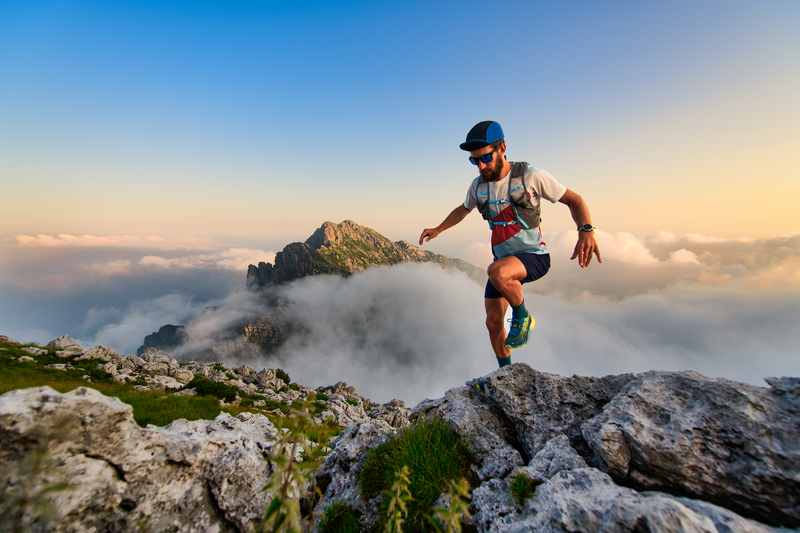
95% of researchers rate our articles as excellent or good
Learn more about the work of our research integrity team to safeguard the quality of each article we publish.
Find out more
HYPOTHESIS AND THEORY article
Front. Cardiovasc. Med. , 18 March 2025
Sec. Heart Failure and Transplantation
Volume 12 - 2025 | https://doi.org/10.3389/fcvm.2025.1535778
As heart transplantation continues to be the gold standard therapy for end-stage heart failure, the imbalance between the supply of hearts, and the demand for them, continues to get worse. In the US alone, with less than 4,000 hearts suitable for transplant and over 100,000 potential recipients, this therapy is only available to a very few. The use of hearts Donated after Circulatory Death (DCD) and Donation after Brain Death (DBD) using ex vivo machine perfusion (EVMP) is a promising approach that has already increased the availability of suitable organs for heart transplantation. EVMP offers the promise of enabling the expansion of the overall number of heart transplants and lower rates of early graft dysfunction. These are realized through (1) safe extension of the time between procurement and transplantation and (2) ex vivo assessment of preserved hearts. Notably, ex vivo perfusion has facilitated the donation of DCD hearts and improved the success of transplantation. Nevertheless, DCD hearts suffer from serious preharvest ischemia/reperfusion injury (IRI). Despite these developments, only 40% of hearts offered for transplantation can be utilized. These devices do offer an opportunity to evaluate donor hearts for transplantation, resuscitate organs previously deemed unsuitable for transplantation, and provide a platform for the development of novel therapeutics to limit cardiac injury. Bone Morphogenetic Protein (BMP) signaling is a new target which holds the potential for ameliorating myocardial IRI. Recent studies have demonstrated that BMP signaling has a significant role in blocking the deleterious effects of injury to the heart. We have designed novel small peptide BMP mimetics that act via activin receptor-like kinase (ALK3), a type I BMP receptor. They are capable of (1) inhibiting inflammation and apoptosis, (2) blocking/reversing the epithelial-mesenchymal transition (EMT) and fibrosis, and (3) promoting tissue regeneration. In this review, we explore the promise that novel therapeutics, including these BMP mimetics, offer for the protection of hearts against myocardial injury during ex vivo transportation for cardiac transplantation. This protection represents a significant advance and a promising ex vivo therapeutic approach to expanding the donor pool by increasing the number of transplantable hearts.
Heart Disease is the leading cause of death in the USA (National Center for Health Statistics 2023, https://www.cdc.gov/nchs/fastats/leading-causes-of-death.htm). In the USA in 2022, there were 702,880 deaths from heart disease and the cost of heart failure accounted for the major sub-proportion of this dread disease, with an annual incidence of congestive heart failure in the US of over 300,000 and an annual mortality of over 100,000 (1). In 2018, there were 6.2 million US adults that had heart failure and in 2020 the cost of heart failure/disease to US society was estimated to be $252.2 billion (https://www.cdc.gov/heart-disease/data-research/facts-stats/index.html).
Congestive heart failure can be caused by several different insults including acute myocardial infarction and other myocardial injuries. The annual adjusted US incidence of hospitalization for acute myocardial infarction is approximately 550,000 for first episodes (2) and 200,000 for recurrent episodes (3). Ultimately, these insults lead to contractile dysfunction mediated at a cellular level by processes including apoptosis, inflammation, and fibrosis (4). It is a complicated pathophysiology involving many different molecular messengers, affecting various cell types and includes cellular differentiation and proliferation (5).
The only definitive therapy available is a heart transplant. While this procedure is lifesaving, it has many complications and is limited by a finite supply of donor hearts. In the US, between 2000 and 2010, the number of heart transplant remained relatively constant at between 3,500 and 4,000 (6). In 2022, there were 3,789 hearts transplanted. Of the 10,000 hearts in the donor pool, approximately 6,000 were deemed unsuitable for transplantation. Because of this attrition in the donated heart pool, many patients die awaiting a suitable donor heart (6). In 2019, 20 patients on the waiting list died each day (7). If we could prevent injury and resuscitate or regenerate some of the hearts deemed unsuitable, we could decrease the time on the waiting list, increase the number of transplants, and save many more lives. In this paper we examine ex vivo therapeutic interventions and conventional interventions that can prevent injury and promote recovery.
The standard procedure for heart transplantation procurement consists of a number of steps involving complex logistic coordination. The procurement process is initiated when a donor is identified at a donor hospital, and the patient has been declared dead. The donor institution then contacts the United Network for Organ Sharing (UNOS) indicating that they have a potential donor. UNOS then screens their list of patients waiting for a heart transplant and contacts the hospital team caring for that patient. The potential recipient institution then initiates a “suitability evaluation”. If the donor's heart is deemed suitable for transplant to the patient selected by UNOS, a team from the recipient hospital is assembled and travels to the donor institution to retrieve the donor's heart. The team then explants the donor's heart and places it in an ice bath for transport to the recipient's hospital. This storage in an ice bath is known as Static Cold Storage (SCS) and has been the standard of care until recently (Figure 1). During SCS the explanted heart does not have a supply of oxygen or nutrients necessary for the generation of energy, which results in ischemic injury. The rationale behind SCS was that hypothermia would minimize the demand for oxygen and essential nutrients, thereby minimizing this ischemic injury. In common medical practice, an effort is made to limit static cold storage time to 3–4 h, beyond which SCS has been shown to increase post-transplant graft dysfunction with attendant medical complications and a higher mortality of the transplant recipient (8–10).
Figure 1. Revolutionizing heart transplantation: exploring donations after brain death (DBD) and donations after circulatory death (DCD).
There are several reasons for not utilizing the available hearts, such as age >50 years, female sex, donor comorbidities like cerebrovascular accident, hypertension, diabetes, left ventricle dysfunction, and elevated troponin levels (11). One type of injury that occurs in the hearts during procurement and transportation between donor and recipient for heart transplant is known to be ischemia-reperfusion injury (IRI) (8). IRI leads to complications in the immediate post-transplant phase, which include injury to the right ventricle (RV), left ventricle (LV), lungs, kidneys, and liver. In the immediate post-operative days, recipient patients may require inotropes, pressors and temporary Mechanical Circulatory Support (MCS).
Ischemia-reperfusion injury is a common concern in organ transplantation, especially when the donor heart has been preserved for a period of time, leading to potential damage during the reperfusion phase after transplantation (12).
Depending on the severity of the ischemia-reperfusion injury, a patient might require temporary MCS like an intra-aortic balloon pump or extracorporeal membrane oxygenation (ECMO) to stabilize their blood pressure and cardiac output until the transplanted heart recovers (Short-term mechanical circulatory support (intra-aortic balloon pump, Impella, extracorporeal membrane oxygenation, TandemHeart (13).
While MCS can be helpful in certain situations, it is not routinely needed after every heart transplant and is typically only used when the patient shows signs of significant circulatory instability due to the reperfusion injury (14). These complications and treatments can lead to prolonged CCU stays and more permanent MCS and/or renal replacement therapy. This type of injury can also increase the likelihood of organ compromise and shorten graft survival after discharge. Data also show that this type of injury correlates with a higher incidence of cardiac fibrosis and higher long-term mortality (15–17).
The advent of ex vivo devices that enable perfusion of hearts during transportation for transplant increases the number of donor hearts available for transplant and provides the possibility of developing new therapies to increase the number of hearts available by preventing ischemic injury, which allows resuscitating and regenerating marginal hearts. The development of OCS®, a device for normothermic perfusion of hearts during transport has been a major step forward towards expanding the number of hearts available for transplant from DCD donors (18). It has also been used to expand the number of donor hearts available from DBD donors by resuscitating marginal hearts (Figure 1) (19). Ex vivo preservation models can also be used for the study of new therapies for the failing heart, which could translate to therapies for the treatment of individuals with heart failure. This would be a game changer for the entire field of heart failure.
Static cold storage has been the traditional heart preservation strategy and standard of care since the first heart transplant and is currently considered a reliable strategy for DBD hearts. It is simple, inexpensive, and able to preserve standard DBD hearts for 3–4 h with acceptable transplantation outcomes (20). But storage at 0°C has been found to be less effective than at slightly higher temperatures—called hypothermic storage. Paragonix has developed a controlled hypothermic static storage device called the Shepapak® (Figure 2) that has been approved by the FDA for the transportation of DBD hearts. The Sherpapak® device has been shown to reduce ischemic injury compared to SCS and extend the time for transport of the donor organ. In a pivotal trial, Paragonix has shown that the Sherpapak® can extend the time between explant and implant from the standard of 3 h to 6 h. This has led to a small increase in the number of hearts available for transplant.
Figure 2. The paragonix sherpapak® cardiac transport system. The heart is submerged in cold cardioplegic solution. The transport system uses proprietary phase change cold packs to maintain temperatures 4–8°C.
During static cold storage there is no source of oxygen or nutrients for the heart. Machine perfusion (MP) devices have been developed to address this issue. Perfusion can supply the metabolic needs of the myocardium, thus minimizing irreversible ischemic cell injury and death. For donor hearts, MP can provide a platform to resuscitate, preserve, assess and even possibly recondition the cardiac function prior to planned transplantation. New heart perfusion systems, which are either hypothermic MP (HMP) or normothermic MP (NMP), have been developed that show some success in preserving animal and/or human hearts (21). Systems are already in active clinical use with encouraging outcomes for both DCD and extended-criteria DBD donors (22). They are already altering clinical practice and facilitating the utilization of donor hearts once considered marginal (19).
Currently, one commercial perfusion system called the organ care system (OCS®) (Figure 3) is available for clinical use (23, 24). A prolonged safe preservation time allows for the utilization of remote donor hearts and functional assessment allows for the utilization of high-risk donor hearts.
Figure 3. This figure illustrates an organ care system (OCS®) heart machine with the schematic diagram, breaking down the processes involved.
One effective means of expanding the number of hearts available for transplantation is to expand the source by including DCD hearts. Because DCD hearts are more fragile than DBD hearts due to injury inherent in the causes for circulatory death and unavoidable ischemia during the period awaiting declaration of death, the SCS method is not considered the best preservation strategy for DCD hearts, as energy-depleted DCD hearts can hardly tolerate additional cold ischemia (25). In recent years, normothermic ex vivo heart perfusion (NMP) has become recognized as a novel strategy for DCD heart preservation. During organ storage NMP can supply donated hearts with oxygenated, warm, and nutrient-enriched blood-based perfusate in a semi-physiological state (26). Therefore, compared with static cold storage, NMP can attenuate myocardial ischemia/reperfusion injury (IRI) injury in DCD hearts, and be used to assess the contractile function of DCD hearts in real-time, prolong storage time, and potentially provide a unique platform for repairing DCD hearts. Moreover, compared with conventional DBD heart transplantation, DCD heart transplantation has provided a similar 30-day and 1-year postoperative survival rate (27, 28). Nevertheless, the inevitable warm ischemia time, which spans from the time systolic blood pressure falls below 50 mmHg after the withdrawal of life-sustaining therapy to the time of reperfusion or cardioplegia, results in more serious myocardial IRI in DCD hearts (29). This injury leads to a higher incidence of primary graft dysfunction (PGD) for the patients undergoing DCD heart transplantation (22, 30, 31).
Transmedics has developed the Organ Care System® (OCS®) (Figure 3), which utilizes normothermic perfusion of the beating heart with oxygenated whole blood. The system is similar to the system of cardiopulmonary bypass used during cardiovascular surgery when the heart is arrested. It has been approved by the FDA for DCD hearts. The use of this technology has increased the number of hearts available for transplant by extending the time for transportation and, therefore, the geographical range for procurement. Since the OCS® device received FDA approval in 2018 for use in transporting DCD hearts, the availability of hearts from DCD donors has steadily increased to 278 transplants in 2022 (32). The OCS® device has shown a one-month mortality rate comparable to that of DBD hearts using static cold storage. The effect on the rate of post-transplant graft dysfunction has not been investigated.
More recently Xvivo has developed a device that uses hypothermic perfusion with diluted whole blood in a system like the OCS® device (33). Neither of these devices eliminate injury from ischemia reperfusion, but they can extend the time from explant to implant and make available DCD hearts. Minimizing the ischemia reperfusion injury could increase the time between explant and implant and reduce the rate of post-transplant graft dysfunction and development of fibrosis, which would be of significant benefit. It is important, therefore, to look beyond mechanical strategies to reduce myocardial IRI in donor hearts.
To mitigate the adverse effects of IRI, a range of heart preservation solutions have been developed, each containing varying concentrations of cellular nutrients, metabolites, electrolytes, and antioxidants. Studies on optimizing perfusate contents and control of resuscitation parameters have produced results that can be used to improve preservation systems for donor hearts (15). The first preservation solution was the Euro Collins solution, formulated in 1960. It was followed by the University of Wisconsin (UW) solution in 1988 (23). Subsequently, due to several modifications to these solutions and the development of new ones, the number of solutions commercially available has grown to more than 150 (34, 35). Currently, the three solutions most used are the HTK solution (Perisoc, Khöler Chemie Pharmaceuticals, Germany), the UW solution (SPS-1, Poland), and the Celsior solution (Institut Georges Lopez, France).
With the advent of normothermic perfusion, it is now feasible to consider an approach to the ex vivo recovery or regeneration of diseased organs that combines the use of a machine perfusion system with one of these ex vivo therapeutic perfusion solutions. Using this approach with marginal donor hearts, in particular DCD hearts that have been rejected for transplantation, could further expand availability, especially for selected clinical indications (36). Currently, most studies investigating ex vivo supported organ reconditioning are executed on an experimental level. In the future, machine perfusion combined with ex vivo therapeutic perfusion may eventually allow the treatment of diseased hearts either in situ or ex vivo followed by autologous reimplantation thereby reducing the number of patients in need of organ transplantation (37–39).
Therapies such as standard cardioplegic solutions (40), antioxidants (41), and anti-inflammatory approaches (42) have been researched to optimize recovery and utilization of donor hearts. We present in section 3.8.1 below a novel therapeutic approach that uses peptide mimetics of BMP-7 to activate the BMP signaling pathway in order to inhibit inflammation, apoptosis, and fibrosis.
Ex vivo machine perfusion provides the opportunity to not only evaluate a donor heart for implantation but also offers the possibility of rehabilitating an injured donor heart. Following metabolic and biochemical markers in the perfusate is the primary, and non-invasive, mode of evaluation. For perspective on how the health of a heart is evaluated during ex vivo machine perfusion, we review here what happens at the cellular level during ischemia.
The lack of oxygen shuts down oxidative metabolism, the Krebs cycle, which takes place in the mitochondria. As long as glucose is present, glycolysis continues producing two ATP and two pyruvate molecules per molecule of glucose. But compared to the approximately 32 ATP effectively produced per molecule of glucose by the complete oxidative metabolic pathway, the absence of oxygen results in a major decrease in metabolic energy production. In the ischemic state with oxidative metabolism shut down, pyruvate produced by glycolysis cannot enter the blocked Krebs cycle. Without a means to remove the pyruvate, glycolysis would also be shut down and the two ATPs that it generates lost. Instead, pyruvate is converted to lactate and excreted from the cell. During SCS, the excreted lactate builds up in the interstitial spaces of the organ tissue and diffuses into the perfusate. Because lactate is in equilibrium with lactic acid, depending on the buffering capacity of the perfusate, increasing lactate concentration results in lowered pH and the danger of acidosis damage to the tissue (43). The CORI cycle, which is located primarily in the liver, is the metabolic pathway that converts lactate back to glucose, at the expense of 6 ATP. During oxidative machine perfusion of the heart, the lactate concentration in the perfusate will not decrease, due to the lack of tissue containing the CORI cycle, but neither should it increase due to resumed oxidative metabolism. Any increase is indicative of a source of continued ischemia and of tissue damage due to damaged mitochondria (44). Metabolic markers of improved heart health include the rate of O2 and glucose use—difference in O2 and glucose concentrations before and after the heart container—and the rate change of lactate concentration.
Under ischemic conditions or when glycogen and glucose concentrations are low, the heart switches to a different source of energy, metabolism of free fatty acids (45). Fatty acids must first be activated by conversion to thioesters, an O2 dependent process. Activated fatty acids are then converted to acetyl-CoA by beta-oxidation, a process that is not dependent on O2. Acetyl-CoA can directly enter the citric acid cycle to produce significant amounts of ATP. Under ischemic conditions where the citric acid cycle has shut down due to lack of O2, fatty acid metabolism ends with acetyl-CoA. In their review, Jaswal et al. (45) note that upon reperfusion “fatty acid β-oxidation also rapidly recovers, leading to an inhibition of pyruvate dehydrogenase and an increased production of lactate and protons” before oxidative metabolism in mitochondria has recovered.
Conditions or treatments affecting circulating free fatty acids may impair myocardial metabolism during hypoxia, ischemia, and reperfusion (46). Fatty acid oxidation is significantly reduced in the ischemic heart compared to a healthy heart due to the ischemic damage sustained during the warm ischemic period prior to procurement, leading to impaired mitochondrial function and reduced ability to utilize fatty acids as a primary energy source.
Upon initiation of ex vivo perfusion, a DCD heart will exhibit low rates of fatty acid oxidation due to the metabolic disruption caused by warm ischemia (47). As the perfusion progresses, a significant metabolic shift occurs where the heart switches from primarily utilizing fatty acids as an energy source to relying more heavily on glucose and lactate, a phenomenon often referred to as a “metabolic switch” due to the altered conditions of ischemia and reperfusion experienced by the DCD heart (48).
Other cellular reactions to ischemia are oxidative stress, inflammation, and apoptosis, which are evidenced by specific biochemical markers such as: HNE (oxidative stress); increased expression of TNF-α, IL-6, NF-κB, and MPO (inflammation); DNA strand breaks, increased expression of Bax, cleaved caspase-3, cytochrome C, and decreased expression of Bcl2 (apoptosis); and expression and secretion of cytokines, chemokines and adhesion molecules (pro-inflammatory markers). Upon organ implantation into a recipient, proinflammatory cytokines, such as IL-1 and TNF-α, and chemokines are produced within hours of reperfusion in grafts. Chemokines mediate early migration of neutrophils and macrophages into the graft (49, 50). Inflammatory activation of graft endothelium (51), platelets, the coagulation cascade, and the complement (52) play important roles in early graft injury and subsequent graft vasculopathy. This response is separate and distinct from immune rejection of the graft.
Ex vivo perfusion systems present a significant opportunity to serve as a development platform for the administration of therapeutic interventions on donor hearts without causing adverse effects on other donor or recipient organs. The central objective of these interventions is to mitigate the impact of IRI, with a particular focus on the potential to rejuvenate marginal organs for viable transplantation. Although these therapeutic applications are still in the preclinical phase and have not yet undergone clinical testing, their preliminary outcomes are promising. The prospective integration of ex vivo therapies has the potential to not only enhance graft viability, but also contributes to expanding the existing donor organ pool, a prospect that has significant potential for the field of transplantation.
Several potential therapies, such as standard cardioplegic solutions (40) antioxidants (41) and anti-inflammatory approach (42) have been studied to optimize recovery and utilization of donor hearts.
Following a cardiac ischemic event, key cellular effects that are targeted by current cell transplantation or therapeutic strategies include: mitochondrial dysfunction (53), oxidative stress (54), cell death pathways (apoptosis, necrosis, autophagy) (55), inflammation (56), and extracellular matrix remodeling/fibrosis (57), with a focus on restoring damaged cardiomyocytes and promoting angiogenesis to revascularize the affected area. Cardiomyocyte-like cells derived from embryonic stem cells or induced pluripotent stem cells (iPSCs): aim to replace damaged cardiomyocytes with functional ones (58). Mesenchymal stem cells (MSCs) possess paracrine effects, promoting angiogenesis, anti-inflammatory activity, and tissue repair (59). Endothelial progenitor cells can contribute to new blood vessel formation, improving blood flow to ischemic regions (60).
Cardiac regeneration and functional recovery after myocardial ischemic injury has been the focus of much study but achievement of this goal remains elusive (4, 61). In mammals, heart injuries such as those induced by myocardial ischemia and myocardial infarction (MI) result in substantial loss of cardiac muscle cells (cardiomyocytes), which are replaced by fibrotic scar tissues. This condition coupled with the very limited regenerative capacity of the adult mammalian heart often leads to heart failure (HF) (62, 63). Finding targets for the development of drugs or harnessing the potential of stem cells has fostered significant scientific endeavor and produced marked strides towards understanding the mediators of the processes involved in myocardial injury (64–69). A great deal of this effort has focused on using stem cells to regenerate cardiac tissue.
A promising therapeutic strategy for cardiac regeneration following myocardial ischemia and infarction is to initiate myocyte regeneration through the transplantation of stem cells. However, the major challenge for the development of such therapies is the limited survival and function of transplanted stem cells (70, 71). Two main cell types are currently under investigation in heart repair from ischemic injury. Mesenchymal stromal cells (MSCs) indirectly support endogenous regenerative capacities after transplantation. Induced pluripotent stem cell-derived cardiomyocytes (iPSC-CMs) directly contribute to the restoration of function by integrating into the damaged myocardium. Intramyocardial or intracoronary delivery of MSCs significantly reduced cardiac scar size after myocardial ischemia in a porcine model (72–74). Recent studies have shown that intravenously injected MSCs can improve myocardial IRI in a porcine model by preventing microvascular obstruction (75). Aggregates of iPSC-CMs, which are termed cardiac spheroids, have been developed to improve engraftment (76). The transplantation of iPSC-derived cardiac spheroids is safe and effective for improving cardiac function in rat and swine HF models (77).
Despite the promising outcomes of stem cell treatment against ischemic heart disease in previous experimental and clinical studies, current evidence supports neither the incorporation of the infused stem cells into the injured myocardium, nor their in vivo differentiation into functional myocytes (78, 79). The observed stem-cell-mediated benefits have been shown to be attributable to the paracrine functions of stem cells. When cardiomyocytes (CM) derived from human induced pluripotent stem cells (hiPSC) are injected in the cardiac tissue, human iPSC-CMs have longer action potential and lower cell-to-cell coupling than adult-like CMs (80). The electrophysiological properties of these immature iPSC-CMs generate electrophysiological gradients that favor arrhythmias (81, 82).
Recent evidence suggests that small extracellular vesicles, called exosomes, appear to play a key role, particularly because they contain microRNAs that have been found to have clinical potential in the treatment of ischemic heart disease (83–85). Although most studies refer to microRNAs as being responsible for the therapeutic role of extra cellular vesicles (EVs) or exosomes, proteins have been reported to contribute as well. For instance, cardiac progenitor cells (CPC)-EVs administrated in the heart 1 h after ligation reduced CD68 + macrophages in the treated rats one month after EV injection (81). Pregnancy-associated plasma protein-A (PAPP-A) was found to be responsible for the therapeutic effect. PAPP-A is a protease responsible for the cleavage of insulin growth factor-1 (IGF-1) binding protein-4, which transports IGF-1. Once released from its complex, IGF-1 can act as an immunomodulator in the heart (82).
Considering the limited availability of autologous stem cells, exosomes derived from their allogeneic and xenogeneic counterparts may provide therapeutic advantages (86). Nevertheless, there are several challenges and shortcomings, such as observed substantial heterogeneity among studies, no significant differences emerged in mortality and ventricular arrhythmia risk in iPSC-CM treatment vs. controls, that need to be abridged before stem cell therapy becomes a norm in clinical settings. A large clinical trial of transendocardial mesenchymal precursor cells in patients with heart failure did not reach statistical significance for its primary and secondary endpoints (87).
Recent animal studies have demonstrated that Normothermic EVHP (NMP) combined with conditioned medium treatment derived from bone marrow mesenchymal stem cells (BMSCs) can alleviate warm IRI in the DCD animal models (88). A 25-min warm ischemia injury significantly increased the level of oxidative stress (increased expression of HNE), inflammation (increased expression of TNF-α, IL-6, NF-κB, and MPO), and apoptosis (increased expression of Bax, cleaved caspase-3, Cytochrome C and DNA strand breaks, and decreased expression of Bcl2) in the DCD hearts. Compared with the DCD-control group, normothermic ex vivo heart perfusion combined with CM treatment decreased the level of oxidative stress (decreased expression of HNE), inflammation (decreased expression of TNF-α, IL-6, and NF-κB), and apoptosis (decreased expression of Bax, Cleaved caspase-3, Cytochrome c, and DNA strand breaks) in the DCD heart after ex vivo heart perfusion, which might alleviate the shortage of donor hearts by adopting DCD hearts (88).
Ex vivo perfusion systems can facilitate the delivery of therapeutic cells, such as stem cells or genetically modified cells, directly to the donor heart. These cells have the potential to promote tissue repair, regeneration, and immunomodulation, further enhancing the heart's viability, or for example, human multipotent stromal cells (89, 90). Recent studies have demonstrated that Normothermic EVHP combined with conditioned medium treatment derived from bone marrow mesenchymal stem cells (BMSCs)) can alleviate warm IRI in the animal models of DCD hearts. Although these strategies have shown great promise in preclinical studies, it is important to note that translating them into clinical practice requires rigorous testing and validation.
Gene transfer techniques can be utilized to introduce specific genes into the donor heart during ex vivo perfusion in animal models. These genes can encode protective factors or enzymes that counteract IRI processes. By isolating the heart in a metabolically and immunologically favorable environment and preventing off-target effects and dilution, it is possible to directly control factors that enhance the success rate of cardiac gene therapy. Gene therapy research during normothermic ex vivo heart perfusion has involved adenovirus mediated gene transfer (91–96) using rabbit or porcine hearts. One study investigated adeno-associated viral (AAV) mediated gene transfer in porcine hearts (97). The most important finding was the ability to achieve durable transgene expression using AAV-mediated gene transfer for up to 35 days following heterotopic transplantation, without signs of systemic off-target expression, rejection, or inflammation in the graft. To date, local immunomodulation and enhanced myocardial tolerance to ischemia-reperfusion injury have been achieved using gene transfer during ex vivo heart perfusion in rodent models (98). Future studies will need to focus on replicating these findings in large animal models and humans.
Manipulating the metabolic pathways within the donor heart during ex vivo perfusion could promote energy production and reduce the negative consequences of metabolic disruption during IRI. By restoring oxygenation and providing metabolic substrates, machine perfusion potentially allows for the correction of metabolic derangements caused by IRI (99).
Targeting specific candidates implicated in organ IRI is challenging due to the complex molecular pathways that are activated. Some of the activated pathways and molecules include the complement cascade, the innate immune response and toll-like receptors (TLRs), CD4T lymphocytes, inflammatory cytokines propagating the post-inflammatory response, nuclear factor κB (NF-κB) leading to production of TNF-α, adhesion molecules, apoptotic pathway activation, and reactive oxygen species (ROS) production and release (100, 101). Pig liver studies rely on using a combination of therapies that block multiple, perhaps redundant, ischemic/reperfusion injury pathways in order to achieve a significant reduction in injury and overall improvement in graft function (102). Various pharmacological agents, such as antioxidants, anti-inflammatory drugs, and vasodilators, have been administered to the donor heart during ex vivo perfusion in animal models. These agents aim to counteract oxidative stress, reduce inflammation, and improve vascular function, ultimately protecting the heart from IRI-related damage.
Various growth factors such as fibroblast growth factor 1 (FGF1) (103), vascular endothelial growth factor (VEGF) (104), bone morphogenetic protein-2 (BMP-2) (105), BMP-10 (106), and systemic factors like thyroid hormones (107) and glucocorticoids (108) have been shown to modulate dedifferentiation and proliferation of endogenous cardiomyocytes, thereby potentially modulating heart regeneration in adult mammals. In a recent study, Vukicevic (109) found that bone morphogenetic protein 1.3 (BMP1.3) levels were elevated in both patients and animal models of myocardial infarction. In a cardiac fibrosis animal model, treatment with a specific monoclonal antibody against BMP1.3 alleviated cardiac fibrosis, reduced collagen deposition and cross-linking, and was paralleled by enhanced cardiomyocyte survival both in vivo and in primary cultures of cardiac cells. Mechanistically, the monoclonal antibody against-BMP1.3 has the effect of inhibiting the TGF-beta pathway, thereby reducing myofibroblast activation and inducing cardio protection through BMP-5. BMP-7 administration stimulated mouse cardiomyocyte cycling at postnatal-day 7, when cardiomyocytes normally cease to proliferate, and enhanced cardiomyocyte regeneration with functional recovery in vivo in adult mice following myocardial infarction (110).
Anti-inflammatory agents can enhance the protective effect of ex vivo dynamic organ preservation by further curbing the inflammatory response during perfusion. In a recent study, a warm ischemic time of 25 min significantly up-regulated the levels of IL-6, TNF-α and NF-κB, an inflammatory response in the DCD heart submitted to EVHP, while treatment with melatonin (N-acetyl-5-methoxytryptamine) led to a significant decrease in the expression of IL-6, TNF-α, and NF-κB in the DCD heart (111).
Myocardial apoptosis involves both the death domain receptor and mitochondrial pathways (Figure 4). Both pathways converge on caspase activation (112). The death receptor pathway is extrinsic, involving cell surface receptors. The mitochondrial pathway is intrinsic, using mitochondria and the endoplasmic reticulum. Evidence indicates that the death receptor and mitochondrial pathways are not isolated systems. Instead, significant crosstalk and “biofeedback” regulates the apoptotic machinery (113). Interception of the apoptosis pathway in porcine hearts using hypothermic perfusion solutions infused with small interfering RNA molecules targeting key apoptotic and inflammatory enzymes has had notable outcomes in diminishing cellular apoptosis and mitigating myocyte injury. Furthermore, this approach has resulted in enhancement of donor myocardial function (114, 115). In a distinct porcine transplantation model, the introduction of oxygen-derived free radical scavengers into the perfusion process has shown the potential to enhance graft functionality while concurrently ameliorating cellular edema (116).
Figure 4. The death receptor and mitochondrial pathways of apoptosis: DNA damage triggers the death receptor and mitochondrial apoptotic pathways. Death ligands that bind to their respective receptors trigger the recruitment of adaptor molecules (FADD), and subsequent recruitment and activation of caspases to mediate apoptosis. Activated caspases can also cleave BID to promote the mitochondrial pathway of apoptosis. This pathway is defined by mitochondrial outer membrane permeabilization (MOMP) and is regulated by the BCL-2 proteins. Pro-apoptotic proteins such as BID or BIM promote BAX or BAK homo-oligomerization in the mitochondrial membrane, whereas anti-apoptotic proteins such as BCL-2 and BCL-xL inhibit this process. De-repressor proteins BAD, PUMA or NOXA bind the anti-apoptotic proteins and reduce the threshold for BAX/BAK activation. MOMP results in cytochrome c release into the cytosol, which promotes APAF-1 oligomerization, caspase activation, and apoptosis.
Yet another preclinical investigation unveiled the efficacy of a therapeutic regimen that couples two inhibitors of the necrosis pathway, resulting in a discernible reduction in IRI among rat cardiomyocytes (114, 117). Therapies that foster angiogenesis have also garnered attention. Examples encompass interventions involving the inclusion of vascular endothelial growth factor (VEGF) (118) and prokinectin receptor-1 (89). These interventions have manifested the capacity to elevate the survival rate of cardiomyocytes subjected to ischemia.
Melatonin, which is mainly synthesized by the pineal gland in mammals, is regarded as an antioxidant (89), anti-inflammatory (119), and anti-apoptotic (120) molecule. It has been shown that the combination of normothermic EVHP and melatonin post-conditioning could be a novel and promising donor heart preservation strategy, which could ameliorate myocardial IRI and improve cardiac function of DCD hearts, thereby increasing the number of transplantable hearts in heart transplantation (111). Given that inflammation and apoptosis lead to the development of fibrosis, Hosseinzadeh et al. (121) have proposed that melatonin could also protect against fibrosis. Below we describe a growth factor mimetic that has been shown to protect against the development of fibrosis and to reverse fibrosis (122).
Several other therapies have been investigated for the treatment of ischemia-reperfusion and advanced decompensated heart failure (123). Most of these therapies targeted pathways downstream in the pathologic processes. As such, they affect only a single arm of a multifaceted process and are therefore limited in their beneficial effects. By looking upstream to targets that have broader effects, we have discovered agents that are safe and block the multifaceted cardiac cellular injury (124). Our target is a bone morphogenic growth factor (BMP) that belongs to the TGF beta superfamily.
BMP-7 signaling exerts important actions on fibroblasts, cardiomyocytes and macrophages. Anti-fibrotic effects of BMP-7 have been reported in many systems and may be mediated, at least in part, through suppression of collagen synthesis by cardiac fibroblasts (125) and through inhibition of endothelial to mesenchymal transition (EndMT) (126). In cardiomyocytes, BMP-7/signaling attenuates hypertrophy by inhibiting TGF-β responses (125). In macrophages, BMP-7 has been reported to promote M2 polarization (127). Considering the absence of endogenous BMP-7 induction in infarcted and remodeling hearts, administration of exogenous BMP-7 has been suggested as a potential strategy to attenuate fibrosis and adverse remodeling.
We have targeted a growth factor that belongs to the TGF beta superfamily, a developmental pathway critical to the formation of organs during embryogenesis. Bone morphogenic protein 7 (BMP-7) is involved in embryogenesis, development and the maintenance of adult tissue homeostasis, and is a potent antagonist of TGF beta action (128–130). It can block TGF beta-induced inflammation and apoptosis, and block/reverse TGF beta-induced fibrosis (126, 131).
The transforming growth factor β (TGF-β) superfamily consists of a large group of pleiotropic cytokines, including TGF-βs, activins, and bone morphogenetic proteins (BMPs), which are critically involved in embryogenesis, development and the maintenance of adult tissue homeostasis. Alterations in BMP signaling pathways often result in severe human diseases (132, 133) including cardiovascular diseases (134). BMP canonical signaling in target cells involves type I and type II receptors and the formation of tetrameric receptor complexes. BMPs preferentially bind type I receptors and recruit type II receptors. The constitutively active type II receptor trans-phosphorylates and activates the type I receptor upon forming the heterotetrameric receptor complex. Activated BMP type I receptors (BMPR-I), often referred to as activin-like kinases (ALKs), have serine/threonine kinase activity. Their canonical cell effectors are the phosphorylated SMAD1/5/8/9 transcription factors (132, 135).
There are also three type II receptors involved in BMP signaling: BMPR-II and activin type II receptors ActR-IIA and ActR-IIB (136–138) while TGF β interacts with the type I receptor (ALK5) and type II receptor (TGFR-II). Activin binds type I receptors ActR-IA (ALK2) and ActR-1B (ALK4) and type II receptors ActR-IIA and ActR-IIB.
BMP-7 binds BMPR-IA (ALK3), BMPR-IB (ALK6), and the activin A receptor type 1 ACVR-I (ALK2) (Figure 5). BMP activates both canonical and non-canonical pathways (Figure 6). In the canonical pathway, it activates BMPR-II, which leads to phosphorylation of Smad-1/5/8, which then complexes with Smad-4 and transmits the signal. In the signaling pathway involving XIAP, TAK1, and TAB1, the receptor complex is typically the first to be activated upon ligand binding, and then it triggers the downstream activation of the TAB1-TAK1 complex, with CIAP playing a role in modulating this activation by interacting with TAB1, not being directly activated by the receptor itself (141, 142).
Figure 5. The TGF beta/BMP/activin pathways are mediated by their specific type I and type II receptors. BMP binds any of three type I receptors: BMPR-IA (ALK3), BMPR-IB (ALK6) and a Type IA activin receptor ActR-IA (ALK2) (138–140).
Figure 6. BMP signaling pathways. BMP mimetic/BMP-7 transduces signals in target cells by binding to a specific membrane bound receptor BMPR-II and phosphorylates BMPR-I, which activates both the canonical and the non-canonical pathways. In the canonical pathway, activated BMPR-II leads to phosphorylation of Smad-1/5/8 which complexes with Smad-4 and translocate the signal. In the non-canonical pathway, p38, Mitogen-activated protein kinase (MAPK), c-Jun-N terminal Kinase (JNK), ERK and NFKB were activated via the activation of X-linked inhibitor of apoptosis protein (XIAP), TGF-beta activated kinase 1 (TAK1) and TAK1 binding protein (TAB1) whereas PI3K/Akt were activated by both BMPR-II and Smad-1/5/8. Altogether, this influences the different transcription factors and regulates the gene expression.
Altogether, this activation influences the different transcription factors and regulates the gene expression. Notably, several reports demonstrate the involvement of BMPR-IA mediated signaling in the protective role of BMP-7 against renal fibrosis (126, 143).
Recent preclinical investigations have demonstrated that endogenous and exogenous rhBMP-7 protect the myocardium against maladaptive phenotypic plasticity in experimental models of clinically relevant heart diseases, which strongly suggests the cardioprotective therapeutic potential of BMP-7-based approaches (144–148).
The cell-type differential bioactivity of BMPs is dependent on the receptors expressed (149). Therefore, whether BMPR-IA signaling may be involved in the cardioprotective effects of BMP-7 was explored. It was observed that BMPR-IA (BMP type 1 receptor a, ALK3) was the most abundantly expressed receptor in the healthy LV myocardium in humans and mice; on the other hand, ACVR1 (Activin type I receptor) was scarcely expressed, and BMPR-IB (BMP type 1 receptor b, ALK6) was virtually absent. The expression of Smad proteins in infarcted or fibrotic myocardium is different from that in the normal myocardium. No matter whether it is assessed in early or late-stage cardiomyopathy, the selective expression of Smad proteins is correlated with cardiac fibrosis and elevated collagen synthesis levels (150, 151). The expression of Smad 2, 3 and 4 is upregulated at the infarct scar as well as in the peri-ischemic border zone (151) and is closely correlated with increased collagen type I expression (152). Crosstalk exists between BMP and TGF-β in their regulation of different signaling pathways. BMP receptor-ligand (e.g., BMP-7) binding can activate Smad1/5/8 signaling and induce the expression of inhibitors of differentiation 2 and 3 (ID2 and ID3). ID2 and ID3 can prevent Smad2/3 phosphorylation and thus counteract TGF-β/Smad signaling (153).
The BMPs have some potentially beneficial therapeutic effects on cardiac development and anti-apoptosis by acting on the Smad proteins. BMP-2 may have therapeutic potential for curing chronic myocardial ischemia by improving the contractility of cardiomyocytes and preventing cardiomyocyte cell death (105). Injection of BMP-2 reduces the infarct size in mice in a left anterior descending artery ligation model. Mice treated with BMP-2 are characterized by reduced cardiomyocyte apoptosis rates. BMP-7 gene therapy limited pathological remodeling in the diabetic heart, conferring an improvement in cardiac function (154). The clinical use of rhBMP-7 in tissue-engineered products has been approved by US and EU agencies to induce localized osteogenesis in orthopedic and maxillofacial applications (155).
The important step in developing a BMP-7 mimetic is first and foremost that of separating out the osteogenic activity that has been shown to lead to the formation of ectopic bone when treating soft tissue injury. Furthermore, the whole BMP-7 protein is subject to inhibition by natural inhibitory proteins such as follistatin, chordin and gremlin (156). A mimetic would likely be immune to this inhibition. Small peptide mimetics are also less likely than biologics to induce an immune response. Peptide mimetics of 20 residues or less are synthesized chemically, which is a much simpler, cheaper, and reliable form of manufacturing than fermentation, the primary method for producing biologics. Any potential therapeutic benefit of restoring BMP-7 function using systemic rhBMP-7 is hampered by bioavailability, induction of neutralizing autoantibodies against BMPs, and a range of potential adverse effects (157).
There have been several publications describing efforts to design and test BMP mimetics (see Table 1). Most of these efforts have found compounds that are osteoinductive and, therefore, have limited applications to the treatment of diseases affecting soft tissues such as the heart, the lung, the liver, the kidney, the pancreas, or cancers (159–161). One compound has been found that is effective in cell-based assays indicating it could be used to treat pulmonary hypertension (162). The compound described is not a true mimetic since it cannot act alone, but rather enhances the effects of BMP-9. Other efforts to find BMP pathway agonists using high throughput screening have had limited success (163–166).
In recent years, studies have advanced our knowledge of the cellular and systemic functions of the BMPs. Therapeutics derived from BMPs have been developed for the treatment of diseases such as cardiovascular and kidney diseases (149, 167–169). The identification of BMP mimetics or BMP agonists remains an attractive strategy (see Table 1). Peptides that are designed from the knuckle region (Figure 7C) of BMP-2, BMP-7 and BMP-9 have been found to display osteogenic activity like the corresponding BMP molecule (159–161, 170). Peptides designed based on the BMP-9 knuckle region have been shown to induce differentiation of murine preosteoblasts (MC3T3-E1 cells) and induce cholinergic differentiation in human SH-SY5Y neuroblastoma cells (171, 172). Furthermore, peptides from the prodomain of BMP-7, namely BFP-1/2/3, have also been shown to induce stronger alkaline phosphatase activity in multipotent bone marrow stromal cells (MBSCs) (173–175). In addition, a BMP-2 knuckle peptide has been shown to possess similar bone inducing activity in vivo but did not induce the side effects observed with BMP-2 (176).
Figure 7. Structure diagrams of the BMP-7 monomer and the region covered by the mimetic. (A) Ribbon diagram showing the secondary structure of the BMP monomer, which contains three structural regions: antiparallel beta sheets of “Finger 1” (with the large terminal loop), “Finger 2” (with the tight beta-turn), and the “Heel” alpha helix. Initial targets for mimetic development were the terminal loops of fingers 1 and 2, loops at the C-terminal, and N-terminal loops at the ends of the heel helix. (B) The region around the beta turn of finger 2 proved to have activity similar to BMP-7 and became the lead for further mimetic development. (C) The “beta-turn” region covered by the mimetic is immediately C-terminal to the “knuckle” region covered by other BMP mimetics. Residue position numbers are based on BMP-2 residue numbers. Secondary structure: beta sheet (>>>>), segments of which are labelled e.g., “b6”; beta turn (bt, tttt). Peptide disulfide bond: C C.
BMP mimetic peptides have the potential for improving specificity. For example, peptide P3 designed from the “wrist area” of BMP-9 enhanced BMP-9-induced Smad1/5 phosphorylation selectively in human pulmonary artery endothelial cells (hPAECs) but inhibited BMP-4-induced Smad1/5 phosphorylation in human dermal microvascular endothelial cells (HMEC-1) (164).
Although these findings are exciting, very few studies have performed in-depth binding and mechanistic investigation of how these peptides achieve their specificity.
As explained in Carlson et al. (177), the design of BMP-7 mimetic peptides THR-123 and THR-184 used the x-ray structure of BMP-7 (178) to identify solvent accessible regions likely involved in receptor binding, then identified and optimized the best leads based on in vitro assays. These peptides, which are anti-inflammatory, anti-apoptotic and anti-fibrotic, cover the tight beta-turn region of finger 2 (Figure 7A). The fact that they display no osteogenic activity and do not bind to BMPR-IB (ALK6) correlates with the fact that osteogenic peptides identified to date (159–161, 170–172) cover the physically separate “knuckle” region (Figure 7C), and provides a physical explanation for the specificity of activity (Figure 8).
Figure 8. THR-123 and THR-184 binding like BMP-7, BMP mimetics THR-123 and THR-184 bind the BMP type II receptor BMPR-II and selective BMP type I receptors actR-IA (ALK2) and BMPR-IA (ALK3). Unlike BMP-7, they do not bind BMPR-IB (ALK6).
The knuckle region covered by the osteogenic peptides and the beta-turn region covered by the non-osteogenic mimetic peptides described here are separate structural regions (see Figure 7C). This separation allows small mimetic peptides to be designed with specific activity. A cyclized BMP-7 derived peptide, THR-123, located in the beta-turn that is C-terminal to the “knuckle” region (see Figure 7B), was reported to successfully reverse established kidney fibrosis in mouse models of acute and chronic renal injury (149).
THR-123 selectively binds the type I receptors ALK2 and ALK3 but does not bind ALK6. (Figure 8). It exhibits robust anti-inflammatory, anti-apoptotic and antifibrotic and regenerative effects in several experimental models of acute and chronic kidney diseases (149). More recently Li et al. (120) have shown that THR-123, effectively induced nongenetic conversion of human pancreatic exocrine cells to insulin-expressing and functional—glucose-responsive—endocrine cells with a capacity for rapid reversal of diabetes in vivo (158). This work independently demonstrates a safer and simpler alternative to genetic reprogramming. Unlike BMP-7, BMP mimetic peptide THR-123 does not induce ectopic bone formation (177).
THR-184, another mimetic peptide of BMP-7 was also designed and optimized. The receptor binding and in vitro assay activity—half maximal effective concentrations (EC50)—of the lead peptide variants were used for structure/activity analysis to arrive at THR-184 as the prime clinical candidate. Both BMP mimetics (THR-123 and THR-184) selectively bind the BMP type I receptors ActR-IA (ALK2) and BMPR-IA (ALK3) and type II receptor BMPR-II. They do not bind BMPR-IB (ALK6) (Figure 8).
THR-184 was evaluated in clinical studies of Acute Kidney injury (NIH, ClinicalTrials.gov Identifier: NCT01830920) and found to be safe and well tolerated. There was a noticeable reduction in the incidence of AKI in the patient subgroup with pre-existing CKD treated with the highest dose of THR-184 (177). In a type 1 diabetes rat model where islet cells are damaged by Tacrolimus, THR-123 regenerates the islet cells and eliminates the diabetic condition (158). Recently, in mice subjected to transverse aortic constriction (TAC), Salido-Medina et al. (122) established the cardioprotective effects of the two BMP-7 mimetics: THR-123 and THR-184. Daily i.p. injection with either peptide during four weeks, starting on the day of TAC surgery, (i) rescued the expression of BMPR-IA (ALK3) and associated pSMAD1/5/(8)9 signaling in the LV, (ii) prevented transcriptional activation of remodeling-associated genes (Col 1a1, β-MHC, BNP and Acta-2), (iii) attenuated LV structural damage (hypertrophy and fibrosis), and (iv) diminished LV dysfunction (systolic and diastolic). We have shown in a rat left anterior descending artery ligation model that treatment with THR-123 protects cardiomyocytes, and limits infarct size after myocardial ischemic and reperfusion injuries (124). Based on the promise of THR-184, we are currently evaluating its application to rescue DCD hearts from IRI in a rodent model (179). The combination of a perfusate containing THR-184 and normothermic EVHP treatment is a novel and promising donor heart preservation strategy to ameliorate myocardial IRI and to improve cardiac function in the DCD hearts, thereby increasing the number of transplantable grafts in heart transplantation.
The supply-demand imbalance of heart allografts available for transplantation continuously grows as more end-stage patients are listed for heart transplantation. Until recently there has been little progress in increasing the supply of hearts (29, 180). The development of Normothermic ex vivo machine perfusion (NEVMP), which (despite its limitations), keeps the heart in a near physiological state is a breakthrough that has expanded the availability of donor heart allogeafts. Coupled with other therapeutic modalities to preserve and regenerate the heart, it could lead to new therapies for patients with advanced heart failure and potentially reduce the number of patients in need of a heart transplant.
NEVMP offers protection from ischemia during transportation and allows for evaluation of organs from DCD donors, which are at a higher risk due to the unavoidable warm ischemic conditions involved during determination of donor death. NEVMP allows repeated perfusate sample monitoring of changes in biomarker release, making possible more precise analysis of inflammatory and injury markers such as TNF alpha, IL-6, IL-8 and IL-10 for graft evaluation. Furthermore, this technique permits timely biomarker evaluation to fit within the limited time of heart transplantation protocols. Several biomarkers, such as troponins and lactate, are rapidly and routinely measured in clinical practice and could be implemented without difficulty. The combined use of several biomarker measurements would likely provide a more robust assessment of myocardial injury. Current imaging modalities could also be used to evaluate cardiac grafts, permitting detailed graft characterization. A high-resolution CT scan can detect myocardial fibrosis with good reliability and sensitivity (181). The contrast-enhanced cardiac computed tomography (CCT) was recently demonstrated to be a potentially accurate alternative to Cardiac Magnetic Resonance for the identification of myocardial fibrosis (182). Of particular relevance is a real-time and non-invasive method of assessing graft function and injury based on mitochondrial oxygenation of heart surface tissue using Resonance Raman Spectroscopy (183). This provides a means of predicting the oxygenated state of mitochondrial cytochrome C during perfusion, possibly enabling the assessment of apoptosis. Moreover, ex vivo systems present a significant opportunity for the administration of therapeutic interventions to donor hearts. To this end, several new therapeutics have been explored for their efficacy in alleviating cardiac IRI. We designed a series of novel BMP mimetic small peptides which exhibit robust anti-inflammatory, anti-apoptotic and anti-fibrotic and regenerative effects to protect cardiomyocytes and alleviate cardiac IRI in vivo. These compounds improve cardiac function and metabolic parameters. Our lead BMP mimetic, THR-184 has been tested clinically and found to be safe and well tolerated by patients in clinical trials. It could quickly enter IND status for the ex vivo preservation of DCD hearts.
Ex vivo normothermic perfusion combined with new therapeutics such as the BMP mimetics is a novel approach that could overcome the major risk of cardiac ischemic injury and provide more favorable organ utilization and potentially expand the donor pool for heart transplantation. Studies of new therapeutic treatments for the preservation and recovery of donor hearts for transplant will likely serve as a path forward for the development of compounds for the treatment of a broader spectrum of patients with heart failure.
Heart Disease is the leading cause of death in the USA. In 2018, there were 6.2 million US adults that had heart failure. In 2022, 702,880 people died from heart disease. In 2019–20 the cost of heart failure/disease to US society was estimated to be $252.2 billion (https://www.cdc.gov/heart-disease/data-research/facts-stats/index.html#cdcreference_3). The only definitive treatment for heart failure is cardiac transplantation.
The development of ex vivo heart prefusion technologies has significantly increased the number and quality of donor hearts available for transplantation and offers a potential means for rehabilitating hearts that would otherwise be rejected. Ischemia is an unavoidable effect of current heart procurement protocols, which leads to Ischemia/reperfusion injury to the transplanted heart and other organs of the recipient and continues to limit the potential of this technology.
This paper presents a therapeutic extension of the current and projected near-term techniques used to preserve the heart for transplantation. Success with this therapy could extend the time between explant and implant from hours to days or possibly weeks and dramatically increase the number of donor hearts acceptable for transplantation (currently less than 40%).
The therapeutic we employ is a growth factor mimetic that has been shown to be effective in animal models at protecting and repairing cardiomyocytes damaged by ischemia/reperfusion injury. Repairing this injury means we can treat heart failure and other organs damaged during pre- and post-transplant.
The original contributions presented in the study are included in the article/Supplementary Material, further inquiries can be directed to the corresponding author.
WC: Conceptualization, Data curation, Formal Analysis, Funding acquisition, Investigation, Methodology, Project administration, Resources, Software, Supervision, Validation, Visualization, Writing – original draft, Writing – review & editing. DB: Conceptualization, Data curation, Writing – original draft, Writing – review & editing. PK: Conceptualization, Writing – original draft, Writing – review & editing. FC: Conceptualization, Writing – original draft, Writing – review & editing. ST: Writing – original draft, Writing – review & editing. PB: Writing – review & editing.
The author(s) declare that no financial support was received for the research and/or publication of this article.
The authors declare that the research was conducted in the absence of any commercial or financial relationships that could be construed as a potential conflict of interest.
The author(s) declared that they were an editorial board member of Frontiers, at the time of submission. This had no impact on the peer review process and the final decision.
The author(s) declare that no Generative AI was used in the creation of this manuscript.
All claims expressed in this article are solely those of the authors and do not necessarily represent those of their affiliated organizations, or those of the publisher, the editors and the reviewers. Any product that may be evaluated in this article, or claim that may be made by its manufacturer, is not guaranteed or endorsed by the publisher.
1. Siddiqi TJ, Khan Minhas AM, Greene SJ, Van Spall HGC, Khan SS, Pandey A, et al. Trends in heart failure-related mortality among older adults in the United States from 1999 to 2019. JACC Heart Fail. (2022) 10(11):851–9. doi: 10.1016/j.jchf.2022.06.012
2. Martin SS, Aday AW, Almarzooq ZI, Anderson CAM, Arora P, Avery CL, et al. 2024 heart disease and stroke statistics: a report of US and global data from the American Heart Association. Circulation. (2024) 149(8):e347–913. doi: 10.1161/CIR.0000000000001209
3. Tsao CW, Aday AW, Almarzooq ZI, Anderson CAM, Arora P, Avery CL, et al. Heart disease and stroke statistics-2023 update: a report from the American Heart Association. Circulation. (2023) 147(8):e93–621.doi: doi: 10.1161/CIR.0000000000001123
4. Bertero A, Murry CE. Hallmarks of cardiac regeneration. Nat Rev Cardiol. (2018) 15(10):579–80. doi: 10.1038/s41569-018-0079-8
5. Hoffman ED Jr, Klees BS, Curtis CA. Overview of the medicare and medicaid programs. Health Care Financ Rev. (2000) 22(1):175–93.25372783
6. Maltes S, Rocha BML, Cunha GJL, Brizido C, Strong C, Tralhao A, et al. Challenges of organ shortage for heart transplant: surviving amidst the chaos of long waiting times. Transplant Direct. (2021) 7(3):e671. doi: 10.1097/TXD.0000000000001122
7. Messer S, Page A, Rushton S, Berman M, Tsui S, Catarino P, et al. The potential of heart transplantation from donation after circulatory death donors within the United Kingdom. J Heart Lung Transplant. (2019) 38(8):872–4. doi: 10.1016/j.healun.2019.04.007
8. Banner NR, Rogers CA, Bonser RS, Group UKCTAS. Effect of heart transplantation on survival in ambulatory and decompensated heart failure. Transplantation. (2008) 86(11):1515–22. doi: 10.1097/TP.0b013e31818b3328
9. Russo MJ, Chen JM, Sorabella RA, Martens TP, Garrido M, Davies RR, et al. The effect of ischemic time on survival after heart transplantation varies by donor age: an analysis of the united network for organ sharing database. J Thorac Cardiovasc Surg. (2007) 133(2):554–9. doi: 10.1016/j.jtcvs.2006.09.019
10. Valero-Masa MJ, Gonzalez-Vilchez F, Almenar-Bonet L, Crespo-Leiro MG, Manito-Lorite N, Sobrino-Marquez JM, et al. Cold ischemia >4 hours increases heart transplantation mortality. An analysis of the Spanish heart transplantation registry. Int J Cardiol. (2020) 319:14–9. doi: 10.1016/j.ijcard.2020.06.009
11. Kilic A, Emani S, Sai-Sudhakar CB, Higgins RS, Whitson BA. Donor selection in heart transplantation. J Thorac Dis. (2014) 6(8):1097–104. doi: 10.3978/j.issn.2072-1439.2014.03.23
12. Fernández AR, Sánchez-Tarjuelo R, Cravedi P, Ochando J, López-Hoyos M. Review: ischemia reperfusion injury-a translational perspective in organ transplantation. Int J Mol Sci. (2020) 21:22. doi: 10.3390/ijms21228549
13. Wong ASK, Sin SWC. Short-term mechanical circulatory support (intra-aortic balloon pump, impella, extracorporeal membrane oxygenation, TandemHeart): a review. Ann Transl Med. (2020) 8(13):829. doi: 10.21037/atm-20-2171
14. Møller JE, Thiele H, Morrow D, Kjærgaard J, Hassager C. Mechanical circulatory support: when, how, and for whom. Eur Heart J. (2025). doi: 10.1093/eurheartj/ehae925
15. Burrage MK, Cheshire C, Hey CY, Azam S, Watson WD, Bhagra S, et al. Comparing cardiac mechanics and myocardial fibrosis in DBD and DCD heart transplant recipients. J Card Fail. (2023) 29(5):834–40. doi: 10.1016/j.cardfail.2022.11.014
16. Hughes A, Okasha O, Farzaneh-Far A, Kazmirczak F, Nijjar PS, Velangi P, et al. Myocardial fibrosis and prognosis in heart transplant recipients. Circ Cardiovasc Imaging. (2019) 12(10):e009060. doi: 10.1161/CIRCIMAGING.119.009060
17. Sun W, Shen X, Wang J, Zhu S, Zhang Y, Wu C, et al. Association between 2D- and 3D-speckle-tracking longitudinal strain and cardiovascular magnetic resonance evidence of diffuse myocardial fibrosis in heart transplant recipients. Front Cardiovasc Med. (2021) 8:727745. doi: 10.3389/fcvm.2021.727745
18. Schroder JN, Patel CB, DeVore AD, Bryner BS, Casalinova S, Shah A, et al. Transplantation outcomes with donor hearts after circulatory death. N Engl J Med. (2023) 388(23):2121–31. doi: 10.1056/NEJMoa2212438
19. Schroder JN, Patel CB, DeVore AD, Casalinova S, Koomalsingh KJ, Shah AS, et al. Increasing utilization of extended criteria donor hearts for transplantation: the OCS heart EXPAND trial. JACC Heart Fail. (2024) 12(3):438–47. doi: 10.1016/j.jchf.2023.11.015
20. Dhital KK, Chew HC, Macdonald PS. Donation after circulatory death heart transplantation. Curr Opin Organ Transplant. (2017) 22(3):189–97. doi: 10.1097/mot.0000000000000419
21. Chew HC, Macdonald PS, Dhital KK. The donor heart and organ perfusion technology. J Thorac Dis. (2019) 11(Suppl 6):S938–45. doi: 10.21037/jtd.2019.02.59
22. Bryner BS, Schroder JN, Milano CA. Heart transplant advances: ex vivo organ-preservation systems. JTCVS Open. (2021) 8:123–7. doi: 10.1016/j.xjon.2021.04.020
23. Mühlbacher F, Langer F, Mittermayer C. Preservation solutions for transplantation. Transplant Proc. (1999) 31(5):2069–70. doi: 10.1016/s0041-1345(99)00265-1
24. Nilsson J, Jernryd V, Qin G, Paskevicius A, Metzsch C, Sjöberg T, et al. A nonrandomized open-label phase 2 trial of nonischemic heart preservation for human heart transplantation. Nat Commun. (2020) 11(1):2976. doi: 10.1038/s41467-020-16782-9
25. Niederberger P, Farine E, Raillard M, Dornbierer M, Freed DH, Large SR, et al. Heart transplantation with donation after circulatory death. Circ Heart Fail. (2019) 12(4):e005517. doi: 10.1161/CIRCHEARTFAILURE.118.005517
26. Lund LH, Khush KK, Cherikh WS, Goldfarb S, Kucheryavaya AY, Levvey BJ, et al. The registry of the international society for heart and lung transplantation: thirty-fourth adult heart transplantation report-2017; focus theme: allograft ischemic time. J Heart Lung Transplant. (2017) 36(10):1037–46. doi: 10.1016/j.healun.2017.07.019
27. Messer S, Cernic S, Page A, Berman M, Kaul P, Colah S, et al. A 5-year single-center early experience of heart transplantation from donation after circulatory-determined death donors. J Heart Lung Transplant. (2020) 39(12):1463–75. doi: 10.1016/j.healun.2020.10.001
28. Messer S, Page A, Axell R, Berman M, Hernández-Sánchez J, Colah S, et al. Outcome after heart transplantation from donation after circulatory-determined death donors. J Heart Lung Transplant. (2017) 36(12):1311–8. doi: 10.1016/j.healun.2017.10.021
29. D’Alessandro D, Schroder J, Meyer DM, Vidic A, Shudo Y, Silvestry S, et al. Impact of controlled hypothermic preservation on outcomes following heart transplantation. J Heart Lung Transplant. (2024) 43(7):1153–61. doi: 10.1016/j.healun.2024.03.010
30. Bracey NA, Maltzman JS. CD8T(regs) SQa-1shing transplant rejection. Sci Immunol. (2023) 8(90):eadn0644. doi: 10.1126/sciimmunol.adn0644
31. Burchill LJ, Ross HJ. Heart transplantation in adults with end-stage congenital heart disease. Future Cardiol. (2012) 8(2):329–42. doi: 10.2217/fca.12.11
32. Alomari M, Garg P, Yazji JH, Wadiwala IJ, Alamouti-Fard E, Hussain MWA, et al. Is the organ care system (OCS) still the first choice with emerging new strategies for donation after circulatory death (DCD) in heart transplant? Cureus. (2022) 14(6):e26281. doi: 10.7759/cureus.26281
33. McGiffin DC, Kure CE, Macdonald PS, Jansz PC, Emmanuel S, Marasco SF, et al. Hypothermic oxygenated perfusion (HOPE) safely and effectively extends acceptable donor heart preservation times: results of the Australian and New Zealand trial. J Heart Lung Transplant. (2024) 43(3):485–95. doi: 10.1016/j.healun.2023.10.020
34. Demmy TL, Biddle JS, Bennett LE, Walls JT, Schmaltz RA, Curtis JJ. Organ preservation solutions in heart transplantation–patterns of usage and related survival. Transplantation. (1997) 63(2):262–9. doi: 10.1097/00007890-199701270-00015
35. Minasian SM, Galagudza MM, Dmitriev YV, Karpov AA, Vlasov TD. Preservation of the donor heart: from basic science to clinical studies. Interact Cardiovasc Thorac Surg. (2014) 20(4):510–9. doi: 10.1093/icvts/ivu432
36. Resch T, Cardini B, Oberhuber R, Weissenbacher A, Dumfarth J, Krapf C, et al. Transplanting marginal organs in the era of modern machine perfusion and advanced organ monitoring. Front Immunol. (2020) 11:631. doi: 10.3389/fimmu.2020.00631
37. MacConmara M, Feizpour CA, Shubin A, Vagefi PA. The role of machine perfusion in liver xenotransplantation. Curr Opin Organ Transplant. (2020) 25(5):477–82. doi: 10.1097/MOT.0000000000000799
38. Markmann JF, Vagefi PA, MacConmara MP. Normothermic machine perfusion increases donor liver use. JAMA Surg. (2022) 157(8):742–3. doi: 10.1001/jamasurg.2022.1424
39. Zulpaite R, Miknevicius P, Leber B, Strupas K, Stiegler P, Schemmer P. Kidney machine perfusion: therapeutic potential. Front Med (Lausanne). (2021) 8:808719. doi: 10.3389/fmed.2021.808719
40. Osorio-Llanes E, Castellar-López J, Rosales-Rada W, Montoya Y, Bustamante J, Zalaquett R, et al. Novel strategies to improve the cardioprotective effects of cardioplegia. Curr Cardiol Rev. (2024) 20(1):e240124226064. doi: 10.2174/011573403X263956231129064455
41. Shi S, Xue F. Current antioxidant treatments in organ transplantation. Oxid Med Cell Longev. (2016) 2016:8678510. doi: 10.1155/2016/8678510
42. Saemann L, Pohl S, Wächter K, Georgevici AI, Köhler C, Jünger J, et al. Cytokine adsorption during ex vivo blood perfusion improves contractility of donation after circulatory death hearts. J Am Heart Assoc. (2024) 13(23):e036872. doi: 10.1161/jaha.124.036872
43. Karwatowska-Prokopczuk E, Nordberg JA, Li HL, Engler RL, Gottlieb RA. Effect of vacuolar proton ATPase on pHi, Ca2+, and apoptosis in neonatal cardiomyocytes during metabolic inhibition/recovery. Circ Res. (1998) 82(11):1139–44. doi: 10.1161/01.res.82.11.1139
44. Li X, Yang Y, Zhang B, Lin X, Fu X, An Y, et al. Correction: lactate metabolism in human health and disease. Signal Transduct Target Ther. (2022) 7(1):372. doi: 10.1038/s41392-022-01206-5
45. Jaswal JS, Keung W, Wang W, Ussher JR, Lopaschuk GD. Targeting fatty acid and carbohydrate oxidation–a novel therapeutic intervention in the ischemic and failing heart. Biochim Biophys Acta. (2011) 1813(7):1333–50. doi: 10.1016/j.bbamcr.2011.01.015
46. Oliver MF. Fatty acids and the risk of death during acute myocardial ischaemia. Clin Sci (Lond). (2015) 128(6):349–55. doi: 10.1042/cs20140404
47. Arnold M, Do P, Davidson SM, Large SR, Helmer A, Beer G, et al. Metabolic considerations in direct procurement and perfusion protocols with DCD heart transplantation. Int J Mol Sci. (2024) 25:8. doi: 10.3390/ijms25084153
48. Trimigno A, Zhao J, Michaud WA, Paneitz DC, Chukwudi CD, Alessandro DA, et al. Metabolic choreography of energy substrates during DCD heart perfusion. Transplant Direct. (2024) 10(9):e1704. doi: 10.1097/txd.0000000000001704
49. Ishii D, Schenk AD, Baba S, Fairchild RL. Role of TNFalpha in early chemokine production and leukocyte infiltration into heart allografts. Am J Transplant. (2010) 10(1):59–68. doi: 10.1111/j.1600-6143.2009.02921.x
50. Jordan JE, Zhao ZQ, Vinten-Johansen J. The role of neutrophils in myocardial ischemia-reperfusion injury. Cardiovasc Res. (1999) 43(4):860–78. doi: 10.1016/s0008-6363(99)00187-x
51. Mikalsen B, Fosby B, Wang J, Hammarstrom C, Bjaerke H, Lundstrom M, et al. Genome-wide transcription profile of endothelial cells after cardiac transplantation in the rat. Am J Transplant. (2010) 10(7):1534–44. doi: 10.1111/j.1600-6143.2010.03157.x
52. Diepenhorst GM, van Gulik TM, Hack CE. Complement-mediated ischemia-reperfusion injury: lessons learned from animal and clinical studies. Ann Surg. (2009) 249(6):889–99. doi: 10.1097/SLA.0b013e3181a38f45
53. Lesnefsky EJ, Chen Q, Tandler B, Hoppel CL. Mitochondrial dysfunction and myocardial ischemia-reperfusion: implications for novel therapies. Annu Rev Pharmacol Toxicol. (2017) 57:535–65. doi: 10.1146/annurev-pharmtox-010715-103335
54. van der Pol A, van Gilst WH, Voors AA, van der Meer P. Treating oxidative stress in heart failure: past, present and future. Eur J Heart Fail. (2019) 21(4):425–35. doi: 10.1002/ejhf.1320
55. Wu S, Ding D, Wang D. Regulated cell death pathways in pathological cardiac hypertrophy. Rev Cardiovasc Med. (2024) 25(10):366. doi: 10.31083/j.rcm2510366
56. Saxena A, Russo I, Frangogiannis NG. Inflammation as a therapeutic target in myocardial infarction: learning from past failures to meet future challenges. Transl Res. (2016) 167(1):152–66. doi: 10.1016/j.trsl.2015.07.002
57. Frangogiannis NG. The extracellular matrix in myocardial injury, repair, and remodeling. J Clin Invest. (2017) 127(5):1600–12. doi: 10.1172/jci87491
58. Yan W, Xia Y, Zhao H, Xu X, Ma X, Tao L. Stem cell-based therapy in cardiac repair after myocardial infarction: promise, challenges, and future directions. J Mol Cell Cardiol. (2024) 188:1–14. doi: 10.1016/j.yjmcc.2023.12.009
59. Poomani MS, Mariappan I, Perumal R, Regurajan R, Muthan K, Subramanian V. Mesenchymal stem cell (MSCs) therapy for ischemic heart disease: a promising frontier. Glob Heart. (2022) 17(1):19. doi: 10.5334/gh.1098
60. Kawamoto A, Losordo DW. Endothelial progenitor cells for cardiovascular regeneration. Trends Cardiovasc Med. (2008) 18(1):33–7. doi: 10.1016/j.tcm.2007.11.004
61. Libby P, Bornfeldt KE, Tall AR. Atherosclerosis: successes, surprises, and future challenges. Circ Res. (2016) 118(4):531–4. doi: 10.1161/circresaha.116.308334
62. Benjamin T, Schumacher C. Sodium-glucose cotransporter-2 inhibitors and urinary tract infections. Ann Intern Med. (2019) 171(12):944. doi: 10.7326/L19-0669
63. Bongiovanni C, Sacchi F, Da Pra S, Pantano E, Miano C, Morelli MB, D’Uva G. Reawakening the intrinsic cardiac regenerative potential: molecular strategies to boost dedifferentiation and proliferation of endogenous cardiomyocytes. Front Cardiovasc Med. (2021) 8:750604. doi: 10.3389/fcvm.2021.750604
64. Bargehr J, Ong LP, Colzani M, Davaapil H, Hofsteen P, Bhandari S, et al. Epicardial cells derived from human embryonic stem cells augment cardiomyocyte-driven heart regeneration. Nat Biotechnol. (2019) 37(8):895–906. doi: 10.1038/s41587-019-0197-9
65. Coulombe KL, Bajpai VK, Andreadis ST, Murry CE. Heart regeneration with engineered myocardial tissue. Annu Rev Biomed Eng. (2014) 16:1–28. doi: 10.1146/annurev-bioeng-071812-152344
66. Efe JA, Hilcove S, Kim J, Zhou H, Ouyang K, Wang G, et al. Conversion of mouse fibroblasts into cardiomyocytes using a direct reprogramming strategy. Nat Cell Biol. (2011) 13(3):215–22. doi: 10.1038/ncb2164
67. Ieda M, Fu JD, Delgado-Olguin P, Vedantham V, Hayashi Y, Bruneau BG, et al. Direct reprogramming of fibroblasts into functional cardiomyocytes by defined factors. Cell. (2010) 142(3):375–86. doi: 10.1016/j.cell.2010.07.002
68. Mummery C. Extraordinary challenge, extraordinary achievement: 2020–2021 year in review. Stem Cell Rep. (2021) 16(6):1391–3. doi: 10.1016/j.stemcr.2021.05.015
69. Zhang J, Bolli R, Garry DJ, Marbán E, Menasché P, Zimmermann WH, et al. Basic and translational research in cardiac repair and regeneration: JACC state-of-the-art review. J Am Coll Cardiol. (2021) 78(21):2092–105. doi: 10.1016/j.jacc.2021.09.019
70. Hare JM, DiFede DL, Rieger AC, Florea V, Landin AM, El-Khorazaty J, et al. Randomized comparison of allogeneic versus autologous mesenchymal stem cells for nonischemic dilated cardiomyopathy: POSEIDON-DCM trial. J Am Coll Cardiol. (2017) 69(5):526–37. doi: 10.1016/j.jacc.2016.11.009
71. Hare JM, Fishman JE, Gerstenblith G, DiFede Velazquez DL, Zambrano JP, Suncion VY, et al. Comparison of allogeneic vs. autologous bone marrow–derived mesenchymal stem cells delivered by transendocardial injection in patients with ischemic cardiomyopathy: the POSEIDON randomized trial. JAMA. (2012) 308(22):2369–79. doi: 10.1001/jama.2012.25321
72. de Jong R, van Hout GP, Houtgraaf JH, Kazemi K, Wallrapp C, Lewis A, et al. Intracoronary infusion of encapsulated glucagon-like peptide-1-eluting mesenchymal stem cells preserves left ventricular function in a porcine model of acute myocardial infarction. Circ Cardiovasc Interv. (2014) 7(5):673–83. doi: 10.1161/circinterventions.114.001580
73. Hu X, Xu Y, Zhong Z, Wu Y, Zhao J, Wang Y, et al. A large-scale investigation of hypoxia-preconditioned allogeneic mesenchymal stem cells for myocardial repair in nonhuman primates: paracrine activity without remuscularization. Circ Res. (2016) 118(6):970–83. doi: 10.1161/circresaha.115.307516
74. Liu CB, Huang H, Sun P, Ma SZ, Liu AH, Xue J, et al. Human umbilical cord-derived mesenchymal stromal cells improve left ventricular function, perfusion, and remodeling in a porcine model of chronic myocardial ischemia. Stem Cells Transl Med. (2016) 5(8):1004–13. doi: 10.5966/sctm.2015-0298
75. Wang J, Chen Z, Dai Q, Zhao J, Wei Z, Hu J, et al. Intravenously delivered mesenchymal stem cells prevent microvascular obstruction formation after myocardial ischemia/reperfusion injury. Basic Res Cardiol. (2020) 115(4):40. doi: 10.1007/s00395-020-0800-8
76. Tabei R, Kawaguchi S, Kanazawa H, Tohyama S, Hirano A, Handa N, et al. Development of a transplant injection device for optimal distribution and retention of human induced pluripotent stem cell‒derived cardiomyocytes. J Heart Lung Transplant. (2019) 38(2):203–14. doi: 10.1016/j.healun.2018.11.002
77. Kawaguchi S, Soma Y, Nakajima K, Kanazawa H, Tohyama S, Tabei R, et al. Intramyocardial transplantation of human iPS cell-derived cardiac spheroids improves cardiac function in heart failure animals. JACC Basic Transl Sci. (2021) 6(3):239–54. doi: 10.1016/j.jacbts.2020.11.017
78. Cai CL, Molkentin JD. The elusive progenitor cell in cardiac regeneration: slip slidin’ away. Circ Res. (2017) 120(2):400–6. doi: 10.1161/circresaha.116.309710
79. Lee SH, Hong JH, Cho KH, Noh JW, Cho HJ. Discrepancy between short-term and long-term effects of bone marrow-derived cell therapy in acute myocardial infarction: a systematic review and meta-analysis. Stem Cell Res Ther. (2016) 7(1):153. doi: 10.1186/s13287-016-0415-z
80. Rosales RM, Mountris KA, Oliván-Viguera A, Pérez-Zabalza M, Cedillo-Servin G, Iglesias-García O, et al. Experimentally-guided in silico design of engineered heart tissues to improve cardiac electrical function after myocardial infarction. Comput Biol Med. (2024) 171:108044. doi: 10.1016/j.compbiomed.2024.108044
81. Barile L, Cervio E, Lionetti V, Milano G, Ciullo A, Biemmi V, et al. Cardioprotection by cardiac progenitor cell-secreted exosomes: role of pregnancy-associated plasma protein-A. Cardiovasc Res. (2018) 114(7):992–1005. doi: 10.1093/cvr/cvy055
82. Santini MP, Lexow J, Borsellino G, Slonimski E, Zarrinpashneh E, Poggioli T, et al. IGF-1Ea induces vessel formation after injury and mediates bone marrow and heart cross-talk through the expression of specific cytokines. Biochem Biophys Res Commun. (2011) 410(2):201–7. doi: 10.1016/j.bbrc.2011.05.081
83. Mani S, Gurusamy N, Ulaganathan T, Paluck AJ, Ramalingam S, Rajasingh J. Therapeutic potentials of stem cell-derived exosomes in cardiovascular diseases. Exp Biol Med (Maywood). (2023) 248(5):434–44. doi: 10.1177/15353702231151960
84. Yin X, Lin L, Fang F, Zhang B, Shen C. Mechanisms and optimization strategies of paracrine exosomes from mesenchymal stem cells in ischemic heart disease. Stem Cells Int. (2023) 2023:6500831. doi: 10.1155/2023/6500831
85. Zhang Z, Zou Y, Song C, Cao K, Cai K, Chen S, et al. Advances in the study of exosomes in cardiovascular diseases. J Adv Res. (2024) 66:133–53. doi: 10.1016/j.jare.2023.12.014
86. Sareen N, Srivastava A, Alagarsamy KN, Lionetti V, Dhingra S. Stem cells derived exosomes and biomaterials to modulate autophagy and mend broken hearts. Biochim Biophys Acta Mol Basis Dis. (2023) 1869(7):166806. doi: 10.1016/j.bbadis.2023.166806
87. Perin EC, Borow KM, Henry TD, Mendelsohn FO, Miller LW, Swiggum E, et al. Randomized trial of targeted transendocardial mesenchymal precursor cell therapy in patients with heart failure. J Am Coll Cardiol. (2023) 81(9):849–63. doi: 10.1016/j.jacc.2022.11.061
88. Zeng Z, Xu L, Xu Y, Ruan Y, Liu D, Li J, et al. Normothermic ex vivo heart perfusion with mesenchymal stem cell-derived conditioned Medium improves myocardial tissue protection in rat donation after circulatory death hearts. Stem Cells Int. (2022) 2022(1):8513812. doi: 10.1155/2022/8513812
89. Arslan F, Lai RC, Smeets MB, Akeroyd L, Choo A, Aguor EN, et al. Mesenchymal stem cell-derived exosomes increase ATP levels, decrease oxidative stress and activate PI3K/Akt pathway to enhance myocardial viability and prevent adverse remodeling after myocardial ischemia/reperfusion injury. Stem Cell Res. (2013) 10(3):301–12. doi: 10.1016/j.scr.2013.01.002
90. Hung SC, Pochampally RR, Chen SC, Hsu SC, Prockop DJ. Angiogenic effects of human multipotent stromal cell conditioned medium activate the PI3K-akt pathway in hypoxic endothelial cells to inhibit apoptosis, increase survival, and stimulate angiogenesis. Stem Cells. (2007) 25(9):2363–70. doi: 10.1634/stemcells.2006-0686
91. Bishawi M, Roan JN, Milano CA, Daneshmand MA, Schroder JN, Chiang Y, et al. A normothermic ex vivo organ perfusion delivery method for cardiac transplantation gene therapy. Sci Rep. (2019) 9(1):8029. doi: 10.1038/s41598-019-43737-y
92. Donahue JK, Kikkawa K, Johns DC, Marban E, Lawrence JH. Ultrarapid, highly efficient viral gene transfer to the heart. Proc Natl Acad Sci U S A. (1997) 94(9):4664–8. doi: 10.1073/pnas.94.9.4664
93. Donahue JK, Kikkawa K, Thomas AD, Marban E, Lawrence JH. Acceleration of widespread adenoviral gene transfer to intact rabbit hearts by coronary perfusion with low calcium and serotonin. Gene Ther. (1998) 5(5):630–4. doi: 10.1038/sj.gt.3300649
94. Lehnart SE, Donahue JK. Coronary perfusion cocktails for in vivo gene transfer. Methods Mol Biol. (2003) 219:213–8. doi: 10.1385/1-59259-350-x:213
95. Lehnart SE, Janssen PM, Franz WM, Donahue JK, Lawrence JH, Marbán E, et al. Preservation of myocardial function after adenoviral gene transfer in isolated myocardium. Am J Physiol Heart Circ Physiol. (2000) 279(3):H986–991. doi: 10.1152/ajpheart.2000.279.3.H986
96. Nagata K, Marbán E, Lawrence JH, Donahue JK. Phosphodiesterase inhibitor-mediated potentiation of adenovirus delivery to myocardium. J Mol Cell Cardiol. (2001) 33(3):575–80. doi: 10.1006/jmcc.2000.1322
97. Pla M, Chiang M, Roki Y, Wang A, Lee C, Smith FH, et al. Gene delivery to porcine cardiac allografts using a myocardial-enhanced adeno-associated viral vector. Hum Gene Ther. (2023) 34(7–8):303–13. doi: 10.1089/hum.2022.241
98. Pellegrini C, Jeppsson A, Taner CB, O’Brien T, Miller VM, Tazelaar HD, et al. Highly efficient ex vivo gene transfer to the transplanted heart by means of hypothermic perfusion with a low dose of adenoviral vector. J Thorac Cardiovasc Surg. (2000) 119(3):493–500. doi: 10.1016/s0022-5223(00)70128-0
99. Aceros H, Der Sarkissian S, Borie M, Pinto Ribeiro RV, Maltais S, Stevens LM, et al. Novel heat shock protein 90 inhibitor improves cardiac recovery in a rodent model of donation after circulatory death. J Thorac Cardiovasc Surg. (2022) 163(2):e187–97. doi: 10.1016/j.jtcvs.2020.03.042
100. Konishi T, Lentsch AB. Hepatic ischemia/reperfusion: mechanisms of tissue injury, repair, and regeneration. Gene Expr. (2017) 17(4):277–87. doi: 10.3727/105221617X15042750874156
101. Luedde T, Assmus U, Wüstefeld T, Meyer zu Vilsendorf A, Roskams T, Schmidt-Supprian M, et al. Deletion of IKK2 in hepatocytes does not sensitize these cells to TNF-induced apoptosis but protects from ischemia/reperfusion injury. J Clin Invest. (2005) 115(4):849–59. doi: 10.1172/JCI23493
102. Goldaracena N, Echeverri J, Spetzler VN, Kaths JM, Barbas AS, Louis KS, et al. Anti-inflammatory signaling during ex vivo liver perfusion improves the preservation of pig liver grafts before transplantation. Liver Transpl. (2016) 22(11):1573–83. doi: 10.1002/lt.24603
103. Engel FB, Hsieh PC, Lee RT, Keating MT. FGF1/p38 MAP kinase inhibitor therapy induces cardiomyocyte mitosis, reduces scarring, and rescues function after myocardial infarction. Proc Natl Acad Sci U S A. (2006) 103(42):15546–51. doi: 10.1073/pnas.0607382103
104. Tao Z, Chen B, Tan X, Zhao Y, Wang L, Zhu T, et al. Coexpression of VEGF and angiopoietin-1 promotes angiogenesis and cardiomyocyte proliferation reduces apoptosis in porcine myocardial infarction (MI) heart. Proc Natl Acad Sci USA. (2011) 108(5):2064–9. doi:doi: 10.1073/pnas.1018925108
105. Ebelt H, Hillebrand I, Arlt S, Zhang Y, Kostin S, Neuhaus H, et al. Treatment with bone morphogenetic protein 2 limits infarct size after myocardial infarction in mice. Shock. (2013) 39(4):353–60. doi: 10.1097/SHK.0b013e318289728a
106. Sun L, Yu J, Qi S, Hao Y, Liu Y, Li Z. Bone morphogenetic protein-10 induces cardiomyocyte proliferation and improves cardiac function after myocardial infarction. J Cell Biochem. (2014) 115(11):1868–76. doi: 10.1002/jcb.24856
107. Hirose K, Payumo AY, Cutie S, Hoang A, Zhang H, Guyot R, et al. Evidence for hormonal control of heart regenerative capacity during endothermy acquisition. Science. (2019) 364(6436):184–8. doi: 10.1126/science.aar2038
108. Pianca N, Sacchi F, Umansky KB, Chirivì M, Iommarini L, Da Pra S, et al. Glucocorticoid receptor antagonization propels endogenous cardiomyocyte proliferation and cardiac regeneration. Nat Cardiovasc Res. (2022) 1(7): 617–33. doi: 10.1038/s44161-022-00090-0
109. Vukicevic S, Colliva A, Kufner V, Martinelli V, Moimas S, Vodret S, et al. Bone morphogenetic protein 1.3 inhibition decreases scar formation and supports cardiomyocyte survival after myocardial infarction. Nat Commun. (2022) 13(1):81. doi: 10.1038/s41467-021-27622-9
110. Bongiovanni C, Bueno-Levy H, Posadas Pena D, Del Bono I, Miano C, Boriati S, et al. BMP7 promotes cardiomyocyte regeneration in zebrafish and adult mice. Cell Rep. (2024) 43(5):114162. doi: 10.1016/j.celrep.2024.114162
111. Lu J, Xu L, Zeng Z, Xue C, Li J, Chen X, et al. Normothermic ex vivo heart perfusion combined with melatonin enhances myocardial protection in rat donation after circulatory death hearts via inhibiting NLRP3 inflammasome-mediated pyroptosis. Front Cell Dev Biol. (2021) 9:733183. doi: 10.3389/fcell.2021.733183
112. Crow MT, Mani K, Nam YJ, Kitsis RN. The mitochondrial death pathway and cardiac myocyte apoptosis. Circ Res. (2004) 95(10):957–70. doi: 10.1161/01.RES.0000148632.35500.d9
113. Reed JC. Mechanisms of apoptosis. Am J Pathol. (2000) 157(5):1415–30. doi: 10.1016/s0002-9440(10)64779-7
114. Kung G, Konstantinidis K, Kitsis RN. Programmed necrosis, not apoptosis, in the heart. Circ Res. (2011) 108(8):1017–36. doi: 10.1161/CIRCRESAHA.110.225730
115. Wei J, Chen S, Xue S, Zhu Q, Liu S, Cui L, et al. Blockade of inflammation and apoptosis pathways by siRNA prolongs cold preservation time and protects donor hearts in a porcine model. Mol Ther Nucleic Acids. (2017) 9:428–39. doi: 10.1016/j.omtn.2017.10.020
116. Qiao S, Zhao WJ, Li HQ, Ao GZ, An JZ, Wang C, et al. Necrostatin-1 analog DIMO exerts cardioprotective effect against ischemia reperfusion injury by suppressing necroptosis via autophagic pathway in rats. Pharmacology. (2021) 106(3-4):189–201. doi: 10.1159/000510864
117. Smith CC, Davidson SM, Lim SY, Simpkin JC, Hothersall JS, Yellon DM. Necrostatin: a potentially novel cardioprotective agent? Cardiovasc Drugs Ther. (2007) 21(4):227–33. doi: 10.1007/s10557-007-6035-1
118. Ruixing Y, Dezhai Y, Hai W, Kai H, Xianghong W, Yuming C. Intramyocardial injection of vascular endothelial growth factor gene improves cardiac performance and inhibits cardiomyocyte apoptosis. Eur J Heart Fail. (2007) 9(4):343–51. doi: 10.1016/j.ejheart.2006.10.007
119. Agil A, Reiter RJ, Jiménez-Aranda A, Ibán-Arias R, Navarro-Alarcón M, Marchal JA, et al. Melatonin ameliorates low-grade inflammation and oxidative stress in young zucker diabetic fatty rats. J Pineal Res. (2013) 54(4):381–8. doi: 10.1111/jpi.12012
120. Li Y, Yang Y, Feng Y, Yan J, Fan C, Jiang S, et al. A review of melatonin in hepatic ischemia/reperfusion injury and clinical liver disease. Ann Med. (2014) 46(7):503–11. doi: 10.3109/07853890.2014.934275
121. Hosseinzadeh A, Pourhanifeh MH, Amiri S, Sheibani M, Irilouzadian R, Reiter RJ, et al. Therapeutic potential of melatonin in targeting molecular pathways of organ fibrosis. Pharmacol Rep. (2024) 76(1):25–50. doi: 10.1007/s43440-023-00554-5
122. Salido-Medina AB, Gil A, Expósito V, Martínez F, Redondo JM, Hurlé MA, et al. BMP7-based peptide agonists of BMPR1A protect the left ventricle against pathological remodeling induced by pressure overload. Biomed Pharmacother. (2022) 149:112910. doi: 10.1016/j.biopha.2022.112910
123. Rico F, Konate C, Josse K, Nargeot E, Barrère-Lemaire J, Boisguérin S. Therapeutic peptides to treat myocardial ischemia-reperfusion injury. Front Cardiovasc Med. (2022) 9:792885. doi: 10.3389/fcvm.2022.792885
124. Subramanian R, Keck PC, Bosukonda D, Carlson FR, Carlson WD. A novel BMP-mimetic inhibits cardiomyocyte apoptosis and inflammation, and protects heart from acute myocardial infarction in rats. Endocr Rev. (2005) 26:3.
125. Merino D, Villar AV, García R, Tramullas M, Ruiz L, Ribas C, et al. BMP-7 attenuates left ventricular remodelling under pressure overload and facilitates reverse remodelling and functional recovery. Cardiovasc Res. (2016) 110(3):331–45. doi: 10.1093/cvr/cvw076
126. Zeisberg EM, Tarnavski O, Zeisberg M, Dorfman AL, McMullen JR, Gustafsson E, et al. Endothelial-to-mesenchymal transition contributes to cardiac fibrosis. Nat Med. (2007) 13(8):952–61. doi: 10.1038/nm1613
127. Urbina P, Singla DK. BMP-7 attenuates adverse cardiac remodeling mediated through M2 macrophages in prediabetic cardiomyopathy. Am J Physiol Heart Circ Physiol. (2014) 307(5):H762–72. doi: 10.1152/ajpheart.00367.2014
128. Bujak M, Frangogiannis NG. The role of TGF-beta signaling in myocardial infarction and cardiac remodeling. Cardiovasc Res. (2007) 74(2):184–95. doi: 10.1016/j.cardiores.2006.10.002
129. Hanna A, Frangogiannis NG. The role of the TGF-β superfamily in myocardial infarction. Front Cardiovasc Med. (2019) 6:140. doi: 10.3389/fcvm.2019.00140
130. Kreisberg RA, Oberman A. Medical management of hyperlipidemia/dyslipidemia. J Clin Endocrinol Metab. (2003) 88(6):2445–61. doi: 10.1210/jc.2003-030388
131. Towbin JA. Scarring in the heart–a reversible phenomenon? N Engl J Med. (2007) 357(17):1767–8. doi: 10.1056/NEJMcibr075397
132. David CJ, Massagué J. Contextual determinants of TGFβ action in development, immunity and cancer. Nat Rev Mol Cell Biol. (2018) 19(7):419–35. doi: 10.1038/s41580-018-0007-0
133. Lowery JW, Rosen V. Bone morphogenetic protein-based therapeutic approaches. Cold Spring Harb Perspect Biol. (2018) 10:4. doi: 10.1101/cshperspect.a022327
134. Luo JY, Zhang Y, Wang L, Huang Y. Regulators and effectors of bone morphogenetic protein signalling in the cardiovascular system. J Physiol. (2015) 593(14):2995–3011. doi: 10.1113/JP270207
135. Dituri F, Cossu C, Mancarella S, Giannelli G. The interactivity between TGFβ and BMP signaling in organogenesis, fibrosis, and cancer. Cells. (2019) 8(10):1130. doi: 10.3390/cells8101130
136. Miyazono K, Maeda S, Imamura T. BMP Receptor signaling: transcriptional targets, regulation of signals, and signaling cross-talk. Cytokine Growth Factor Rev. (2005) 16(3):251–63. doi: 10.1016/j.cytogfr.2005.01.009
137. Rosenzweig BL, Imamura T, Okadome T, Cox GN, Yamashita H, ten Dijke P, et al. Cloning and characterization of a human type II receptor for bone morphogenetic proteins. Proc Natl Acad Sci U S A. (1995) 92(17):7632–6. doi: 10.1073/pnas.92.17.7632
138. ten Dijke P, Yamashita H, Sampath TK, Reddi AH, Estevez M, Riddle DL, et al. Identification of type I receptors for osteogenic protein-1 and bone morphogenetic protein-4. J Biol Chem. (1994) 269(25):16985–8. doi: 10.1016/S0021-9258(17)32506-1
139. Koenig BB, Cook JS, Wolsing DH, Ting J, Tiesman JP, Correa PE, et al. Characterization and cloning of a receptor for BMP-2 and BMP-4 from NIH 3T3 cells. Mol Cell Biol. (1994) 14(9):5961–74. doi: 10.1128/mcb.14.9.5961
140. Macías-Silva M, Hoodless PA, Tang SJ, Buchwald M, Wrana JL. Specific activation of Smad1 signaling pathways by the BMP7 type I receptor, ALK2. J Biol Chem. (1998) 273(40):25628–36. doi: 10.1074/jbc.273.40.25628
141. Hirata Y, Takahashi M, Morishita T, Noguchi T, Matsuzawa A. Post-Translational modifications of the TAK1-TAB Complex. Int J Mol Sci. (2017) 18(1):205. doi: 10.3390/ijms18010205
142. Zsiros V, Dóczi N, Petővári G, Pop A, Erdei Z, Sebestyén A, Kiss AL. BMP-induced non-canonical signaling is upregulated during autophagy-mediated regeneration in inflamed mesothelial cells. Sci Rep. (2023) 13(1):10426. doi: 10.1038/s41598-023-37453-x
143. Budi EH, Schaub JR, Decaris M, Turner S, Derynck R. TGF-β as a driver of fibrosis: physiological roles and therapeutic opportunities. J Pathol. (2021) 254(4):358–73. doi: 10.1002/path.5680
144. Frangogiannis NG. Cardiac fibrosis: cell biological mechanisms, molecular pathways and therapeutic opportunities. Mol Aspects Med. (2019) 65:70–99. doi: 10.1016/j.mam.2018.07.001
145. Koitabashi N, Danner T, Zaiman AL, Pinto YM, Rowell J, Mankowski J, et al. Pivotal role of cardiomyocyte TGF-β signaling in the murine pathological response to sustained pressure overload. J Clin Invest. (2011) 121(6):2301–12. doi: 10.1172/JCI44824
146. Villar AV, Cobo M, Llano M, Montalvo C, González-Vílchez F, Martín-Durán R, et al. Plasma levels of transforming growth factor-beta1 reflect left ventricular remodeling in aortic stenosis. PLoS One. (2009) 4(12):e8476. doi: 10.1371/journal.pone.0008476
147. Villar AV, García R, Llano M, Cobo M, Merino D, Lantero A, et al. BAMBI (BMP and activin membrane-bound inhibitor) protects the murine heart from pressure-overload biomechanical stress by restraining TGF-β signaling. Biochim Biophys Acta. (2013) 1832(2):323–35. doi: 10.1016/j.bbadis.2012.11.007
148. Zeisberg M, Hanai J, Sugimoto H, Mammoto T, Charytan D, Strutz F, et al. BMP-7 counteracts TGF-beta1-induced epithelial-to-mesenchymal transition and reverses chronic renal injury. Nat Med. (2003) 9(7):964–8. doi: 10.1038/nm888
149. Sugimoto H, LeBleu VS, Bosukonda D, Keck P, Taduri G, Bechtel W, et al. Activin-like kinase 3 is important for kidney regeneration and reversal of fibrosis. Nat Med. (2012) 18(3):396–404. doi: 10.1038/nm.2629
150. Dixon IM, Hao J, Reid NL, Roth JC. Effect of chronic AT(1) receptor blockade on cardiac smad overexpression in hereditary cardiomyopathic hamsters. Cardiovasc Res. (2000) 46(2):286–97. doi: 10.1016/s0008-6363(00)00035-3
151. Hao J, Ju H, Zhao S, Junaid A, Scammell-La Fleur T, Dixon IM. Elevation of expression of smads 2, 3, and 4, decorin and TGF-beta in the chronic phase of myocardial infarct scar healing. J Mol Cell Cardiol. (1999) 31(3):667–78. doi: 10.1006/jmcc.1998.0902
152. Hao J, Wang B, Jones SC, Jassal DS, Dixon IM. Interaction between angiotensin II and smad proteins in fibroblasts in failing heart and in vitro. Am J Physiol Heart Circ Physiol. (2000) 279(6):H3020–3030. doi: 10.1152/ajpheart.2000.279.6.H3020
153. Weiskirchen R, Meurer SK. BMP-7 counteracting TGF-beta1 activities in organ fibrosis. Front Biosci (Landmark Ed). (2013) 18(4):1407–34. doi: 10.2741/4189
154. Tate M, Perera N, Prakoso D, Willis AM, Deo M, Oseghale O, et al. Bone morphogenetic protein 7 gene delivery improves cardiac structure and function in a murine model of diabetic cardiomyopathy. Front Pharmacol. (2021) 12:719290. doi: 10.3389/fphar.2021.719290
155. Tampe B, Tampe D, Nyamsuren G, Klöpper F, Rapp G, Kauffels A, et al. Pharmacological induction of hypoxia-inducible transcription factor ARNT attenuates chronic kidney failure. J Clin Invest. (2018) 128(7):3053–70. doi: 10.1172/JCI89632
156. Narasimhulu CA, Singla DK. The role of bone morphogenetic protein 7 (BMP-7) in inflammation in heart diseases. Cells. (2020) 9:2. doi: 10.3390/cells9020280
157. Schuette A, Moghaddam A, Seemann P, Duda GN, Schmidmaier G, Schomburg L. Treatment with recombinant human bone morphogenetic protein 7 leads to a transient induction of neutralizing autoantibodies in a subset of patients. BBA Clin. (2016) 6:100–7. doi: 10.1016/j.bbacli.2016.08.001
158. Qadir MMF, Álvarez-Cubela S, Klein D, van Dijk J, Muñiz-Anquela R, Moreno-Hernández YB, et al. Single-cell resolution analysis of the human pancreatic ductal progenitor cell niche. Proc Natl Acad Sci U S A. (2020) 117(20):10876–87. doi: 10.1073/pnas.1918314117
159. Kirkwood K, Rheude B, Kim YJ, White K, Dee KC. In vitro mineralization studies with substrate-immobilized bone morphogenetic protein peptides. Journal of Oral Implantology. (2003) 29(2):57–65. doi: 10.1563/1548-1336(2003)029%3C0057:IVMSWS%3E2.3.CO;2
160. Saito A, Suzuki Y, Ogata S, Ohtsuki C, Tanihara M. Activation of osteo-progenitor cells by a novel synthetic peptide derived from the bone morphogenetic protein-2 knuckle epitope. Biochim Biophys Acta. (2003) 1651(1–2):60–7. doi: 10.1016/s1570-9639(03)00235-8
161. Suzuki Y, Tanihara M, Suzuki K, Saitou A, Sufan W, Nishimura Y. Alginate hydrogel linked with synthetic oligopeptide derived from BMP-2 allows ectopic osteoinduction in vivo. J Biomed Mater Res. (2000) 50(3):405–9. doi: 10.1002/(SICI)1097-4636(20000605)50:3%3C405::AID-JBM15%3E3.0.CO;2-Z
162. Tong Z, Guo J, Glen RC, Morrell NW, Li W. A bone morphogenetic protein (BMP)-derived peptide based on the type I receptor-binding site modifies cell-type dependent BMP signalling. Sci Rep. (2019) 9(1):13446. doi: 10.1038/s41598-019-49758-x
163. Bradford STJ, Ranghini EJ, Grimley E, Lee PH, Dressler GR. High-throughput screens for agonists of bone morphogenetic protein (BMP) signaling identify potent benzoxazole compounds. J Biol Chem. (2019) 294(9):3125–36. doi: 10.1074/jbc.RA118.006817
164. Feng Y, Jin MY, Liu DW, Wei L. Bone morphogenetic protein (BMP) 7 expression is regulated by the E3 ligase UBE4A in diabetic nephropathy. Arch Physiol Biochem. (2020) 126(5):416–9. doi: 10.1080/13813455.2018.1551905
165. Genthe JR, Min J, Farmer DM, Shelat AA, Grenet JA, Lin W, et al. Ventromorphins: a new class of small molecule activators of the canonical BMP signaling pathway. ACS Chem Biol. (2019) 14(7):1673. doi: 10.1021/acschembio.9b00488
166. Vrijens K, Lin W, Cui J, Farmer D, Low J, Pronier E, et al. Identification of small molecule activators of BMP signaling. PLoS One. (2013) 8(3):e59045. doi: 10.1371/journal.pone.0059045
167. Kang Q, Sun MH, Cheng H, Peng Y, Montag AG, Deyrup AT, et al. Characterization of the distinct orthotopic bone-forming activity of 14 BMPs using recombinant adenovirus-mediated gene delivery. Gene Ther. (2004) 11(17):1312–20. doi: 10.1038/sj.gt.3302298
168. Long L, Ormiston ML, Yang X, Southwood M, Graf S, Machado RD, et al. Selective enhancement of endothelial BMPR-II with BMP9 reverses pulmonary arterial hypertension. Nat Med. (2015) 21(7):777–85. doi: 10.1038/nm.3877
169. Rosen H, Glukmann V, Feldmann T, Fridman E, Lichtstein D. Short-term effects of cardiac steroids on intracellular membrane traffic in neuronal NT2 cells. Cell Mol Biol (Noisy-le-grand). (2006) 52(8):78–86. https://www.ncbi.nlm.nih.gov/pubmed/1753574017535740
170. Chen Y, Webster TJ. Increased osteoblast functions in the presence of BMP-7 short peptides for nanostructured biomaterial applications. J Biomed Mater Res A. (2009) 91(1):296–304. doi: 10.1002/jbm.a.32246
171. Bergeron E, Senta H, Mailloux A, Park H, Lord E, Faucheux N. Murine preosteoblast differentiation induced by a peptide derived from bone morphogenetic proteins-9. Tissue Eng Part A. (2009) 15(11):3341–9. doi: 10.1089/ten.TEA.2009.0189
172. Lauzon MA, Drevelle O, Faucheux N. Peptides derived from the knuckle epitope of BMP-9 induce the cholinergic differentiation and inactivate GSk3beta in human SH-SY5Y neuroblastoma cells. Sci Rep. (2017) 7(1):4695. doi: 10.1038/s41598-017-04835-x
173. Kim HK, Kim JH, Park DS, Park KS, Kang SS, Lee JS, et al. Osteogenesis induced by a bone forming peptide from the prodomain region of BMP-7. Biomaterials. (2012) 33(29):7057–63. doi: 10.1016/j.biomaterials.2012.06.036
174. Kim HK, Lee JS, Kim JH, Seon JK, Park KS, Jeong MH, et al. Bone-forming peptide-2 derived from BMP-7 enhances osteoblast differentiation from multipotent bone marrow stromal cells and bone formation. Exp Mol Med. (2017) 49(5):e328. doi: 10.1038/emm.2017.40
175. Lee RT. Adult cardiac stem cell concept and the process of science. Circulation. (2018) 138(25):2940–2. doi: 10.1161/CIRCULATIONAHA.118.036407
176. Bain JL, Bonvallet PP, Abou-Arraj RV, Schupbach P, Reddy MS, Bellis SL. Enhancement of the regenerative potential of anorganic bovine bone graft utilizing a polyglutamate-modified BMP2 peptide with improved binding to calcium-containing materials. Tissue Eng Part A. (2015) 21(17–18):2426–36. doi: 10.1089/ten.TEA.2015.0160
177. Carlson WD, Keck PC, Bosukonda D, Carlson FR. A process for the design and development of novel bone morphogenetic protein-7 (BMP-7) mimetics with an example: tHR-184 [hypothesis and theory]. Front Pharmacol. (2022) 13:864509. doi: 10.3389/fphar.2022.864509
178. Griffith DL, Keck PC, Sampath TK, Rueger DC, Carlson WD. Three-dimensional structure of recombinant human osteogenic protein 1: structural paradigm for the transforming growth factor beta superfamily. Proc Natl Acad Sci U S A. (1996) 93(2):878–83. doi: 10.1073/pnas.93.2.878
179. Pendexter CA, Bolger-Chen M, Lopera Higuita M, Cronin SEJ, Rabi SA, Osho AA, et al. Modified Langendorff perfusion for extended perfusion times of rodent cardiac grafts. JoVE (2024) (208):e66815. doi:doi: 10.3791/66815
180. D'Alessandro AM, Peltier JW, Phelps JE. Increasing organ donations after cardiac death by increasing DCD support among health care professionals: a case report. Am J Transplant. (2008) 8(4):897–904. doi: 10.1111/j.1600-6143.2008.02155.x
181. Gerber AJ, Peterson BS. Measuring transference phenomena with fMRI. J Am Psychoanal Assoc. (2006) 54(4):1319–25. doi: 10.1177/00030651060540040105
182. Penso M, Babbaro M, Moccia S, Baggiano A, Carerj ML, Guglielmo M, et al. A deep-learning approach for myocardial fibrosis detection in early contrast-enhanced cardiac CT images [original research]. Front Cardiovasc Med. (2023) 10:1151705. doi: 10.3389/fcvm.2023.1151705
Keywords: Bone morphogenetic protein, BMP, mimetics, THR-123, THR-184, NMP, TGF, Ex-vivo heart machine perfusion
Citation: Carlson WD, Bosukonda D, Keck PC, Bey P, Tessier SN and Carlson FR (2025) Cardiac preservation using ex vivo organ perfusion: new therapies for the treatment of heart failure by harnessing the power of growth factors using BMP mimetics like THR-184. Front. Cardiovasc. Med. 12:1535778. doi: 10.3389/fcvm.2025.1535778
Received: 27 November 2024; Accepted: 27 February 2025;
Published: 18 March 2025.
Edited by:
Matteo Cameli, University of Siena, ItalyReviewed by:
Hector Rodriguez Cetina Biefer, University Hospital Zürich, SwitzerlandCopyright: © 2025 Carlson, Bosukonda, Keck, Bey, Tessier and Carlson. This is an open-access article distributed under the terms of the Creative Commons Attribution License (CC BY). The use, distribution or reproduction in other forums is permitted, provided the original author(s) and the copyright owner(s) are credited and that the original publication in this journal is cited, in accordance with accepted academic practice. No use, distribution or reproduction is permitted which does not comply with these terms.
*Correspondence: William D. Carlson, d2lsbGlhbS5jYXJsc29uQHRoZXJhcGV1dGljc2J5ZGVzaWduLmNvbQ==
Disclaimer: All claims expressed in this article are solely those of the authors and do not necessarily represent those of their affiliated organizations, or those of the publisher, the editors and the reviewers. Any product that may be evaluated in this article or claim that may be made by its manufacturer is not guaranteed or endorsed by the publisher.
Research integrity at Frontiers
Learn more about the work of our research integrity team to safeguard the quality of each article we publish.