- 1Medical Experimental Center, First Teaching Hospital of Tianjin University of Traditional Chinese Medicine, National Clinical Research Center for Chinese Medicine Acupuncture and Moxibustion, Tianjin, China
- 2Key Laboratory of Translational Research of TCM Prescription and Syndrome, Tianjin, China
- 3College of Traditional Chinese Medicine, Beijing University of Traditional Chinese Medicine, Beijing, China
- 4School of Medical Technology, Tianjin University of Traditional Chinese Medicine, Tianjin, China
Cardiovascular diseases (CVDs), characterized by a high incidence rate and high mortality, have become the leading cause of death globally. CVDs include coronary heart disease, stroke, hypertension, and peripheral vascular diseases. In China, the death rate of CVDs ranks the first in all major diseases. At present, the main methods to treat ischemic heart disease are drug therapy, intervention and operation. These methods only alleviate symptoms of heart failure and myocardial ischemia and improve patients' quality of life by partially restoring myocardial reperfusion. Due to the extensive irreversible necrosis of myocardial cells caused by ischemia and hypoxia, these methods cannot reverse the damage, resulting in suboptimal long-term outcomes. Although mature cardiomyocytes have been proved not to be terminally differentiated cells, they have very limited ability of regeneration and proliferation, so they can not completely replace the damaged myocardium and restore the contractile function. Although heart transplantation can replace the damaged heart, its clinical application and promotion are limited by the source of donor, expensive cost, immune rejection, and ethical problems. It has become an urgent task for clinical medicine to seek new and better treatment. The main content of this paper is to explore the application of stem cells and gene technology in the treatment of myocardial infarction (MI).
1 Introduction
Myocardial infarction (MI), commonly known as a heart attack, remains a leading cause of morbidity and mortality worldwide. Despite advances in emergency medical interventions such as reperfusion therapy, many patients still suffer from significant long-term cardiac dysfunction due to irreversible myocardial damage. The limited regenerative capacity of the adult heart presents a major challenge in the treatment and recovery process (1). Therefore, exploring innovative therapeutic strategies to promote myocardial repair and improve cardiac function has become a critical area of research. In recent years, two promising approaches have emerged as potential game-changers in the treatment of myocardial infarction: stem cell therapy and gene therapy (2, 3). Stem cell therapy involves the use of various types of stem cells, such as mesenchymal stem cells (MSCs) and induced pluripotent stem cells (iPSCs), to replace damaged myocardial cells and stimulate the regeneration of cardiac tissue (4, 5). These cells can differentiate into cardiomyocytes, endothelial cells, and smooth muscle cells, thereby contributing to the structural and functional restoration of the heart. Additionally, stem cells can secrete paracrine factors that promote angiogenesis, reduce inflammation, and inhibit apoptosis, creating a more favorable environment for cardiac repair (6). On the other hand, gene therapy focuses on the delivery of specific genes to modify the expression of target proteins within cardiac cells. This approach aims to enhance the intrinsic regenerative capacity of the heart by upregulating genes involved in cardiomyocyte proliferation, survival, and differentiation. Overexpression of certain growth factors or transcription factors can stimulate the endogenous cardiac progenitor cells to proliferate and differentiate into functional cardiomyocytes (7, 8). Gene therapy can also target pathways involved in fibrosis and remodeling, thereby preventing adverse structural changes in the heart following an infarction (9, 10). However, several challenges remain to be addressed, including optimizing cell delivery methods, ensuring long-term gene expression, and minimizing potential side effects (11–14). This review aims to provide an in-depth analysis of the current progress, mechanisms of action, and future prospects of these two therapeutic approaches, highlighting their potential to transform the clinical management of myocardial infarction and improve patient outcomes.
2 Cell therapy of myocardial infarction
Cell therapy is becoming a new strategy to avoid the adverse effects of heart disease. Many experimental and clinical studies have shown that the transplantation of cells into damaged myocardium has obtained gratifying results. Of note, these data show that the transplanted stem cells can differentiate into the main heart cell types and improve heart function. However, some clinical trials show contradictory results.
The occurrence and development of heart disease include various pathological changes of heart structure and function. MI and coronary artery disease are the most common heart diseases in Western countries. MI can lead to myocardial remodeling, including left ventricular dilation, cardiac hypertrophy, formation of fibrotic scar tissue, and reduction of myocardial cells (15). Interventional therapies can improve the patients' quality of life, but these treatments cannot prevent further heart failure (16). In animal models, cell-based therapies can not only replace dead cardiomyocytes but also inhibit cardiac remodeling. In particular, neonatal, fetal, and adult cardiomyocytes (17), skeletal myoblasts (18), human cord blood cells (19), endothelial progenitor cells (20), embryonic stem cells (21), bone marrow stem cells (22), mesenchymal stem cells (23) and induced pluripotent stem cells are used for myocardial regeneration after MI (Figure 1).
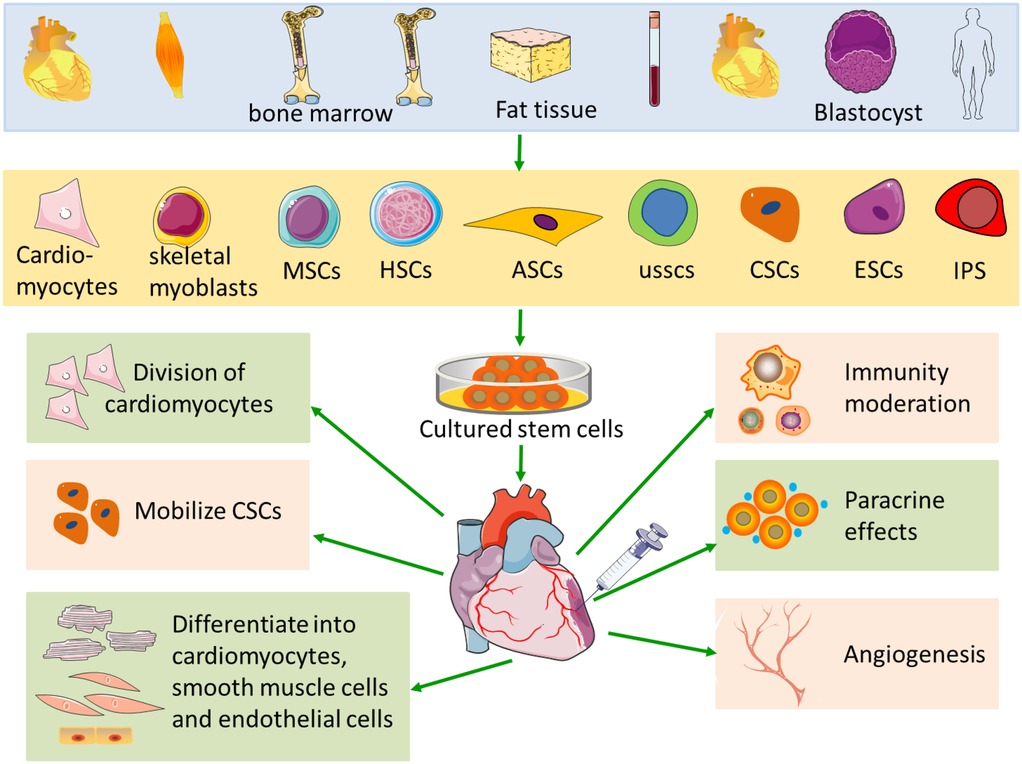
Figure 1. Schematic of stem cell therapy in MI. MI necessitates the use of various cell types for myocardial regeneration. Each cell type has its own unique advantages and disadvantages when applied in the context of MI. MSCs, mesenchymal stem cells; HSCs, hematopoietic stem cells; ASCs, adults stem cells; USSCs, unrestricted somatic stem cell; CSCs, cardiac stem cells; ESCs, embryonic stem cells; iPS, inducible pluripotent stem cells.
Many literatures have proved that cell transplantation is a good strategy to repair damaged myocardium. In this section, we introduce the advantages and disadvantages of different cell types used in MI (Table 1), some laboratory and clinical cell transplantation attempts, and further explore the limitations of various types of cells used for regeneration of damaged myocardium.
2.1 Cardiomyocytes
During the development and formation of the heart in the embryonic stage, cardiomyocytes rapidly proliferate and differentiate into form mature cardiomyocytes, which constitute the structure of the heart (24). In most mammals, during the last round of replication, cardiac myocytes undergo DNA synthesis and mitosis without cytoplasmic division, while cells in other organs undergo cytoplasmic division at the same time to form two different daughter cells (25). In adult cardiomyocytes, there are often mononuclear, binucleate, or even trinuclear cardiomyocytes with undifferentiated cytoplasm. Previous research has long suggested that in response to adult heart injury, cardiomyocytes primarily rely on hypertrophy rather than cell division to meet the increased demand for blood supply. This is characterized by an enlargement of cardiomyocyte volume. However, recent studies have challenged this traditional view by demonstrating that cardiomyocytes can indeed undergo division, not only in normal myocardium but also in infarcted regions. This new evidence suggests a more complex and dynamic role of cardiomyocytes in the context of cardiac injury and repair (26, 27). Neonatal human hearts might have the capacity of repairing myocardial injury and recovering cardiac function after MI (28). However, the division of cardiomyocytes after myocardial injury is not enough to repair the damaged myocardium. So far, numerous studies have shown that in many heart diseases, including acute MI, ischemic heart failure, chronic myocardial ischemia and hereditary heart disease, transplantation of neonatal, fetal, or adult cardiomyocytes is effective, and these cells can repair the damaged myocardium after transplantation (29). These results show that the transplanted cells can successfully differentiate into the myocardium and improve contractile function. Fetal, neonatal, and adult cardiomyocyte transplantation is likely to successfully repair the damaged heart. Compared with adult cardiomyocytes, fetal and neonatal cardiomyocytes have a better effect on repairing damaged myocardium. This is because the genetic material of neonatal and fetal cardiomyocytes is not mature yet, and they have stronger plasticity compared with adult cardiomyocytes. However, these kinds of cardiomyocytes cannot be used in clinical practice, because the acquisition of these cells requires heart biopsy.
2.2 Skeletal myoblasts
Skeletal muscle is one of the three main muscle types in the human body. Skeletal muscle fibers are the basic functional elements of these cells; they produce the mechanical forces necessary for muscle contraction, similar to cardiomyocytes. However, unlike cardiomyocytes, skeletal myoblasts still maintain their regeneration ability after injury. Given this ability, skeletal myoblasts seem to be a suitable new source of cardiac cell therapy. Besides, autogenous skeletal myoblasts were used to solve the problem of the source of donor cells. The source of donor cells has been limiting the application of adult cardiomyocytes and neonatal cardiomyocytes. To sum up, skeletal myoblasts have the advantage of resistance to ischemia, autologous transplantation, and high proliferative potential (30).
Previous studies have shown that transplantation of autologous skeletal myoblasts into the damaged heart can improve the systolic and diastolic properties of the damaged heart. Some clinical studies found skeletal myoblasts transplantation to be a feasible and sufficient treatment for MI, playing an important role in improving cardiac function by suppressing fibrosis, reducing left ventricular wall stress, and improving regional wall motion (31, 32). However, in another study of autogenous transplantation of skeletal myoblasts, the phase I clinical trial failed to prove that transplantation of skeletal myoblasts can improve the condition of patients with ischemic cardiomyopathy. This experiment found that compared with the placebo group, the frequency of arrhythmias in the cell transplantation group increased (33). These side effects observed in patients limit the clinical application of skeletal myoblasts.
2.3 Adult stem cells
2.3.1 Mesenchymal stem cells (MSCs)
Adult stem cells exist in all organs of the body. The best source of adult stem cells is bone marrow (BM). Bone marrow stem cell groups commonly used in experiments and clinical include hematopoietic stem cells (HSCs), c-kit+ cells, SCA+ cells, MSCs, and bone marrow-derived mononuclear cells (BMMNC) (34). MSCs derived from bone marrow are easy to differentiate and proliferate in vitro. In vitro and vivo experiments show that they can differentiate into osteoblasts, chondrocytes, monocytes, and adipocytes. The repair mechanisms of MSCs in MI might include autocrine effects, paracrine effects, and endocrine-like effects. The autocrine effects of MSCs could promote survival, proliferation, and possible differentiation. The paracrine pathway could secrete trophic factors acting on adjacent cells, and promote angiogenesis and inhibit fibrosis. Endocrine-like pathways secreted trophic factors circulating in vascular or lymphatic systems to induce the repair effect.
In fact, many in vitro studies have shown that MSCs have the potential to differentiate into cardiomyocytes in the presence of retinoic acid, DMSO, and 5-aza, or when co-cultured with cardiomyocytes. Animal experiments showed that MSCs transplantation after MI inhibited ventricular remodeling and improved cardiac function. The remarkable effect of MSCs is mainly due to its paracrine effect on host cardiomyocytes, rather than cell fusion and differentiation. Moreover, MSCs overexpressing survival-promoting factor Akt can protect myocardium 72 h after transplantation. Recent studies showed that the secretion of exosomes played an important role in the effects of cardioprotection. Exosomes transferred bioactive molecules including miRNAs and proteins between cells, which helped improve angiogenesis, proliferation, and suppress apoptosis (35). An analysis of mesenchymal stem cell proteomes successfully identified the MSC-specific nascent proteins in the infarcted hearts, which might help to reflect the reparative effects of MSCs (36).
2.3.2 Hematopoietic stem cells (HSCs)
Another group of bone marrow-derived stem cell is HSCs, which have the potential to differentiate into multi-lineal blood cells including lymphocytes and stromal cells. There was a study proved that HSCs possessed the unique ability to differentiate into cardiomyocytes (37). However, more evidence showed HSCs could only differentiate into hematopoietic lineage cells but not into cardiomyocytes (38, 39). The specific proteins of HSCs are CD34+ and CD133+ in human, Lin+, and c-kit+ in mice. At different stages of differentiation, HSCs no longer express these cell surface markers (40). HSCs are used for myocardial repairation in different animal models. However, these data have produced contradictory results. In one study, mice were ligated with the left anterior descending branch and then transplanted with HSC derived bone marrow cells with both Lin and c-kit positive. After 9 days of cell transplantation, endothelial cells (ECs) and smooth muscle cells (SMCs) from donors could be detected. Moreover, compared with the control group, the ventricular function of the treatment group was significantly improved. However, this finding has been questioned by subsequent articles, which show that after hematopoietic stem cell transplantation, no myocardial regeneration or improvement of cardiac function has been found (41). In a clinical study of chronic MI patients, intramyocardial injection of specific bone marrow HSCs in the injury area enhanced myocardium regeneration and improved cardiac contractility, and without adverse side effects events occurred (42). In general, HSCs are not considered as an ideal stem cell group for myocardial regeneration.
2.3.3 Adipose-derived stem cells (ASCs)
It has been proved that stem cells in adipose tissue are pluripotent, immature, and self-renewing. ASCs are not subject to ethical challenges and can be harvested in an effective manner such as surgical scraps from the surgical specimens. ASCs can be isolated from human and animal adipose tissues including abdomen, thigh, and upper limb, and play roles in tissue regeneration and repair by secreting growth factors, cytokines, neurotrophic factors, and angiogenic factors (43). Many studies have shown the advantage of injecting ASCs into damaged myocardium. For example, in a study, ASCs injected into the myocardium can differentiate into cardiomyocytes, and preconditioned ASCs attenuate cardiac fibrosis after MI (44). In LPS-induced cardiomyocyte damage, ASCs showed a cardioprotective effect (45). Other studies have shown that transplanted ASCs play a role through the secretion of growth factors, which can mobilize the stem cells of the heart itself to reach the damaged site and repair the damaged myocardium (46).
2.3.4 Cord blood stem cells (CBSCs)
CBSCs are very promising for tissue repair and regeneration because cord blood (CB) contains a variety of stem cells, and CB is easy to collect and preserve. In addition to HSCs and MSCs, CBSCs contain many adult stem cells that are not limited by application. Although the number of these cells is small, they can be easily separated and expanded without losing the pluripotency of cells. In vivo and vitro, CBSCs can differentiate into osteoblasts, chondrocytes, adipocytes, and nerve cells (47). The advantages of this cell as a cardiac therapeutic cell have been proved in the porcine model of MI. This experiment shows that the transplanted CBSCs has the ability of myocardial regeneration (48). In the model of MI of rats, CBSCs can repair damaged myocardium and induce angiogenesis, to improve heart function (19, 49, 50). Although these cells have the potential of myocardial regeneration, they have not been used in the clinical treatment of heart disease. Moreover, to avoid immune rejection, the use of CBSCs in clinical trials requires patients store cells at birth for later transplantation.
2.3.5 Cardiac stem cells (CSCs)
Studies have shown that the heart itself contains some natural multifunctional cardiac stem cells (CSCs). CSCs have been successfully isolated from mouse and human heart biopsy tissues and cultured in vitro. After transplanted into the infarcted heart, these cells can differentiate into cardiomyocytes, vascular smooth muscle cells (VSMCs), and ECs (51). However, the renewal rate of CSCs is low and decreases from 1% at age 25 years to 0.45% at age 75 years (27). A clinical trial involving patients with MI demonstrated the safety, feasibility, and potential therapeutic effect of adult cardiac stem cell transplantation (52). In animal models, the results have been published to prove the effect of CSCs on cardiac repair and regeneration (53). Nevertheless, other studies showed that CSCs didn't harbor the ability for cardiomyocyte regeneration or improve cardiac function (54, 55). As CSCs proliferate at a low frequency, methods that motivate cardiomyocyte proliferation may become a crucial research field in the future.
2.4 Embryonic stem cells (ESCs)
ESCs are pluripotent and undifferentiated cells, which can maintain the ability of self-renewal for a long time in vitro. In addition, under specific physiological or experimental conditions, ESCs can differentiate into specific cells, including nerve cells, ECs, VSMCs, and cardiomyocytes (56). The primitive three-dimensional cell structure formed by ESCs in vitro is called embryoid body (EBS). Embryoids contain cells from all three germ layers, such as cardiomyocytes, neural cells, and hematopoietic cells (57). Current studies show that ESCs can be induced to produce paracrine and autocrine effects, release cytokines, thus affecting the endogenous cell microenvironment and improving heart function. The exact factors and mechanisms are not clear, and some promising intermediates are being further studied. For example, tissue inhibitor of metalloproteinase1 (TIMP1), a growth factor of fibrosis and anti-apoptosis, has been shown to promote myocardial repair. Although the research of TIMP-1 has achieved gratifying results (58), its mechanism is still under study.
There are two ways for ESCs to help cardiac regeneration after heart injury: one is to form the main types of cardiac cells through differentiation, the other is to release anti-apoptosis and anti-fibrosis factors. However, the application of ESCs in heart cell therapy is restricted by ethics, because these stem cells were isolated from embryos. In addition, the histocompatibility and teratoma formation should be considered in the application of embryonic stem cells. The use of ESCs requires the use of immunosuppressive drugs to overcome the differences in histocompatibility and inhibit cell rejection after transplantation. Teratomas are stem cell-derived tumors, which are a mixture of cells from three germ layers. They are differentiated cell clusters formed by undifferentiated or out of control differentiation of stem cells. There is a potential risk of forming teratomas after ESCs transplantation (59, 60). The good news is that current studies have shown teratoma formation in vivo can be avoided when the number of ESCs transplanted is limited to 5 × 104 or less (61).
In a pig model of MI, human ESCs-derived cardiomyocytes could regenerate the infarcted cardiomyocytes, but give rise to ventricular tachyarrhythmias at the same time (62). To solve the problem of the low rate of stem cell survival that injected into the myocardium, a study of co-transplanting biomaterial with ESC-derived cardiomyocytes into the infarcted myocardium of rat MI model was performed (63). And the results showed that the combination therapy improved cardiac function and lowered the rates of induced arrhythmias.
2.5 Inducible pluripotent stem cells (iPSCs)
Yamanaka and his team (64) induced cell groups with similar characteristics to ESCs, induced pluripotent stem cells (iPSCs). Through retroviral transduction, they made adult mouse fibroblasts express four transcription factors: (1) krueppel like factor 4 (KLF4), (2) SRY related HMG box transcription factor (Sox2), (3) octamer binding transcription factor 3/4 (OCT3/4), and (4) myeloma gene (c-myc). These embryonic-like cells show the characteristics of embryonic stem cells, such as the expression of four cell surface markers, the formation of embryonic stem cells like clones, totipotency, and the ability to self-renew. Moreover, iPSCs can differentiate into cells of the three germ cells, including hematopoietic, neural stem cells and cardiomyocytes (65). And compared to ESCs, autologous iPSCs transplantation has the advantage of avoiding the ethical issues and immunological rejection.
iPSCs derived from fibroblasts injected into the heart confirmed the potential of these cells for cardiac cell therapy, which improved the contractile function of the ischemic heart. These iPSCs spontaneously integrate into the host myocardium and differentiate into major cardiomyocyte types. Besides, iPSCs cells have been obtained by expressing OCT3/4, Sox2, KLF4, and c-myc in H9C2 cells (66). Some studies have reported the benefit of iPSCs-derived cardiomyocytes in transplantation for heart regeneration in mouse, rat, or pig MI models (66–68). When transplanted into the infarcted heart, this kind of cells can reduce the loss of myocardial cells, reduce the formation of fibrosis scars, and significantly improve the heart function. In a rat MI model, co-transplanted adipose tissue derived- micro vessels with human induced pluripotent stem cells-derived cardiomyocytes could improve the survival of iPSCs-derived cardiomyocytes and enhance the functional recovery of the heart. Suggesting a method help to increase the viability of stem cells, promote cardiomyocyte maturation and decrease arrhythmogenicity (69). In addition, these cells can provide specific iPSCs for patients, which are very useful in the establishment of disease models and drug screening for various diseases (70), so they bring great hope for regenerative medicine.
However, before any clinical application, the safety of iPSCs must be fully considered. C-myc is a well-known protooncogene, which can activate the expression of tumorforming genes in host tissues (71). The current research can get iPSCs without using the c-myc gene, but the reprogramming efficiency of this iPSCs is quite low (72). In addition, to avoid the risk of insertion mutagenesis, non-viral iPSCs are being studied (73–75). In these studies, iPSCs were induced from adult cells by small molecules or recombinant proteins, such as polyarginine. Other studies have also involved the use of non-viral vectors or non-integrated attachment vectors that are not integrated into the genome. These methods can induce pluripotency of cells without using genetic tools.
3 Gene therapy for MI
In 1989, transgenic technology was first introduced into the cardiovascular field. Although many basic and clinical studies have been carried out, the clinical application of transgenic technology needs further verification. This is because, in most clinical studies, the inherent problem of immunogenicity of recombinant virus vectors is difficult to avoid. Gene therapy provides a very attractive solution for the treatment of ischemic heart disease. It can continuously provide therapeutic protein for the focus and may reverse the pathophysiology of AMI. The use of new gene structures for modification enables gene expression to be turned on or off according to the intracellular environment, minimizing waste caused by unrestricted protein synthesis or inhibition. Based on stem cell therapy, combined with gene therapy, can promote myocardial regeneration, and has a higher success rate. Therefore, gene therapy may delay or even avoid the need for heart transplantation by reversing the pathology. This section will discuss the research progress of gene therapy for MI.
3.1 Concept of gene therapy
Gene therapy is to compensate or correct diseases caused by gene defects and abnormalities by introducing foreign normal genes into target cells, so that the products of foreign gene expression can treat the disease.
According to the function of inserting genes, gene therapy can be divided into three categories: (1) Substituting or repairing for missing or defective genes, such as, AAV-CRISPR/Cas9-mediated gene correction can partially mediate LDLR expression and effectively ameliorate AS phenotypes in mutants, providing a potential therapeutic approach for the treatment of patients with familial hypercholesterolemia (76); (2) Enhancing endogenous physiological function by promoting gene expression, such as improving atherosclerotic vascular endothelial cells by overexpression of eNOS gene; (3) Destroy the expression or function of harmful endogenous genes, such as using antisense oligonucleotides (ODNs) to destroy E2F transcription factors to inhibit AS.
3.1.1 Non-viral vector
The anionic liposome is a kind of common non-viral vector, which transfers genes by entering host cells through encapsulation. Liposome is almost non-toxic and non-immunogenic, so it is a relatively good carrier to transfer DNA into cells. However, the DNA carried by the liposome will be transported to the lysosome after being swallowed into cells, which will lead to low transfection efficiency. In response to this limitation, scientists have developed a liposome vector for fusion genes (77).
3.1.2 Virus vector
Viral vector is a kind of replication-defective viral plasmid, which only retains the ability to enter the target cell and transfer its genetic material into the chromosome of the target cell. According to their sources, virus vectors can be divided into the following categories:
3.1.2.1 Retroviral vector
Retrovirus is a kind of RNA virus, which can integrate the transferred gene into the host chromosome. However, gag, pol, and env genes are integrated into packaging cell lines such as 293 T cells to synthesize capsid proteins and polymerases necessary for virus replication. Retroviral vectors have many advantages, including a long time of transgene expression, large capacity of DNA coating, high virus titer, and so on. However, its ability to infect non-dividing cells is weak, which limits its application to a certain extent.
3.1.2.2 Adenovirus vector
The gene of adenovirus vector is a double-stranded DNA molecule with a length of 36 KB. Adenovirus vector is the main vector for gene therapy of cardiovascular diseases in vivo. Adenovirus vector has many advantages: (1) It is easy to prepare; (2) It has a high virus titer; (3) It has a strong gene transfer effect; (4) It has a large capacity of covering DNA; (5) It is superior to retrovirus in that it can infect both static and slow dividing cells. However, the adenovirus vector itself has some shortcomings. The transgenic expression time of adenovirus as a gene vector is short, and can trigger the immune response of the host.
3.1.2.3 Adeno-associated virus vector
Adeno-associated virus is a small DNA virus, it must rely on adenovirus or other viruses to replicate. Adeno-associated virus can carry 3–4 kb foreign gene. Similar to adenoviruses, adeno-associated viruses can infect both stationary and nondividing cells.
Gene therapy can synthesize or inhibit a specific protein, leading to changes in the structure or function of the target tissue. To carry out gene therapy, the first step is to design a stable sequence of genes of interest with promoter/enhancer. Then, the vector containing the sequence was constructed. Then, the target gene can be expressed by transferring the gene into the target cell.
The efficiency of naked plasmid DNA transfection is very low, but the target cells can be transduced by adeno-associated virus, retrovirus, and non-viral vector system. The special medical catheter can inject the carrier with the target gene into the coronary artery or the heart muscle. Besides, pericardial administration and intramyocardial injection through minimally invasive surgery have been used.
3.2 Gene therapy for promoting angiogenesis
3.2.1 Gene therapy of vascular endothelial growth factor (VEGF)
VEGF may be the most concerned growth factor, which can promote the angiogenesis of the ischemic heart. VEGF binding to specific receptors on the surface of endothelial cells plays an essential role in the process of angiogenesis. The mammalian genome encodes five subtypes of the VEGF family, which are VEGF-A, VEGF-B, VEGF-C, VEGF-D, and placental growth factor (78). VEGF-A and VEGF-B conduct signals through VEFG receptor 1 and VEFG receptor 2, regulating vascular physiological function and development (79).
VEGF-A plays a key role in cardiac angiogenesis. The transcripts encoding VEGF-121 and VEGF-165 can be detected in most tissues and cells expressing the VEGF gene. Gene therapy for VEGF-165 is considered highly effective in promoting angiogenesis (80). VEGF-165 gene can induce obvious angiogenesis and improve the short axis contraction rate after MI in rats or rabbits by non-viral therapy (81). In the pig or dog models of MI, VEGF-165 can decrease the infarct area, improve the left ventricular contractility (82), increase cardiac wall thickness and strength (83), and improve myocardial survival rate (84), thus significantly improving the overall function of the heart.
In a clinical study, VEGF gene therapy didn't improve myocardial abnormalities but show anti-ischemic effects (85). In another study, there was a significant improvement in myocardial blood flow and angina pectoris symptoms (86). VEGF gene therapy could also mobilize the immune system and endothelial progenitor cell (87) and seemed the long-term safety (11).
Gene therapy to promote angiogenesis is not without defects, and the unregulated expression limits the effectiveness and safety of VEGF gene therapy. To solve this problem, the gene structure of expression switch regulated by extracellular environment is under study.
3.2.2 Gene therapy of hepatocyte growth factor (HGF)
HGF is secreted by mesenchymal cells, which can play various roles in epithelial target cells. HGF binds to the tyrosine kinase receptor on the surface of endothelial cells, which affects the migration, proliferation, protease expression, infiltration, and angiogenesis of endothelial cells.
In animal models of heart diseases, HGF gene therapy showed the abilities of decreasing the inflammatory levels, improving cardiac function (88), increasing arteriole density, and angiogenesis (89). HGF could also inhibit apoptosis (90) and improve the contractile ability of the heart (91). Combined application of fibroblast growth factor-2 and HGF showed a more potent and durable effect of angiogenesis, and effectively reduced cardiac remodeling and improved left ventricular functions (92). HGF gene therapy combined with ultrasound-mediated microbubble breaking technology can improve the rat heart function and the efficiency of gene transfection in MI model (93). HGF-expressing MSCs improved angiogenesis, cell viability, and cardiac function (94, 95), indicating a strategy that could be used to enhance the efficacy of cell therapy. In clinical trials of patients showed that adenovirus carrying HGF is safe and potentially effective in enhancing echocardiographic indexes (96, 97), but the sample size was too small. Therefore, the results need to be confirmed in studies with a larger sample size, and a great deal of work is necessary for the clinical practice of HGF gene therapy in MI.
3.2.3 Gene therapy of fibroblast growth factor (FGF)
Fibroblast growth factors (FGFs) bind to tyrosine kinase receptors, regulate cell mitosis and cell survival. FGFs promote tissue growth and affect cell proliferation and division (98). FGF family members are classified according to their affinity ligands and tissue distribution. FGF-1 and FGF-2 promote endothelial cell proliferation and tube formation. FGF-4 and FGF-5 are secretory FGFs, while FGF-1 and FGF-2 are mainly intracellular, so FGF-4 and FGF-5 have greater advantages, they have paracrine and endocrine functions.
In a mice model of MI/R, FGF-1 played roles in cardio-protection through protecting cardiac functional recovery and increasing cell survival of ischemia heart (99). In MI mouse models, FGF induced the accumulation of HIF-1α, promotion of angiogenesis and inhibition of apoptotic effects in cardiac endothelial cells, and improved cardiac remodeling (100). FGF could also reduce oxidative stress (101), attenuate cardiac fibrosis (102), and FGF21 showed antiarrhythmic property (103). In the pig model of MI, FGF-4 gene therapy can enhance perfusion and improve the dysfunction caused by MI (104). FGF-5 gene therapy can not only improve blood supply (105) but also improve local myocardial dysfunction by stimulating mitosis of myocardial cells (106).
3.3 Gene therapy to reduce muscle reperfusion injury
3.3.1 Gene therapy and oxidative stress
Among many cardiovascular diseases, including MI, myocardial ischemia/reperfusion injury, atherosclerosis, endothelial dysfunction, restenosis, hypertension, cardiomyopathy, and heart failure, oxidative damage is the most serious injury. Reactive oxygen species (ROS) produced in R/I injury not only cause lipid peroxidation and protein oxidation but also affect calcium channels induced by various Calmodulins, such as ryanodine receptors (RyR), sarcoplasmic reticulum (SR) Ca2+ ATPase (SERCA), and 1,4,5-inositol triphosphate (IP3), thus increasing calcium entry into cardiomyocytes and causing cell damage (107). The expression of many antioxidant enzymes, including superoxide dismutase (SOD), glutathione peroxidase (GPX), catalase, or heme oxygenase-1 (HO-1), is induced by inflammation or other stimuli. The production of these antioxidant enzymes is a protective mechanism to eliminate ROS. SOD has three subtypes to catalyze the disproportionation of superoxide anion, thus reducing the oxidative damage of cells (108). Gene therapy with extracellular isoform of SOD attenuated myocardial injury through increasing antioxidant enzymes in rabbit and mouse model of MI (109, 110).
3.3.2 Gene therapy of endothelial nitric oxide synthetase (eNOS)
In the CVDs, nitric oxide (NO) is a signal molecule with multiple protective functions (111). In mammals, it is synthesized by L-arginine under the action of endothelial nitric oxide synthetase, and NO mainly plays a protective role on the myocardium. Adenovirus-mediated human eNOS gene therapy after MI operation could reduce MI area, apoptosis, JNK phosphorylation level, caspase-3 activity, and improve cardiac remodeling (112). ENOS can reduce the level of fibrosis, improve endothelial progenitor cells and vascular function after MI (113). Gene engineering with eNOs of EPCs from patients with coronary artery disease showed improvement in EPCs migration, differentiation, and angiogenesis in vitro, possibly through a paracrine effect (114). A combinational approach of hydrogel loaded with ADSCs and plasmid DNA-eNOs was used to treat MI rats, and the results showed that this approach could improve the heart functions, including infraction size, fibrosis area, and vessel density (115). Adenoviral transfected of human eNOS gene into MSCs remarkably enhanced capillary density and hemodynamic parameters (116).
3.4 Gene therapy for inhibition of cardiomyocyte apoptosis
3.4.1 Heat shock proteins (HSPs)
HSPs act as molecular chaperones, responsible for protein folding, intracellular protein transport, denaturation, or modification of other proteins under heat or other stimuli. MIMI Overexpression of HSP20 improved cardiac contractile function, increased SR Ca-Cycling in cardiomyocytes, and played a cardioprotective role in heart disease (117). In a MI model, overexpressed HSP20-mediated cardiomyocyte exosomes increased cell survival by activating Akt signaling pathway and decreasing tumor necrosis factor-α (TNF-α) and interleukin-1β (IL-1β) (118). HSP27 significantly protected the heart from apoptosis or oxidative stress against MIRI (119). Overexpression of HSP60 protected myocytes from apoptosis by decreasing cytochrome c release, caspase-3 activation, and enhancing ATP recovery (120). HSP70 gene pre-therapy can reduce the area of MI in rabbits (121). Therefore, gene therapy with HSP can reduce the apoptosis after myocardial ischemia, and HSPs can protect the heart after MI.
3.4.2 Mitogen-activated protein kinase (MAPK)
The amplification of mitogen-activated protein kinase (MAPK) is composed of extracellular signal-regulated protein kinase (ERK), p38 kinase, and c-Jun N-terminal protein kinase (JNK). MAPK is a key regulator of cell survival and death. Poor ventricular remodeling after infarction is associated with decreased p38 signal. p38-regulated/activated protein kinase deficiency decreased angiogenesis and enhanced myocardial fibrosis and cardiomyocytes apoptosis (122). Co-transfection of wild-type (WT) p38 kinase and activated MKK3b (the protein is the activator upstream of p38 kinase) reduced MI area and apoptosis, increased capillary density, decreased fibrosis, and improved ejection fraction in rat MI/R model (123). But p38 inhibitors were also reported to improve cardiac performance (124). These results suggested the bidirectional effects of p38 at different time points of the disease process.
3.4.3 Insulin-like growth factor-1 (IGF-1)
IGF-1 is mainly secreted by the liver. It is an endocrine hormone, but it is produced in the form of paracrine/endocrine in the target tissue. IGF-1 is produced by the stimulation of growth hormone, and its secretion is inhibited when it is malnutrition and insensitive to growth hormone. IGF-1 can inhibit programmed cell death, activate AKT signaling pathway, and promote cell growth and proliferation. IGF-1 overexpressed mesenchymal stem cells can promote the migration, growth, and proliferation of bone marrow-derived mesenchymal stem cells by activating the AKT/SFRP2 signaling pathway, and inhibit cell apoptosis (125). In a porcine AMI model, microencapsulated IGF-1 therapy reduced the infarct area and improved cardiac function (126).
3.5 Other gene therapy to inhibit cardiomyocyte apoptosis
3.5.1 Tumor necrosis factor (TNF)
TNF is produced by activated macrophages. It can activate apoptosis, inflammation and inhibit tumor formation through the TNF receptor. Soluble TNF- α receptor 1 (sTNFR1) is an antagonist of TNF. In the MI model of mice, sTNFR1 gene therapy can reduce the infarct area and improve the heart function (127). However, in a human study, TNF-α antagonism decreases systemic inflammation but enhances platelet activation, and is not recommended for therapeutic strategy in patients of AMI (128).
3.5.2 Leukemia inhibitory factor (LIF)
LIF is an IL-6-related cytokine, which can regulate cell differentiation, growth, proliferation, tissue regeneration, inflammation, and immune response (129). In the ischemic border area, the transfer and overexpression of the LIF gene can reduce the degree of fibrosis, increase the left ventricular wall thickness, reduce the apoptosis in the ischemic area, and induce neovascularization (130). In addition, LIF partly mobilizes stem cell-derived cardiomyocyte regeneration through the activation of cardiac stem or precursor cells (131).
3.5.3 Sonic hedgehog (Shh)
Shh is a protein-related to the Hedgehog signaling pathway in mammals, which plays a key role in organ formation and development. Injection of naked DNA encoding the human Shh gene into ischemic myocardium showed that it can protect ventricular function by enhancing angiogenesis, inhibiting apoptosis, and inhibiting fibrosis (132). In pig models of myocardial ischemia reperfusion, the activation of Shh improved cardiac function, alleviated reperfusion arrhythmias, and decreased infarct area (133).
3.5.4 Kallikrein
Kallikrein kinin system is a system that can control blood pressure, cause pain and inflammation. It is mediated by vasorelaxant kinin and lysine bradykinin, which are produced by the hydrolysis of their precursor kininogen by kallikrein. In the MI model of rats, gene therapy of kallikrein can increase capillary density, reduce apoptosis, reduce endothelial dysfunction and improve cardiac function after MI (134), and the mechanism is possibly concerned with the kinin B2 receptor-Akt-GSK-3beta and VEGF signaling pathways (135).
3.5.5 Cd151
The cell surface protein encoded by the CD151 gene plays an important role in cell development, growth, activation, and migration. In pig and rabbit models of MI, CD151 gene therapy can promote angiogenesis and improve heart function (136–138).
3.5.6 Akt protein kinase
Akt protein kinase family members are related to the signaling pathways of cell survival and apoptosis inhibition. AKT1 is also associated with angiogenesis. Adenovirus mediated Akt gene therapy can reduce the infarct area, enhance myocardial contractility, and intracellular calcium content of heart (139). Therefore, Akt gene therapy has the potential to treat heart failure.
3.5.7 Bcl-2 protein
Bcl-2 plays an antiapoptotic role in mitochondria by neutralizing Bax and inhibiting the release of cytochrome c. Overexpression of Bcl-2 can promote cell survival and inhibit apoptosis. In the ischemia-reperfusion model of mice, compared with the wild type mice, the transgenic mice with super surface human Bcl-2 gene reduced the MI area and improved the ejection fraction (140). BCL2 gene therapy can reduce apoptosis, the extent of ventricular dilation, and the reduction of wall thickness in the rabbit model of ischemia-reperfusion (141).
3.5.8 Cardiac Troponin-1 (CT-1)
CT-1, a member of the interleukin-6 family, can protect the heart from ischemia-reperfusion injury and play a key role in cardiac repair and cardiac hypertrophy. In the mouse model of MI, CT-1 gene therapy inhibited apoptosis, reduced the area of MI, reduced the activity of caspase, and improved the ventricular pressure index (142). And CT-1 could increase the engraftment of mesenchymal stromal cells to improve the effect of stem cell therapy (143).
3.5.9 Sphingosine kinase (SphK)
SphK is a lipid kinase, which is related to the metabolism of sphingosine. SphK1 catalyzes the formation of sphingosine-1-phosphate (S1P), which activates eNOS through its receptor S1P1, induces endothelial cell chemotaxis, and maintains vascular integrity (144). In the rat model of MI, SphK1 gene therapy can maintain the systolic and diastolic function of the heart and improve the peak systolic flow rate (145).
Therefore, gene therapy with overexpression of TNF, LIF, Shh protein, kallikrein, CD151, Akt, Bcl-2, inhibitor of apoptosis, CT-1 and SphK can improve various pathological parameters related to ischemic heart disease.
4 Discussion
4.1 Clinical trial of stem cell transplantation
Many clinical trials have used cell transplants to treat heart disease. Some clinical trials successfully evaluated the feasibility of using skeletal myoblasts to treat patients with ischemic heart failure (31, 32). In addition, the increased frequency of arrhythmia in the experimental group makes the safety of the application of skeletal myoblasts to be verified. ESCs-derived cardiac progenitors embedded into a fibrin scaffold were transplanted into the infarct heart of the patient (146). Some clinical trials use bone marrow derived stem cell groups to test the safety and feasibility of their therapeutic applications. Some clinical trials do not choose to use BMCs, but a specific type of cells in BMCs, such as CD34+ stem cells (147). The effect of intramyocardial injection of bone marrow-derived cells on cardiac function in patients with acute myocardial infarction (AMI) was studied (148, 149). The data showed that during the 6-month follow-up period, the systolic function of patients injected with BMCs was significantly improved. In another clinical trial, 200 patients with AMI were injected with bone marrow-derived mononuclear cells, bone marrow-derived CD34+CXCR4+ cells, and placebo. This study did not find a significant improvement in the left ventricular ejection fraction (LVEF) in the treatment group. However, the authors suggest that cyto-therapy may be more appropriate for patients with more severe conditions (150). There was a study demonstrated the safety and feasibility of intracardiac injection of circulating progenitor cells (CPCs) or bone marrow-derived progenitor cells (BMC) (151). In an experiment, 120 patients with AMI with PCI were treated by bone marrow mononuclear cells (BMCs), the results showed the BMCs had no significant effect on improvement of left ventricular (LV) function (152). In another trial, there were no definitive answers acquired regarding the effect of bone marrow-derived mononuclear cell because of the unexpected low recruitment and event rates (153). The use of allogeneic cardiac stem cells- cardiosphere-derived cells (CDCs) were also utilized to treat patients with heart disease and showed the safety and feasibility of these cells (52).
In general, the conflicting results observed in various adult stem cell transplantation experiments indicate that cell therapy for MI needs further study. Divergent results indicate a lack of knowledge about the mechanisms by which cells survive and function in the repair of infarcted myocardium. Despite advances in cardiac cell therapy over the past decade, we are still looking for the best cell types for cardiac repair. It is encouraging that many factors have been found to promote stem cells to differentiate into cardiomyocytes. So far, the genetic and molecular mechanisms of cell homing, myocardial differentiation, and myocardial repair after cell transplantation are still unknown. Therefore, the research in this field needs more attention. More importantly, the safety problems found in various clinical trials, including the increase of arrhythmia attack frequency, the formation of teratoma, and the immune rejection of transplanted cells, need to be solved through more clinical trials.
4.2 Problems in gene therapy
Gene therapy improves cardiac function by promoting angiogenesis, reducing muscle reperfusion injury, and inhibiting apoptosis (Figure 2). However, for most experimental treatments, the safety of gene therapy for ischemic heart disease is very important. Although clinical trials have proved its short-term safety, the long-term observation effect of more than ten years is still lacking. Many confounding factors make it difficult to standardize the grouping of patients in clinical trials, including drug use and the medical environment. When evaluating results, objective rather than subjective evaluation may minimize the impact. The drug-related factors, including dosage, gene transfer efficiency, pharmacokinetics, and pharmacodynamics of individual therapy, must be accurate, because these factors may be different in different patients. The effectiveness analysis of treatment cost should also be considered, because the acquisition of gene therapy vectors is very cumbersome, requiring professional equipment and personnel, and the operation of gene therapy is also invasive to nature. Besides, the cost-benefit ratio of specific gene therapy is not comparable with the existing drugs for ischemic heart disease. Widespread use of small animals for preclinical research may lead to premature overzeal. Gene therapy experiments in large animals will provide a better research perspective. And an orchestration of gene therapy, cell therapy, and biocompatible materials might be a promising research field.
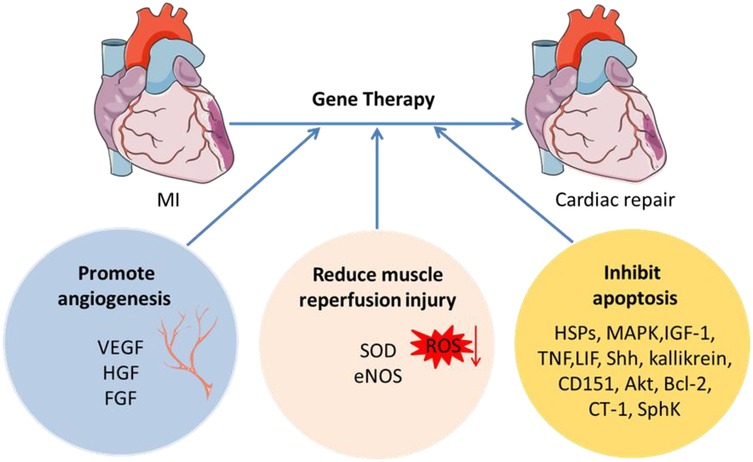
Figure 2. Schematic of gene therapy in MI. Gene therapy improves cardiac function by promoting angiogenesis, reducing muscle reperfusion injury, and inhibiting apoptosis. VEGF, vascular endothelial growth factor; HGF, hepatocyte growth factor; FGF, fibroblast growth factor; ROS, reactive oxygen species; SOD, superoxide dismutase; eNOS, endothelial nitric oxide synthetase; HSPs, heat shock proteins; MAPK, mitogen-activated protein kinase; IGF-1, insulin-like growth factor-1; TNF, tumor necrosis factor; LIF, leukemia inhibitory factor; Shh, sonic hedgehog; CT-1, Cardiac Troponin-1; SphK, sphingosine kinase.
4.3 Challenges and limitations
Although stem cell and gene therapy hold immense promise for treating cardiovascular diseases, translating these innovative strategies from the research laboratory to clinical practice presents significant challenges (154). Firstly, the immune system can recognize and attack therapeutic cells or vectors, leading to adverse reactions. Strategies to mitigate immune responses include using immune-privileged sites for cell delivery, modifying vectors to evade immune detection, and transiently suppressing the patient's immune system (155). Additionally, developing personalized therapies using autologous cells can reduce the risk of immune rejection. Precise targeting of therapeutic genes or cells to the affected tissue is crucial but challenging. Off-target effects can lead to unintended consequences. Advances in gene editing technologies, such as CRISPR/Cas9, allow for more precise targeting (156). Combining these technologies with advanced delivery systems, such as viral vectors or nanoparticles, can enhance the accuracy and efficiency of therapeutic delivery. Moreover, navigating the complex regulatory landscape and addressing ethical concerns are critical for clinical translation. Establishing clear guidelines and standards for stem cell and gene therapy research and clinical applications can facilitate regulatory approval (157). Engaging with regulatory bodies early in the development process and ensuring transparency in clinical trials are essential steps (158). As we know, high costs associated with the development and production of stem cell and gene therapies limit their accessibility. Developing cost-effective manufacturing processes and exploring alternative funding models, such as public-private partnerships, can make these therapies more affordable. Additionally, advocating for policies that support the development and reimbursement of novel therapies can improve patient access (159).
Recent years have seen significant progress in the clinical application of stem cell and gene therapy. For example, successful gene therapy trials for inherited blood disorders and certain genetic diseases have demonstrated the potential for long-term clinical benefits. Similarly, advancements in mesenchymal stem cell therapy have shown promise in treating various inflammatory and degenerative conditions (160).
4.4 Future directions
4.4.1 Optimizing cell delivery and engraftment
One of the major challenges in stem cell therapy for MI is the poor survival and engraftment of transplanted cells. Future research should focus on optimizing cell delivery methods to enhance cell retention and integration into the damaged myocardium. Techniques such as intramyocardial injection, trans-endocardial delivery, and intracoronary infusion have shown varying degrees of success, but further refinement is needed. Preconditioning stem cells to withstand the ischemic environment, genetic modification to improve cell viability, and the use of paracrine factors to support cell function are being explored. For instance, mesenchymal stem cells (MSCs) can be engineered to secrete higher levels of growth factors and anti-inflammatory cytokines, which can improve their therapeutic efficacy (4, 161). Additionally, combining stem cells with biomaterials or scaffolds can improve cell survival and promote tissue regeneration (2, 162).
4.4.2 Personalized and targeted therapies
The development of personalized therapies tailored to individual patients' needs is a key future direction. Advances in induced pluripotent stem cells (iPSCs) offer the potential for patient-specific cell therapies, reducing the risk of immune rejection (163). Additionally, gene editing technologies such as CRISPR/Cas9 can be used to correct genetic defects or enhance the therapeutic properties of cells (164). Targeted delivery systems, including nanoparticles and exosomes, can improve the precision of gene therapy, ensuring that therapeutic genes are expressed specifically in the damaged myocardium.
4.4.3 Large animal models and clinical trials
Preclinical studies in large animal models are essential for validating the efficacy and safety of stem cell and gene therapies before clinical translation. Large animals such as pigs and non-human primates share similar pathophysiology with humans, making them valuable for testing delivery strategies and assessing long-term outcomes (7, 165). Well-designed clinical trials, including randomized controlled trials with standardized protocols, are also crucial for evaluating the therapeutic potential of these interventions in humans.
5 Conclusion
Stem cell and gene therapy represent transformative approaches in the treatment of myocardial infarction, offering hope for improved cardiac function and reduced morbidity. While significant progress has been made, continued research and development are necessary to refine these therapies and address the remaining challenges. By leveraging advancements in technology, optimizing clinical protocols, and fostering collaborative efforts, we can pave the way for these innovative treatments to become standard clinical practices. The future of MI treatment lies in the successful translation of stem cell and gene therapy from the laboratory to the bedside, ultimately improving patient outcomes and quality of life.
Author contributions
SG: Writing – original draft. DL: Writing – original draft. BW: Writing – review & editing. HZ: Writing – review & editing. LC: Validation, Supervision, Writing – review & editing.
Funding
The author(s) declare financial support was received for the research, authorship, and/or publicationof this article. This work was supported by grants from Innovation Incubation Project of First Teaching Hospital of Tianjin University of Traditional Chinese Medicine (XB2024003 and ZZ2024007) for SG and DL, National Natural Science Foundation of China project (81904054) for SG. Science and Technology Development Fund of Tianjin Education Commission for Higher Education (2024ZD007) for SG.
Conflict of interest
The authors declare that the research was conducted in the absence of any commercial or financial relationships that could be construed as a potential conflict of interest.
Generative AI statement
The author(s) declare that no Generative AI was used in the creation of this manuscript.
Publisher's note
All claims expressed in this article are solely those of the authors and do not necessarily represent those of their affiliated organizations, or those of the publisher, the editors and the reviewers. Any product that may be evaluated in this article, or claim that may be made by its manufacturer, is not guaranteed or endorsed by the publisher.
References
1. Salari N, Morddarvanjoghi F, Abdolmaleki A, Rasoulpoor S, Khaleghi AA, Hezarkhani LA, et al. The global prevalence of myocardial infarction: a systematic review and meta-analysis. BMC Cardiovasc Disord. (2023) 23(1):206. doi: 10.1186/s12872-023-03231-w
2. Yan W, Xia Y, Zhao H, Xu X, Ma X, Tao L. Stem cell-based therapy in cardiac repair after myocardial infarction: promise, challenges, and future directions. J Mol Cell Cardiol. (2024) 188:1–14. doi: 10.1016/j.yjmcc.2023.12.009
3. Zhang Q, Wang L, Wang S, Cheng H, Xu L, Pei G, et al. Signaling pathways and targeted therapy for myocardial infarction. Signal Transduct Target Ther. (2022) 7(1):78. doi: 10.1038/s41392-022-00925-z
4. Barrère-Lemaire S, Vincent A, Jorgensen C, Piot C, Nargeot J, Djouad F. Mesenchymal stromal cells for improvement of cardiac function following acute myocardial infarction: a matter of timing. Physiol Rev. (2024) 104(2):659–725. doi: 10.1152/physrev.00009.2023
5. Martínez-Falguera D, Iborra-Egea O, Gálvez-Montón C. iPSC therapy for myocardial infarction in large animal models: land of hope and dreams. Biomedicines. (2021) 9(12):1836. doi: 10.3390/biomedicines9121836
6. Ala M. The beneficial effects of mesenchymal stem cells and their exosomes on myocardial infarction and critical considerations for enhancing their efficacy. Ageing Res Rev. (2023) 89:101980. doi: 10.1016/j.arr.2023.101980
7. Bois A, Grandela C, Gallant J, Mummery C, Menasché P. Revitalizing the heart: strategies and tools for cardiomyocyte regeneration post-myocardial infarction. NPJ Regen Med. (2025) 10(1):6. doi: 10.1038/s41536-025-00394-2
8. Shewale B, Ebrahim T, Samal A, Dubois N. Molecular regulation of cardiomyocyte maturation. Curr Cardiol Rep. (2025) 27(1):32. doi: 10.1007/s11886-024-02189-1
9. Lerchenmüller C, Hastings MH, Rabolli CP, Betge F, Roshan M, Liu LX, et al. CITED4 gene therapy protects against maladaptive cardiac remodeling after ischemia/reperfusion injury in mice. Mol Ther. (2024) 32(10):3683–94. doi: 10.1016/j.ymthe.2024.07.018
10. Song MH, Yoo J, Kwon DA, Chepurko E, Cho S, Fargnoli A, et al. Modified mRNA-mediated CCN5 gene transfer ameliorates cardiac dysfunction and fibrosis without adverse structural remodeling. Int J Mol Sci. (2024) 25(11):6262. doi: 10.3390/ijms25116262
11. Kukuła K, Urbanowicz A, Kłopotowski M, Dąbrowski M, Pręgowski J, Kądziela J, et al. Long-term follow-up and safety assessment of angiogenic gene therapy trial VIF-CAD: transcatheter intramyocardial administration of a bicistronic plasmid expressing VEGF-A165/bFGF cDNA for the treatment of refractory coronary artery disease. Am Heart J. (2019) 215:78–82. doi: 10.1016/j.ahj.2019.06.009
12. Fernández-Avilés F, Sanz-Ruiz R, Bogaert J, Casado Plasencia A, Gilaberte I, Belmans A, et al. Safety and efficacy of intracoronary infusion of allogeneic human cardiac stem cells in patients with ST-segment elevation myocardial infarction and left ventricular dysfunction. Circ Res. (2018) 123(5):579–89. doi: 10.1161/CIRCRESAHA.118.312823
13. Traverse JH, Henry TD, Pepine CJ, Willerson JT, Chugh A, Yang PC, et al. TIME trial: effect of timing of stem cell delivery following ST-elevation myocardial infarction on the recovery of global and regional left ventricular function: final 2-year analysis. Circ Res. (2018) 122(3):479–88. doi: 10.1161/CIRCRESAHA.117.311466
14. Lang CI, Dahmen A, Vasudevan P, Lemcke H, Gäbel R, Öner A, et al. Cardiac cell therapies for the treatment of acute myocardial infarction in mice: systematic review and meta-analysis. Cytotherapy. (2023) 25(6):640–52. doi: 10.1016/j.jcyt.2023.01.013
15. Thygesen K, Alpert JS, Jaffe AS, Chaitman BR, Bax JJ, Morrow DA, et al. Fourth universal definition of myocardial infarction (2018). Eur Heart J. (2019) 40(3):237–69. doi: 10.1093/eurheartj/ehy462
16. Cleland JG, Torabi A, Khan NK. Epidemiology and management of heart failure and left ventricular systolic dysfunction in the aftermath of a myocardial infarction. Heart. (2005) 91(Suppl 2):ii7–13; discussion ii31, ii43–8. doi: 10.1136/hrt.2005.062026
17. Zhu F, Meng Q, Yu Y, Shao L, Shen Z. Adult cardiomyocyte proliferation: a new insight for myocardial infarction therapy. J Cardiovasc Transl Res. (2020) 14(3):457–66. doi: 10.1007/s12265-020-10067-8
18. Povsic TJ, O'connor CM, Henry T, Taussig A, Kereiakes DJ, Fortuin FD, et al. A double-blind, randomized, controlled, multicenter study to assess the safety and cardiovascular effects of skeletal myoblast implantation by catheter delivery in patients with chronic heart failure after myocardial infarction. Am Heart J. (2011) 162(4):654–62.e1. doi: 10.1016/j.ahj.2011.07.020
19. Zhao L, Cheng G, Choksi K, Samanta A, Girgis M, Soder R, et al. Transplantation of human umbilical cord blood-derived cellular fraction improves left ventricular function and remodeling after myocardial ischemia/reperfusion. Circ Res. (2019) 125(8):759–72. doi: 10.1161/CIRCRESAHA.119.315216
20. Kim H, Kim S, Baek SH, Kwon SM. Pivotal cytoprotective mediators and promising therapeutic strategies for endothelial progenitor cell-based cardiovascular regeneration. Stem Cells Int. (2016) 2016:8340257. doi: 10.1155/2016/8340257
21. Wu Q, Wang J, Tan WLW, Jiang Y, Wang S, Li Q, et al. Extracellular vesicles from human embryonic stem cell-derived cardiovascular progenitor cells promote cardiac infarct healing through reducing cardiomyocyte death and promoting angiogenesis. Cell Death Dis. (2020) 11(5):354. doi: 10.1038/s41419-020-2508-y
22. Zarniko N, Skorska A, Steinhoff G, David R, Gaebel R. Dose-independent therapeutic benefit of bone marrow stem cell transplantation after MI in mice. Biomedicines. (2020) 8(6):157. doi: 10.3390/biomedicines8060157
23. Sid-Otmane C, Perrault LP, Ly HQ. Mesenchymal stem cell mediates cardiac repair through autocrine, paracrine and endocrine axes. J Transl Med. (2020) 18(1):336. doi: 10.1186/s12967-020-02504-8
24. Yuan X, Braun T. Multimodal regulation of cardiac myocyte proliferation. Circ Res. (2017) 121(3):293–309. doi: 10.1161/CIRCRESAHA.117.308428
25. Kadow ZA, Martin JF. Distinguishing cardiomyocyte division from binucleation. Circ Res. (2018) 123(9):1012–4. doi: 10.1161/CIRCRESAHA.118.313971
26. Piero A, Jan K. Ventricular myocytes are not terminally differentiated in the adult mammalian heart. Circ Res. (1998) 83(1):1–14. doi: 10.1161/01.RES.83.1.1
27. Bergmann O, Bhardwaj RD, Bernard S, Zdunek S, Barnabe-Heider F, Walsh S, et al. Evidence for cardiomyocyte renewal in humans. Science. (2009) 324(5923):98–102. doi: 10.1126/science.1164680
28. Haubner BJ, Schneider J, Schweigmann U, Schuetz T, Dichtl W, Velik-Salchner C, et al. Functional recovery of a human neonatal heart after severe myocardial infarction. Circ Res. (2016) 118(2):216–21. doi: 10.1161/CIRCRESAHA.115.307017
29. Scorsin M, Marotte F, Sabri A, Le Dref O, Demirag M, Samuel JL, et al. Can grafted cardiomyocytes colonize peri-infarct myocardial areas? Circulation. (1996) 94(9 Suppl):37–40.
30. Segers VF, Lee RT. Stem-cell therapy for cardiac disease. Nature. (2008) 451(7181):937–42. doi: 10.1038/nature06800
31. Yamamoto R, Miyagawa S, Toda K, Kainuma S, Yoshioka D, Yoshikawa Y, et al. Long-term outcome of ischemic cardiomyopathy after autologous myoblast cell-sheet implantation. Ann Thorac Surg. (2019) 108(5):e303–e6. doi: 10.1016/j.athoracsur.2019.03.028
32. Miyagawa S, Domae K, Yoshikawa Y, Fukushima S, Nakamura T, Saito A, et al. Phase I clinical trial of autologous stem cell-sheet transplantation therapy for treating cardiomyopathy. J Am Heart Assoc. (2017) 6(4):e003918. doi: 10.1161/JAHA.116.003918
33. Menasche P, Alfieri O, Janssens S, Mckenna W, Reichenspurner H, Trinquart L, et al. The myoblast autologous grafting in ischemic cardiomyopathy (MAGIC) trial: first randomized placebo-controlled study of myoblast transplantation. Circulation. (2008) 117(9):1189–200. doi: 10.1161/CIRCULATIONAHA.107.734103
34. Orlic D, Kajstura J, Chimenti S, Jakoniuk I, Anderson SM, Li B, et al. Bone marrow cells regenerate infarcted myocardium. Nature. (2001) 410(6829):701–5. doi: 10.1038/35070587
35. Sun L, Zhu W, Zhao P, Zhang J, Lu Y, Zhu Y, et al. Down-regulated exosomal MicroRNA-221 - 3p derived from senescent mesenchymal stem cells impairs heart repair. Front Cell Dev Biol. (2020) 8:263. doi: 10.3389/fcell.2020.00263
36. Han D, Yang J, Zhang E, Liu Y, Boriboun C, Qiao A, et al. Analysis of mesenchymal stem cell proteomes in situ in the ischemic heart. Theranostics. (2020) 10(24):11324–38. doi: 10.7150/thno.47893
37. Eisenberg LM, Burns L, Eisenberg CA. Hematopoietic cells from bone marrow have the potential to differentiate into cardiomyocytes in vitro. Anat Rec A Discov Mol Cell Evol Biol. (2003) 274(1):870–82. doi: 10.1002/ar.a.10106
38. Balsam LB, Wagers AJ, Christensen JL, Kofidis T, Weissman IL, Robbins RC. Haematopoietic stem cells adopt mature haematopoietic fates in ischaemic myocardium. Nature. (2004) 428(6983):668–73. doi: 10.1038/nature02460
39. Murry CE, Soonpaa MH, Reinecke H, Nakajima H, Nakajima HO, Rubar TM, et al. Haematopoietic stem cells do not transdifferentiate into cardiac myocytes in myocardial infarcts. Nature. (2004) 428(6983):664–8. doi: 10.1038/nature02446
40. Balsam LB, Robbins RC. Haematopoietic stem cells and repair of the ischaemic heart. Clin Sci. (2005) 109(6):483–92. doi: 10.1042/CS20050087
41. Deten A, Volz HC, Clamors S, Leiblein S, Briest W, Marx G, et al. Hematopoietic stem cells do not repair the infarcted mouse heart. Cardiovasc Res. (2005) 65(1):52–63. doi: 10.1016/j.cardiores.2004.11.009
42. Aceves JL, Lopez RV, Teran PM, Escobedo CM, Marroquin Mucino MA, Castillo GG, et al. Autologous CXCR4+ hematopoietic stem cells injected into the scar tissue of chronic myocardial infarction patients normalizes tissue contractility and perfusion. Arch Med Res. (2020) 51(2):135–44. doi: 10.1016/j.arcmed.2019.12.014
43. Bajek A, Gurtowska N, Olkowska J, Kazmierski L, Maj M, Drewa T. Adipose-derived stem cells as a tool in cell-based therapies. Arch Immunol Ther Exp. (2016) 64(6):443–54. doi: 10.1007/s00005-016-0394-x
44. Lee TM, Harn HJ, Chiou TW, Chuang MH, Chen CH, Chuang CH, et al. Preconditioned adipose-derived stem cells ameliorate cardiac fibrosis by regulating macrophage polarization in infarcted rat hearts through the PI3K/STAT3 pathway. Lab Invest. (2019) 99(5):634–47. doi: 10.1038/s41374-018-0181-x
45. Chen TS, Battsengel S, Kuo CH, Pan LF, Lin YM, Yao CH, et al. Stem cells rescue cardiomyopathy induced by P. Gingivalis-LPS via miR-181b. J Cell Physiol. (2018) 233(8):5869–76. doi: 10.1002/jcp.26386
46. Rehman J, Traktuev D, Li J, Merfeld-Clauss S, Temm-Grove CJ, Bovenkerk JE, et al. Secretion of angiogenic and antiapoptotic factors by human adipose stromal cells. Circulation. (2004) 109(10):1292–8. doi: 10.1161/01.CIR.0000121425.42966.F1
47. Kogler G, Sensken S, Airey JA, Trapp T, Muschen M, Feldhahn N, et al. A new human somatic stem cell from placental cord blood with intrinsic pluripotent differentiation potential. J Exp Med. (2004) 200(2):123–35. doi: 10.1084/jem.20040440
48. Kim BO, Tian H, Prasongsukarn K, Wu J, Angoulvant D, Wnendt S, et al. Cell transplantation improves ventricular function after a myocardial infarction: a preclinical study of human unrestricted somatic stem cells in a porcine model. Circulation. (2005) 112(9 Suppl):I96–104. doi: 10.1161/01.CIRCULATIONAHA.105.524678
49. Correa A, Ottoboni GS, Senegaglia AC, Capriglione LGA, Miyague NI, Leite LMB, et al. Expanded CD133(+) cells from human umbilical cord blood improved heart function in rats after severe myocardial infarction. Stem Cells Int. (2018) 2018:5412478. doi: 10.1155/2018/5412478
50. Peng Y, Chen B, Zhao J, Peng Z, Xu W, Yu G. Effect of intravenous transplantation of hUCB-MSCs on M1/M2 subtype conversion in monocyte/macrophages of AMI mice. Biomed Pharmacother. (2019) 111:624–30. doi: 10.1016/j.biopha.2018.12.095
51. Liu S, Chen J, Shi J, Zhou W, Wang L, Fang W, et al. M1-like macrophage-derived exosomes suppress angiogenesis and exacerbate cardiac dysfunction in a myocardial infarction microenvironment. Basic Res Cardiol. (2020) 115(2):22. doi: 10.1007/s00395-020-0781-7
52. Makkar RR, Kereiakes DJ, Aguirre F, Kowalchuk G, Chakravarty T, Malliaras K, et al. Intracoronary ALLogeneic heart STem cells to achieve myocardial regeneration (ALLSTAR): a randomized, placebo-controlled, double-blinded trial. Eur Heart J. (2020) 41(36):3451–8. doi: 10.1093/eurheartj/ehaa541
53. Grigorian-Shamagian L, Liu W, Fereydooni S, Middleton RC, Valle J, Cho JH, et al. Cardiac and systemic rejuvenation after cardiosphere-derived cell therapy in senescent rats. Eur Heart J. (2017) 38(39):2957–67. doi: 10.1093/eurheartj/ehx454
54. Zhao ZA, Han X, Lei W, Li J, Yang Z, Wu J, et al. Lack of cardiac improvement after cardiosphere-derived cell transplantation in aging mouse hearts. Circ Res. (2018) 123(10):e21–31. doi: 10.1161/CIRCRESAHA.118.313005
55. Kasai-Brunswick TH, Costa AR, Barbosa RA, Farjun B, Mesquita FC, Silva Dos Santos D, et al. Cardiosphere-derived cells do not improve cardiac function in rats with cardiac failure. Stem Cell Res Ther. (2017) 8(1):36. doi: 10.1186/s13287-017-0481-x
56. Jiang Y, Lian XL. Heart regeneration with human pluripotent stem cells: prospects and challenges. Bioactive Materials. (2020) 5(1):74–81. doi: 10.1016/j.bioactmat.2020.01.003
57. Zeevaert K, Elsafi Mabrouk MH, Wagner W, Goetzke R. Cell mechanics in embryoid bodies. Cells. (2020) 9(10):2270. doi: 10.3390/cells9102270
58. Glass C, Singla DK. Overexpression of TIMP-1 in embryonic stem cells attenuates adverse cardiac remodeling following myocardial infarction. Cell Transplant. (2012) 21(9):1931–44. doi: 10.3727/096368911X627561
59. Lee AS, Tang C, Rao MS, Weissman IL, Wu JC. Tumorigenicity as a clinical hurdle for pluripotent stem cell therapies. Nat Med. (2013) 19(8):998–1004. doi: 10.1038/nm.3267
60. International Stem Cell I. Assessment of established techniques to determine developmental and malignant potential of human pluripotent stem cells. Nat Commun. (2018) 9(1):1925. doi: 10.1038/s41467-018-04011-3
61. Nussbaum J, Minami E, Laflamme MA, Virag JA, Ware CB, Masino A, et al. Transplantation of undifferentiated murine embryonic stem cells in the heart: teratoma formation and immune response. FASEB J. (2007) 21(7):1345–57. doi: 10.1096/fj.06-6769com
62. Romagnuolo R, Masoudpour H, Porta-Sanchez A, Qiang B, Barry J, Laskary A, et al. Human embryonic stem cell-derived cardiomyocytes regenerate the infarcted pig heart but induce ventricular tachyarrhythmias. Stem Cell Rep. (2019) 12(5):967–81. doi: 10.1016/j.stemcr.2019.04.005
63. Tan Y, Wang L, Chen G, Liu W, Li Z, Wang Y, et al. Hyaluronate supports hESC-cardiomyocyte cell therapy for cardiac regeneration after acute myocardial infarction. Cell Prolif. (2020) 53(12):e12942. doi: 10.1111/cpr.12942
64. Takahashi K, Yamanaka S. Induction of pluripotent stem cells from mouse embryonic and adult fibroblast cultures by defined factors. Cell. (2006) 126(4):663–76. doi: 10.1016/j.cell.2006.07.024
65. Burridge PW, Matsa E, Shukla P, Lin ZC, Churko JM, Ebert AD, et al. Chemically defined generation of human cardiomyocytes. Nat Methods. (2014) 11(8):855–60. doi: 10.1038/nmeth.2999
66. Singla DK, Long X, Glass C, Singla RD, Yan B. Induced pluripotent stem (iPS) cells repair and regenerate infarcted myocardium. Mol Pharm. (2011) 8(5):1573–81. doi: 10.1021/mp2001704
67. Gao L, Wang L, Wei Y, Krishnamurthy P, Walcott GP, Menasche P, et al. Exosomes secreted by hiPSC-derived cardiac cells improve recovery from myocardial infarction in swine. Sci Transl Med. (2020) 12(561):eaay1318. doi: 10.1126/scitranslmed.aay1318
68. Jiang X, Yang Z, Dong M. Cardiac repair in a murine model of myocardial infarction with human induced pluripotent stem cell-derived cardiomyocytes. Stem Cell Res Ther. (2020) 11(1):297. doi: 10.1186/s13287-020-01811-7
69. Sun X, Wu J, Qiang B, Romagnuolo R, Gagliardi M, Keller G, et al. Transplanted microvessels improve pluripotent stem cell-derived cardiomyocyte engraftment and cardiac function after infarction in rats. Sci Transl Med. (2020) 12(562):eaax2992. doi: 10.1126/scitranslmed.aax2992
70. Park IH, Arora N, Huo H, Maherali N, Ahfeldt T, Shimamura A, et al. Disease-specific induced pluripotent stem cells. Cell. (2008) 134(5):877–86. doi: 10.1016/j.cell.2008.07.041
71. Eilers M, Eisenman RN. Myc’s broad reach. Genes Dev. (2008) 22(20):2755–66. doi: 10.1101/gad.1712408
72. Nakagawa M, Koyanagi M, Tanabe K, Takahashi K, Ichisaka T, Aoi T, et al. Generation of induced pluripotent stem cells without myc from mouse and human fibroblasts. Nat Biotechnol. (2008) 26(1):101–6. doi: 10.1038/nbt1374
73. Lee CH, Ingrole RSJ, Gill HS. Generation of induced pluripotent stem cells using elastin like polypeptides as a non-viral gene delivery system. Biochim Biophys Acta Mol Basis Dis. (2020) 1866(4):165405. doi: 10.1016/j.bbadis.2019.01.031
74. Zhu K, Li J, Lai H, Yang C, Guo C, Wang C. Reprogramming fibroblasts to pluripotency using arginine-terminated polyamidoamine nanoparticles based non-viral gene delivery system. Int J Nanomed. (2014) 9:5837–47. doi: 10.2147/IJN.S73961
75. Zhou H, Wu S, Joo JY, Zhu S, Han DW, Lin T, et al. Generation of induced pluripotent stem cells using recombinant proteins. Cell Stem Cell. (2009) 4(5):381–4. doi: 10.1016/j.stem.2009.04.005
76. Zhao H, Li Y, He L, Pu W, Yu W, Li Y, et al. In vivo AAV-CRISPR/Cas9-mediated gene editing ameliorates atherosclerosis in familial hypercholesterolemia. Circulation. (2020) 141(1):67–79. doi: 10.1161/CIRCULATIONAHA.119.042476
77. Kaneda Y. Development of liposomes and pseudovirions with fusion activity for efficient gene delivery. Curr Gene Ther. (2011) 11(6):434–41. doi: 10.2174/156652311798192789
78. Zhao T, Zhao W, Chen Y, Ahokas RA, Sun Y. Vascular endothelial growth factor (VEGF)-A: role on cardiac angiogenesis following myocardial infarction. Microvasc Res. (2010) 80(2):188–94. doi: 10.1016/j.mvr.2010.03.014
79. Tammela T, Enholm B, Alitalo K, Paavonen K. The biology of vascular endothelial growth factors. Cardiovasc Res. (2005) 65(3):550–63. doi: 10.1016/j.cardiores.2004.12.002
80. Rissanen TT, Yla-Herttuala S. Current status of cardiovascular gene therapy. Mol Ther. (2007) 15(7):1233–47. doi: 10.1038/sj.mt.6300175
81. Hao X, Mansson-Broberg A, Grinnemo KH, Siddiqui AJ, Dellgren G, Brodin LA, et al. Myocardial angiogenesis after plasmid or adenoviral VEGF-A(165) gene transfer in rat myocardial infarction model. Cardiovasc Res. (2007) 73(3):481–7. doi: 10.1016/j.cardiores.2006.10.011
82. Bulysheva AA, Hargrave B, Burcus N, Lundberg CG, Murray L, Heller R. Vascular endothelial growth factor-A gene electrotransfer promotes angiogenesis in a porcine model of cardiac ischemia. Gene Ther. (2016) 23(8-9):649–56. doi: 10.1038/gt.2016.35
83. Jacquier A, Higgins CB, Martin AJ, Do L, Saloner D, Saeed M. Injection of adeno-associated viral vector encoding vascular endothelial growth factor gene in infarcted swine myocardium: mR measurements of left ventricular function and strain. Radiology. (2007) 245(1):196–205. doi: 10.1148/radiol.2451061077
84. Ferrarini M, Arsic N, Recchia FA, Zentilin L, Zacchigna S, Xu X, et al. Adeno-associated virus-mediated transduction of VEGF165 improves cardiac tissue viability and functional recovery after permanent coronary occlusion in conscious dogs. Circ Res. (2006) 98(7):954–61. doi: 10.1161/01.RES.0000217342.83731.89
85. Kastrup J, Jorgensen E, Ruck A, Tagil K, Glogar D, Ruzyllo W, et al. Direct intramyocardial plasmid vascular endothelial growth factor-A165 gene therapy in patients with stable severe angina pectoris A randomized double-blind placebo-controlled study: the euroinject one trial. J Am Coll Cardiol. (2005) 45(7):982–8. doi: 10.1016/j.jacc.2004.12.068
86. Hartikainen J, Hassinen I, Hedman A, Kivela A, Saraste A, Knuuti J, et al. Adenoviral intramyocardial VEGF-DDeltaNDeltaC gene transfer increases myocardial perfusion reserve in refractory angina patients: a phase I/IIa study with 1-year follow-up. Eur Heart J. (2017) 38(33):2547–55. doi: 10.1093/eurheartj/ehx352
87. Eibel B, Markoski MM, Rodrigues CG, Dipp T, De Salles FB, Giusti I, et al. VEGF gene therapy cooperatively recruits molecules from the immune system and stimulates cell homing and angiogenesis in refractory angina. Cytokine. (2017) 91:44–50. doi: 10.1016/j.cyto.2016.12.005
88. Rong SL, Wang XL, Wang YC, Wu H, Zhou XD, Wang ZK, et al. Anti-inflammatory activities of hepatocyte growth factor in post-ischemic heart failure. Acta Pharmacol Sin. (2018) 39(10):1613–21. doi: 10.1038/aps.2018.14
89. Sonnenberg SB, Rane AA, Liu CJ, Rao N, Agmon G, Suarez S, et al. Delivery of an engineered HGF fragment in an extracellular matrix-derived hydrogel prevents negative LV remodeling post-myocardial infarction. Biomaterials. (2015) 45:56–63. doi: 10.1016/j.biomaterials.2014.12.021
90. Jayasankar V, Woo YJ, Bish LT, Pirolli TJ, Chatterjee S, Berry MF, et al. Gene transfer of hepatocyte growth factor attenuates postinfarction heart failure. Circulation. (2003) 108(Suppl 1):II230–6. doi: 10.1161/01.cir.0000087444.53354.6612970238
91. Cho KR, Choi JS, Hahn W, Kim DS, Park JS, Lee DS, et al. Therapeutic angiogenesis using naked DNA expressing two isoforms of the hepatocyte growth factor in a porcine acute myocardial infarction model. Eur J Cardiothorac Surg. (2008) 34(4):857–63. doi: 10.1016/j.ejcts.2008.05.045
92. Banquet S, Gomez E, Nicol L, Edwards-Levy F, Henry JP, Cao R, et al. Arteriogenic therapy by intramyocardial sustained delivery of a novel growth factor combination prevents chronic heart failure. Circulation. (2011) 124(9):1059–69. doi: 10.1161/CIRCULATIONAHA.110.010264
93. Kondo I, Ohmori K, Oshita A, Takeuchi H, Fuke S, Shinomiya K, et al. Treatment of acute myocardial infarction by hepatocyte growth factor gene transfer: the first demonstration of myocardial transfer of a “functional” gene using ultrasonic microbubble destruction. J Am Coll Cardiol. (2004) 44(3):644–53. doi: 10.1016/j.jacc.2004.04.042
94. Park BW, Jung SH, Das S, Lee SM, Park JH, Kim H, et al. In vivo priming of human mesenchymal stem cells with hepatocyte growth factor-engineered mesenchymal stem cells promotes therapeutic potential for cardiac repair. Sci Adv. (2020) 6(13):eaay6994. doi: 10.1126/sciadv.aay6994
95. Zhu K, Wu M, Lai H, Guo C, Li J, Wang Y, et al. Nanoparticle-enhanced generation of gene-transfected mesenchymal stem cells for in vivo cardiac repair. Biomaterials. (2016) 74:188–99. doi: 10.1016/j.biomaterials.2015.10.010
96. Meng H, Chen B, Tao Z, Xu Z, Wang L, Weizhu J, et al. Safety and efficacy of adenovirus carrying hepatocyte growth factor gene by percutaneous endocardial injection for treating post-infarct heart failure: a phase IIa clinical trial. Curr Gene Ther. (2018) 18(2):125–30. doi: 10.2174/1566523218666180404162209
97. Yang ZJ, Zhang YR, Chen B, Zhang SL, Jia EZ, Wang LS, et al. Phase I clinical trial on intracoronary administration of ad-hHGF treating severe coronary artery disease. Mol Biol Rep. (2009) 36(6):1323–9. doi: 10.1007/s11033-008-9315-3
98. Ornitz DM, Itoh N. Fibroblast growth factors. Genome Biol. (2001) 2(3):REVIEWS3005. doi: 10.1186/gb-2001-2-3-reviews3005
99. Palmen M, Daemen MJ, De Windt LJ, Willems J, Dassen WR, Heeneman S, et al. Fibroblast growth factor-1 improves cardiac functional recovery and enhances cell survival after ischemia and reperfusion: a fibroblast growth factor receptor, protein kinase C, and tyrosine kinase-dependent mechanism. J Am Coll Cardiol. (2004) 44(5):1113–23. doi: 10.1016/j.jacc.2004.05.067
100. Rao Z, Shen D, Chen J, Jin L, Wu X, Chen M, et al. Basic fibroblast growth factor attenuates injury in myocardial infarction by enhancing hypoxia-inducible factor-1 alpha accumulation. Front Pharmacol. (2020) 11:1193. doi: 10.3389/fphar.2020.01193
101. Tong G, Liang Y, Xue M, Chen X, Wang J, An N, et al. The protective role of bFGF in myocardial infarction and hypoxia cardiomyocytes by reducing oxidative stress via Nrf2. Biochem Biophys Res Commun. (2020) 527(1):15–21. doi: 10.1016/j.bbrc.2020.04.053
102. Fan Z, Xu Z, Niu H, Sui Y, Li H, Ma J, et al. Spatiotemporal delivery of basic fibroblast growth factor to directly and simultaneously attenuate cardiac fibrosis and promote cardiac tissue vascularization following myocardial infarction. J Controlled Release. (2019) 311-312:233–44. doi: 10.1016/j.jconrel.2019.09.005
103. Li J, Xu C, Liu Y, Li Y, Du S, Zhang R, et al. Fibroblast growth factor 21 inhibited ischemic arrhythmias via targeting miR-143/EGR1 axis. Basic Res Cardiol. (2020) 115(2):9. doi: 10.1007/s00395-019-0768-4
104. Gao MH, Lai NC, Mckirnan MD, Roth DA, Rubanyi GM, Dalton N, et al. Increased regional function and perfusion after intracoronary delivery of adenovirus encoding fibroblast growth factor 4: report of preclinical data. Hum Gene Ther. (2004) 15(6):574–87. doi: 10.1089/104303404323142024
105. Giordano FJ, Ping P, Mckirnan MD, Nozaki S, Demaria AN, Dillmann WH, et al. Intracoronary gene transfer of fibroblast growth factor-5 increases blood flow and contractile function in an ischemic region of the heart. Nat Med. (1996) 2(5):534–9. doi: 10.1038/nm0596-534
106. Suzuki G, Lee TC, Fallavollita JA, Canty JM Jr. Adenoviral gene transfer of FGF-5 to hibernating myocardium improves function and stimulates myocytes to hypertrophy and reenter the cell cycle. Circ Res. (2005) 96(7):767–75. doi: 10.1161/01.RES.0000162099.01268.d1
107. Zima AV, Blatter LA. Redox regulation of cardiac calcium channels and transporters. Cardiovasc Res. (2006) 71(2):310–21. doi: 10.1016/j.cardiores.2006.02.019
108. Wu J, Hecker JG, Chiamvimonvat N. Antioxidant enzyme gene transfer for ischemic diseases. Adv Drug Delivery Rev. (2009) 61(4):351–63. doi: 10.1016/j.addr.2009.01.005
109. Li Q, Bolli R, Qiu Y, Tang XL, Guo Y, French BA. Gene therapy with extracellular superoxide dismutase protects conscious rabbits against myocardial infarction. Circulation. (2001) 103(14):1893–8. doi: 10.1161/01.CIR.103.14.1893
110. Konkalmatt PR, Beyers RJ, O'connor DM, Xu Y, Seaman ME, French BA. Cardiac-selective expression of extracellular superoxide dismutase after systemic injection of adeno-associated virus 9 protects the heart against post-myocardial infarction left ventricular remodeling. Circulation Cardiovascular Imaging. (2013) 6(3):478–86. doi: 10.1161/CIRCIMAGING.112.000320
111. Daiber A, Xia N, Steven S, Oelze M, Hanf A, Kroller-Schon S, et al. New therapeutic implications of endothelial nitric oxide synthase (eNOS) function/dysfunction in cardiovascular disease. Int J Mol Sci. (2019) 20(1):187. doi: 10.3390/ijms20010187
112. Smith RS Jr, Agata J, Xia CF, Chao L, Chao J. Human endothelial nitric oxide synthase gene delivery protects against cardiac remodeling and reduces oxidative stress after myocardial infarction. Life Sci. (2005) 76(21):2457–71. doi: 10.1016/j.lfs.2004.11.028
113. Fraccarollo D, Widder JD, Galuppo P, Thum T, Tsikas D, Hoffmann M, et al. Improvement in left ventricular remodeling by the endothelial nitric oxide synthase enhancer AVE9488 after experimental myocardial infarction. Circulation. (2008) 118(8):818–27. doi: 10.1161/CIRCULATIONAHA.107.717702
114. Kaur S, Kumar TR, Uruno A, Sugawara A, Jayakumar K, Kartha CC. Genetic engineering with endothelial nitric oxide synthase improves functional properties of endothelial progenitor cells from patients with coronary artery disease: an in vitro study. Basic Res Cardiol. (2009) 104(6):739–49. doi: 10.1007/s00395-009-0039-x
115. Wang W, Tan B, Chen J, Bao R, Zhang X, Liang S, et al. An injectable conductive hydrogel encapsulating plasmid DNA-eNOs and ADSCs for treating myocardial infarction. Biomaterials. (2018) 160:69–81. doi: 10.1016/j.biomaterials.2018.01.021
116. Chen L, Zhang Y, Tao L, Yang Z, Wang L. Mesenchymal stem cells with eNOS over-expression enhance cardiac repair in rats with myocardial infarction. Cardiovasc Drugs Ther. (2017) 31(1):9–18. doi: 10.1007/s10557-016-6704-z
117. Qian J, Vafiadaki E, Florea SM, Singh VP, Song W, Lam CK, et al. Small heat shock protein 20 interacts with protein phosphatase-1 and enhances sarcoplasmic reticulum calcium cycling. Circ Res. (2011) 108(12):1429–38. doi: 10.1161/CIRCRESAHA.110.237644
118. Yu DW, Ge PP, Liu AL, Yu XY, Liu TT. HSP20-mediated Cardiomyocyte exosomes improve cardiac function in mice with myocardial infarction by activating akt signaling pathway. Eur Rev Med Pharmacol Sci. (2019) 23(11):4873–81. doi: 10.26355/eurrev_201906_18075
119. Wu J, Chen S, Liu Y, Liu Z, Wang D, Cheng Y. Therapeutic perspectives of heat shock proteins and their protein-protein interactions in myocardial infarction. Pharmacol Res. (2020) 160:105162. doi: 10.1016/j.phrs.2020.105162
120. Lin KM, Lin B, Lian IY, Mestril R, Scheffler IE, Dillmann WH. Combined and individual mitochondrial HSP60 and HSP10 expression in cardiac myocytes protects mitochondrial function and prevents apoptotic cell deaths induced by simulated ischemia-reoxygenation. Circulation. (2001) 103(13):1787–92. doi: 10.1161/01.CIR.103.13.1787
121. Okubo S, Wildner O, Shah MR, Chelliah JC, Hess ML, Kukreja RC. Gene transfer of heat-shock protein 70 reduces infarct size in vivo after ischemia/reperfusion in the rabbit heart. Circulation. (2001) 103(6):877–81. doi: 10.1161/01.CIR.103.6.877
122. Zhao YT, Du J, Yano N, Wang H, Wang J, Dubielecka PM, et al. p38-Regulated/activated protein kinase plays a pivotal role in protecting heart against ischemia-reperfusion injury and preserving cardiac performance. Am J Physiol Cell Physiol. (2019) 317(3):C525–C33. doi: 10.1152/ajpcell.00122.2019
123. Tenhunen O, Soini Y, Ilves M, Rysa J, Tuukkanen J, Serpi R, et al. P38 kinase rescues failing myocardium after myocardial infarction: evidence for angiogenic and anti-apoptotic mechanisms. FASEB J. (2006) 20(11):1907–9. doi: 10.1096/fj.05-5618fje
124. Heusch P, Canton M, Aker S, Van De Sand A, Konietzka I, Rassaf T, et al. The contribution of reactive oxygen species and p38 mitogen-activated protein kinase to myofilament oxidation and progression of heart failure in rabbits. Br J Pharmacol. (2010) 160(6):1408–16. doi: 10.1111/j.1476-5381.2010.00793.x
125. Lin M, Liu X, Zheng H, Huang X, Wu Y, Huang A, et al. IGF-1 enhances BMSC viability, migration, and anti-apoptosis in myocardial infarction via secreted frizzled-related protein 2 pathway. Stem Cell Res Ther. (2020) 11(1):22. doi: 10.1186/s13287-019-1544-y
126. Baez-Diaz C, Blanco-Blazquez V, Sanchez-Margallo FM, Bayes-Genis A, Gonzalez I, Abad A, et al. Microencapsulated insulin-like growth factor-1 therapy improves cardiac function and reduces fibrosis in a porcine acute myocardial infarction model. Sci Rep. (2020) 10(1):7166. doi: 10.1038/s41598-020-64097-y
127. Sugano M, Tsuchida K, Hata T, Makino N. In vivo transfer of soluble TNF-alpha receptor 1 gene improves cardiac function and reduces infarct size after myocardial infarction in rats. FASEB J. (2004) 18(7):911–3. doi: 10.1096/fj.03-1148fje
128. Padfield GJ, Din JN, Koushiappi E, Mills NL, Robinson SD, Cruden Nle M, et al. Cardiovascular effects of tumour necrosis factor alpha antagonism in patients with acute myocardial infarction: a first in human study. Heart. (2013) 99(18):1330–5. doi: 10.1136/heartjnl-2013-303648
129. Zhang C, Liu J, Wang J, Hu W, Feng Z. The emerging role of leukemia inhibitory factor in cancer and therapy. Pharmacol Ther. (2020) 221:107754. doi: 10.1016/j.pharmthera.2020.107754
130. Zou Y, Takano H, Mizukami M, Akazawa H, Qin Y, Toko H, et al. Leukemia inhibitory factor enhances survival of cardiomyocytes and induces regeneration of myocardium after myocardial infarction. Circulation. (2003) 108(6):748–53. doi: 10.1161/01.CIR.0000081773.76337.44
131. Kanda M, Nagai T, Takahashi T, Liu ML, Kondou N, Naito AT, et al. Leukemia inhibitory factor enhances endogenous cardiomyocyte regeneration after myocardial infarction. PLoS One. (2016) 11(5):e0156562. doi: 10.1371/journal.pone.0156562
132. Kusano KF, Pola R, Murayama T, Curry C, Kawamoto A, Iwakura A, et al. Sonic hedgehog myocardial gene therapy: tissue repair through transient reconstitution of embryonic signaling. Nat Med. (2005) 11(11):1197–204. doi: 10.1038/nm1313
133. Ghaleh B, Thireau J, Cazorla O, Soleti R, Scheuermann V, Bize A, et al. Cardioprotective effect of sonic hedgehog ligand in pig models of ischemia reperfusion. Theranostics. (2020) 10(9):4006–16. doi: 10.7150/thno.40461
134. Agata J, Chao L, Chao J. Kallikrein gene delivery improves cardiac reserve and attenuates remodeling after myocardial infarction. Hypertension. (2002) 40(5):653–9. doi: 10.1161/01.HYP.0000036035.41122.99
135. Yao YY, Yin H, Shen B, Smith RS Jr, Liu Y, Gao L, et al. Tissue kallikrein promotes neovascularization and improves cardiac function by the akt-glycogen synthase kinase-3beta pathway. Cardiovasc Res. (2008) 80(3):354–64. doi: 10.1093/cvr/cvn223
136. Zuo HJ, Liu ZX, Liu XC, Yang J, Liu T, Wen S, et al. Assessment of myocardial blood perfusion improved by CD151 in a pig myocardial infarction model. Acta Pharmacol Sin. (2009) 30(1):70–7. doi: 10.1038/aps.2008.10
137. Yang SL, Tang KQ, Tao JJ, Wan AH, Lin YD, Nan SL, et al. Delivery of CD151 by ultrasound microbubbles in rabbit myocardial infarction. Cardiology. (2016) 135(4):221–7. doi: 10.1159/000446639
138. Zuo H, Liu Z, Liu X, Yang J, Liu T, Wen S, et al. CD151 gene delivery after myocardial infarction promotes functional neovascularization and activates FAK signaling. Mol Med. (2009) 15(9-10):307–15. doi: 10.2119/molmed.2009.00025
139. Cittadini A, Monti MG, Iaccarino G, Di Rella F, Tsichlis PN, Di Gianni A, et al. Adenoviral gene transfer of akt enhances myocardial contractility and intracellular calcium handling. Gene Ther. (2006) 13(1):8–19. doi: 10.1038/sj.gt.3302589
140. Brocheriou V, Hagege AA, Oubenaissa A, Lambert M, Mallet VO, Duriez M, et al. Cardiac functional improvement by a human bcl-2 transgene in a mouse model of ischemia/reperfusion injury. J Gene Med. (2000) 2(5):326–33. doi: 10.1002/1521-2254(200009/10)2:5%3C326::AID-JGM133%3E3.0.CO;2-1
141. Chatterjee S, Stewart AS, Bish LT, Jayasankar V, Kim EM, Pirolli T, et al. Viral gene transfer of the antiapoptotic factor bcl-2 protects against chronic postischemic heart failure. Circulation. (2002) 106(12 Suppl 1):I212–7.12354736
142. Ruixing Y, Jinzhen W, Dezhai Y, Jiaquan L. Cardioprotective role of cardiotrophin-1 gene transfer in a murine model of myocardial infarction. Growth Factors. (2007) 25(4):286–94. doi: 10.1080/08977190701781289
143. Bortolotti F, Ruozi G, Falcione A, Doimo S, Dal Ferro M, Lesizza P, et al. In vivo functional selection identifies cardiotrophin-1 as a cardiac engraftment factor for mesenchymal stromal cells. Circulation. (2017) 136(16):1509–24. doi: 10.1161/CIRCULATIONAHA.117.029003
144. Jozefczuk E, Guzik TJ, Siedlinski M. Significance of sphingosine-1-phosphate in cardiovascular physiology and pathology. Pharmacol Res. (2020) 156:104793. doi: 10.1016/j.phrs.2020.104793
145. Duan HF, Wang H, Yi J, Liu HJ, Zhang QW, Li LB, et al. Adenoviral gene transfer of sphingosine kinase 1 protects heart against ischemia/reperfusion-induced injury and attenuates its postischemic failure. Hum Gene Ther. (2007) 18(11):1119–28. doi: 10.1089/hum.2007.036
146. Menasche P, Vanneaux V, Hagege A, Bel A, Cholley B, Cacciapuoti I, et al. Human embryonic stem cell-derived cardiac progenitors for severe heart failure treatment: first clinical case report. Eur Heart J. (2015) 36(30):2011–7. doi: 10.1093/eurheartj/ehv189
147. Losordo DW, Schatz RA, White CJ, Udelson JE, Veereshwarayya V, Durgin M, et al. Intramyocardial transplantation of autologous CD34+ stem cells for intractable angina: a phase I/IIa double-blind, randomized controlled trial. Circulation. (2007) 115(25):3165–72. doi: 10.1161/CIRCULATIONAHA.106.687376
148. Wollert KC, Meyer GP, Lotz J, Ringes-Lichtenberg S, Lippolt P, Breidenbach C, et al. Intracoronary autologous bone-marrow cell transfer after myocardial infarction: the BOOST randomised controlled clinical trial. Lancet. (2004) 364(9429):141–8. doi: 10.1016/S0140-6736(04)16626-9
149. Wollert KC, Meyer GP, Muller-Ehmsen J, Tschope C, Bonarjee V, Larsen AI, et al. Intracoronary autologous bone marrow cell transfer after myocardial infarction: the BOOST-2 randomised placebo-controlled clinical trial. Eur Heart J. (2017) 38(39):2936–43. doi: 10.1093/eurheartj/ehx188
150. Tendera M, Wojakowski W, Ruzyllo W, Chojnowska L, Kepka C, Tracz W, et al. Intracoronary infusion of bone marrow-derived selected CD34+CXCR4+ cells and non-selected mononuclear cells in patients with acute STEMI and reduced left ventricular ejection fraction: results of randomized, multicentre myocardial regeneration by intracoronary infusion of selected population of stem cells in acute myocardial infarction (REGENT) trial. Eur Heart J. (2009) 30(11):1313–21. doi: 10.1093/eurheartj/ehp073
151. Schachinger V, Assmus B, Britten MB, Honold J, Lehmann R, Teupe C, et al. Transplantation of progenitor cells and regeneration enhancement in acute myocardial infarction: final one-year results of the TOPCARE-AMI trial. J Am Coll Cardiol. (2004) 44(8):1690–9. doi: 10.1016/j.jacc.2004.08.014
152. Traverse JH, Henry TD, Pepine CJ, Willerson JT, Zhao DX, Ellis SG, et al. Effect of the use and timing of bone marrow mononuclear cell delivery on left ventricular function after acute myocardial infarction: the TIME randomized trial. Jama. (2012) 308(22):2380–9. doi: 10.1001/jama.2012.28726
153. Mathur A, Fernandez-Aviles F, Bartunek J, Belmans A, Crea F, Dowlut S, et al. The effect of intracoronary infusion of bone marrow-derived mononuclear cells on all-cause mortality in acute myocardial infarction: the BAMI trial. Eur Heart J. (2020) 41(38):3702–10. doi: 10.1093/eurheartj/ehaa651
154. Lee NK, Chang JW. Manufacturing cell and gene therapies: challenges in clinical translation. Ann Lab Med. (2024) 44(4):314–23. doi: 10.3343/alm.2023.0382
155. Apostolou E, Blau H, Chien K, Lancaster MA, Tata PR, Trompouki E, et al. Progress and challenges in stem cell biology. Nat Cell Biol. (2023) 25(2):203–6. doi: 10.1038/s41556-023-01087-y
156. Kohn DB, Chen YY, Spencer MJ. Successes and challenges in clinical gene therapy. Gene Ther. (2023) 30(10-11):738–46. doi: 10.1038/s41434-023-00390-5
157. Talib S, Shepard KA. Unleashing the cure: overcoming persistent obstacles in the translation and expanded use of hematopoietic stem cell-based therapies. Stem Cells Transl Med. (2020) 9(4):420–6. doi: 10.1002/sctm.19-0375
158. Cao J, Hao J, Wang L, Tan Y, Tian Y, Li S, et al. Developing standards to support the clinical translation of stem cells. Stem Cells Transl Med. (2021) 10(Suppl 2):S85–s95. doi: 10.1002/sct3.13035
159. Daley GQ, Hyun I, Apperley JF, Barker RA, Benvenisty N, Bredenoord AL, et al. Setting global standards for stem cell research and clinical translation: the 2016 ISSCR guidelines. Stem Cell Rep. (2016) 6(6):787–97. doi: 10.1016/j.stemcr.2016.05.001
160. Mei R, Wan Z, Yang C, Shen X, Wang R, Zhang H, et al. Advances and clinical challenges of mesenchymal stem cell therapy. Front Immunol. (2024) 15:1421854. doi: 10.3389/fimmu.2024.1421854
161. Liu Y, Wang M, Yu Y, Li C, Zhang C. Advances in the study of exosomes derived from mesenchymal stem cells and cardiac cells for the treatment of myocardial infarction. Cell Commun Signal. (2023) 21(1):202. doi: 10.1186/s12964-023-01227-9
162. Smagul S, Kim Y, Smagulova A, Raziyeva K, Nurkesh A, Saparov A. Biomaterials loaded with growth factors/cytokines and stem cells for cardiac tissue regeneration. Int J Mol Sci. (2020) 21(17):5952. doi: 10.3390/ijms21175952
163. Clavellina D, Balkan W, Hare JM. Stem cell therapy for acute myocardial infarction: mesenchymal stem cells and induced pluripotent stem cells. Expert Opin Biol Ther. (2023) 23(10):951–67. doi: 10.1080/14712598.2023.2245329
164. Zannas AS, Jia M, Hafner K, Baumert J, Wiechmann T, Pape JC, et al. Epigenetic upregulation of FKBP5 by aging and stress contributes to NF-κB-driven inflammation and cardiovascular risk. Proc Natl Acad Sci U S A. (2019) 116(23):11370–9. doi: 10.1073/pnas.1816847116
Keywords: myocardial infarction, stem cells, cell transplantation, gene technology, therapy, safety
Citation: Gao S, Li D, Wang B, Zhang H and Chen L (2025) Two promising approaches in the treatment of myocardial infarction: stem cells and gene therapy. Front. Cardiovasc. Med. 12:1540066. doi: 10.3389/fcvm.2025.1540066
Received: 5 December 2024; Accepted: 31 January 2025;
Published: 19 February 2025.
Edited by:
Qiuwang Zhang, St Michael's Hospital, CanadaReviewed by:
Jing Li, University of Toronto, CanadaYanzhuo Ma, Bethune International Peace Hospital, China
Copyright: © 2025 Gao, Li, Wang, Zhang and Chen. This is an open-access article distributed under the terms of the Creative Commons Attribution License (CC BY). The use, distribution or reproduction in other forums is permitted, provided the original author(s) and the copyright owner(s) are credited and that the original publication in this journal is cited, in accordance with accepted academic practice. No use, distribution or reproduction is permitted which does not comply with these terms.
*Correspondence: Shan Gao, dGluYXhpYW9qdWFuODkxMUAxMjYuY29t; Lu Chen, Y2hlbmx1dGp1dGNtQHRqdXRjbS5lZHUuY24=
†These authors have contributed equally to this work