical applications could provide timely and effective interventions for patients with acute cardiovascular conditions.
Moreover, RNA therapeutics foster the possibility of personalized medicine (53) (Table 1). The heterogeneity of CVDs necessitates the development of tailored therapies that consider individual genetic backgrounds, comorbidities, and specific disease presentations (54). RNA-based approaches, particularly when integrated with genomic data, enable clinicians to select optimal therapies for individual patients (55). For instance, genetic profiling to identify patients with specific mutations in target genes may allow for the design of personalized ASOs that effectively modulate these genetic anomalies (53). Such personalized strategies could enhance therapeutic outcomes, as exemplified by the clinical trials involving the ASO mipomersen, which demonstrated significant reductions in low-density lipoprotein (LDL) levels in patients with FH (56). However, while the promise of custom-tailored therapies is evident, challenges surrounding individual variability in response to RNA therapies and the ethical implications of genetic testing need to be carefully navigated (18).
Finally, RNA therapeutics exhibit a potential for sustained effects through innovative delivery methods, such as LNPs and exosomes, which can enhance the bioavailability and stability of RNA molecules in circulation (Table 1) (57). This approach not only extends the therapeutic window but could also reduce the frequency of dosing, improving patient adherence to treatment regimens (58). For example, studies have demonstrated that LNPs can effectively deliver RNA payloads to target tissues, resulting in sustained gene silencing over extended periods (59–61). However, while the initial results are encouraging, it is imperative to critically evaluate the long-term safety and efficacy of these delivery systems, as concerns regarding immunogenicity and potential systemic toxicity must be addressed in ongoing and future trials (62).
3 RNA therapeutics in CVDs
RNA therapeutics have emerged as a promising strategy in the management of various CVDs leveraging their ability to modulate gene expression at the transcriptional or translational level. The clinical research landscape surrounding these therapies has rapidly evolved, showcasing their potential in targeting lipid metabolism, improving cardiac function, and addressing a range of genetic disorders (Table 2). Key milestones in the clinical development of RNA therapeutics include the first clinical trial of mipomersen for homozygous familial hypercholesterolemia (FH) in 2010, which demonstrated its potential to lower LDL cholesterol levels (63). Subsequently, in 2015, long-term data from mipomersen treatment in patients with FH confirmed its sustained efficacy and safety profile (64). In 2017, inclisiran, an siRNA therapeutic targeting PCSK9, emerged as a promising agent for lipid management, showing significant reductions in LDL cholesterol levels in patients at high cardiovascular risk (65). Building on these findings, the ORION-3 trial in 2020 provided further evidence of inclisiran's long-term efficacy and safety in reducing LDL cholesterol (66). Most recently, in 2022, olpasiran, an siRNA targeting lipoprotein(a), demonstrated a significant reduction in Lp(a) levels in patients with atherosclerotic cardiovascular disease, marking a potential breakthrough in managing this challenging risk factor (67) (Figure 2).
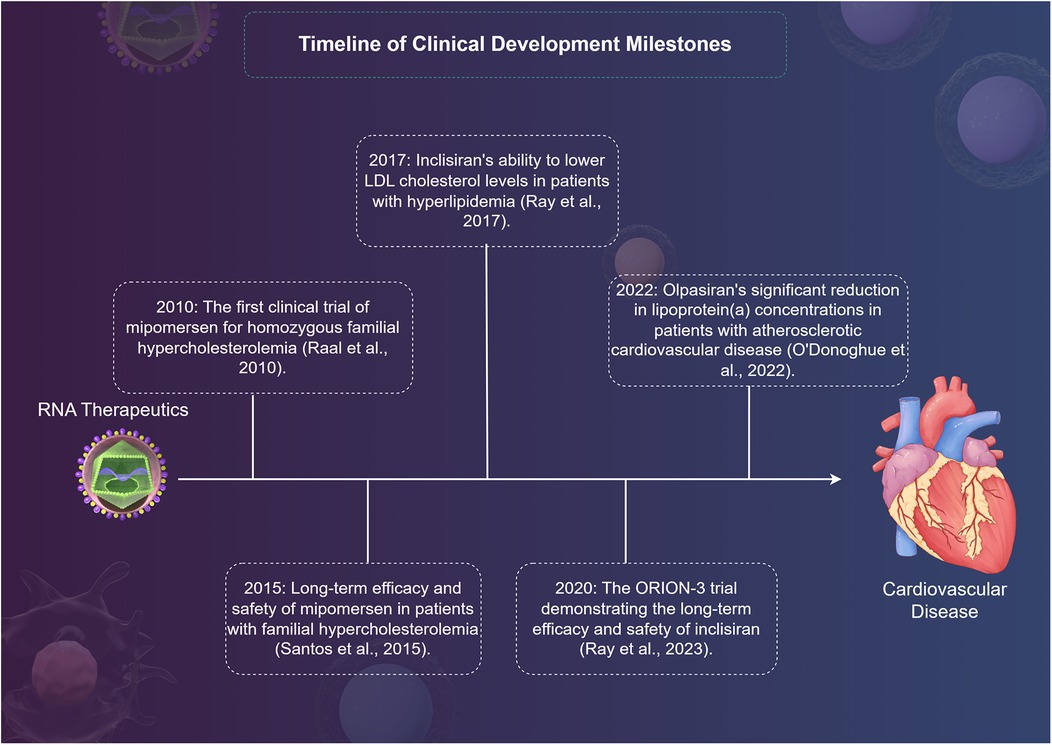
Figure 2. A timeline of clinical development milestones for RNA therapy in cardiovascular disease by Figdraw.
3.1 Hypertension
Hypertension, a major risk factor for cardiovascular diseases (CVDs), has emerged as a promising target for RNA therapeutics. Recent studies have shown that RNA interference (RNAi) can be used to silence genes involved in blood pressure regulation, offering a novel approach to hypertension management. RNAi-based therapies target specific genes involved in the renin-angiotensin system, a key pathway in blood pressure regulation. For example, small interfering RNA (siRNA) targeting angiotensinogen, a key component of the RAS, has demonstrated significant blood pressure-lowering effects in preclinical models (68). By silencing the angiotensinogen gene, RNAi therapies can reduce the production of angiotensin II, a potent vasoconstrictor, thereby lowering blood pressure.
Recent clinical trials have shown promising results. For instance, zilebesiran, an siRNA therapeutic targeting angiotensinogen, has been evaluated in the KARDIA-1 trial, demonstrating significant blood pressure reductions in patients with mild to moderate hypertension (69). The KARDIA-2 trial further confirmed the efficacy and safety of zilebesiran as an add-on therapy in patients with inadequately controlled hypertension (70). These studies highlight the potential of RNAi-based therapies to provide long-lasting blood pressure control with minimal side effects.
The development of RNA therapeutics for hypertension is still in its early stages, but the initial results are encouraging. Future research should focus on optimizing delivery methods to enhance the efficiency and safety of RNAi therapies. Additionally, long-term studies are needed to evaluate the sustained effects and potential side effects of these treatments in diverse patient populations.
3.2 Hypercholesterolemia
Hypercholesterolemia, particularly FH, has been a primary target for RNA-based therapies due to the associated cardiovascular risks. Mipomersen, an antisense oligonucleotide targeting apolipoprotein B, has demonstrated significant efficacy in reducing LDL cholesterol levels in patients with homozygous FH (63). It was approved by the U.S. Food and Drug Administration (FDA) in 2012 for this indication. However, the European Medicines Agency (EMA) rejected its marketing authorization application in 2013 due to concerns regarding its safety profile and the clinical benefit-risk ratio (64). Despite the approval in the US, ongoing monitoring of its long-term safety and efficacy is essential, as highlighted by the results from the open-label extension study (64). In a long-term study by Santos et al. (64), mipomersen treatment for 104 weeks reduced atherosclerotic lipoproteins and showed comparable safety. Despite these findings, the study's limitations include a relatively small sample size and potential selection bias, which may affect the generalizability of the results. While studies highlight the effectiveness of mipomersen in lipid lowering efficacy, particularly in different genetic backgrounds, warrant critical evaluation. For instance, while mipomersen's lipoprotein effects are well-documented, real-world effectiveness in diverse populations may vary (71). These differences underscore the need for careful patient selection and continuous monitoring in clinical practice.
Inclisiran, a siRNA that targets proprotein convertase subtilisin/kexin Type 9(PCSK9), shows significant promise as an effective therapy for lowering LDL cholesterol levels, particularly in patients with FH. The ORION-4 trial illustrated inclisiran's ability to maintain lower cholesterol levels through biannual dosing, emphasizing its ease of administration and potential for long-term adherence (65). Additionally, long-term efficacy reported in the open-label extension 3 (ORION-3) reinforces inclisiran's potential for sustained lipid management in high-risk populations (66). However, the trial's limitations include the potential for bias due to the open-label extension phase, which may influence patient and physician behavior. Additionally, long-term safety data are still limited, highlighting the need for further longitudinal studies to fully assess its safety profile.
Moreover, the introduction of volanesorsen, a novel antisense drug targeting apolipoprotein C-III (APOC3), has gained attention. A study revealed its ability to significantly lower triglycerides and atherogenic lipoproteins, positioning it as a valuable addition to the therapeutic arsenal against CVDs exacerbated by hypertriglyceridemia (72). It was approved by the EMA in 2018 for the treatment of patients with homozygous familial chylomicronemia syndrome (72). Conversely, it was rejected by the FDA in 2019 due to concerns regarding its safety and efficacy, particularly in relation to injection-site reactions and neurological adverse events (72). This discrepancy in regulatory decisions highlights the ongoing debate about the risk-benefit profile of volanesorsen and the need for further research to address these concerns.
3.3 Lipoprotein(a) management
Lipoprotein(a) [Lp(a)] is increasingly recognized as a significant independent risk factor for cardiovascular events. Disruption of Lp(a) synthesis presents a novel therapeutic avenue. A study exploring olpasiran, a siRNA that reduces Lp(a) levels, exhibited promising outcomes in lowering Lp(a) cholesterol and correcting LDL cholesterol levels (67). O'Donoghue et al. (67) reported that olpasiran significantly reduced Lp(a) cholesterol levels. However, the study's limitations include a short follow-up period, which may not fully capture long-term efficacy and safety outcomes. Furthermore, the trial's population was predominantly Caucasian, raising questions about the generalizability of the results to diverse populations. Koren et al. (73) also demonstrated the effective preclinical and clinical development of siRNA-targeting strategies against Lp(a), reinforcing the therapeutic prospect of RNA molecules in mitigating cardiovascular risks tied to elevated Lp(a) levels. These studies depict a burgeoning field that targets previously hard-to-manage lipid fractions, indicating the promising trajectory of RNA therapeutics in CVDs.
Despite these advancements, variability exists in the response to Lp(a) lowering therapies across studies and populations. Factors such as genetic polymorphisms and concurrent metabolic conditions can influence individual responses to RNA therapeutics. Hence, critically assessing patient backgrounds and potential biomarkers prior to treatment initiation remains essential.
3.4 Heart failure
Heart failure remains an area burdened by high morbidity and mortality rates. RNA-based therapies hold promise in addressing underlying mechanisms of cardiac dysfunction. Serelaxin, a recombinant form of relaxin acquired via mRNA technology, was evaluated in patients with acute heart failure. Teerlink et al. (74) verified that serelaxin treatment contributed positively to heart failure outcomes by improving clinical symptoms and inflammatory parameters. However, the study's limitations include a relatively small sample size and the lack of a long-term follow-up period to assess sustained benefits. Additionally, Gan et al. (13) showcased the potential of intradermal delivery of modified mRNA encoding VEGF-A, which promoted angiogenesis in diabetic patients undergoing procedures like coronary artery bypass grafting. Here, RNA therapeutics not only treat existing conditions but may also foster regenerative capabilities in cardiovascular tissues.
One of the pioneering studies involved targeting microRNA-132, which is associated with cardiac hypertrophy and remodeling. Täubel et al. (75) demonstrated that antisense therapy against microRNA-132 was safe and well-tolerated without significant adverse effects, indicating its potential as a novel therapeutic strategy in HF. Additionally, the application of CDR132l has been evaluated in patients following myocardial infarction with reduced ejection fraction (76). The HF-REVERT trial aims to determine its efficacy in improving functional outcomes and cardiac remodeling. Initial results appear promising, yet long-term effects and comprehensive safety profiles are still under investigation. The trial's limitations include the potential for bias due to the single-center design and the need for larger, multicenter studies to confirm the findings.
3.5 Vascular and inflammatory conditions
Emerging RNA therapies, such as those targeting angiopoietin-like proteins, have demonstrated capabilities in altering lipid and lipoprotein profiles in relevant inflammatory conditions (77). Therapies targeting angiopoietin-like protein 3 (ANGPTL3) with arcturus RNAi oligonucleotide-angiopoietin-like protein 3(ARO-ANG3) present a novel approach in managing abnormal lipid levels, although more extensive trials are warranted to illustrate disease-modifying effects across broader patient demographics (77). Moreover, therapeutic angiogenesis may enhance outcomes for coronary artery disease patients undergoing surgery (14). The ongoing EPICCURE trial investigates the safety of AZD8601, a vascular endothelial growth factor-A165 (VEGF-A165) mRNA, administered via epicardial injections during revascularization. The study aims to evaluate safety and exploratory efficacy through various imaging and assessment methods (14). The ongoing exploration of RNA strategies underscores their potential across various pathophysiological processes tied to CVDs.
The landscape of RNA therapeutics for CVDs management reflects significant promise, with clinical trials providing essential insights into their efficacy and safety (19). The variety of approaches leveraging RNA technology illustrates its adaptability and innovation in targeting specific disease mechanisms (9). Nonetheless, consistency in outcomes, long-term safety, and comparative effectiveness with existing therapies must guide future research. A comprehensive understanding of patient-specific factors will be essential in optimizing these advanced therapeutic modalities and achieving better clinical outcomes in CVDs. As the field continues to evolve, thorough evaluation of ongoing and future studies will be essential in establishing RNA therapeutics as a mainstay in CVDs management.
3.6 Thrombosis and fibrinolysis
Thrombosis and fibrinolysis play crucial roles in the pathogenesis of CVDs, particularly in conditions such as myocardial infarction and stroke. Targeting these pathways offers a promising avenue for therapeutic intervention. RNA therapeutics have shown potential in modulating thrombosis and fibrinolysis through various mechanisms.While the research on RNA therapeutics targeting thrombosis and fibrinolysis is still in its early stages, several preclinical studies have demonstrated their potential. For example, a preclinical study of an siRNA therapeutic targeting TF showed promising results in terms of safety and efficacy (68). Future research should focus on optimizing delivery methods, improving stability, and evaluating long-term safety and efficacy in larger clinical trials.
4 Safety and efficacy of RNA therapeutics
RNA therapeutics have shown varying success in clinical trials for cardiovascular conditions, with some trials achieving significant improvements in patient outcomes, while others have revealed limitations in efficacy (18). Safety concerns arise, particularly regarding off-target effects and immune responses. Understanding these factors is crucial for optimizing treatment protocols and ensuring the long-term success of RNA-based therapies.
4.1 Overview of clinical trial results
Clinical trials evaluating RNA therapeutics for CVDs have demonstrated both promise and variability in outcomes. For instance, inclisiran, an siRNA targeting PCSK9, achieved sustained LDL cholesterol reductions with biannual dosing in the ORION-4 trial, highlighting its potential for long-term lipid management (65). Conversely, early trials of mRNA encoding SERCA2a for heart failure showed transient improvements in left ventricular function but failed to demonstrate durable benefits in larger cohorts, underscoring challenges in achieving consistent therapeutic effects (80, 81). Similarly, while siRNA-based therapies like zilebesiran (targeting angiotensinogen) significantly lowered blood pressure in hypertensive patients (69, 70), trials of miRNA-132 inhibitors (e.g., CDR132l) reported mixed results, with modest effects on cardiac remodeling (75, 76). These discrepancies emphasize the need to address variability in patient selection, dosing regimens, and delivery efficiency. Recent meta-analyses further suggest that RNA therapeutics exhibit higher efficacy in genetically defined subgroups (e.g., familial hypercholesterolemia) compared to heterogeneous populations, necessitating precision medicine approaches (82).
4.2 Safety profiles of different RNA modalities
4.2.1 Off-target effects
One of the critical safety concerns associated with RNA therapeutics is the potential for off-target effects (83). RNA molecules, especially siRNAs and miRNAs, can interact with unintended mRNAs, leading to unanticipated biological consequences (84). For example, the administration of siRNAs can inadvertently downregulate essential genes, which can result in detrimental effects (85). For example, early siRNA candidates targeting apolipoprotein B (mipomersen) were associated with hepatic steatosis, likely due to off-target suppression of lipid metabolism genes (63, 71). Similarly, miR-122 inhibitors, while effective in reducing hepatitis C viral load, inadvertently disrupted cholesterol homeostasis, leading to dyslipidemia (86). Advances in bioinformatic design tools (e.g., machine learning algorithms for predicting siRNA specificity) and chemical modifications (e.g., 2′-O-methylation) have mitigated these risks, as evidenced by newer siRNA therapies like olpasiran, which selectively lowers lipoprotein(a) without significant off-target activity (67, 73).
4.2.2 Immune responses
Another significant safety concern is the immune response to RNA therapeutics (87). Unmodified RNA has the potential to stimulate innate immune pathways, leading to inflammatory responses (88). Studies have shown that RNA-based therapies can trigger cytokine storms and other immune reactions, prompting a need for the development of modified RNA formats that minimize immunogenicity (89, 90). Recent advancements have introduced chemical modifications to mRNA to improve stability and reduce immune recognition (91). Modified nucleotides, such as 5-methylcytidine or 2ʹ-O-methylation, have been shown to dampen immune activation while enhancing translation efficiency (92). These modifications have achieved promising outcomes in several preclinical settings but necessitate careful monitoring in human studies to fully evaluate safety (93).
4.2.3 Organ-specific toxicity
Accumulation of RNA therapeutics in non-target tissues remains a concern. For example, LNPs preferentially localize to the liver but may deposit in the spleen or kidneys, raising risks of immune cell activation or renal toxicity (94). Preclinical studies of VEGF-A mRNA revealed edema in non-cardiac tissues due to systemic leakage, necessitating optimized delivery routes (e.g., localized epicardial injections in the EPICCURE trial) (14). Viral vectors, such as AAVs, pose risks of hepatotoxicity and neutralizing antibody formation, limiting their re-dosing potentia (95).
4.2.4 Long-term safety
Longitudinal data remain sparse. While mipomersen showed acceptable safety over 104 weeks in familial hypercholesterolemia (64), volanesorsen (targeting APOC3) was linked to thrombocytopenia and injection-site reactions, prompting FDA rejection despite EMA approval (72). Emerging platforms, such as exosome-mediated delivery, offer improved biocompatibility but require rigorous evaluation of biodistribution and chronic toxicity (96).
5 Advancements in RNA delivery systems for cardiovascular therapeutics
The effective use of RNA therapeutics for CVDs significantly depends on the performance of delivery systems (19). The distinct challenges posed by RNA molecules, including their natural instability, vulnerability to degradation, and relatively large size, require innovative strategies to guarantee their proper uptake in targeted tissues (18).
5.1 Novel delivery systems
5.1.1 Viral vectors
Viral vectors have long been utilized for gene therapy; however, their application in RNA therapeutics is gaining traction (97). Adeno-associated viruses (AAVs) and lentiviral vectors have shown promise due to their ability to facilitate sustained expression of the therapeutic RNA after delivery (88). These vectors can be engineered to exhibit tissue tropism, enhancing their delivery to specific cell types in the cardiovascular system (88) (Figure 3). Nevertheless, concerns regarding safety, such as immunogenic responses and insertional mutagenesis, limit their clinical applicability (95). The recent advent of less immunogenic variants of AAVs and the use of transient expression vectors may help mitigate such risks while advancing the therapeutic potential of RNA interventions (88).
5.1.2 Lipid nanoparticles
Lipid nanoparticles (LNPs) have emerged as one of the most promising platforms for RNA delivery due to their ability to encapsulate RNA molecules, enhance cellular uptake, and facilitate endosomal escape (94) (Figure 3). LNPs can be engineered to improve their pharmacokinetic properties and target specific tissues through modifications that alter surface charge, lipid composition, and size (98). Recent studies demonstrate that LNPs containing mRNA encoding proteins such as SERCA2a successfully ameliorate heart function in animal models of heart failure (99, 100). The versatility and scalability of LNPs systems make them a frontrunner in RNA delivery, however, ongoing challenges such as potential immunogenicity and stability during storage must be addressed (101).
5.1.3 Exosomes
Exosomes, small extracellular vesicles secreted by various cell types, have emerged as innovative delivery vehicles thanks to their natural ability to facilitate intercellular communication (96). Exosomes can encapsulate RNA molecules, providing inherent biocompatibility and reducing the likelihood of immune rejection (96) (Figure 3). Furthermore, their lipophilic membranes enhance their ability to penetrate target cells (96). Research has indicated that exosome-mediated delivery of miRNAs can significantly modulate pathological processes in CVDs, with promising results in preclinical models of atherosclerosis and heart failure (102, 103). However, optimizing the isolation, characterization, and loading of therapeutic RNA into exosomes remains an ongoing challenge that necessitates further investigation (104).
5.2 Innovations in delivery methods enhancing cardiovascular targeting
A multitude of recent innovations are focused on improving the targeted delivery of RNA therapeutics to the cardiovascular system (19). These include the development of ligand-targeted delivery systems, use of biodegradable polymers, and advancement in needle-free injection systems (19).
Ligand-targeted delivery leverages specific ligands that bind selectively to receptors overexpressed on cardiac or vascular cells (105). For instance, the conjugation of RNA constructs to antibodies or peptides that target inflammation markers has demonstrated enhanced uptake in diseased tissues (62). Moreover, biodegradable polymers represent a promising strategy for controlled and sustained release of RNA therapeutics (93). Such systems protect RNA from degradation while facilitating gradual release, enhancing therapeutic efficacy and minimizing the need for repetitive dosing (106). Finally, advancements in needle-free injection systems have improved the feasibility of delivering RNA therapeutics via intradermal or transdermal routes, promoting patient compliance (107). These innovations have the potential to simplify the delivery process while achieving greater concentration at the target site.
5.3 Comparative analysis and challenges of delivery methods
To provide a clear comparison of the various RNA delivery methods discussed, the following Table 3 summarizes their key features. This comparative analysis highlights the unique features and potential applications of each delivery method in the context of RNA therapeutics for CVDs. While LNPs and exosome-mediated delivery offer significant advantages in terms of biocompatibility and targeting potential, ongoing research is focused on addressing their respective challenges to optimize their performance in clinical settings.
The delivery of RNA therapeutics faces several critical challenges. First, RNA is prone to degradation by ribonucleases present in blood and tissues, which necessitates protective formulations to enhance its stability (105). Additionally, the hydrophilic nature of RNA molecules creates barriers to cellular uptake, limiting their bioavailability in the target tissues (105). Furthermore, the desired specificity of RNA delivery remains a significant hurdle. Effective targeting requires distinguishing between healthy tissues and sites of pathological relevance, such as ischemic myocardium or atherosclerotic plaques (108). Achieving this specificity minimizes off-target effects, which can lead to unwanted systemic consequences (108). Current delivery methods often struggle with these issues, highlighting the urgent need for refined strategies that can deliver RNA therapeutics efficiently and safely to the cardiovascular system.
6 Emerging strategies and future directions
The field of CVDs management is undergoing rapid transformation, with RNA therapeutics leading the way in innovative approaches (105). Emerging strategies are centered on personalized medicine, and the roles of artificial intelligence (AI) and bioinformatics, as well as anticipated future directions in clinical applications and ongoing research.
Emerging RNA therapies, such as those targeting angiopoietin-like proteins, have demonstrated capabilities in altering lipid and lipoprotein profiles in relevant inflammatory conditions (77). Therapies targeting ANGPTL3 with ARO-ANG3 present a novel approach in managing abnormal lipid levels, although more extensive trials are warranted to illustrate disease-modifying effects across broader patient demographics (77). A recent review comprehensively discusses several potential RNA therapeutics under development, emphasizing the diversity of targets and mechanisms being explored (109). These include novel siRNA and mRNA approaches aimed at modulating key pathways in vascular inflammation and atherosclerosis. The ongoing EPICCURE trial, investigating the safety of AZD8601 (a VEGF-A165 mRNA administered via epicardial injections during revascularization), exemplifies the translational potential of RNA strategies in improving outcomes for coronary artery disease patients (14). The integration of these innovative therapies underscores the dynamic landscape of RNA therapeutics in addressing unmet clinical needs in cardiovascular medicine.
Personalized medicine, which tailors treatment based on individual patient characteristics, is increasingly feasible with RNA technologies (53). Genomic profiling allows for the identification of specific genetic mutations and expression profiles that can be targeted effectively with RNA therapeutics (110). For instance, the stratification of patients based on the expression levels of relevant miRNAs has shown promise in predicting responses to treatments (111). Recent studies have demonstrated that employing patient-specific RNA profiles can guide bespoke therapies, such as tailored cancer immunotherapies (112), personalized gene editing for genetic disorders (113), and customized RNA vaccines for infectious diseases (114). However, the integration of personalized approaches remains challenging due to inconsistencies in RNA expression across populations and the complexity of biomarker identification (82). Hence, rigorous validation of RNA signatures in diverse cohorts is essential to optimize personalized treatment strategies and establish clinical relevance (82).
The integration of AI and bioinformatics into RNA therapeutic development is an exciting frontier with the potential to enhance numerous aspects of research and clinical application (115). AI algorithms can analyze large-scale genomic and transcriptomic datasets, identifying patterns and predicting responses to RNA therapeutics (84). For example, machine learning models have been used to discover novel miRNA targets and predict their interactions with specific mRNAs, accelerating the discovery of potential therapeutic candidates (116). Bioinformatics tools are equally crucial for understanding the complex regulatory networks governing RNA dynamics and their implications in disease processes (117). Nevertheless, challenges remain in validating AI predictions within biological contexts (118). A critical evaluation of AI-generated hypotheses through experimental validation is necessary to ensure their translational relevance and applicability in clinical settings (119). Establishing collaborative frameworks between computational scientists and cardiovascular researchers will be vital to leverage the full potential of these technologies (119).
Looking ahead, the future of RNA therapeutics in CVDs management appears promising yet complex (62). Future research must prioritize addressing the key challenges in delivery mechanisms, safety, and efficacy discussed in previous sections. The development of novel delivery vehicles that enhance tissue-targeting and minimize immune responses is critical for the successful translation of RNA-based therapies from bench to bedside. Additionally, continued efforts should focus on the refinement of regulatory frameworks to accommodate the unique challenges posed by RNA therapies, ensuring a robust pathway for clinical approval while maintaining patient safety (20). As the field evolves, the collaboration between academic, governmental, and industry stakeholders will be crucial in fostering an environment conducive to innovation. Finally, larger-scale clinical trials are warranted to assess the long-term efficacy and safety profiles of RNA therapeutics in diverse patient populations. Emphasis should also be placed on conducting comparative effectiveness research to ascertain the relative benefits of RNA modalities against existing therapies, thus providing clearer guidance for clinicians in decision-making processes.
circRNAs have emerged as promising biomarkers and therapeutic agents in various diseases, including CVDs. These non-coding RNAs are characterized by their covalently closed loop structures, which make them highly stable in biological samples. In CVDs, circRNAs have been identified as potential biomarkers and therapeutic targets. For instance, circRNA_0001946 has been shown to be significantly upregulated in patients with coronary artery disease and can be used as a potential biomarker for early diagnosis (120). Additionally, circRNA_000911 has been identified as a regulator of vascular smooth muscle cell proliferation and could serve as a therapeutic target for atherosclerosis (121). The integration of circRNA studies into RNA therapeutic research could provide new insights into the mechanisms of CVDs and offer novel therapeutic strategies. Future research should focus on further exploring the roles of circRNAs in CVDs and developing circRNA-based therapies to improve patient outcomes.
While personalized RNA therapies hold immense potential for tailored treatment, they also raise several ethical concerns that warrant careful consideration. One primary issue is the risk of genetic discrimination. As these therapies rely on genomic profiling, there is a possibility that patients' genetic information could be misused, leading to discrimination in employment or insurance (110). Ensuring patient confidentiality and establishing robust data protection policies are crucial to mitigate this risk. Another ethical challenge is the question of informed consent. Patients must be fully informed about the potential benefits and risks of personalized RNA therapies, including the uncertainties associated with long-term safety and efficacy (111). Efforts to make these therapies more affordable and accessible are necessary to ensure fair distribution of healthcare resources. Addressing these ethical issues is vital to the successful implementation of personalized RNA therapies in clinical practice. Future research should focus not only on the scientific and technical aspects but also on the development of ethical guidelines and policies to govern the use of these innovative treatments.
7 Conclusion
In summary, this review has demonstrated that RNA therapeutics represent a promising frontier in the management of CVDs, showing potential in personalized treatment approaches and combination therapies. The evidence highlights the significant advancements in RNA modalities, despite challenges such as safety profiles and delivery mechanisms. As these innovations evolve, continued research and collaboration across disciplines are essential to optimize therapeutic strategies and establish robust clinical applications. By fostering interdisciplinary partnerships, we can accelerate the translation of RNA-based interventions into clinical practice, ultimately improving patient outcomes in cardiovascular care.
Author contributions
WD: Funding acquisition, Validation, Writing – original draft, Writing – review & editing. ZX: Conceptualization, Software, Writing – review & editing. ZH: Conceptualization, Writing – review & editing. DL: Writing – review & editing. HW: Writing – review & editing. ZZ: Writing – review & editing. SJ: Data curation, Supervision, Writing – original draft, Writing – review & editing.
Funding
The author(s) declare that financial support was received for the research and/or publication of this article. Project category: “Gansu Science and Technology Plan Funded project”, project number: 24RCKD001, project name: Deep integration of medical and health care to promote the integration of clinical rehabilitation and promote the high-quality development of health services.
Acknowledgments
We would like to thank the Third Affiliated Hospital of Gansu University of Chinese Medicine and the Gansu Provincial Science and Technology Program for their support and facilitation of this review.
Conflict of interest
The authors declare that the research was conducted in the absence of any commercial or financial relationships that could be construed as a potential conflict of interest.
Generative AI statement
The author(s) declare that no Generative AI was used in the creation of this manuscript.
Publisher's note
All claims expressed in this article are solely those of the authors and do not necessarily represent those of their affiliated organizations, or those of the publisher, the editors and the reviewers. Any product that may be evaluated in this article, or claim that may be made by its manufacturer, is not guaranteed or endorsed by the publisher.
References
1. Goldsborough E 3rd, Osuji N, Blaha MJ. Assessment of cardiovascular disease risk: a 2022 update. Endocrinol Metab Clin North Am. (2022) 51(3):483–509. doi: 10.1016/j.ecl.2022.02.005
2. Sebastian SA. Cardiovascular disease risk communication: strategies, impact, and future directions. Curr Probl Cardiol. (2024) 49(5):102490. doi: 10.1016/j.cpcardiol.2024.102490
3. Saba L, Antignani PL, Gupta A, Cau R, Paraskevas KI, Poredos P, et al. International union of angiology (IUA) consensus paper on imaging strategies in atherosclerotic carotid artery imaging: from basic strategies to advanced approaches. Atherosclerosis. (2022) 354:23–40. doi: 10.1016/j.atherosclerosis.2022.06.1014
4. Denfeld QE, Turrise S, MacLaughlin EJ, Chang PS, Clair WK, Lewis EF, et al. Preventing and managing falls in adults with cardiovascular disease: a scientific statement from the American Heart Association. Circ Cardiovasc Qual Outcomes. (2022) 15(6):e000108. doi: 10.1161/hcq.0000000000000108
5. Leong DP, Joseph PG, McKee M, Anand SS, Teo KK, Schwalm JD, et al. Reducing the global burden of cardiovascular disease, Part 2: prevention and treatment of cardiovascular disease. Circ Res. (2017) 121(6):695–710. doi: 10.1161/circresaha.117.311849
6. Virani SS, Alonso A, Benjamin EJ, Bittencourt MS, Callaway CW, Carson AP, et al. Heart disease and stroke statistics-2020 update: a report from the American Heart Association. Circulation. (2020) 141(9):e139–596. doi: 10.1161/cir.0000000000000757
7. Raisi-Estabragh Z, Manisty CH, Cheng RK, Lopez Fernandez T, Mamas MA. Burden and prognostic impact of cardiovascular disease in patients with cancer. Heart. (2023) 109(24):1819–26. doi: 10.1136/heartjnl-2022-321324
8. Tousoulis D, Oikonomou E, Vogiatzi G, Vardas P. Cardiovascular disease and socioeconomic status. Eur Heart J. (2020) 41(34):3213–4. doi: 10.1093/eurheartj/ehaa405
10. Meo SA, Bukhari IA, Akram J, Meo AS, Klonoff DC. COVID-19 vaccines: comparison of biological, pharmacological characteristics and adverse effects of Pfizer/BioNTech and Moderna vaccines. Eur Rev Med Pharmacol Sci. (2021) 25(3):1663–9. doi: 10.26355/eurrev_202102_24877
11. Traber GM, Yu AM. RNAi-based therapeutics and novel RNA bioengineering technologies. J Pharmacol Exp Ther. (2023) 384(1):133–54. doi: 10.1124/jpet.122.001234
12. Rosenson RS, Gaudet D, Hegele RA, Ballantyne CM, Nicholls SJ, Lucas KJ, et al. Zodasiran, an RNAi therapeutic targeting ANGPTL3, for mixed hyperlipidemia. N Engl J Med. (2024) 391(10):913–25. doi: 10.1056/NEJMoa2404147
13. Gan LM, Lagerström-Fermér M, Carlsson LG, Arfvidsson C, Egnell AC, Rudvik A, et al. Intradermal delivery of modified mRNA encoding VEGF-A in patients with type 2 diabetes. Nat Commun. (2019) 10(1):871. doi: 10.1038/s41467-019-08852-4
14. Anttila V, Saraste A, Knuuti J, Jaakkola P, Hedman M, Svedlund S, et al. Synthetic mRNA encoding VEGF-A in patients undergoing coronary artery bypass grafting: design of a phase 2a clinical trial. Mol Ther Methods Clin Dev. (2020) 18:464–72. doi: 10.1016/j.omtm.2020.05.030
15. Diener C, Keller A, Meese E. Emerging concepts of miRNA therapeutics: from cells to clinic. Trends Genet. (2022) 38(6):613–26. doi: 10.1016/j.tig.2022.02.006
16. Bernstein DL, Jiang X, Rom S. let-7 microRNAs: their role in cerebral and cardiovascular diseases, inflammation, Cancer, and Their Regulation. Biomedicines. (2021) 9(6):606. doi: 10.3390/biomedicines9060606
17. Zou Y, Sun X, Wang Y, Yan C, Liu Y, Li J, et al. Single siRNA nanocapsules for effective siRNA brain delivery and glioblastoma treatment. Adv Mater. (2023) 35(16):e2300777. doi: 10.1002/adma.202300777
18. Lucas T, Dimmeler S. RNA Therapeutics for treatment of cardiovascular diseases: promises and challenges. Circ Res. (2016) 119(7):794–7. doi: 10.1161/circresaha.116.308730
19. Dzau VJ, Hodgkinson CP. RNA therapeutics for the cardiovascular system. Circulation. (2024) 149(9):707–16. doi: 10.1161/circulationaha.123.067373
20. Vervaeke P, Borgos SE, Sanders NN, Combes F. Regulatory guidelines and preclinical tools to study the biodistribution of RNA therapeutics. Adv Drug Deliv Rev. (2022) 184:114236. doi: 10.1016/j.addr.2022.114236
21. Bejar N, Tat TT, Kiss DL. RNA therapeutics: the next generation of drugs for cardiovascular diseases. Curr Atheroscler Rep. (2022) 24(5):307–21. doi: 10.1007/s11883-022-01007-9
22. Sundaram P, Kurniawan H, Byrne ME, Wower J. Therapeutic RNA aptamers in clinical trials. Eur J Pharm Sci. (2013) 48(1–2):259–71. doi: 10.1016/j.ejps.2012.10.014
24. Weng Y, Li C, Yang T, Hu B, Zhang M, Guo S, et al. The challenge and prospect of mRNA therapeutics landscape. Biotechnol Adv. (2020) 40:107534. doi: 10.1016/j.biotechadv.2020.107534
25. Lucas T, Bonauer A, Dimmeler S. RNA therapeutics in cardiovascular disease. Circ Res. (2018) 123(2):205–20. doi: 10.1161/circresaha.117.311311
26. Zampetaki A, Maegdefessel L. Editorial: Entering the RNA wonderland: opportunities and challenges for RNA therapeutics in the cardiovascular system. Front Physiol. (2020) 11:60. doi: 10.3389/fphys.2020.00060
27. Wang X, Wu DH, Senyo SE. mRNA therapy for myocardial infarction: a review of targets and delivery vehicles. Front Bioeng Biotechnol. (2022) 10:1037051. doi: 10.3389/fbioe.2022.1037051
28. Schlake T, Thran M, Fiedler K, Heidenreich R, Petsch B, Fotin-Mleczek M. mRNA: a novel avenue to antibody therapy? Mol Ther. (2019) 27(4):773–84. doi: 10.1016/j.ymthe.2019.03.002
29. Maurer MS, Kale P, Fontana M, Berk JL, Grogan M, Gustafsson F, et al. Patisiran treatment in patients with transthyretin cardiac amyloidosis. N Engl J Med. (2023) 389(17):1553–65. doi: 10.1056/NEJMoa2300757
30. Saw PE, Song EW. siRNA therapeutics: a clinical reality. Sci China Life Sci. (2020) 63(4):485–500. doi: 10.1007/s11427-018-9438-y
31. Lu TX, Rothenberg ME. MicroRNA. J Allergy Clin Immunol. (2018) 141(4):1202–7. doi: 10.1016/j.jaci.2017.08.034
32. Sessa F, Salerno M, Esposito M, Cocimano G, Pomara C. miRNA dysregulation in cardiovascular diseases: current opinion and future perspectives. Int J Mol Sci. (2023) 24(6):5192. doi: 10.3390/ijms24065192
33. Long J, Danesh FR. Promises and challenges of miRNA therapeutics. Am J Physiol Renal Physiol. (2022) 323(6):F673–f674. doi: 10.1152/ajprenal.00251.2022
34. Papadopoulos N, Lennartsson J. The PDGF/PDGFR pathway as a drug target. Mol Aspects Med. (2018) 62:75–88. doi: 10.1016/j.mam.2017.11.007
35. Zhou J, Rossi J. Aptamers as targeted therapeutics: current potential and challenges. Nat Rev Drug Discov. (2017) 16(3):181–202. doi: 10.1038/nrd.2016.199
36. Ray KK, Wright RS, Kallend D, Koenig W, Leiter LA, Raal FJ, et al. Two phase 3 trials of inclisiran in patients with elevated LDL cholesterol. N Engl J Med. (2020) 382(16):1507–19. doi: 10.1056/NEJMoa1912387
37. Qin S, Tang X, Chen Y, Chen K, Fan N, Xiao W, et al. mRNA-based therapeutics: powerful and versatile tools to combat diseases. Signal Transduction Targeted Ther. (2022) 7(1):166. doi: 10.1038/s41392-022-01007-w
38. He Y, Sun MM, Zhang GG, Yang J, Chen KS, Xu WW, et al. Targeting PI3K/Akt signal transduction for cancer therapy. Signal Transduction Targeted Ther. (2021) 6(1):425. doi: 10.1038/s41392-021-00828-5
39. Ma Q, Zhang X, Yang J, Li H, Hao Y, Feng X. Optimization of the 5ʹ untranslated region of mRNA vaccines. Sci Rep. (2024) 14(1):19845. doi: 10.1038/s41598-024-70792-x
40. Liu B, Pan T. Building better mRNA for therapeutics. Nat Biotechnol. (2024):1–3. doi: 10.1038/s41587-024-02424-8
41. Shang R, Lee S, Senavirathne G, Lai EC. microRNAs in action: biogenesis, function and regulation. Nat Rev Genet. (2023) 24(12):816–33. doi: 10.1038/s41576-023-00611-y
42. Kapplingattu SV, Bhattacharya S, Adlakha YK. MiRNAs as major players in brain health and disease: current knowledge and future perspectives. Cell Death Discov. (2025) 11(1):7. doi: 10.1038/s41420-024-02283-x
43. Feng M-G, Liu C-F, Chen L, Feng W-B, Liu M, Hai H, et al. MiR-21 attenuates apoptosis-triggered by amyloid-β via modulating PDCD4/PI3K/AKT/GSK-3β pathway in SH-SY5Y cells. Biomed Pharmacother. (2018) 101:1003–7. doi: 10.1016/j.biopha.2018.02.043
44. Fallah A, Imani Fooladi AA, Havaei SA, Mahboobi M, Sedighian H. Recent advances in aptamer discovery, modification and improving performance. Biochem Biophys Rep. (2024) 40:101852. doi: 10.1016/j.bbrep.2024.101852
45. Vu CQ, Rotkrua P, Soontornworajit B, Tantirungrotechai Y. Effect of PDGF-B aptamer on PDGFRβ/PDGF-B interaction: molecular dynamics study. J Mol Graphics Modell. (2018) 82:145–56. doi: 10.1016/j.jmgm.2018.04.012
46. Yuhan J, Zhu L, Zhu L, Huang K, He X, Xu W. Cell-specific aptamers as potential drugs in therapeutic applications: a review of current progress. J Controlled Release. (2022) 346:405–20. doi: 10.1016/j.jconrel.2022.04.039
47. Kulkarni JA, Witzigmann D, Thomson SB, Chen S, Leavitt BR, Cullis PR, et al. The current landscape of nucleic acid therapeutics. Nat Nanotechnol. (2021) 16(6):630–43. doi: 10.1038/s41565-021-00898-0
48. Damase TR, Sukhovershin R, Boada C, Taraballi F, Pettigrew RI, Cooke JP. The limitless future of RNA therapeutics. Front Bioeng Biotechnol. (2021) 9:628137. doi: 10.3389/fbioe.2021.628137
49. Uijl E, Mirabito Colafella KM, Sun Y, Ren L, van Veghel R, Garrelds IM, et al. Strong and sustained antihypertensive effect of small interfering RNA targeting liver angiotensinogen. Hypertension. (2019) 73(6):1249–57. doi: 10.1161/hypertensionaha.119.12703
50. Sarzani R, Spannella F, Di Pentima C, Giulietti F, Landolfo M, Allevi M. Molecular therapies in cardiovascular diseases: small interfering RNA in atherosclerosis, heart failure, and hypertension. Int J Mol Sci. (2023) 25(1):328. doi: 10.3390/ijms25010328
51. Cooke JP, Youker KA. Future impact of mRNA therapy on cardiovascular diseases. Methodist Debakey Cardiovasc J. (2022) 18(5):64–73. doi: 10.14797/mdcvj.1169
52. Kim B, Zhao W, Tang SY, Levin MG, Ibrahim A, Yang Y, et al. Endothelial lipid droplets suppress eNOS to link high fat consumption to blood pressure elevation. J Clin Invest. (2023) 133(24):e173160. doi: 10.1172/jci173160
53. Tani H. Recent advances and prospects in RNA drug development. Int J Mol Sci. (2024) 25(22):12284. doi: 10.3390/ijms252212284
54. Xu J, Guo J, Liu T, Yang C, Meng Z, Libby P, et al. Differential roles of eosinophils in cardiovascular disease. Nat Rev Cardiol. (2024) 21:1–18. doi: 10.1038/s41569-024-01071-5
55. Kaczmarek JC, Kowalski PS, Anderson DG. Advances in the delivery of RNA therapeutics: from concept to clinical reality. Genome Med. (2017) 9(1):60. doi: 10.1186/s13073-017-0450-0
56. Chen R, Lin S, Chen X. The promising novel therapies for familial hypercholesterolemia. J Clin Lab Anal. (2022) 36(7):e24552. doi: 10.1002/jcla.24552
57. Geary RS, Norris D, Yu R, Bennett CF. Pharmacokinetics, biodistribution and cell uptake of antisense oligonucleotides. Adv Drug Deliv Rev. (2015) 87:46–51. doi: 10.1016/j.addr.2015.01.008
58. Yin W, Rogge M. Targeting RNA: a transformative therapeutic strategy. Clin Transl Sci. (2019) 12(2):98–112. doi: 10.1111/cts.12624
59. Han X, Gong N, Xue L, Billingsley MM, El-Mayta R, Shepherd SJ, et al. Ligand-tethered lipid nanoparticles for targeted RNA delivery to treat liver fibrosis. Nat Commun. (2023) 14(1):75. doi: 10.1038/s41467-022-35637-z
60. Anthiya S, Öztürk SC, Yanik H, Tavukcuoglu E, Şahin A, Datta D, et al. Targeted siRNA lipid nanoparticles for the treatment of KRAS-mutant tumors. J Controlled Release. (2023) 357:67–83. doi: 10.1016/j.jconrel.2023.03.016
61. Dammes N, Goldsmith M, Ramishetti S, Dearling JLJ, Veiga N, Packard AB, et al. Conformation-sensitive targeting of lipid nanoparticles for RNA therapeutics. Nat Nanotechnol. (2021) 16(9):1030–8. doi: 10.1038/s41565-021-00928-x
62. Birgaoanu M, Sachse M, Gatsiou A. RNA Editing therapeutics: advances, challenges and perspectives on combating heart disease. Cardiovasc Drugs Ther. (2023) 37(2):401–11. doi: 10.1007/s10557-022-07391-3
63. Raal FJ, Santos RD, Blom DJ, Marais AD, Charng MJ, Cromwell WC, et al. Mipomersen, an apolipoprotein B synthesis inhibitor, for lowering of LDL cholesterol concentrations in patients with homozygous familial hypercholesterolaemia: a randomised, double-blind, placebo-controlled trial. Lancet. (2010) 375(9719):998–1006. doi: 10.1016/s0140-6736(10)60284-x
64. Santos RD, Duell PB, East C, Guyton JR, Moriarty PM, Chin W, et al. Long-term efficacy and safety of mipomersen in patients with familial hypercholesterolaemia: 2-year interim results of an open-label extension. Eur Heart J. (2015) 36(9):566–75. doi: 10.1093/eurheartj/eht549
65. Ray KK, Landmesser U, Leiter LA, Kallend D, Dufour R, Karakas M, et al. Inclisiran in patients at high cardiovascular risk with elevated LDL cholesterol. N Engl J Med. (2017) 376(15):1430–40. doi: 10.1056/NEJMoa1615758
66. Ray KK, Troquay RPT, Visseren FLJ, Leiter LA, Scott Wright R, Vikarunnessa S, et al. Long-term efficacy and safety of inclisiran in patients with high cardiovascular risk and elevated LDL cholesterol (ORION-3): results from the 4-year open-label extension of the ORION-1 trial. Lancet Diabetes Endocrinol. (2023) 11(2):109–19. doi: 10.1016/s2213-8587(22)00353-9
67. O'Donoghue ML, Rosenson RS, Gencer B, López JAG, Lepor NE, Baum SJ, et al. Small interfering RNA to reduce lipoprotein(a) in cardiovascular disease. N Engl J Med. (2022) 387(20):1855–64. doi: 10.1056/NEJMoa2211023
68. Mullick AE, Yeh ST, Graham MJ, Engelhardt JA, Prakash TP, Crooke RM. Blood pressure lowering and safety improvements with liver angiotensinogen inhibition in models of hypertension and kidney injury. Hypertension. (2017) 70(3):566–76. doi: 10.1161/hypertensionaha.117.09755
69. Desai AS, Webb DJ, Taubel J, Casey S, Cheng Y, Robbie GJ, et al. Zilebesiran, an RNA interference therapeutic agent for hypertension. N Engl J Med. (2023) 389(3):228–38. doi: 10.1056/NEJMoa2208391
70. Saxena M, Aswad A, Badariene J, Kazi F, Karns AD, Neutel JM, et al. Zilebesiran as add-on therapy in patients with hypertension inadequately controlled with a standard antihypertensive medication: efficacy and safety results from the kardia-2 study. J Hypertens. (2024) 42(Suppl 1):e115. doi: 10.1097/01.hjh.0001020464.05231.4a
71. Khan SA, Naz A, Qamar Masood M, Shah R. Meta-Analysis of inclisiran for the treatment of hypercholesterolemia. Am J Cardiol. (2020) 134:69–73. doi: 10.1016/j.amjcard.2020.08.018
72. Alexander VJ, Xia S, Hurh E, Hughes SG, O'Dea L, Geary RS, et al. N-acetyl galactosamine-conjugated antisense drug to APOC3 mRNA, triglycerides and atherogenic lipoprotein levels. Eur Heart J. (2019) 40(33):2785–96. doi: 10.1093/eurheartj/ehz209
73. Koren MJ, Moriarty PM, Baum SJ, Neutel J, Hernandez-Illas M, Weintraub HS, et al. Preclinical development and phase 1 trial of a novel siRNA targeting lipoprotein(a). Nat Med. (2022) 28(1):96–103. doi: 10.1038/s41591-021-01634-w
74. Teerlink JR, Voors AA, Ponikowski P, Pang PS, Greenberg BH, Filippatos G, et al. Serelaxin in addition to standard therapy in acute heart failure: rationale and design of the RELAX-AHF-2 study. Eur J Heart Fail. (2017) 19(6):800–9. doi: 10.1002/ejhf.830
75. Täubel J, Hauke W, Rump S, Viereck J, Batkai S, Poetzsch J, et al. Novel antisense therapy targeting microRNA-132 in patients with heart failure: results of a first-in-human phase 1b randomized, double-blind, placebo-controlled study. Eur Heart J. (2021) 42(2):178–88. doi: 10.1093/eurheartj/ehaa898
76. Bauersachs J, Solomon SD, Anker SD, Antorrena-Miranda I, Batkai S, Viereck J, et al. Efficacy and safety of CDR132l in patients with reduced left ventricular ejection fraction after myocardial infarction: rationale and design of the HF-REVERT trial. Eur J Heart Fail. (2024) 26(3):674–82. doi: 10.1002/ejhf.3139
77. Watts GF, Schwabe C, Scott R, Gladding P, Ballantyne C. RNAi inhibition of angiopoietin-like protein 3 (ANGPTL3) with ARO-ANG3 mimics the lipid and lipoprotein profile of familial combined hypolipidemia. Eur Heart J. (2020) 41(Supplement_2):ehaa946.3331. doi: 10.1093/ehjci/ehaa946.3331
78. Leiter LA, Teoh H, Kallend D, Wright RS, Landmesser U, Wijngaard PLJ, et al. Inclisiran lowers LDL-C and PCSK9 irrespective of diabetes Status: the ORION-1 randomized clinical trial. Diabetes Care. (2019) 42(1):173–6. doi: 10.2337/dc18-1491
79. Raal F, Abelson M, Blignaut S, Burgess L, Coetzer S, Ebrahim I, et al. Safety and efficacy of inclisiran in South African patients at high cardiovascular risk: a subanalysis of the ORION phase III clinical trials. S Afr Med J. (2022) 112(6):426–32. doi: 10.7196/SAMJ.2022.v112i6.16253
80. Greenberg B, Yaroshinsky A, Zsebo KM, Butler J, Felker GM, Voors AA, et al. Design of a phase 2b trial of intracoronary administration of AAV1/SERCA2a in patients with advanced heart failure: the CUPID 2 trial (calcium up-regulation by percutaneous administration of gene therapy in cardiac disease phase 2b). JACC Heart Fail. (2014) 2(1):84–92. doi: 10.1016/j.jchf.2013.09.008
81. Hulot JS, Salem JE, Redheuil A, Collet JP, Varnous S, Jourdain P, et al. Effect of intracoronary administration of AAV1/SERCA2a on ventricular remodelling in patients with advanced systolic heart failure: results from the AGENT-HF randomized phase 2 trial. Eur J Heart Fail. (2017) 19(11):1534–41. doi: 10.1002/ejhf.826
82. Winkle M, El-Daly SM, Fabbri M, Calin GA. Noncoding RNA therapeutics—challenges and potential solutions. Nat Rev Drug Discov. (2021) 20(8):629–51. doi: 10.1038/s41573-021-00219-z
83. Thatte AS, Billingsley MM, Weissman D, Melamed JR, Mitchell MJ. Emerging strategies for nanomedicine in autoimmunity. Adv Drug Deliv Rev. (2024) 207:115194. doi: 10.1016/j.addr.2024.115194
84. Bereczki Z, Benczik B, Balogh OM, Marton S, Puhl E, Pétervári M, et al. Mitigating off-target effects of small RNAs: conventional approaches, network theory and artificial intelligence. Br J Pharmacol. (2024) 182(2):340–79. doi: 10.1111/bph.17302
85. Garbo S, Maione R, Tripodi M, Battistelli C, Therapeutics NR. The mine of non-coding. Int J Mol Sci. (2022) 23(13):7471. doi: 10.3390/ijms23137471
86. López-Longarela B, Morrison EE, Tranter JD, Chahman-Vos L, Léonard JF, Gautier JC, et al. Direct detection of miR-122 in hepatotoxicity using dynamic chemical labeling overcomes stability and isomiR challenges. Anal Chem. (2020) 92(4):3388–95. doi: 10.1021/acs.analchem.9b05449
87. Paunovska K, Loughrey D, Dahlman JE. Drug delivery systems for RNA therapeutics. Nat Rev Genet. (2022) 23(5):265–80. doi: 10.1038/s41576-021-00439-4
88. Zhu H, Luo H, Chang R, Yang Y, Liu D, Ji Y, et al. Protein-based delivery systems for RNA delivery. J Controlled Release. (2023) 363:253–74. doi: 10.1016/j.jconrel.2023.09.032
89. Sioud M. How the initial discovery of modified RNA enabled evasion of innate immune responses and facilitated the development of RNA therapeutics. Scand J Immunol. (2023) 98(1):e13282. doi: 10.1111/sji.13282
90. Agrawal S. Considerations for creating the next generation of RNA therapeutics: oligonucleotide chemistry and innate immune responses to nucleic acids. Nucleic Acid Ther. (2024) 34(2):37–51. doi: 10.1089/nat.2024.29009.sud
91. Son S, Lee K. Development of mRNA vaccines/therapeutics and their delivery system. Mol Cells. (2023) 46(1):41–7. doi: 10.14348/molcells.2023.2165
92. Ehret F, Zhou CY, Alexander SC, Zhang D, Devaraj NK. Site-Specific covalent conjugation of modified mRNA by tRNA guanine transglycosylase. Mol Pharm. (2018) 15(3):737–42. doi: 10.1021/acs.molpharmaceut.7b00356
93. Uchida S, Lau CYJ, Oba M, Miyata K. Polyplex designs for improving the stability and safety of RNA therapeutics. Adv Drug Deliv Rev. (2023) 199:114972. doi: 10.1016/j.addr.2023.114972
94. Jeong M, Lee Y, Park J, Jung H, Lee H. Lipid nanoparticles (LNPs) for in vivo RNA delivery and their breakthrough technology for future applications. Adv Drug Deliv Rev. (2023) 200:114990. doi: 10.1016/j.addr.2023.114990
95. Jiang Y, Jiang B, Wang Z, Li Y, Cheung JCW, Yin B, et al. Nucleic acid armor: fortifying RNA therapeutics through delivery and targeting innovations for immunotherapy. Int J Mol Sci. (2024) 25(16):8888. doi: 10.3390/ijms25168888
96. Murphy DE, de Jong OG, Evers MJW, Nurazizah M, Schiffelers RM, Vader P. Natural or synthetic RNA delivery: a stoichiometric comparison of extracellular vesicles and synthetic nanoparticles. Nano Lett. (2021) 21(4):1888–95. doi: 10.1021/acs.nanolett.1c00094
97. Ma Y, Li S, Lin X, Chen Y. Bioinspired spatiotemporal management toward RNA therapies. ACS Nano. (2023) 17(24):24539–63. doi: 10.1021/acsnano.3c08219
98. Jung HN, Lee SY, Lee S, Youn H, Im HJ. Lipid nanoparticles for delivery of RNA therapeutics: current status and the role of in vivo imaging. Theranostics. (2022) 12(17):7509–31. doi: 10.7150/thno.77259
99. Chen X, Zhang X, Gross S, Houser SR, Soboloff J. Acetylation of SERCA2a, another target for heart failure treatment? Circ Res. (2019) 124(9):1285–7. doi: 10.1161/circresaha.119.315017
100. Lai P, Hille SS, Subramanian H, Wiegmann R, Roser P, Müller OJ, et al. Remodelling of cAMP dynamics within the SERCA2a microdomain in heart failure with preserved ejection fraction caused by obesity and type 2 diabetes. Cardiovasc Res. (2024) 120(3):273–85. doi: 10.1093/cvr/cvad178
101. Parot J, Mehn D, Jankevics H, Markova N, Carboni M, Olaisen C, et al. Quality assessment of LNP-RNA therapeutics with orthogonal analytical techniques. J Controlled Release. (2024) 367:385–401. doi: 10.1016/j.jconrel.2024.01.037
102. Hosen MR, Goody PR, Zietzer A, Nickenig G, Jansen F. MicroRNAs as master regulators of atherosclerosis: from pathogenesis to novel therapeutic options. Antioxid Redox Signal. (2020) 33(9):621–44. doi: 10.1089/ars.2020.8107
103. Han C, Yang J, Sun J, Qin G. Extracellular vesicles in cardiovascular disease: biological functions and therapeutic implications. Pharmacol Ther. (2022) 233:108025. doi: 10.1016/j.pharmthera.2021.108025
104. Choudry MW, Riaz R, Raza MH, Nawaz P, Ahmad B, Jahan N, et al. Development of non-viral targeted RNA delivery vehicles—a key factor in success of therapeutic RNA. J Drug Target. (2024) 33(2):171–84. doi: 10.1080/1061186x.2024.2416241
105. Chia SPS, Pang JKS, Soh BS. Current RNA strategies in treating cardiovascular diseases. Mol Ther. (2024) 32(3):580–608. doi: 10.1016/j.ymthe.2024.01.028
106. Friesen JJ, Blakney AK. Trends in the synthetic polymer delivery of RNA. J Gene Med. (2024) 26(2):e3672. doi: 10.1002/jgm.3672
107. Subramanian G, Kalidasan K, Quah S, Han QCG, Chan J, Wacker MG, et al. Breaking barriers: innovative approaches for skin delivery of RNA therapeutics. Int J Pharm. (2024) 661:124435. doi: 10.1016/j.ijpharm.2024.124435
108. Huang CK, Kafert-Kasting S, Thum T. Preclinical and clinical development of noncoding RNA therapeutics for cardiovascular disease. Circ Res. (2020) 126(5):663–78. doi: 10.1161/circresaha.119.315856
109. Paterek A, Załęska-Kocięcka M, Surzykiewicz M, Wojdyńska Z, Leszek P, Mączewski M. Non-coding RNA therapeutics in the treatment of heart failure. Eur Heart J Cardiovasc Pharmacother. (2024) 10(4):353–60. doi: 10.1093/ehjcvp/pvae027
110. Palomaki GE, Melillo S, Neveux L, Douglas MP, Dotson WD, Janssens AC, et al. Use of genomic profiling to assess risk for cardiovascular disease and identify individualized prevention strategies–a targeted evidence-based review. Genet Med. (2010) 12(12):772–84. doi: 10.1097/GIM.0b013e3181f8728d
111. Wu J, Song J, Wang C, Niu D, Li H, Liu Y, et al. Identification of serum microRNAs for cardiovascular risk stratification in dyslipidemia subjects. Int J Cardiol. (2014) 172(1):232–4. doi: 10.1016/j.ijcard.2013.12.214
112. Dagher OK, Schwab RD, Brookens SK, Posey AD Jr. Advances in cancer immunotherapies. Cell. (2023) 186(8):1814–1814.e1. doi: 10.1016/j.cell.2023.02.039
113. Wu T, Liu C, Zou S, Lyu R, Yang B, Yan H, et al. An engineered hypercompact CRISPR-Cas12f system with boosted gene-editing activity. Nat Chem Biol. (2023) 19(11):1384–93. doi: 10.1038/s41589-023-01380-9
114. Lokras AG, Bobak TR, Baghel SS, Sebastiani F, Foged C. Advances in the design and delivery of RNA vaccines for infectious diseases. Adv Drug Deliv Rev. (2024) 213:115419. doi: 10.1016/j.addr.2024.115419
115. Luo Y, Liu L, He Z, Zhang S, Huo P, Wang Z, et al. TREAT: therapeutic RNAs exploration inspired by artificial intelligence technology. Comput Struct Biotechnol J. (2022) 20:5680–9. doi: 10.1016/j.csbj.2022.10.011
116. Wei J, Lotfy P, Faizi K, Baungaard S, Gibson E, Wang E, et al. Deep learning and CRISPR-Cas13d ortholog discovery for optimized RNA targeting. Cell Syst. (2023) 14(12):1087–1102.e13. doi: 10.1016/j.cels.2023.11.006
117. Thi HV, Hoang TN, Le NQ, Chu DT. Application of data science and bioinformatics in RNA therapeutics. Prog Mol Biol Transl Sci. (2024) 203:83–97. doi: 10.1016/bs.pmbts.2023.12.019
118. Niazi SK. RNA therapeutics: a healthcare paradigm shift. Biomedicines. (2023) 11(5):1275. doi: 10.3390/biomedicines11051275
119. Zhang J, Lang M, Zhou Y, Zhang Y. Predicting RNA structures and functions by artificial intelligence. Trends Genet. (2023) 40(1):94–107. doi: 10.1016/j.tig.2023.10.001
120. Zeng Y, Du WW, Wu Y, Yang Z, Awan FM, Li X, et al. A circular RNA binds to and activates AKT phosphorylation and nuclear localization reducing apoptosis and enhancing cardiac repair. Theranostics. (2017) 7(16):3842–55. doi: 10.7150/thno.19764
Keywords: cardiovascular diseases, RNA therapeutics, mRNA, siRNA, miRNA, RNA aptamers, RNA delivery systems
Citation: Dui W, Xiaobin Z, Haifeng Z, Lijuan D, Wenhui H, Zhengfeng Z and Jinling S (2025) Harnessing RNA therapeutics: novel approaches and emerging strategies for cardiovascular disease management. Front. Cardiovasc. Med. 12:1546515. doi: 10.3389/fcvm.2025.1546515
Received: 18 December 2024; Accepted: 3 March 2025;
Published: 20 March 2025.
Edited by:
DeLisa Fairweather, Mayo Clinic Florida, United StatesReviewed by:
Zhengwu Sun, Dalian Municipal Central Hospital, ChinaMichał Mączewski, Medical Centre for Postgraduate Education, Poland
Simona Brillante, Telethon Institute of Genetics and Medicine (TIGEM), Italy
Victor Hugo Joaquim, University of São Paulo, Brazil
Copyright: © 2025 Dui, Xiaobin, Haifeng, Lijuan, Wenhui, Zhengfeng and Jinling. This is an open-access article distributed under the terms of the Creative Commons Attribution License (CC BY). The use, distribution or reproduction in other forums is permitted, provided the original author(s) and the copyright owner(s) are credited and that the original publication in this journal is cited, in accordance with accepted academic practice. No use, distribution or reproduction is permitted which does not comply with these terms.
*Correspondence: Song Jinling, OTUzNDczMDcwQHFxLmNvbQ==