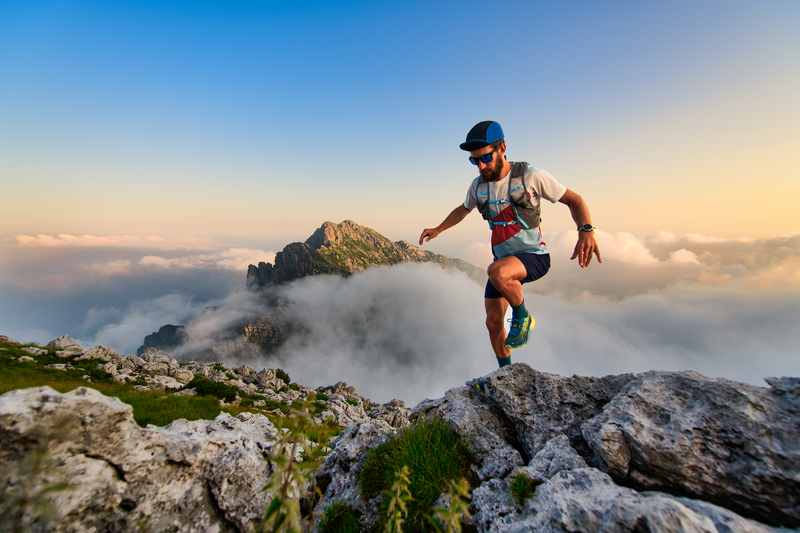
95% of researchers rate our articles as excellent or good
Learn more about the work of our research integrity team to safeguard the quality of each article we publish.
Find out more
REVIEW article
Front. Cardiovasc. Med. , 04 February 2025
Sec. Clinical and Translational Cardiovascular Medicine
Volume 12 - 2025 | https://doi.org/10.3389/fcvm.2025.1541278
Atrial cardiomyopathy is a multifaceted heart disease characterized by structural and functional abnormalities of the atria and is closely associated with atrial fibrillation and its complications. Its etiology involves a number of factors, including genetic, infectious, immunologic, and metabolic factors. Recent research has highlighted the critical role of the gut microbiota in the pathogenesis of atrial cardiomyopathy, and this is consistent with the gut–heart axis having major implications for cardiac health. The aim of this work is to bridge the knowledge gap regarding the interactions between the gut microbiota and atrial cardiomyopathy, with a particular focus on elucidating the mechanisms by which gut dysbiosis may induce atrial remodeling and dysfunction. This article provides an overview of the role of the gut microbiota in the pathogenesis of atrial cardiomyopathy, including changes in the composition of the gut microbiota and the effects of its metabolites. We also discuss how diet and exercise affect atrial cardiomyopathy by influencing the gut microbiota, as well as possible future therapeutic approaches targeting the gut–heart axis. A healthy gut microbiota can prevent disease, but ecological dysbiosis can lead to a variety of symptoms, including the induction of heart disease. We focus on the pathophysiological aspects of atrial cardiomyopathy, the impact of gut microbiota dysbiosis on atrial structure and function, and therapeutic strategies exploring modulation of the microbiota for the treatment of atrial cardiomyopathy. Finally, we discuss the role of gut microbiota in the treatment of atrial cardiomyopathy, including fecal microbiota transplantation and oral probiotics or prebiotics. Our study highlights the importance of gut microbiota homeostasis for cardiovascular health and suggests that targeted interventions on the gut microbiota may pave the way for innovative preventive and therapeutic strategies targeting atrial cardiomyopathy.
Atrial cardiomyopathy (ACM) is a myocardial disease that primarily affects the atria. It typically presents as structural changes, dysfunction, and abnormal electrophysiological features of the atria (1–3). The etiology of ACM is complex and includes genetic factors, infection, immune dysregulation, and metabolic abnormalities. Regardless of the etiology, atrial systolic dysfunction results in atrial enlargement and fibrosis, and the clinical features of ACM are usually closely associated with atrial fibrillation and its complications, which are characterized by an increased risk of arrhythmias and stroke.
The gut microbiota (GM) is a complex ecosystem containing trillions of bacteria, viruses, fungi, and other microorganisms (4–6). These organisms play important roles in digestion, immunity, and metabolism. Metabolites from the GM can exert effects independently or through pathways involving host metabolism, thereby contributing to either the maintenance of health or the progression of disease (7), such as atherosclerotic cardiovascular disease (CVD) (8). Indeed, improving human health by modulating the biosynthesis of microbial metabolites is an emerging frontier in pharmaceutical research (9).
Gut dysbiosis is a disorder of the intestinal microbiota characterized by a decrease in the number and diversity of beneficial bacteria and an increase in the number of harmful bacteria (10). This microbial imbalance has been linked to the etiology of a range of health disorders, including gastrointestinal disorders, metabolic syndrome, and cardiovascular disease (11–15). Dysbiosis can be caused by a variety of pathogenic factors, such as inadequate dietary intake, use of antibiotics, psychological stress, and chronic diseases (16). Many metabolites, such as short-chain fatty acids (SCFAs) (17, 18), bile acids (19, 20), and trimethylamine N-oxides (TMAO) (21–23), are affected by gut microbial–host interactions, which in turn affect intestinal health and function, as well as various metabolic pathways in the host (24–26) (Figure 1).
Figure 1. Associations between the gut microbiota, atrial cardiomyopathy, and cardiovascular diseases along potential interventions. Microbiome dysbiosis may lead to an increase in opportunistic pathogens or a decrease in SCFA-producing bacteria, which may lead to TMAO accumulation as well as a decrease in SCFA, all of which may contribute to the development of atrial cardiomyopathy and exacerbate the progression of CVDs. Conversely, interventions such as healthy diet, moderate exercise, prebiotic/probiotic use, and fecal microbiota transplantation offer opportunities to indirectly influence CVD progression. SCFA, short chain fatty acid; TMAO, trimethylamine-N-oxide; LPS, lipopolysaccharides; CVDs, cardiovascular diseases (By Figdraw).
ACM is a disease that affects the structure and function of the heart. In recent years, research has focused on the relationship between gut health and heart disease. Dysbiosis of the gut microbiota may influence the development of ACM through several mechanisms: inflammatory responses, changes in metabolites, and effects of the immune system. Certain circulating metabolites may increase the risk of cardiovascular events and subsequent pathological responses due to changes in the microbiota (27, 28). Several studies have confirmed the existence of a gut–heart axis (29, 30). Thus, the relationship between the GM and underlying cardiac disease has been well studied. However, the relationship between the GM and the cardiac pathological condition ACM has not been characterized to date. To address this issue, we review the existing literature and discuss the role of the GM in the occurrence and development of ACM. Based on our findings, modification of the microbiota appears to be a potential therapeutic approach to prevent the progression of ACM.
Atrial hypertrophy, fibrosis, and remodeling are the major morphologic changes observed in the atrial structure (31–33). Atrial fibrosis is invariably associated with atrial hypertrophy, which is induced by prolonged pressure or volume overload and ultimately leads to proliferation and hypertrophic dilatation of atrial myocytes (34, 35). Atrial fibrosis is a major feature of ACM and manifests as an abnormal accumulation of collagen within the atrial myocardium (16, 33). This pathological deposition leads to changes in the electrophysiological properties of the atria and increases susceptibility to arrhythmias (36). In addition, as the disease progresses, the atria undergo significant geometric and structural changes, including atrial wall thickening and ventricular dilatation, which may affect overall cardiac function (37).
In addition to atrial enlargement, atrial wall fibrosis, and the presence of atrial anatomic abnormalities (1, 2), other structural changes occur in ACM, including atrial trabecular hyperplasia, atrial microarteriovenous atheromatosis, and intra-atrial thrombosis (38–40). Together, these changes contribute to the complex pathophysiology of ACM, highlighting the need for a comprehensive understanding of the multiple effects of the disease on cardiac structure and function.
The atria play both active and passive roles in the cardiac cycle, which can be divided into three distinct phases: the atrial storage phase, the atrial conduction phase, and the atrial contraction phase. During the atrial storage phase, the atria accommodate venous return by adjusting the interval between closure and opening of the atrioventricular (AV) valve during ventricular systole. The ability of the atria to store blood depends on atrial compliance and diastolic function (41). Simultaneously, as intra-atrial pressure rises and the ventricles actively relax, the atria enter the atrial conduit phase with the onset of AV valve opening. During the initial phase of ventricular diastole, blood from the atria, systemic veins, and pulmonary veins passively fills the ventricles, while the atria play the role of conduit. The pressure gradient between the atria and the ventricles gradually decreases, causing ventricular filling to slow or stop in mid-diastole, a phase known as diastole. Finally, at the end of ventricular diastole, the atria actively push the remaining blood into the ventricles by contracting. In healthy individuals, the atria contribute approximately 40%, 35%, and 25% to ventricular filling during the blood storage, inflow, and contraction phases, respectively (42). In atrial fibrillation (AF), ventricular filling is more dependent on the conduit phase because of the loss of systolic function and the significant reduction in blood storage due to atrial stiffness (43).
The main functional changes in ACM are manifested by altered electrophysiological properties and impairment of atrial systolic and diastolic function. ACM induces electrophysiological abnormalities, such as decreased electrical conduction velocity and increased autoregulation, which can lead to electrical instability of the atria and an increased tendency to develop atrial fibrillation and other arrhythmogenic events (1, 32, 41, 44).
In addition, atrial contractility is often impaired in ACM due to atrial myocyte dysfunction and dynamic changes in intra-atrial pressure. This dysfunction reduces the ability of the atria to push blood during ventricular contraction. At the same time, diastolic atrial function is also impaired, as evidenced by the inability of the diastolic atria to adequately fill with blood (1, 40, 43). This deficiency leads to an increase in intra-atrial pressure, which exacerbates structural remodeling of the atria.
These functional abnormalities highlight the intricate interplay between electrophysiological and mechanistic alterations in atrial electrophysiology and mechanics in the pathogenesis of ACM, and a nuanced approach is required to understand and manage this complex heart disease.
The electrophysiologic characteristics of ACM are closely related to a range of cellular and molecular mechanisms. These mechanisms include the electrophysiological properties of atrial myocytes, intercellular electrical coupling, and the process of atrial remodeling. Atrial myocytes in patients with ACM can exhibit different electrophysiological characteristics (1, 38, 45), such as changes in action potential duration and alterations in ion channel function. These changes may affect atrial excitability and conduction, thereby inducing arrhythmias. The presence of atrial fibrosis in ACM leads to reduced intercellular electrical coupling. Reduced coupling leads to heterogeneous propagation of electrical signals within the atria, which in turn leads to instability of electrical activity within the atria and an increased risk of reentrant cardioversion excitation (35, 38, 44–46). ACM is closely associated with atrial remodeling (47), which is a multifaceted process involving structural changes, apoptosis, and the initiation of fibrosis. During the remodeling process, the electrophysiological characteristics of atrial myocytes undergo progressive changes, ultimately leading to deterioration of atrial electrophysiological function and subsequent arrhythmias (1, 38, 40, 44, 46).
In summary, the electrophysiological features of ACM are central to a complete understanding of the disease. These features include a shortened effective atrial refractory period, delayed atrial conduction, and increased reentrant excitation, all of which are closely associated with alterations in atrial structure and function (39). By elucidating these electrophysiological features and their underlying cellular and molecular mechanisms, clinicians can more accurately assess and reduce the risk of arrhythmias in patients with ACM.
The development of ACM is closely related to the electrophysiological activity of the atria, particularly the atrial remodeling process. The atria play an important role in cardiac function, including the regulation of left ventricular filling pressures and cardiovascular function. Structural and functional changes in the atria affect these physiological processes and contribute to the development of ACM (Figure 2).
Figure 2. Metabolites derived from the gut microbiota are closely linked to mechanisms that promote atrial remodeling. Alterations in the composition of the gut microbiota can initiate changes in metabolic pathways, which may lead to the translocation of bacteria, their fragments, and products into the circulation. This process can intensify the pro-inflammatory environment and induce metabolic dysregulation, creating a conducive setting for the development of cardiovascular disease. Specifically, LPS activates the NLRP3 inflammasome, resulting in the production of IL-1β and IL-18, which contribute to atrial fibrosis. Furthermore, LPS may enhance oxidative stress by activating the NF-κB pathway or inhibiting the expression of Nrf2. A reduction in the production of SCFAs may lead to an overproduction of TNF-α and IL-6, inflammatory factors that activate the Wnt pathway and induce pathological changes in atrial cardiomyocytes. Concurrently, the decrease in SCFAs may also inhibit Nrf2 production, further exacerbating oxidative stress and promoting atrial remodeling. Collectively, these metabolites derived from gut microbiota have a significant impact on atrial electrophysiology and structural remodeling. LPS, lipopolysaccharide; SCFAs, short-chain fatty acids; IL-1β, interleukin-1 beta; IL-6, interleukin-6; IL-18, interleukin-18; TNF-α, tumor necrosis factor-alpha (By Figdraw).
The Wnt pathway plays an important role in ACM. Studies have shown that activation of the Wnt pathway is associated with the development of ACM (48, 49). This pathway influences the structural remodeling and electrophysiological properties of the atria by regulating cell proliferation and differentiation (50, 51). The GM influences the host's physiological state through its metabolic products, particularly SCFAs (52–54). These SCFAs modulate immune responses and inflammation levels, thereby indirectly affecting the Wnt signaling pathway (55, 56). Research indicates that certain SCFAs may promote cardiac cell proliferation and repair by regulating the Wnt signaling pathway (57–59). Dysbiosis of the GM is often associated with chronic low-grade inflammation, which can activate the Wnt signaling pathway, leading to pathological changes in atrial cardiomyocytes (60). Specifically, inflammatory cytokines such as tumor necrosis factor-alpha and interleukin-6 (IL-6) can enhance the transmission of Wnt signals through various pathways, thereby promoting the progression of ACM (61).
The NLRP3 inflammasome is a critical component of the immune response, capable of detecting intracellular pathogens and damage signals (62, 63). Dysbiosis of the GM leads to elevated levels of lipopolysaccharides (LPS), which activate the NLRP3 inflammasome via Toll-like receptors (64, 65). The activation of the NLRP3 inflammasome results in the release of pro-inflammatory cytokines, such as interleukin-1 beta (IL-1β) and interleukin-18 (IL-18), which contribute to atrial remodeling and electrophysiological abnormalities (66–68). Furthermore, the release of these cytokines promotes atrial fibrosis, thereby increasing the risk of AF (69, 70). Similarly, an imbalance in the GM can impair cardiac function and elevate the risk of cardiovascular events (71, 72).
ACM is a pathological condition characterized by alterations in atrial structure and function, frequently associated with AF and heart failure (HF) (73, 74). Nrf2 is a pivotal transcription factor that regulates antioxidant responses and maintains cellular homeostasis. In the context of cardiovascular health, Nrf2 plays a critical role by mitigating oxidative stress and inflammatory responses, thereby protecting cardiac function (75–77). Oxidative stress has been shown to significantly contribute to the development of atrial cardiomyopathy (78–80). As oxidative stress levels increase, calcium homeostasis in cardiac cells is disrupted, leading to changes in the electrophysiological properties of the atrium and the initiation of arrhythmias (81–83). Dysbiosis of GM may promote the progression of ACM through several pathways: 1. Dysbiosis can lead to increased intestinal permeability, allowing endotoxins to enter the circulation and activate a systemic inflammatory response. This inflammatory state may further exacerbate oxidative stress by activating NF-κB and other pathways while down-regulating Nrf2 (84–86). 2. Metabolites such as SCFAs, produced by gut microbes, have protective effects on heart health. However, dysbiosis leads to a reduction in these beneficial metabolites, impairing Nrf2 activation and promoting the development of atrial cardiomyopathy (87, 88). 3. Autonomic nervous system regulation: The GM and its metabolites may influence the cardiac autonomic nervous system through direct or indirect mechanisms, subsequently affecting the electrophysiological characteristics of the heart. This may occur by modulating Nrf2 expression and activity (86, 89, 90).
Several key molecules play important roles in the cellular and molecular mechanisms of ACM that affect atrial structure and function. These molecules include cyclooxygenase-2 (COX-2), endothelin-1 (ET-1), and other factors (91) that play key roles in cardiac remodeling, atrial dysfunction, vasoconstriction, and inflammatory responses.
COX-2 is a key enzyme in prostaglandin biosynthesis and is involved in a variety of physiological and pathological processes. Its functions are manifold and include the following three factors. (1) The inflammatory response: COX-2 is upregulated in response to inflammation and its expression level is significantly increased in patients with ACM. It mediates the local inflammatory response through the synthesis of prostaglandins, which affect atrial function and morphology (92, 93). (2) Cardiac remodeling: COX-2 expression is increased in conditions such as myocardial infarction and HF, leading to increased cardiac remodeling and functional decline. Pharmacological inhibition of COX-2 has been shown to improve cardiac function, making it a promising target for therapeutic intervention (94, 95). (3) Apoptosis: COX-2 activity is associated with cardiomyocyte apoptosis, which in turn promotes atrial fibrosis and dysfunction.
In summary, COX-2 has a major impact on the pathogenesis of myocardial ischemia through its involvement in inflammation, cardiac remodeling, and apoptosis, highlighting its potential as a therapeutic target to mitigate disease progression (96).
ET-1 is a potent vasoconstrictor with important effects on the heart and blood vessels. Its major functions are as follows. (1) Vasoconstriction: By binding to endothelin receptors, ET-1 induces vascular smooth muscle contraction and elevated blood pressure (97–99), which plays an important role in the pathophysiology of ACM. (2) Cardiac remodeling: ET-1 promotes cardiomyocyte proliferation and fibrosis, which leads to structural changes in the atria, triggering atrial dysfunction and AF (100). (3) Promotion of the inflammatory response: ET-1 is also involved in regulation of the inflammatory response and can stimulate the release of cytokines that exacerbate atrial damage and fibrosis (101).
In addition to COX-2 and ET-1, a number of other important molecules are involved in ACM as follows. (1) Neurotrophic factors, such as brain-derived neurotrophic factor, which play an important role in adaptive remodeling of the heart and affect cardiomyocyte survival and function (102, 103). (2) Cytokines, such as tumor necrosis factor-alpha (TNFα) and IL-6, which can contribute to inflammation and apoptosis in ACM, further exacerbating cardiac dysfunction (104). (3) Atrial natriuretic peptide, a hormone secreted by the atria with diuretic and antihypertensive effects that reduces atrial workload and improves cardiac function (105–107).
Sequencing technology has advanced dramatically over the past decade, allowing researchers around the world to assess how genetic modifications affect human health. Humans develop symbiotic relationships with microorganisms from an early age (108). Factors such as environment (109, 110), proximity to other humans and animals (111), diet (112, 113), genetics (114–116), and temporal changes (117–119) influence the microbial communities in our skin, mouth, and gut (120, 121). In terms of its impact, the GM has been likened to a previously unknown organ; it is extensively metabolized and carries 150 times more genes than the human genome, providing the host with a range of metabolic capabilities that would otherwise be unavailable (122). Unlike the human genome, the GM is relatively plastic. It can be rapidly altered by factors such as diet, drugs, probiotics, and metabolites produced by microbes. Therefore, intentional modification of the GM may have health consequences. Transgenesis is increasingly recognized as an important target for drugs, and certain microbes have been shown to inactivate or activate certain exogenous substances, thereby altering the effects of various therapeutic drugs (123). We are just beginning to understand the systemic effects of whole transgenes on the entire metabolite pool.
Studies have shown that a healthy GM correlates with high microbial diversity and abundance (124–128), which is largely influenced by the host's diet, lifestyle, and genetic predisposition. GM diversity is typically assessed using two parameters, namely species richness and species evenness. A robust gut may contain approximately 1,000 different bacterial species (129, 130), with inter-individual variation attributable to dietary, environmental, and genetic factors. Under healthy conditions, GMs are characterized by a relatively even distribution of bacterial species; however, under pathological conditions such as inflammatory bowel disease or ACM, certain species may overgrow, leading to dysbiosis (131). Transgenic diversity affects not only digestive and metabolic processes, but also the immune system, neuroendocrine regulation, and the cardiovascular health of the host (132).
The composition and function of the GM is critical to the maintenance of overall health. It is closely linked to nutrient metabolism, immunomodulation, and pharmacokinetics, and is essential for maintaining the integrity of the GM. Dysregulation of the GM can lead to a number of health problems, particularly affecting cardiovascular health (131).
It is hypothesized that gut dysbiosis has multiple effects on the etiology and progression of ACM through the following mechanisms. (1) The inflammatory response: Gut dysbiosis is often associated with increased systemic inflammation. Bacterial metabolites (e.g., lipopolysaccharides) can cross the intestinal barrier as a result of increased permeability, triggering a systemic inflammatory cascade that leads to cardiac tissue damage and the progression of ACM (133, 134). (2) Effects of metabolites: Gut microbial metabolites such as SCFAs have anti-inflammatory and cardioprotective properties. Interfering with genetic modifications may reduce SCFA production, which may decrease cardioprotective effects and increase susceptibility to acute myocardial infarction (135, 136). (3) Dysfunction of the intestinal barrier: Dysbiosis of the transgenic microbiota induces intestinal epithelial dysfunction, which increases intestinal permeability and facilitates the translocation of endogenous toxins and bacteria into the systemic circulation. This translocation can trigger an inflammatory response that may lead to the development of ACM (133, 137, 138). (4) Microbiota diversity: Empirical evidence suggests that patients with HF have reduced microbiota diversity, which may be associated with the development of ACM. Genomes with reduced diversity may be less effective in maintaining gut and immune health, thereby increasing the risk of cardiovascular disease (139, 140).
The interactions between gut dysbiosis and ACM are complex and involve inflammatory responses, metabolite synthesis, gut barrier integrity, and microbial diversity. Enhancing microbial diversity and metabolic function by modulating the gut microbiota may be a strategy to reduce the risk of ACM.
In recent years, an increasing number of studies have demonstrated a significant correlation between the composition of the GM and its metabolites and the incidence of a variety of cardiovascular diseases, with a particular focus on ACM (8, 9, 16, 141), a cardiac disorder characterized by structural and functional changes in the atria that can lead to severe arrhythmias, including AF. The relationship between the GM and AF remains to be elucidated (142, 143). The hypothesis is that GM or its metabolic by-products have effects on distal cellular targets, as indicated by the presence of key metabolites. These include SCFAs (the major end products of microbial fermentation of dietary fiber), TMAO, and lipopolysaccharides (26, 144, 145).
The left atrium (LA) serves three primary functions: fluid storage, conduit, and contraction. The interplay among these functions is essential for optimal ventricular filling and cardiac output, with the LA often being the first to respond to left ventricular (LV) diastolic dysfunction. Over time, however, the LA loses its contractile function, leading to mechanical failure and structural changes (146). Patients with LV diastolic dysfunction in the preclinical phase of HF have an abnormal LA strain and left atrial volume index, although the prevalence of abnormal strain is generally high (147). The dependence on the contribution of the LA to LV filling increases as LV diastolic dysfunction progresses (148). Some patients eventually progress to the clinical stage of HF. The presence of abnormal left strain during the reservoir phase is independently associated with incident HF despite a normal left atrial volume index (149). The risk of HF events associated with LA structural and functional abnormalities is not related to the left ventricular ejection fraction or natriuretic peptide levels (150). Alterations in atrial mechanics, particularly the LA, play an important role in various aspects of HF with a preserved ejection fraction. Emerging evidence suggests that gut ecological dysregulation has an impact on clinical HF and its subtypes (e.g., HF with a preserved ejection fraction) (151). Although the underlying mechanisms of the gut–heart axis during HF remain largely unknown, increased filling pressures and impaired diastolic blood pressure, which may lead to a progressive decrease in cardiac output, have been proposed as main drivers of gut ecological dysregulation (152). These changes in gut composition are characterized by a decrease in microbial α-diversity and a decrease in the number of beneficial bacteria, such as those with the potential to produce SCFAs (151). At the same time, the number of pathogenic bacteria in the gut ecosystem increases. In addition, gut dysbiosis has been shown to affect human health by modulating the host's circulating metabolite profile. This has been attributed to the ability of the GM to produce a wide range of functional metabolites that can enter the circulation alone or in concert with host metabolic processes (153).
Gut dysbiosis leads to reduced SCFA production, which triggers metabolic dysregulation and systemic inflammation, both of which are potential risk factors for the development of ACM.
With the development of gut dysbiosis, intestinal barrier function is compromised, allowing endogenous bacterial components (e.g., lipopolysaccharides) to enter the circulation and trigger a systemic inflammatory response (15). Studies have shown that chronic inflammation is closely associated with the development of atrial cardiomyopathy. Elevated levels of inflammatory factors such as TNF-α and IL-6 may damage atrial myocytes and remodel the atrial structure, leading to the development of ACM (104).
In addition to affecting the host immune response, GM also affects cardiac function through the production of various metabolites. For example, some metabolites produced by the GM, such as TMAO (20–22), have been shown to be associated with an increased risk of cardiovascular disease, and accumulation of TMAO may indirectly affect the electrophysiological properties of the atria by promoting atherosclerosis and myocardial damage, leading to ACM (15).
Changes in metabolites due to gut dysbiosis affect the electrophysiological properties of the heart (154). Studies have shown that metabolites produced by the GM affect the electrical activity of the heart, leading to changes in cardiac autoregulation and excitability. These changes can lead to atrial myocardial remodeling and electrical conduction abnormalities, increasing the risk of AF and other arrhythmias (8, 15).
To prevent ACM, it is important to maintain a good glycemic balance. This can be achieved through a healthy diet (high in fiber, low in sugar, high in prebiotics and probiotics) (126, 127, 151), moderate exercise, and good lifestyle habits. By promoting the growth of beneficial microorganisms, intestinal barrier function can be improved and systemic inflammatory responses can be reduced, which in turn promotes cardiovascular health.
The gut–heart axis is a biological mechanism that describes how the GM affects heart health through multiple pathways (155). Studies have shown that the GM may interact with the heart in the following ways. (1) The inflammatory response: Gut dysbiosis leads to increased intestinal permeability, which allows endogenous toxins and bacterial metabolites to enter the bloodstream and trigger systemic inflammation, which is thought to be an important mechanism in cardiovascular disease (133). (2) Metabolites: The GM produces SCFAs and other metabolites that may affect cardiac metabolism and function (156, 157). (3) Neurological signaling: The GM interacts with the central nervous system via the vagus nerve and affects autonomic regulation of the heart (158).
Gut dysbiosis is strongly associated with the development of ACM (16). Reduced GM diversity can lead to inflammation and fibrosis of cardiac tissue, which in turn can lead to atrial dysfunction (159, 160). Gut dysbiosis is usually associated with chronic low-grade inflammation, and this inflammatory state may promote electrophysiological remodeling of the atria, increasing the risk of AF. Changes in GM composition can strongly influence the synthesis of metabolites, and TMAO is one such metabolite that has been extensively studied for its role in increasing the risk of cardiovascular disease. The mechanisms of action of TMAO are diverse and include modulation of tissue sterol metabolism (26, 161, 162), which may alter cholesterol distribution and metabolism; enhancement of endothelial cell activation, which promotes vascular inflammation (26, 163–165); and stimulation of the pro-fibrotic pathway (166), which may contribute to pathological remodeling of cardiovascular tissue. These metabolites may affect the heart through a variety of mechanisms, including improvement of endothelial function and suppression of inflammatory responses. The gut–heart axis also includes transgenes that may affect heart health through neural mechanisms. Studies have shown that the GM can affect autonomic homeostasis in the heart by modulating vagal activity, which may play a role in ACM (167).
A growing number of studies have examined the association between the GM and metabolic and CVD, including coronary heart disease and HF (168, 169). Macrogenomic analyses of various CVD patient populations have demonstrated significant differences in GM composition in the presence or absence of CVD and HF (170, 171). In addition, metabolomics-based clinical studies (172, 173) and mechanistic studies in animal models have further confirmed the potential causal role of the genome in the development of CVD and hyperlipidemia (174–176). GM produces a diverse array of metabolites, including SCFAs, amines, and phenolic compounds. These metabolites significantly contribute to the pathophysiology of ACM through multiple pathways: they modulate immune responses, alter cardiac metabolism, and interact with receptors in cardiac tissue (177).
In clinical observations, many studies have found significant differences in the GM composition of AF patients compared with healthy individuals. It has been established that the metabolic interactions between the gut and the host play a pivotal role in the development of AF (178). For example, it has been shown that certain GM compositions are significantly decreased in AF patients, while others are increased, which may be related to the pathogenesis of AF (179, 180). Dysregulation of the GM has been demonstrated to result in alterations to cardiac structure and function, thereby elevating the risk of AF (181). In addition, changes in GM are strongly correlated with a patient's cardiac functional status and metabolic profile, suggesting that gut health may be potentially valuable in the prevention and treatment of ACM. Research indicates that GM and its metabolites may significantly influence the development of HF. Patients diagnosed with HF often exhibit a pronounced imbalance in their GM, which may be closely associated with the pathophysiological mechanisms underlying ACM (182). Changes in GM not only affect the direct symptoms of ACM but may also indirectly contribute to the onset of heart failure by modulating cardiovascular risk factors, including metabolic syndrome, obesity, and diabetes (177, 183, 184).
Laboratory studies also support a link between the GM and ACM. For example, a study in an animal model found that modulating genetic changes improved ventricular function and reduced the risk of developing atrial myopathy. Specifically, antibiotic intervention significantly improved atrial structure and electrophysiological properties in an animal model, suggesting that changes in the GM may directly affect cardiac physiology (185).
Currently, numerous clinical studies and fundamental experiments are in progress to elucidate the precise mechanisms by which GM and its metabolites contribute to ACM (21). Researchers have employed a variety of advanced technologies, including high-throughput sequencing and metabolomics analysis, to gain a comprehensive understanding of the interactions between GM and cardiovascular health.
Future research should focus on enhancing outcomes for patients with ACM by modulating GM through interventions such as probiotics or dietary modifications. This strategy represents a novel therapeutic approach with significant potential for clinical applications (186, 187).
Fiber is an important source of energy for the body. Increased intake of whole grains, legumes, fruits, and vegetables may improve heart health by promoting the growth of beneficial bacteria and increasing the production of SCFAs. Epidemiologic studies have shown that adequate fiber intake helps prevent dyslipidemia and atherosclerotic vascular disease (188). Omega-3 fatty acids have anti-inflammatory properties and can be obtained from foods such as fish, nuts, and seeds (189). These fatty acids are not only good for the heart, but also help improve the composition of the blood. Adequate hydration helps maintain a healthy digestive system and promotes gut microbial balance (190).
Lifestyle modifications can significantly slow the progression of ACM by influencing the GM and its metabolites (191–194). Engaging in moderate exercise and ensuring adequate sleep can promote the growth of beneficial microbes, thereby enhancing heart health. Regular physical activity has been shown to improve GM diversity and foster the proliferation of advantageous bacteria. Research indicates that exercise enhances gut barrier function and reduces systemic inflammation, both of which contribute to slowing the progression of ACM (195–198). Additionally, exercise increases the heart's pumping capacity and electrophysiological stability, thereby lowering the risk of arrhythmias associated with ACM (199–202). Conversely, sleep deprivation is linked to an elevated risk of various cardiovascular diseases, while quality sleep facilitates physical repair and supports immune function (203, 204). Studies have demonstrated that improved sleep quality can help restore GM balance and mitigate inflammatory responses, which is beneficial for managing ACM (205, 206).
Probiotics are live microorganisms that are beneficial to the health of the host (207). Common probiotics include Lactobacillus and Bifidobacterium, and supplementation with these probiotics may help to restore the GM balance. Prebiotics are food ingredients that promote the growth of beneficial microorganisms, such as inulin and oligofructose. Supplementation with prebiotics may increase the number and activity of beneficial bacteria in the gut (208). Probiotics are typically administered through oral supplements or fermented foods, both of which are user-friendly and well-accepted by patients (182, 209, 210). They play a crucial role in regulating intestinal microbiota, enhancing intestinal barrier function, and reducing intestinal inflammation, thereby contributing to overall health improvement (211, 212). Research has indicated that specific probiotics may positively influence cardiovascular health by lowering blood pressure and improving lipid profiles, which could indirectly benefit patients with ACM (213). In comparison to pharmacological treatments, probiotics generally exhibit fewer side effects, which are often benign and self-limiting (214). However, the health effects of various probiotic strains can differ significantly, and some may have limited effectiveness in ameliorating ACM (215, 216). Consequently, selecting appropriate probiotics is essential. The market offers a wide range of probiotic products; however, the absence of uniform quality control and standardization can result in variable efficacy (217). Furthermore, the benefits of probiotics often necessitate long-term usage to sustain their effects, which may pose convenience challenges for patients requiring consistent dietary supplementation (218). Although preliminary studies suggest potential benefits of probiotics on cardiovascular health, there remains a lack of robust clinical evidence supporting their application in ACM, necessitating further research to confirm their efficacy (219).
Fecal microbiota transplantation is an emerging therapy that restores the diversity and function of gut microbes by transplanting the GM from healthy donors into patients (220–223). FMT has been shown to effectively restore the intestinal microbiome in patients, thereby correcting gut dysbiosis (186, 224–226). This restoration may alleviate systemic inflammatory responses, potentially benefiting cardiovascular health. Several studies indicate that FMT can reduce myocardial damage and improve cardiac function by reestablishing a healthy microbiota (21, 227). This is particularly significant for patients with ACM, as enhanced atrial function could decrease the incidence of AF (178, 228). FMT may exert its effects by modulating the immune system, which plays a crucial role in cardiovascular diseases where immune-inflammatory responses are among the key pathophysiological mechanisms. The efficacy of FMT can be optimized through personalized adjustments based on an individual's unique microbiome profile, thereby enhancing therapeutic outcomes (224, 229). However, FMT involves the transplantation of fecal matter from healthy donors to patients, which poses risks of infectious disease transmission (186). Although stringent screening protocols are implemented, caution remains essential. The effectiveness of FMT varies among individuals, and some patients may not experience significant improvements. Additionally, the duration of therapeutic effects can be limited, with some patients experiencing relapses shortly after treatment (186). The acceptability of FMT may be challenged due to its association with fecal matter, both among patients and healthcare providers (186). Ethical concerns may also impede its widespread adoption and application (230). Currently, there is limited research on the long-term effects and safety of FMT in patients with atrial cardiomyopathy, underscoring the need for large-scale clinical trials to validate its sustained efficacy (231).
Gut dysbiosis, recognized as a significant correlate of several diseases, has emerged as a prominent factor in ACM. It is involved in the systemic inflammatory profile of the host and modulates the oxidative state via the gut–heart axis. The influence of the GM on systemic health through the regulation of immune responses, inflammatory mediators, metabolic pathways, and nervous system function is now widely recognized as substantial and should not be overlooked. Consequently, maintaining a diverse and balanced GM is essential for optimal health, with potential preventive and therapeutic implications for certain diseases. However, the precise mechanisms by which the GM exerts its influence on health require further elucidation, particularly in the context of probiotic interventions. Current research into the interplay between heart disease and the GM is predominantly limited to animal models, with a paucity of large-scale clinical trials and an even more limited number of positive results. Future research efforts should focus on delineating the specific mechanisms of the gut microbiota and their potential applications in disease prevention and treatment, thereby providing a sound scientific basis for improving human health.
TS: Conceptualization, Data curation, Formal Analysis, Investigation, Methodology, Project administration, Resources, Software, Visualization, Writing – original draft, Writing – review & editing. BS: Conceptualization, Data curation, Methodology, Supervision, Writing – review & editing. BL: Formal Analysis, Funding acquisition, Methodology, Resources, Supervision, Validation, Visualization, Writing – review & editing.
The author(s) declare financial support was received for the research, authorship, and/or publication of this article. This study was supported by the Natural Science Foundation of Shandong Province (grant numbers: ZR2023MH136) and Taishan Scholars Program of Shandong Province tsqn, China (NO.tsqn202306402).
Figure support was provided by Figdraw.
The authors declare that the research was conducted in the absence of any commercial or financial relationships that could be construed as a potential conflict of interest.
The author(s) declare that no Generative AI was used in the creation of this manuscript.
All claims expressed in this article are solely those of the authors and do not necessarily represent those of their affiliated organizations, or those of the publisher, the editors and the reviewers. Any product that may be evaluated in this article, or claim that may be made by its manufacturer, is not guaranteed or endorsed by the publisher.
1. Goette A, Kalman JM, Aguinaga L, Akar J, Cabrera JA, Chen SA, et al. EHRA/HRS/APHRS/SOLAECE expert consensus on atrial cardiomyopathies: definition, characterization, and clinical implication. Europace. (2016) 18:1455–90. doi: 10.1093/europace/euw161
2. Kittipibul V, Laufer-Perl M, Balakumaran K, Costanzo MR, Marwick TH, Alenezi F, et al. Atrial mechanics, atrial cardiomyopathy and impact of atrial interventions. J Card Fail. (2024) 30:1355–66. doi: 10.1016/j.cardfail.2024.06.017
3. Ninni S, Algalarrondo V, Brette F, Lemesle G, Fauconnier J. Left atrial cardiomyopathy: pathophysiological insights, assessment methods and clinical implications. Arch Cardiovasc Dis. (2024) 117:283–96. doi: 10.1016/j.acvd.2024.02.001
4. Binda C, Lopetuso LR, Rizzatti G, Gibiino G, Cennamo V, Gasbarrini A. Actinobacteria: a relevant minority for the maintenance of gut homeostasis. Dig Liver Dis. (2018) 50:421–8. doi: 10.1016/j.dld.2018.02.012
5. Benson TW, Conrad KA, Li XS, Wang Z, Helsley RN, Schugar RC, et al. Gut microbiota–derived trimethylamine N-oxide contributes to abdominal aortic aneurysm through inflammatory and apoptotic mechanisms. Circulation. (2023) 147:1079–96. doi: 10.1161/CIRCULATIONAHA.122.060573
6. Rinninella E, Raoul P, Cintoni M, Franceschi F, Miggiano GAD, Gasbarrini A, et al. What is the healthy gut Microbiota composition? A changing ecosystem across age, environment, diet, and diseases.microorganisms. (2019) 7(1):14. doi: 10.3390/microorganisms7010014
7. Donia MS, Fischbach MA. Small molecules from the human microbiota. Science. (2015) 349. doi: 10.1126/science.1254766
8. Witkowski M, Weeks TL, Hazen SL. Gut microbiota and cardiovascular disease. Circ Res. (2020) 127:553–70. doi: 10.1161/CIRCRESAHA.120.316242
9. Brown JM, Hazen SL. Targeting of microbe-derived metabolites to improve human health: the next frontier for drug discovery. J Biol Chem. (2017) 292:8560–8. doi: 10.1074/jbc.R116.765388
10. Singh P, Meenatchi R, Ahmed ZHT, Thacharodi A, Rohinth M, Kumar RRS, et al. Implications of the gut microbiome in cardiovascular diseases: association of gut microbiome with cardiovascular diseases, therapeutic interventions and multi-omics approach for precision medicine. Med Microecol. (2024) 19. doi: 10.1016/j.medmic.2023.100096
11. Cui X, Ye L, Li J, Jin L, Wang W, Li S, et al. Metagenomic and metabolomic analyses unveil dysbiosis of gut microbiota in chronic heart failure patients. Sci Rep. (2018) 8(1):635. doi: 10.1038/s41598-017-18756-2
12. Da Silva HE, Teterina A, Comelli EM, Taibi A, Arendt BM, Fischer SE, et al. Nonalcoholic fatty liver disease is associated with dysbiosis independent of body mass index and insulin resistance. Sci Rep. (2018) 8(1):1466. doi: 10.1038/s41598-018-19753-9
13. Allen RM, Zhao S, Ramirez Solano MA, Zhu W, Michell DL, Wang Y, et al. Bioinformatic analysis of endogenous and exogenous small RNAs on lipoproteins. J Extracell Vesicles. (2018) 7(1):1506198. doi: 10.1080/20013078.2018.1506198
14. Molinaro A, Nemet I, Bel Lassen P, Chakaroun R, Nielsen T, Aron-Wisnewsky J, et al. Microbially produced imidazole propionate is associated with heart failure and mortality. JACC Heart Fail. (2023) 11: 810–21. doi: 10.1016/j.jchf.2023.03.008
15. Zaher A, Elsaygh J, Peterson SJ, Weisberg IS, Parikh MA, Frishman WH. The interplay of microbiome dysbiosis and cardiovascular disease. Cardiol Rev. (2024). doi: 10.1097/crd.0000000000000701
16. Xu H, Yang F, Bao Z. Gut microbiota and myocardial fibrosis. Eur J Pharmacol. (2023) 940:175355. doi: 10.1016/j.ejphar.2022.175355
17. Martin-Gallausiaux C, Marinelli L, Blottière HM, Larraufie P, Lapaque N. SCFA: mechanisms and functional importance in the gut. Proc Nutr Soc. (2020) 80:37–49. doi: 10.1017/S0029665120006916
18. Sanna S, van Zuydam NR, Mahajan A, Kurilshikov A, Vich Vila A, Võsa U, et al. Causal relationships among the gut microbiome, short-chain fatty acids and metabolic diseases. Nat Genet. (2019) 51:600–5. doi: 10.1038/s41588-019-0350-x
19. Zhang Z, Lv T, Wang X, Wu M, Zhang R, Yang X, et al. Role of the microbiota–gut–heart axis between bile acids and cardiovascular disease. Biomed Pharmacother. (2024) 174:116567. doi: 10.1016/j.biopha.2024.116567
20. Zheng H, Zhang X, Li C, Wang D, Shen Y, Lu J, et al. BCAA Mediated microbiota-liver-heart crosstalk regulates diabetic cardiomyopathy via FGF21. Microbiome. (2024) 12(1):157. doi: 10.1186/s40168-024-01872-3
21. Zhang Y, Wang Y, Ke B, Du J. TMAO: how gut microbiota contributes to heart failure. Transl Res. (2021) 228:109–25. doi: 10.1016/j.trsl.2020.08.007
22. Cheng T-Y, Lee T-W, Li S-J, Lee T-I, Chen Y-C, Kao Y-H, et al. Short-chain fatty acid butyrate against TMAO activating endoplasmic-reticulum stress and PERK/IRE1-axis with reducing atrial arrhythmia. J Adv Res. (2024) S2090-1232(24):00332–1. doi: 10.1016/j.jare.2024.08.009
23. Wilcox J, Skye SM, Graham B, Zabell A, Li XS, Li L, et al. Dietary choline supplements, but not eggs, raise fasting TMAO levels in participants with normal renal function: a randomized clinical trial. Am J Med. (2021) 134:1160–9.e3. doi: 10.1016/j.amjmed.2021.03.016
24. Gu Y, Jin Q, Hu J, Wang X, Yu W, Wang Z, et al. Causality of genetically determined metabolites and metabolic pathways on osteoarthritis: a two-sample Mendelian randomization study. J Transl Med. (2023) 21(1):357. doi: 10.1186/s12967-023-04165-9
25. Saaoud F, Liu L, Xu K, Cueto R, Shao Y, Lu Y, et al. Aorta- and liver-generated TMAO enhances trained immunity for increased inflammation via ER stress/mitochondrial ROS/glycolysis pathways. JCI Insight. (2023) 8(1):e158183. doi: 10.1172/jci.insight.158183
26. Wang Z, Klipfell E, Bennett BJ, Koeth R, Levison BS, DuGar B, et al. Gut flora metabolism of phosphatidylcholine promotes cardiovascular disease. Nature. (2011) 472:57–63. doi: 10.1038/nature09922
27. Chakaroun RM, Olsson LM, Bäckhed F. The potential of tailoring the gut microbiome to prevent and treat cardiometabolic disease. Nat Rev Cardiol. (2022) 20:217–35. doi: 10.1038/s41569-022-00771-0
28. Zhou X, Li J, Guo J, Geng B, Ji W, Zhao Q, et al. Gut-dependent microbial translocation induces inflammation and cardiovascular events after ST-elevation myocardial infarction. Microbiome. (2018) 6(1):66. doi: 10.1186/s40168-018-0441-4
29. Ahlawat S, Asha Sharma KK. Gut–organ axis: a microbial outreach and networking. Lett Appl Microbiol. (2020) 72:636–68. doi: 10.1111/lam.13333
30. Yukino-Iwashita M, Nagatomo Y, Kawai A, Taruoka A, Yumita Y, Kagami K, et al. Short-chain fatty acids in gut–heart axis: their role in the pathology of heart failure. J Pers Med. (2022) 12(11):1805. doi: 10.3390/jpm12111805
31. Yamaguchi T. Atrial structural remodeling and atrial fibrillation substrate: a histopathological perspective. J Cardiol. (2024) S0914-5087(24):00096-0. doi: 10.1016/j.jjcc.2024.05.007
32. Yamaguchi T, Otsubo T, Takahashi Y, Nakashima K, Fukui A, Hirota K, et al. Atrial structural remodeling in patients with atrial fibrillation is a diffuse fibrotic process: evidence from high-density voltage mapping and atrial biopsy. J Am Heart Assoc. (2022) 11(6):e024521. doi: 10.1161/JAHA.121.024521
33. Jalife J, Kaur K. Atrial remodeling, fibrosis, and atrial fibrillation. Trends Cardiovasc Med. (2015) 25:475–84. doi: 10.1016/j.tcm.2014.12.015
34. Burstein B, Nattel S. Atrial fibrosis: mechanisms and clinical relevance in atrial fibrillation. J Am Coll Cardiol. (2008) 51:802–9. doi: 10.1016/j.jacc.2007.09.064
35. Dzeshka MS, Snezhitskiy V, Shantsila E. Cardiac fibrosis in patients with atrial fibrillation: mechanisms and clinical implications. J Am Coll Cardiol. (2015) 66(8):943–59. doi: 10.1016/j.jacc.2015.06.1313
36. Xintarakou A, Tzeis S, Psarras S, Asvestas D, Vardas P. Atrial fibrosis as a dominant factor for the development of atrial fibrillation: facts and gaps. Europace. (2020) 22(3):342–51. doi: 10.1093/europace/euaa009
37. Ciacci AFM, Manani KA, Evans TS, Peters NS, Christensen K. Understanding the transition from paroxysmal to persistent atrial fibrillation. Phys Rev Res. (2020) 2:023311. doi: 10.1103/PhysRevResearch.2.023311
38. Goette A, Lendeckel U. Atrial cardiomyopathy: pathophysiology and clinical consequences. Cells. (2021) 10(10):2605. doi: 10.3390/cells10102605
39. Li M, Ning Y, Tse G, Saguner AM, Wei M, Day JD, et al. Atrial cardiomyopathy: from cell to bedside. ESC Heart Failure. (2022) 9:3768–84. doi: 10.1002/ehf2.14089
40. Guichard JB, Nattel S. Atrial cardiomyopathy: a useful notion in cardiac disease management or a passing fad? J Am Coll Cardiol. (2017) 70(6):756–65. doi: 10.1016/j.jacc.2017.06.033
41. Hoit BD. Assessing atrial mechanical remodeling and its consequences. Circulation. (2005) 112:304–6. doi: 10.1161/CIRCULATIONAHA.105.547331
42. Cianciulli TF. Two-dimensional speckle tracking echocardiography for the assessment of atrial function. World J Cardiol. (2010) 2:163–70. doi: 10.4330/wjc.v2.i7.163
43. Inoue K, Khan FH, Remme EW, Ohte N, García-Izquierdo E, Chetrit M, et al. Determinants of left atrial reservoir and pump strain and use of atrial strain for evaluation of left ventricular filling pressure. Eur Heart J Cardiovasc Imaging. (2022) 23:61–70. doi: 10.1093/ehjci/jeaa415
44. Qin D, Mansour MC, Ruskin JN, Heist EK. Atrial fibrillation–mediated cardiomyopathy. Circ Arrhythm Electrophysiol. (2019) 12(12):e007809. doi: 10.1161/circep.119.007809
45. La Rosa G, Morillo CA, Quintanilla JG, Doltra A, Mont L, Rodríguez-Mañero M, et al. Practical approach for atrial cardiomyopathy characterization in patients with atrial fibrillation. Revista Española de Cardiología (English Edition). (2024) 77:656–66. doi: 10.1016/j.rec.2024.02.009
46. Boriani G, Gerra L, Mantovani M, Tartaglia E, Mei DA, Imberti JF, et al. Atrial cardiomyopathy: an entity of emerging interest in the clinical setting. Eur J Intern Med. (2023) 118:14–21. doi: 10.1016/j.ejim.2023.10.023
47. Benussi S, de Maat GE. Atrial remodelling and function: implications for atrial fibrillation surgery. Eur J Cardiothorac Surg. (2018) 53:i2–8. doi: 10.1093/ejcts/ezx340
48. Liu Y, Neogi A, Mani A. The role of wnt signalling in development of coronary artery disease and its risk factors. Open Biol. (2020) 10(10):200128. doi: 10.1098/rsob.200128
49. Tao H, Yang J-J, Shi K-H, Li J. Wnt signaling pathway in cardiac fibrosis: new insights and directions. Metab Clin Exp. (2016) 65:30–40. doi: 10.1016/j.metabol.2015.10.013
50. Yu M, Qin K, Fan J, Zhao G, Zhao P, Zeng W, et al. The evolving roles of wnt signaling in stem cell proliferation and differentiation, the development of human diseases, and therapeutic opportunities. Genes Dis. (2024) 11(3):101026. doi: 10.1016/j.gendis.2023.04.042
51. Liu J, Xiao Q, Xiao J, Niu C, Li Y, Zhang X, et al. Wnt/β-catenin signalling: function, biological mechanisms, and therapeutic opportunities. Signal Transduct Target Ther. (2022) 7(1):3. doi: 10.1038/s41392-021-00762-6
52. Brown EM, Clardy J, Xavier RJ. Gut microbiome lipid metabolism and its impact on host physiology. Cell Host Microbe. (2023) 31(2):173–86. doi: 10.1016/j.chom.2023.01.009
53. Kim S, Seo S-U, Kweon M-N. Gut microbiota-derived metabolites tune host homeostasis fate. Semin Immunopathol. (2024) 46(1–2):2. doi: 10.1007/s00281-024-01012-x
54. Sasidharan Pillai S, Gagnon CA, Foster C, Ashraf AP. Exploring the gut Microbiota: key insights into its role in obesity, metabolic syndrome, and type 2 diabetes. J Clin Endocrinol Metab. (2024) 109(11):2709–19. doi: 10.1210/clinem/dgae499
55. Campos-Perez W, Martinez-Lopez E. Effects of short chain fatty acids on metabolic and inflammatory processes in human health. Biochim Biophys Acta Mol Cell Biol Lipids. (2021) 1866(5):158900. doi: 10.1016/j.bbalip.2021.158900
56. Yao Y, Cai X, Fei W, Ye Y, Zhao M, Zheng C. The role of short-chain fatty acids in immunity, inflammation and metabolism. Crit Rev Food Sci Nutr. (2020) 62(1):1–12. doi: 10.1080/10408398.2020.1854675
57. Gong R, Jiang Z, Zagidullin N, Liu T, Cai B. Regulation of cardiomyocyte fate plasticity: a key strategy for cardiac regeneration. Signal Transduct Target Ther. (2021) 6(1):31. doi: 10.1038/s41392-020-00413-2
58. Teo J-L, Kahn M. The wnt signaling pathway in cellular proliferation and differentiation: a tale of two coactivators. Adv Drug Delivery Rev. (2010) 62(12):1149–55. doi: 10.1016/j.addr.2010.09.012
59. Wang T, Wang X, Ren W, Sun Z, Zhang Y, Wu N, et al. Cardiomyocyte proliferation: advances and insights in macrophage-targeted therapy for myocardial injury. Genes Dis. (2024. doi: 10.1016/j.gendis.2024.101332
60. van den Munckhof ICL, Kurilshikov A, ter Horst R, Riksen NP, Joosten LAB, Zhernakova A, et al. Role of gut microbiota in chronic low-grade inflammation as potential driver for atherosclerotic cardiovascular disease: a systematic review of human studies. Obes Rev. (2018) 19(12):1719–34. doi: 10.1111/obr.12750
61. Bhol NK, Bhanjadeo MM, Singh AK, Dash UC, Ojha RR, Majhi S, et al. The interplay between cytokines, inflammation, and antioxidants: mechanistic insights and therapeutic potentials of various antioxidants and anti-cytokine compounds. Biomed Pharmacother. (2024) 178:117177. doi: 10.1016/j.biopha.2024.117177
62. Xu J, Núñez G. The NLRP3 inflammasome: activation and regulation. Trends Biochem Sci. (2023) 48(4):331–44. doi: 10.1016/j.tibs.2022.10.002
63. Kelley N, Jeltema D, Duan Y, He Y. The NLRP3 inflammasome: an overview of mechanisms of activation and regulation. Int J Mol Sci. (2019) 20(13):3328. doi: 10.3390/ijms20133328
64. Candelli M, Franza L, Pignataro G, Ojetti V, Covino M, Piccioni A, et al. Interaction between lipopolysaccharide and gut Microbiota in inflammatory bowel diseases. Int J Mol Sci. (2021) 22(12):6242. doi: 10.3390/ijms22126242
65. Chen L, Zhang L, Hua H, Liu L, Mao Y, Wang R. Interactions between toll-like receptors signaling pathway and gut microbiota in host homeostasis. Immun Inflamm Dis. (2024) 12(7):e1356. doi: 10.1002/iid3.1356
66. Ramachandran R, Manan A, Kim J, Choi S. NLRP3 Inflammasome: a key player in the pathogenesis of life-style disorders. Exp Mol Med. (2024) 56(7):1488–500. doi: 10.1038/s12276-024-01261-8
67. Huang Y, Xu W, Zhou R. NLRP3 Inflammasome activation and cell death. Cell Mol Immunol. (2021) 18(9):2114–27. doi: 10.1038/s41423-021-00740-6
68. Swanson KV, Deng M, Ting JPY. The NLRP3 inflammasome: molecular activation and regulation to therapeutics. Nat Rev Immunol. (2019) 19(8):477–89. doi: 10.1038/s41577-019-0165-0
69. Ajoolabady A, Nattel S, Lip GYH, Ren J. Inflammasome signaling in atrial fibrillation. J Am Coll Cardiol. (2022) 79(23):2349–66. doi: 10.1016/j.jacc.2022.03.379
70. Zhou X, Liu H, Feng F, Kang G-J, Liu M, Guo Y, et al. Macrophage IL-1β mediates atrial fibrillation risk in diabetic mice. JCI Insight. (2024) 9(15):e171102. doi: 10.1172/jci.insight.171102
71. Matacchione G, Piacenza F, Pimpini L, Rosati Y, Marcozzi S. The role of the gut microbiota in the onset and progression of heart failure: insights into epigenetic mechanisms and aging. Clin Epigenetics. (2024) 16(1):175. doi: 10.1186/s13148-024-01786-9
72. Xu Q, Wang W, Li Y, Cui J, Zhu M, Liu Y, et al.The oral-gut microbiota axis: a link in cardiometabolic diseases. NPJ Biofilms Microbiomes. (2025) 11(1):11. doi: 10.1038/s41522-025-00646-5
73. Schnabel RB, Marinelli EA, Arbelo E, Boriani G, Boveda S, Buckley CM, et al. Early diagnosis and better rhythm management to improve outcomes in patients with atrial fibrillation: the 8th AFNET/EHRA consensus conference. Europace. (2023) 25(1):6–27. doi: 10.1093/europace/euac062
74. Kosmala W, Przewłocka-Kosmala M. Atrial cardiomyopathy. ESC Heart Failure. (2024. doi: 10.1002/ehf2.15158
75. He F, Ru X, Wen T. NRF2, A transcription factor for stress response and beyond. Int J Mol Sci. (2020) 21(13):4777. doi: 10.3390/ijms21134777
76. Ngo V, Duennwald ML. Nrf2 and oxidative stress: a general overview of mechanisms and implications in human disease. Antioxidants. (2022) 11(12):2345. doi: 10.3390/antiox11122345
77. Karunatilleke NC, Fast CS, Ngo V, Brickenden A, Duennwald ML, Konermann L, et al. Nrf2, the Major regulator of the cellular oxidative stress response, is partially disordered. Int J Mol Sci. (2021) 22(14):7434. doi: 10.3390/ijms22147434
78. Pizzino G, Irrera N, Cucinotta M, Pallio G, Mannino F, Arcoraci V, et al. Oxidative stress: harms and benefits for human health. Oxid Med Cell Longevity. (2017) 2017(1):8416763. doi: 10.1155/2017/8416763
79. Wang W, Kang PM. Oxidative stress and antioxidant treatments in cardiovascular diseases. Antioxidants. (2020) 9(12):1292. doi: 10.3390/antiox9121292
80. Forman HJ, Zhang H. Targeting oxidative stress in disease: promise and limitations of antioxidant therapy. Nat Rev Drug Discovery. (2021) 20(9):689–709. doi: 10.1038/s41573-021-00233-1
81. Münzel T, Camici GG, Maack C, Bonetti NR, Fuster V, Kovacic JC. Impact of oxidative stress on the heart and vasculature. J Am Coll Cardiol. (2017) 70(2):212–29. doi: 10.1016/j.jacc.2017.05.035
82. Dridi H, Santulli G, Bahlouli L, Miotto MC, Weninger G, Marks AR. Mitochondrial calcium overload plays a causal role in oxidative stress in the failing heart. Biomolecules. (2023) 13(9):1409. doi: 10.3390/biom13091409
83. Johnson E, Albakri JS, Allemailem KS, Sultan A, Alwanian WM, Alrumaihi F, et al. Mitochondrial dysfunction and calcium homeostasis in heart failure: exploring the interplay between oxidative stress and cardiac remodeling for future therapeutic innovations. Curr Probl Cardiol. (2025) 50(3):102968. doi: 10.1016/j.cpcardiol.2024.102968
84. Li Z, Wan M, Wang M, Duan J, Jiang S. Modulation of gut microbiota on intestinal permeability: a novel strategy for treating gastrointestinal related diseases. Int Immunopharmacol. (2024) 137:112416. doi: 10.1016/j.intimp.2024.112416
85. Dmytriv TR, Storey KB, Lushchak VI. Intestinal barrier permeability: the influence of gut microbiota, nutrition, and exercise. Front Physiol. (2024) 15:1380713. doi: 10.3389/fphys.2024.1380713
86. Ma Q, Xing C, Long W, Wang HY, Liu Q, Wang R-F. Impact of microbiota on central nervous system and neurological diseases: the gut-brain axis. J Neuroinflammation. (2019) 16(1):53. doi: 10.1186/s12974-019-1434-3
87. Mann ER, Lam YK, Uhlig HH. Short-chain fatty acids: linking diet, the microbiome and immunity. Nat Rev Immunol. (2024) 24(8):577–95. doi: 10.1038/s41577-024-01014-8
88. Haghikia A, Zimmermann F, Schumann P, Jasina A, Roessler J, Schmidt D, et al. Propionate attenuates atherosclerosis by immune-dependent regulation of intestinal cholesterol metabolism. Eur Heart J. (2022) 43(6):518–33. doi: 10.1093/eurheartj/ehab644
89. He Y, Wang K, Su N, Yuan C, Zhang N, Hu X, et al. Microbiota–gut–brain axis in health and neurological disease: interactions between gut microbiota and the nervous system. J Cell Mol Med. (2024) 28(18):e70099. doi: 10.1111/jcmm.70099
90. Macpherson AJ, Pachnis V, Prinz M. Boundaries and integration between microbiota, the nervous system, and immunity. Immunity. (2023) 56(8):1712–26. doi: 10.1016/j.immuni.2023.07.011
91. da Costa Santiago H, Corral RS, Guerrero NA, Cuervo H, Gironès N, Fresno M. Trypanosoma cruzi infection and endothelin-1 cooperatively activate pathogenic inflammatory pathways in cardiomyocytes. PLoS Negl Trop Dis. (2013) 7(2):e2034. doi: 10.1371/journal.pntd.0002034
92. Chao T-F, Liu C-J, Chen S-J, Wang K-L, Lin Y-J, Chang S-L, et al. The association between the use of non-steroidal anti-inflammatory drugs and atrial fibrillation: a nationwide case–control study. Int J Cardiol. (2013) 168:312–6. doi: 10.1016/j.ijcard.2012.09.058
93. Park BM, Gao S, Cha SA, Kim SH. Attenuation of renovascular hypertension by cyclooxygenase-2 inhibitor partly through ANP release. Peptides. (2015):69:1–8. doi: 10.1016/j.peptides.2015.03.022
94. Pullen AB, Kain V, Serhan CN, Halade GV. Molecular and cellular differences in cardiac repair of male and female mice. J Am Heart Assoc. (2020) 9(8):e015672. doi: 10.1161/JAHA.119.015672
95. Cai Z-L, Shen B, Yuan Y, Liu C, Xie Q-W, Hu T-T, et al. The effect of HMGA1 in LPS-induced myocardial inflammation. Int J Biol Sci. (2020) 16:1798–810. doi: 10.7150/ijbs.39947
96. Pang L, Cai Y, Tang EHC, Yan D, Kosuru R, Li H, et al. Cox-2 inhibition protects against hypoxia/reoxygenation-induced cardiomyocyte apoptosis via akt-dependent enhancement of iNOS expression. Oxid Med Cell Longevity. (2016) 2016. doi: 10.1155/2016/3453059
97. Goodwin AT, Marchbank AJ, Gray CC, Jayakumar J, Yacoub MH. Coronary vasoconstriction to endothelin-1 increases with age before and after ischaemia and reperfusion. Cardiovasc Res. (1999) 41(3):554–62. doi: 10.1016/S0008-6363(98)00253-3
98. Westby CM, Weil BR, Greiner JJ, Stauffer BL, Desouza CA. Endothelin-1 vasoconstriction and the age-related decline in endothelium-dependent vasodilatation in men. Clin Sci. (2011) 120:485–91. doi: 10.1042/CS20100475
99. Spratt JC GJ, Patel N, Strachan FE, Rankin AJ, Webb DJ. Systemic ETA receptor antagonism with BQ-123 blocks ET-1 induced forearm vasoconstriction and decreases peripheral vascular resistance in healthy men. Br J Pharmacol. (2001) 134(3):648–54. doi: 10.1038/sj.bjp.0704304
100. Archer CR, Robinson EL, Drawnel FM, Roderick HL. Endothelin-1 promotes hypertrophic remodelling of cardiac myocytes by activating sustained signalling and transcription downstream of endothelin type A receptors. Cell Signal. (2017) 36:240–54. doi: 10.1016/j.cellsig.2017.04.010
101. Gupta SC, Patchva S, Aggarwal BB. Therapeutic roles of curcumin: lessons learned from clinical trials. AAPS J. (2012) 15:195–218. doi: 10.1208/s12248-012-9432-8
102. Bai PY, Jia DL, Pan LH, Liu CB, Liu J, Luo W, et al. Environmental eustress improves postinfarction cardiac repair via enhancing cardiac macrophage survival. Sci Adv. (2022) 8(17):eabm3436. doi: 10.1126/sciadv.abm3436
103. Bahls M, Könemann S, Markus MRP, Wenzel K, Friedrich N, Nauck M, et al. Brain-derived neurotrophic factor is related with adverse cardiac remodeling and high NTproBNP. Sci Rep. (2019) 9(1):15421. doi: 10.1038/s41598-019-51776-8
104. Zhang H, Dhalla NS. The role of pro-inflammatory cytokines in the pathogenesis of cardiovascular disease. Int J Mol Sci. (2024) 25. doi: 10.3390/ijms25021082
105. Katare RG, Tarazón E, Roselló-Lletí E, Rivera M, Ortega A, Molina-Navarro MM, et al. RNA Sequencing analysis and atrial natriuretic peptide production in patients with dilated and ischemic cardiomyopathy. PLoS One. (2014) 9. doi: 10.1371/journal.pone.0090157
106. Abassi Z, Khoury EE, Karram T, Aronson D. Edema formation in congestive heart failure and the underlying mechanisms. Front Cardiovasc Med. (2022) 9. doi: 10.3389/fcvm.2022.933215
107. Gladysheva IP, Sullivan RD, Reed GL. Falling corin and ANP activity levels accelerate development of heart failure and cardiac fibrosis. Front Cardiovasc Med. (2023) 10:1120487. doi: 10.3389/fcvm.2023.1120487
108. Dominguez-Bello MG, Blaser MJ, Ley RE, Knight R. Development of the human gastrointestinal Microbiota and insights from high-throughput sequencing. Gastroenterology. (2011) 140:1713–9. doi: 10.1053/j.gastro.2011.02.011
109. Ursell LK, Treuren WV, Metcalf JL, Pirrung M, Gewirtz A, Knight R. Replenishing our defensive microbes. BioEssays. (2013) 35:810–7. doi: 10.1002/bies.201300018
110. Donald K, Finlay BB. Early-life interactions between the microbiota and immune system: impact on immune system development and atopic disease. Nat Rev Immunol. (2023) 23:735–48. doi: 10.1038/s41577-023-00874-w
111. Song SJ, Lauber C, Costello EK, Lozupone CA, Humphrey G, Berg-Lyons D, et al. Cohabiting family members share microbiota with one another and with their dogs. eLife. (2013) 2:e00458. doi: 10.7554/eLife.00458
112. Wu GD, Hoffmann C, Bittinger K, Chen YY, Keilbaugh SA, Bewtra M, et al. Linking long-term dietary patterns with gut microbial enterotypes. Science. (2011) 334(6052):105–8. doi: 10.1126/science.1208344
113. David LA, Maurice CF, Carmody RN, Gootenberg DB, Button JE, Wolfe BE, et al. Diet rapidly and reproducibly alters the human gut microbiome. Nature. (2013) 505:559–63. doi: 10.1038/nature12820
114. Gibson MK, Crofts TS, Dantas G. Antibiotics and the developing infant gut microbiota and resistome. Curr Opin Microbiol. (2015) 27:51–6. doi: 10.1016/j.mib.2015.07.007
115. Langille MGI, Zaneveld J, Caporaso JG, McDonald D, Knights D, Reyes JA, et al. Predictive functional profiling of microbial communities using 16S rRNA marker gene sequences. Nat Biotechnol. (2013) 31:814–21. doi: 10.1038/nbt.2676
116. Tierney BT, Yang Z, Luber JM, Beaudin M, Wibowo MC, Baek C, et al. The landscape of genetic content in the gut and oral human microbiome. Cell Host Microbe. (2019) 26:283–95.e8. doi: 10.1016/j.chom.2019.07.008
117. Chen L, Wang D, Garmaeva S, Kurilshikov A, Vich Vila A, Gacesa R, et al. The long-term genetic stability and individual specificity of the human gut microbiome. Cell. (2021) 184:2302–15.e12. doi: 10.1016/j.cell.2021.03.024
118. Deng K, Shuai M, Zhang Z, Jiang Z, Fu Y, Shen L, et al. Temporal relationship among adiposity, gut microbiota, and insulin resistance in a longitudinal human cohort. BMC Med. (2022) 20(1):171. doi: 10.1186/s12916-022-02376-3
119. Erny D, Hrabě de Angelis AL, Jaitin D, Wieghofer P, Staszewski O, David E, et al. Host microbiota constantly control maturation and function of microglia in the CNS. Nat Neurosci. (2015) 18:965–77. doi: 10.1038/nn.4030
120. Consortium HMP. Structure, function and diversity of the healthy human microbiome. Nature. (2012) 486:207–14. doi: 10.1038/nature11234
121. Costello EK, Hamady M, Fierer N, Gordon JI, Knight R. Bacterial community variation in human body habitats across space and time. Science. (2009) 326(5960):1694–7. doi: 10.1126/science.1177486
122. Qin J, Li R, Raes J, Arumugam M, Burgdorf KS, Manichanh C, et al. A human gut microbial gene catalogue established by metagenomic sequencing. Nature. (2010) 464:59–65. doi: 10.1038/nature08821
123. Haiser HJ, Turnbaugh PJ. Is it time for a metagenomic basis of therapeutics? Science. (2012) 336:1253–5. doi: 10.1126/science.1224396
124. Marra AHM, Kondo S, Erkosar B, Lemaitre B. Drosophila Antimicrobial peptides and lysozymes regulate gut microbiota composition and abundance. mBio. (2021) 12(4):e0082421. doi: 10.1128/mBio.00824-21
125. Rogers GB, Keating DJ, Young RL, Wong ML, Licinio J, Wesselingh S. From gut dysbiosis to altered brain function and mental illness: mechanisms and pathways. Mol Psychiatry. (2016) 21:738–48. doi: 10.1038/mp.2016.50
126. Hills R, Pontefract B, Mishcon H, Black C, Sutton S, Theberge C. Gut microbiome: profound implications for diet and disease. Nutrients. (2019) 11(7):1613. doi: 10.3390/nu11071613
127. Sergeev IN, Aljutaily T, Walton G, Huarte E. Effects of synbiotic supplement on human gut Microbiota, body composition and weight loss in obesity.nutrients. (2020) 12(1):222. doi: 10.3390/nu12010222
128. Rosenberg E. Diversity of bacteria within the human gut and its contribution to the functional unity of holobionts. NPJ Biofilms Microbiomes. (2024) 10(1):134. doi: 10.1038/s41522-024-00580-y
129. Fujisaka S, Watanabe Y, Tobe K. The gut microbiome: a core regulator of metabolism. J Endocrinol. (2023) 256(3):e220111. doi: 10.1530/JOE-22-0111
130. Ma J, Piao X, Mahfuz S, Long S, Wang J. The interaction among gut microbes, the intestinal barrier and short chain fatty acids. Animal Nutrition. (2022) 9:159–74. doi: 10.1016/j.aninu.2021.09.012
131. Wang B, Yao M, Lv L, Ling Z, Li L. The human Microbiota in health and disease. Engineering. (2017) 3(1):71–82. doi: 10.1016/J.ENG.2017.01.008
132. Fan Y, Pedersen O. Gut microbiota in human metabolic health and disease. Nat Rev Microbiol. (2020) 19:55–71. doi: 10.1038/s41579-020-0433-9
133. Di Vincenzo F, Del Gaudio A, Petito V, Lopetuso LR, Scaldaferri F. Gut microbiota, intestinal permeability, and systemic inflammation: a narrative review. Intern Emerg Med. (2023) 19:275–93. doi: 10.1007/s11739-023-03374-w
134. Ma Z, Chu J, Feng S, Guo C, Xue B, He K, et al. Immunological mechanisms of inflammatory diseases caused by gut microbiota dysbiosis: a review. Biomed Pharmacother. (2023) 164:114985. doi: 10.1016/j.biopha.2023.114985
135. Kim CH. Complex regulatory effects of gut microbial short-chain fatty acids on immune tolerance and autoimmunity. Cell Mol Immunol. (2023) 20:341–50. doi: 10.1038/s41423-023-00987-1
136. van der Hee B, Wells JM. Microbial regulation of host physiology by shortchain fatty acids. Trends Microbiol. (2021) 29:700–12. doi: 10.1016/j.tim.2021.02.001
137. Chelakkot C, Ghim J, Ryu SH. Mechanisms regulating intestinal barrier integrity and its pathological implications. Exp Mol Med. (2018) 50:1–9. doi: 10.1038/s12276-018-0126-x
138. Shin W, Kim HJ. Intestinal barrier dysfunction orchestrates the onset of inflammatory host–microbiome cross-talk in a human gut inflammation-on-a-chip. Proc Natl Acad Sci USA. (2018) 115(45):E10539–47. doi: 10.1073/pnas.1810819115
139. Sun W, Du D, Fu T, Han Y, Li P, Ju H. Alterations of the gut Microbiota in patients with severe chronic heart failure. Front Microbiol. (2022) 12:813289. doi: 10.3389/fmicb.2021.813289
140. Masenga SK, Hamooya B, Hangoma J, Hayumbu V, Ertuglu LA, Ishimwe J, et al. Recent advances in modulation of cardiovascular diseases by the gut microbiota. J Hum Hypertens. (2022) 36:952–9. doi: 10.1038/s41371-022-00698-6
141. Loh JS, Mak WQ, Tan LKS, Ng CX, Chan HH, Yeow SH, et al. Microbiota–gut–brain axis and its therapeutic applications in neurodegenerative diseases. Signal Transduct Target Ther. (2024) 9(1):37. doi: 10.1038/s41392-024-01743-1
142. Gawałko M, Agbaedeng TA, Saljic A, Müller DN, Wilck N, Schnabel R, et al. Gut microbiota, dysbiosis and atrial fibrillation. Arrhythmogenic mechanisms and potential clinical implications. Cardiovasc Res. (2022) 118:2415–27. doi: 10.1093/cvr/cvab292
143. Linz D, Gawałko M, Sanders P, Penders J, Li N, Nattel S, et al. Does gut microbiota affect atrial rhythm? Causalities and speculations. Eur Heart J. (2021) 42:3521–5. doi: 10.1093/eurheartj/ehab467
144. Pluznick JL. Microbial short-chain fatty acids and blood pressure regulation. Curr Hypertens Rep. (2017) 19(4):25. doi: 10.1007/s11906-017-0722-5
145. Arab JP, Martin-Mateos RM, Shah VH. Gut–liver axis, cirrhosis and portal hypertension: the chicken and the egg. Hepatol Int. (2017) 12:24–33. doi: 10.1007/s12072-017-9798-x
146. Carpenito M, Fanti D, Mega S, Benfari G, Bono MC, Rossi A, et al. The central role of left atrium in heart failure. Front Cardiovasc Med. (2021) 813(8):704762. doi: 10.3389/fcvm.2021.704762
147. Morris DA, Belyavskiy E, Aravind-Kumar R, Kropf M, Frydas A, Braunauer K, et al. Potential usefulness and clinical relevance of adding left atrial strain to left atrial volume index in the detection of left ventricular diastolic dysfunction. JACC Cardiovasc Imaging. (2018) 11:1405–15. doi: 10.1016/j.jcmg.2017.07.029
148. Vanoverschelde JL, Raphael D, Robert AR, Cosyns JR. Left ventricular filling in dilated cardiomyopathy: relation to functional class and hemodynamics. J Am Coll Cardiol. (1990) 15(6):1288–95. doi: 10.1016/S0735-1097(10)80016-6
149. Potter EL, Ramkumar S, Kawakami H, Yang H, Wright L, Negishi T, et al. Association of asymptomatic diastolic dysfunction assessed by left atrial strain with incident heart failure. JACC Cardiovasc Imaging. (2020) 13:2316–26. doi: 10.1016/j.jcmg.2020.04.028
150. Inciardi RM, Claggett B, Minamisawa M, Shin S-H, Selvaraj S, Gonçalves A, et al. Association of left atrial structure and function with heart failure in older adults. J Am Coll Cardiol. (2022) 79:1549–61. doi: 10.1016/j.jacc.2022.01.053
151. Tang WHW, Li DY, Hazen SL. Dietary metabolism, the gut microbiome, and heart failure. Nat Rev Cardiol. (2018) 16:137–54. doi: 10.1038/s41569-018-0108-7
152. Krack A, Sharma R, Figulla HR, Anker SD. The importance of the gastrointestinal system in the pathogenesis of heart failure. Eur Heart J. (2005) 26:2368–74. doi: 10.1093/eurheartj/ehi389
153. Agus A, Clément K, Sokol H. Gut microbiota-derived metabolites as central regulators in metabolic disorders. Gut. (2021) 70:1174–82. doi: 10.1136/gutjnl-2020-323071
154. Wen Y, Sun Z, Xie S, Hu Z, Lan Q, Sun Y, et al. Intestinal Flora derived metabolites affect the occurrence and development of cardiovascular disease. J Multidiscip Healthc. (2022) 15:2591–603. doi: 10.2147/JMDH.S367591
155. Trøseid M, Andersen GØ, Broch K, Hov JR. The gut microbiome in coronary artery disease and heart failure: current knowledge and future directions. EBioMedicine. (2020) 52:102649. doi: 10.1016/j.ebiom.2020.102649
156. Rau M, Rehman A, Dittrich M, Groen AK, Hermanns HM, Seyfried F, et al. Fecal SCFAs and SCFA-producing bacteria in gut microbiome of human NAFLD as a putative link to systemic T-cell activation and advanced disease. United European Gastroenterol J. (2018) 6:1496–507. doi: 10.1177/2050640618804444
157. O'Riordan KJ, Collins MK, Moloney GM, Knox EG, Aburto MR, Fülling C, et al. Short chain fatty acids: microbial metabolites for gut-brain axis signalling. Mol Cell Endocrinol. (2022) 546:111572. doi: 10.1016/j.mce.2022.111572
158. Ojeda J, Ávila A, Vidal PM. Gut microbiota interaction with the central nervous system throughout life. J Clin Med. (2021) 10(6):1299. doi: 10.3390/jcm10061299
159. Tsai H-J, Tsai W-C, Hung W-C, Hung W-W, Chang C-C, Dai C-Y, et al. Gut Microbiota and subclinical cardiovascular disease in patients with type 2 diabetes Mellitus. Nutrients. (2021) 13(8):2679. doi: 10.3390/nu13082679
160. Luo Q, Hu Y, Chen X, Luo Y, Chen J, Wang H. Effects of gut Microbiota and metabolites on heart failure and its risk factors: a two-sample Mendelian randomization study. Front Nutr. (2022) 9:899746. doi: 10.3389/fnut.2022.899746
161. Koeth RA, Wang Z, Levison BS, Buffa JA, Org E, Sheehy BT, et al. Intestinal microbiota metabolism of l-carnitine, a nutrient in red meat, promotes atherosclerosis. Nat Med. (2013) 19:576–85. doi: 10.1038/nm.3145
162. Warrier M, Shih Diana M, Burrows Amy C, Ferguson D, Gromovsky Anthony D, Brown Amanda L, et al. The TMAO-generating enzyme flavin monooxygenase 3 is a central regulator of cholesterol balance. Cell Rep. (2015) 10:326–38. doi: 10.1016/j.celrep.2014.12.036
163. Seldin MM, Meng Y, Qi H, Zhu W, Wang Z, Hazen SL, et al. Trimethylamine N-oxide promotes vascular inflammation through signaling of mitogen-activated protein kinase and nuclear factor-κB. J Am Heart Assoc. (2016) 5(2):e002767. doi: 10.1161/JAHA.115.002767
164. Yue C, Yang X, Li J, Chen X, Zhao X, Chen Y, et al. Trimethylamine N-oxide prime NLRP3 inflammasome via inhibiting ATG16L1-induced autophagy in colonic epithelial cells. Biochem Biophys Res Commun. (2017) 490:541–51. doi: 10.1016/j.bbrc.2017.06.075
165. Li T, Chen Y, Gua C, Li X. Elevated circulating trimethylamine N-oxide levels contribute to endothelial dysfunction in aged rats through vascular inflammation and oxidative stress. Front Physiol. (2017) 8:350. doi: 10.3389/fphys.2017.00350
166. Organ CL, Otsuka H, Bhushan S, Wang Z, Bradley J, Trivedi R, et al. Choline diet and its gut microbe–derived metabolite, trimethylamine N-oxide. Exacerbate Pressure Overload–Induced Heart Failure. Circ Heart Fail. (2016) 9(1):e002314. doi: 10.1161/circheartfailure.115.002314
167. Chakraborty P, Banerjee D, Majumder P, Sarkar J. Gut microbiota nexus: exploring the interactions with the brain, heart, lungs, and skin axes and their effects on health. Med Microecol. (2024) `20. doi: 10.1016/j.medmic.2024.100104
168. Tang WHW, Bäckhed F, Landmesser U, Hazen SL. Intestinal Microbiota in cardiovascular health and disease. J Am Coll Cardiol. (2019) 73:2089–105. doi: 10.1016/j.jacc.2019.03.024
169. Brown JM, Hazen SL. Microbial modulation of cardiovascular disease. Nat Rev Microbiol. (2018) 16:171–81. doi: 10.1038/nrmicro.2017.149
170. Jie Z, Xia H, Zhong S-L, Feng Q, Li S, Liang S, et al. The gut microbiome in atherosclerotic cardiovascular disease. Nat Commun. (2017) 8(1):845. doi: 10.1038/s41467-017-00900-1
171. Karlsson FH, Fåk F, Nookaew I, Tremaroli V, Fagerberg B, Petranovic D, et al. Symptomatic atherosclerosis is associated with an altered gut metagenome. Nat Commun. (2012) 3:1245. doi: 10.1038/ncomms2266
172. Roberts AB, Gu X, Buffa JA, Hurd AG, Wang Z, Zhu W, et al. Development of a gut microbe–targeted nonlethal therapeutic to inhibit thrombosis potential. Nat Med. (2018) 24:1407–17. doi: 10.1038/s41591-018-0128-1
173. Buffa JA, Romano KA, Copeland MF, Cody DB, Zhu W, Galvez R, et al. The microbial gbu gene cluster links cardiovascular disease risk associated with red meat consumption to microbiota l-carnitine catabolism. Nat Microbiol. (2021) 7:73–86. doi: 10.1038/s41564-021-01010-x
174. Lindskog Jonsson A, Caesar R, Akrami R, Reinhardt C, Fåk Hållenius F, Borén J, et al. Impact of gut Microbiota and diet on the development of atherosclerosis in apoe−/− mice. Arterioscler Thromb Vasc Biol. (2018) 38:2318–26. doi: 10.1161/ATVBAHA.118.311233
175. Wilck N, Matus MG, Kearney SM, Olesen SW, Forslund K, Bartolomaeus H, et al. Salt-responsive gut commensal modulates TH17 axis and disease. Nature. (2017) 55:1585–9. doi: 10.1038/nature24628
176. Zhu W, Romano KA, Li L, Buffa JA, Sangwan N, Prakash P, et al. Gut microbes impact stroke severity via the trimethylamine N-oxide pathway. Cell Host Microbe. (2021) 29:1199–208.e5. doi: 10.1016/j.chom.2021.05.002
177. Mojsak P, Rey-Stolle F, Parfieniuk E, Kretowski A, Ciborowski M. The role of gut microbiota (GM) and GM-related metabolites in diabetes and obesity. A review of analytical methods used to measure GM-related metabolites in fecal samples with a focus on metabolites’ derivatization step. J Pharmaceut Biomed Analysis. (2020) 191:113617. doi: 10.1016/j.jpba.2020.113617
178. Al-Kaisey AM, Figgett W, Hawson J, Mackay F, Joseph SA, Kalman JM. Gut Microbiota and atrial fibrillation: pathogenesis, mechanisms and therapies. Arrhythm Electrophysiol Rev. (2023) 12:e14. doi: 10.15420/aer.2022.33
179. Lu D, Zou X, Zhang H. The relationship between atrial fibrillation and intestinal flora with its metabolites. Front Cardiovasc Med. (2022) 9:948755. doi: 10.3389/fcvm.2022.948755
180. Palmu J, Börschel CS, Ortega-Alonso A, Markó L, Inouye M, Jousilahti P, et al. Gut microbiome and atrial fibrillation—results from a large population-based study. eBioMedicine. (2023) 91:104583. doi: 10.1016/j.ebiom.2023.104583
181. Da Dalt L, Cabodevilla AG, Goldberg IJ, Norata GD. Cardiac lipid metabolism, mitochondrial function, and heart failure. Cardiovasc Res. (2023) 119(10):1905–14. doi: 10.1093/cvr/cvad100
182. Companys J, Pla-Pagà L, Calderón-Pérez L, Llauradó E, Solà R, Pedret A, et al. Fermented dairy products, probiotic supplementation, and cardiometabolic diseases: a systematic review and meta-analysis. Adv Nutr. (2020) 11(4):834–63. doi: 10.1093/advances/nmaa030
183. Yang W, Cong Y. Gut microbiota-derived metabolites in the regulation of host immune responses and immune-related inflammatory diseases. Cell Mol Immunol. (2021) 18(4):866–77. doi: 10.1038/s41423-021-00661-4
184. Rooks MG, Garrett WS. Gut microbiota, metabolites and host immunity. Nat Rev Immunol. (2016) 16(6):341–52. doi: 10.1038/nri.2016.42
185. Liu Y, Du Z, Lu Y, Ma Y, Yang Y, Osmanaj F, et al. Gut microbiota metabolism disturbance is associated with postoperative atrial fibrillation after coronary artery bypass grafting. NPJ Cardiovasc Health. (2024) 1. doi: 10.1038/s44325-024-00003-z
186. Tian H, Wang X, Fang Z, Li L, Wu C, Bi D, et al. Fecal microbiota transplantation in clinical practice: present controversies and future prospects. hLife. (2024) 2(6):269–83. doi: 10.1016/j.hlife.2024.01.006
187. Karakasis P, Theofilis P, Vlachakis PK, Korantzopoulos P, Patoulias D, Antoniadis AP, et al. Atrial fibrosis in atrial fibrillation: mechanistic insights, diagnostic challenges, and emerging therapeutic targets. Int J Mol Sci. (2024) 26(1):209. doi: 10.3390/ijms26010209
188. Mach F, Baigent C, Catapano AL, Koskinas KC, Casula M, Badimon L, et al. 2019 ESC/EAS guidelines for the management of dyslipidaemias: lipid modification to reduce cardiovascular risk. Eur Heart J. (2020) 41: 111–88. doi: 10.1093/eurheartj/ehz455
189. Calder PC. Omega-3 fatty acids and inflammatory processes: from molecules to man. Biochem Soc Trans. (2017) 45(5):1105–15. doi: 10.1042/BST20160474
190. Singh RK, Chang H-W, Yan D, Lee KM, Ucmak D, Wong K, et al. Influence of diet on the gut microbiome and implications for human health. J Transl Med. (2017) 141(16):e750–72. doi: 10.1186/s12967-017-1175-y
191. Ghodeshwar GK, Dube A, Khobragade D. Impact of lifestyle modifications on cardiovascular health: a narrative review. Cureus. (2023) 15(7):e42616. doi: 10.7759/cureus.42616
192. Chung MK, Eckhardt LL, Chen LY, Ahmed HM, Gopinathannair R, Joglar JA, et al. Lifestyle and risk factor modification for reduction of atrial fibrillation: a scientific statement from the American Heart Association. Circulation. (2020) 141(16):e750–e77. doi: 10.1161/CIR.0000000000000748
193. Vermeer JR, van den Broek JLPM, Dekker LRC. Impact of lifestyle risk factors on atrial fibrillation: mechanisms and prevention approaches—a narrative review. Int J Cardiol Cardiovasc Risk Prev. (2024) 23:200344. doi: 10.1016/j.ijcrp.2024.200344
194. Kaminsky LA, German C, Imboden M, Ozemek C, Peterman JE, Brubaker PH. The importance of healthy lifestyle behaviors in the prevention of cardiovascular disease. Prog Cardiovasc Dis. (2022) 70:8–15. doi: 10.1016/j.pcad.2021.12.001
195. Monda V, Villano I, Messina A, Valenzano A, Esposito T, Moscatelli F, et al. Exercise modifies the gut Microbiota with positive health effects. Oxid Med Cell Longevity. (2017) 2017(1):3831972. doi: 10.1155/2017/3831972
196. Varghese S, Rao S, Khattak A, Zamir F, Chaari A. Physical exercise and the gut microbiome: a bidirectional relationship influencing health and performance. Nutrients. (2024) 16(21):3663. doi: 10.3390/nu16213663
197. Mailing LJ, Allen JM, Buford TW, Fields CJ, Woods JA. Exercise and the gut microbiome: a review of the evidence, potential mechanisms, and implications for human health. Exerc Sport Sci Rev. (2019) 47(2):75–85. doi: 10.1249/jes.0000000000000183
198. Zhang L, Li H, Song Z, Liu Y, Zhang X. Dietary strategies to improve exercise performance by modulating the gut Microbiota. Foods. (2024) 13(11):1680. doi: 10.3390/foods13111680
199. Lavie CJ, Arena R, Swift DL, Johannsen NM, Sui X, Lee D-C, et al. Exercise and the cardiovascular system. Circ Res. (2015) 117(2):207–19. doi: 10.1161/CIRCRESAHA.117.305205
200. Finocchiaro G, Mannakkara NN. Exercise and the heart: benefits, risks and adverse effects of exercise training. Rev Cardiovasc Med. (2023) 24(3):94. doi: 10.31083/j.rcm2403094
201. Kemi OJ. Exercise and calcium in the heart. Curr Opin Physiol. (2023) 32:100644. doi: 10.1016/j.cophys.2023.100644
202. Rowe SJ, Paratz ED, Foulkes SJ, Janssens K, Spencer LW, Fahy L, et al. Understanding exercise capacity: from elite athlete to HFpEF. Can J Cardiol. (2023) 39(11):S323–S34. doi: 10.1016/j.cjca.2023.08.007
203. Amin KD, Thakkar A, Budampati T, Matai S, Akkaya E, Shah NP. A good night’s rest: a contemporary review of sleep and cardiovascular health. Am J Preventive Cardiology. (2025) 21:100924. doi: 10.1016/j.ajpc.2024.100924
204. Chattu VK, Manzar MD, Kumary S, Burman D, Spence DW, Pandi-Perumal SR. The global problem of insufficient sleep and its serious public health implications. Healthcare. (2018) 7(1):1. doi: 10.3390/healthcare7010001
205. Scott AJ, Webb TL, Martyn-St James M, Rowse G, Weich S. Improving sleep quality leads to better mental health: a meta-analysis of randomised controlled trials. Sleep Med Rev. (2021) 60:101556. doi: 10.1016/j.smrv.2021.101556
206. Xie W, Lu D, Liu S, Li J, Li R. The optimal exercise intervention for sleep quality in adults: a systematic review and network meta-analysis. Prev Med. (2024) 183:107955. doi: 10.1016/j.ypmed.2024.107955
207. Szliszka E, Czuba ZP, Domino M, Mazur B, Zydowicz G, Krol W. Ethanolic extract of propolis (EEP) enhances the apoptosis- inducing potential of TRAIL in cancer cells. Molecules. (2009) 14:738–54. doi: 10.3390/molecules14020738
208. Sanders ME, Merenstein DJ, Reid G, Gibson GR, Rastall RA. Probiotics and prebiotics in intestinal health and disease: from biology to the clinic. Na Rev Gastroenterol Hepatol. (2019) 16:605–16. doi: 10.1038/s41575-019-0173-3
209. Nithya A, Misra S, Panigrahi C, Dalbhagat CG, Mishra HN. Probiotic potential of fermented foods and their role in non-communicable diseases management: an understanding through recent clinical evidences. Food Chem Adv. (2023) 3:100381. doi: 10.1016/j.focha.2023.100381
210. Oniszczuk A, Oniszczuk T, Gancarz M, Szymańska J. Role of gut Microbiota, probiotics and prebiotics in the cardiovascular diseases. Molecules. (2021) 26(4):1172. doi: 10.3390/molecules26041172
211. Kok CR, Hutkins R. Yogurt and other fermented foods as sources of healthpromoting bacteria. Nutr Rev. (2018) 76(Suppl 1):4–15. doi: 10.1093/nutrit/nuy056
212. Warman DJ, Jia H, Kato H. The potential roles of probiotics, resistant starch, and resistant proteins in ameliorating inflammation during aging (inflammaging). Nutrients. (2022) 14(4):747. doi: 10.3390/nu14040747
213. Wu H, Chiou J. Potential benefits of probiotics and prebiotics for coronary heart disease and stroke. Nutrients. (2021) 13(8):2878. doi: 10.3390/nu13082878
214. Maftei N-M, Raileanu CR, Balta AA, Ambrose L, Boev M, Marin DB, et al. The potential impact of probiotics on human health: an update on their health-promoting properties. Microorganisms. (2024) 12(2):234. doi: 10.3390/microorganisms12020234
215. McFarland LV, Evans CT, Goldstein EJC. Strain-specificity and diseasespecificity of probiotic efficacy: a systematic review and meta-analysis. Front Med (Lausanne). (2018) 5:124. doi: 10.3389/fmed.2018.00124
216. McFarland LV. Efficacy of single-strain probiotics versus multi-strain mixtures: systematic review of strain and disease specificity. Dig Dis Sci. (2020) 66(3):694–704. doi: 10.1007/s10620-020-06244-z
217. Foligné B, Daniel C, Pot B. Probiotics from research to market: the possibilities, risks and challenges. Curr Opin Microbiol. (2013) 16(3):284–92. doi: 10.1016/j.mib.2013.06.008
218. Hradicka P, Adamkova P, Lenhardt L, Gancarcikova S, Iannaccone SF, Demeckova V. Addressing safety concerns of long-term probiotic use: in vivo evidence from a rat model. J Funct Foods. (2023) 104:105521. doi: 10.1016/j.jff.2023.105521
219. Pavlidou E, Fasoulas A, Mantzorou M, Giaginis C. Clinical evidence on the potential beneficial effects of probiotics and prebiotics in cardiovascular disease. Int J Mol Sci. (2022) 23(24):15898. doi: 10.3390/ijms232415898
220. Xu H-M, Huang H-L, Zhou Y-L, Zhao H-L, Xu J, Shou D-W, et al. Fecal Microbiota transplantation: a new therapeutic attempt from the gut to the brain. Gastroenterol Res Pract. (2021):2021:1–20. doi: 10.1155/2021/6699268
221. Kim J-H, Kim K, Kim W. Gut microbiota restoration through fecal microbiota transplantation: a new atopic dermatitis therapy. Exp Mol Med. (2021) 53:907–16. doi: 10.1038/s12276-021-00627-6
222. Qiao L, Yang G, Wang P, Xu C. The potential role of mitochondria in the microbiota-gut-brain axis: implications for brain health. Pharmacol Res. (2024) 209:107434. doi: 10.1016/j.phrs.2024.107434
223. Yang R, Chen Z, Cai J. Fecal microbiota transplantation: emerging applications in autoimmune diseases. J Autoimmun. (2023) 141:103038. doi: 10.1016/j.jaut.2023.103038
224. Novelle MG, Naranjo-Martínez B, López-Cánovas JL, Díaz-Ruiz A. Fecal microbiota transplantation, a tool to transfer healthy longevity. Ageing Res Rev. (2025) 103:102585. doi: 10.1016/j.arr.2024.102585
225. Oliva-Hemker M, Kahn SA, Steinbach WJ, Cohen MB, Brumbaugh D, Cole C, et al. Fecal Microbiota transplantation: information for the pediatrician. Pediatrics. (2023) 152(6):e2023062922. doi: 10.1542/peds.2023-062922
226. Wang Y, Zhang S, Borody TJ, Zhang F. Encyclopedia of fecal microbiota transplantation: a review of effectiveness in the treatment of 85 diseases. Chin Med J. (2022) 135(16):1927–39. doi: 10.1097/cm9.0000000000002339
227. Hu X-F, Zhang W-Y, Wen Q, Chen W-J, Wang Z-M, Chen J, et al. Fecal microbiota transplantation alleviates myocardial damage in myocarditis by restoring the microbiota composition. Pharmacol Res. (2019) 139:412–21. doi: 10.1016/j.phrs.2018.11.042
228. Fender AC, Dobrev D. A gut feeling: lipopolysaccharide links gut dysbiosis with inflammatory atrial cardiomyopathy, obesity, and atrial fibrillation. Can J Cardiol. (2022) 38(12):1976–8. doi: 10.1016/j.cjca.2022.09.025
229. Mańkowska-Wierzbicka D, Stelmach-Mardas M, Gabryel M, Tomczak H, Skrzypczak-Zielińska M, Zakerska-Banaszak O, et al. The effectiveness of multi-session FMT treatment in active ulcerative colitis patients: a pilot study. Biomedicines. (2020) 8(8):268. doi: 10.3390/biomedicines8080268
230. Ma Y, Liu J, Rhodes C, Nie Y, Zhang F. Ethical issues in fecal Microbiota transplantation in practice. Am J Bioeth. (2017) 17(5):34–45. doi: 10.1080/15265161.2017.1299240
Keywords: atrial cardiomyopathy, gut microbiota, gut-heart axis, dysbiosis, inflammation, remodeling, short-chain fatty acids, trimethylamine n-oxide
Citation: Sun T, Song B and Li B (2025) Gut microbiota and atrial cardiomyopathy. Front. Cardiovasc. Med. 12:1541278. doi: 10.3389/fcvm.2025.1541278
Received: 10 December 2024; Accepted: 20 January 2025;
Published: 4 February 2025.
Edited by:
Robert Koeth, Cleveland Clinic, United StatesReviewed by:
Zhengwu Sun, Dalian Municipal Central Hospital, ChinaCopyright: © 2025 Sun, Song and Li. This is an open-access article distributed under the terms of the Creative Commons Attribution License (CC BY). The use, distribution or reproduction in other forums is permitted, provided the original author(s) and the copyright owner(s) are credited and that the original publication in this journal is cited, in accordance with accepted academic practice. No use, distribution or reproduction is permitted which does not comply with these terms.
*Correspondence: Bo Li, bGlib3N1Ym1pdEAxNjMuY29t
Disclaimer: All claims expressed in this article are solely those of the authors and do not necessarily represent those of their affiliated organizations, or those of the publisher, the editors and the reviewers. Any product that may be evaluated in this article or claim that may be made by its manufacturer is not guaranteed or endorsed by the publisher.
Research integrity at Frontiers
Learn more about the work of our research integrity team to safeguard the quality of each article we publish.