- Department of Cardiovascular Ultrasound, Nanjing First Hospital, Nanjing Medical University, Nanjing, China
Atherosclerosis is a slow, progressive disease that is closely associated with major adverse cardiovascular events. Early diagnosis and risk assessment of atherosclerosis can effectively improve the prognosis and reduce the occurrence of adverse cardiovascular events in the later stage. A variety of invasive and non-invasive imaging modalities are important tools for diagnosing lesions, monitoring the efficacy of treatments, and predicting associated risk events. This review mainly introduces the four commonly used non-invasive imaging modalities in clinical practice and intravascular imaging such as optical coherence tomography, intravascular ultrasound imaging, and near-infrared spectroscopy, compares the advantages and disadvantages in the diagnosis of vulnerable plaques, and briefly summarizes the new progressions of each.
1 Introduction
Atherosclerosis, a chronic, multifactorial, complex disease, is the primary cause of a life-threatening cardiovascular disease, which causes much morbidity, hospitalization, and mortality worldwide (1). After decades of silent progression, atherosclerotic plaque rupture with thrombus formation is the principal pathophysiological mechanism responsible for the development of acute coronary syndromes (1, 2). Traditionally, the clinical assessment of atherosclerosis focuses on the degree of luminal narrowing. As the concept of the “vulnerable plaque” or “unstable plaque” is gradually recognized, it is more comprehensive to identify patient “cardiovascular vulnerability” that inclines to direct assessment of the plaque or indirect statistical risk scores through the morphological and hemodynamic factors in the clinical practice.
The vulnerable plaque is commonly understood as the plaque with specific structural characteristics such as thin fibrous caps, large lipid cores, positive vascular remodeling, intraplaque hemorrhage, macrophage infiltration, and neovascularization (3, 4). Conventional catheter-based coronary angiography is the gold standard that provides direct visualization of the degree of luminal narrowing. However, it fails to assess the hemodynamic consequences of stenoses and the accurate structural features under the endothelium (4). In recent years, several imaging modalities with varying sensitivities and specificities have emerged as the times require. This review aims to summarize the novel imaging modalities for the assessment of vulnerable plaque and prediction, focus on advantages and limitations respectively, and discuss the prospect in the early diagnosis of atherosclerotic vulnerable plaques.
2 Non-invasive imaging
Non-invasive imaging, like computed tomography (CT), magnetic resonance (MR), non-invasive ultrasound techniques, and positron emission tomography (PET), contributes to estimative risk stratification and prediction in low-intermediate-risk populations.
2.1 Computed tomography (CT)
CT is a common diagnostic imaging method used for the examination of various diseases with the characteristics of fast scanning time and clear images. Apart from functional assessment by CT like myocardial CT perfusion or non-invasive CT-derived fractional flow reserve that concentrates on hemodynamically significant stenoses (5), CT imaging can easily detect calcification, which leads to a direct quantitative assessment of coronary artery calcium score (CACS) (4, 6). The high-risk plaque features increase as the Agatston CACS increases (7). Nevertheless, the prognosis is not perfect for patients with zero or minimal calcium score.
While non-contrast CT is limited to quantifying the burden of non-calcified and vulnerable plaques or stenosis, patients can have the intravenous administration of iodine contrast media to get more information (8). Different from conventional angiography, computed tomography angiography (CTA) enables better visualization in three dimensions by the reconstruction and identification of certain characteristics of vulnerable plaques, such as positive remodeling, low-attenuation plaque, napkin ring sign (9) (Figure 1) and spotty calcification (Table 1). Multi-directional imaging with a relatively high spatial resolution (e.g., up to 0.23 mm spatial resolution of last-generation CT scans) and fast scanning speed is suitable for the observation of early vascular lesions that are not easy to find. In the Scottish Computed Tomography of the HEART (SCOT-HEART) trial, compared with standard care, CTA reduced the clinical outcomes-related secondary endpoint of the rate of cardiovascular death or myocardial infarction at 20 months, and increased diagnostic certainty and the frequency of a diagnosis of coronary heart disease at 6 weeks (10). In the PROMISE trial, the investigators also reported that CTA was associated with a 34% relative reduction in all-cause death and myocardial infarction at 12 months (10).
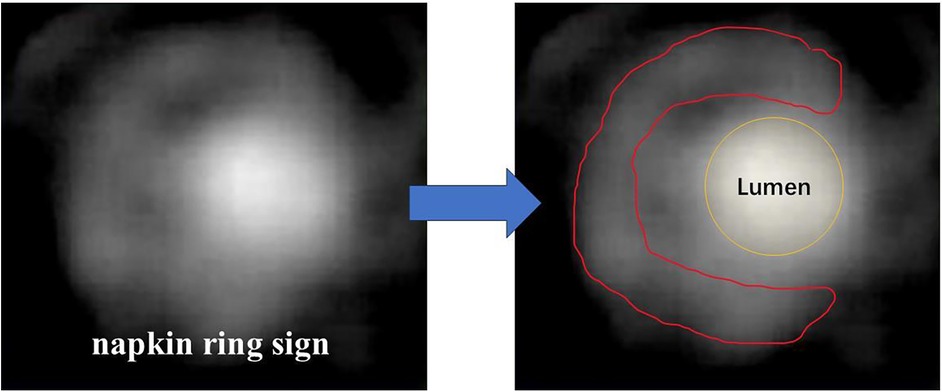
Figure 1. Typical images of napkin ring sign. Lumen contour (yellow line) and fibrous tissue contour (red line) are shown.
Clinical evidence indicates that the phenotype of perivascular adipose tissue (PVAT) is closely related to inflammation and metabolism of the adjacent vasculature, regarded as one of the pathogenesis of atherosclerosis (11). CTA is currently the main imaging modality for evaluating inflammation of PVAT. Recently, a CTA-based biomarker, the peri coronary fat attenuation index, detecting active vascular microcalcification or inflammation by analyzing the three-dimensional changes of CT attenuation from the PVAT around diseased artery segments, is considered a novel metric of inflammation (12, 13). Sun JT, et al. (14) designed a prospective study extending the findings to suggest that the pericoronary fat attenuation index may be a reliable indicator associated with plaque vulnerability. As a result, the fat attenuation index can significantly improve cardiac risk identification and prognosis in both primary and secondary prevention. Similarly, epicardial adipose tissue (EAT) has unique properties, which produce cytokines that nourish the heart in healthy conditions. However, chronic inflammatory disorders can lead to deranged adipogenesis causing atrial and ventricular fibrosis. While high-risk plaque burden identified through assessment of low-attenuation non-calcified coronary plaque volume on CTA was found to concern prognosis, Yamaura H, et al. (15) also proposed that EAT volume is independently associated with increased LAP volume. The combination of CACS and EAT volume provides a non-invasive method of identifying lesions that may develop severe coronary events.
Among the non-invasive imaging in the cardiovascular field, CT is widely used in clinical practice, such as measuring CACS in asymptomatic population and having a CTA scan in patients with acute chest pain and low (about 0–2) Thrombolysis in Myocardial Infarction risk score (5). However, due to its limited sensitivity and specificity, CT is not perfect. Although the CT imaging data are now available at low x-ray exposure, the radiation exposure should be considered as well. Moreover, as for CTA, the contrast medium needed also puts patients at risk of allergic reactions, contrast-induced nephropathy, and so on. Due to an insufficient imaging resolution, CTA has limited accuracy in identifying an intraplaque hemorrhage and a large lipid core and is poor in predicting plaque erosion, which is the potential culprit of about one-third of acute events (2).
2.2 Magnetic resonance (MR)
As is known, MR imaging has the advantages of multi-sequence imaging, multidirectional reconstruction, and excellent soft tissue resolution. While several high-risk plaque features can be detected in CTA as mentioned, MR can provide more detailed and comprehensive imaging. For example, magnetic resonance angiography can accurately detect thin fibrous caps which is difficult to discriminate in CTA (16, 17). The multiple sequences of MR give it a great advantage in distinguishing plaque components. In non-contrast T1-weighted sequences, for instance, lipid-rich necrotic plaques, or intraplaque hemorrhage, displayed on T1-weighted sequences tend to have short T1 values, that is, high signal intensity. Therefore, high-intensity plaques are regarded as vulnerable lesions.
Currently, vessel wall high-resolution magnetic resonance imaging (VW-HRMRI) plays an important role in early diagnosis and clinical treatment. There is high consistency between VW-HRMRI and histopathology in detecting vulnerable plaques, such as qualitative analysis of the characteristics of intraplaque hemorrhage, intraplaque inflammation and neovascularization, plaque surface ulceration, positive vascular remodeling, lipid-rich necrotic cores and thin fibrous caps, and quantitative analysis of common parameters include plaque volume and thickness, the normalized wall index, the remodeling index and the vascular stenosis ratio, and so on (3). However, VW-HRMRI is limited to a single plaque or vascular segment, challenging the evaluation over larger sections of the arterial tree.
MR based on gadolinium, of which the accumulation in the arterial wall is correlated with endothelial permeability and inflammation, can provide better spatial resolution. Plasma concentrations of myeloperoxidase (MPO) can predict future adverse coronary events and are most likely associated with plaque instability (18). Gadolinium-enhanced MR imaging has been shown to non-invasively distinguish increased MPO activity in vulnerable plaques (19); thus it has the potential to identify high-risk plaques and evaluate therapies. However, the contrast agent based on gadolinium chelates is concerned with systemic toxicity and deposits. To address the concerns, Evans RJ, et al. (20) developed a non-toxic and high relaxivity probe based on superparamagnetic iron oxide nanoparticles, which accumulate in similar regions to an elastin-targeted agent, for visualizing plaque burden. Although the novel contrast agent is not specific for vulnerable plaques, it may contribute to improving specific contrast agents in the future. What's more, with the advancement of nanotechnology, more and more attention is paid to the development of targeted medical imaging and therapeutic strategies. Bagalkot V, et al. (21) reported lipid–latex hybrid nanoparticles selectively targeting inflammatory macrophages like plaque M1 macrophages and releasing a load of therapeutic materials like anti-inflammatory drugs. Though the nanoparticles cannot pass regulatory requirements for clinical translation, the principles of phagocytic targeting can be generalized to any other biocompatible and biodegradable nanoparticles. Foamy macrophages can secrete a large number of inflammatory cytokines to play a role in the development and rupture of atherosclerotic plaques which cause the vulnerability of plaques. Among inflammatory cytokines, IL−6 is thought to be possibly used as an independent marker for predicting future cardiovascular events (22). Mo H, et al. (23) prepared IL-6-targeted superparamagnetic iron oxide nanoparticles synthesized by a chemical condensation reaction and demonstrated its IL-6-targeted characteristic, as well as the usefulness for detection of vulnerable plaques with good sensitivity, specificity, and biocompatibility. Another example is that Dai Y, et al. (24) modified Gd-doped Prussian blue with polyethyleneimine, rhodamine, and dextran sulfate via electrostatic adsorption, which reduced inflammation, apoptosis, and the formation of foam cells.
Compared with CT, MR has certain advantages including better evaluation of soft tissue, lack of blooming artifacts, and no exposure to radiation, but its limitations, including a relatively long scan time for the assessment in vivo due to its high signal-to-noise ratio, and contraindications like patients with claustrophobia or metal devices, cannot be ignored either. Also, the low spatial resolution of MR (0.6 mm) makes it poor in clinical practice, for example, detection of coronary arteries is challenging for MR due to their small diameter, deep location, and influence by cardiac cycles and diaphragmatic movements. Without available data, the prediction of plaque erosion, just like CT, is worrying (2). Maybe the development of motion correction techniques and image reconstruction is expected to be better adapted to clinical applications in the future.
2.3 Non-invasive ultrasound
Ultrasound, as a widely used diagnostic tool in clinical practice, is portable, non-radiation, and relatively low-cost. Nowadays more novel ultrasound techniques have been developed, occupying an important position in the cardiovascular field. In addition to intravascular ultrasound imaging, non-invasive methods can also be used for the evaluation of vulnerable plaques. Table 2 summarizes the role of different non-invasive ultrasound in the detection of vulnerable plaques.
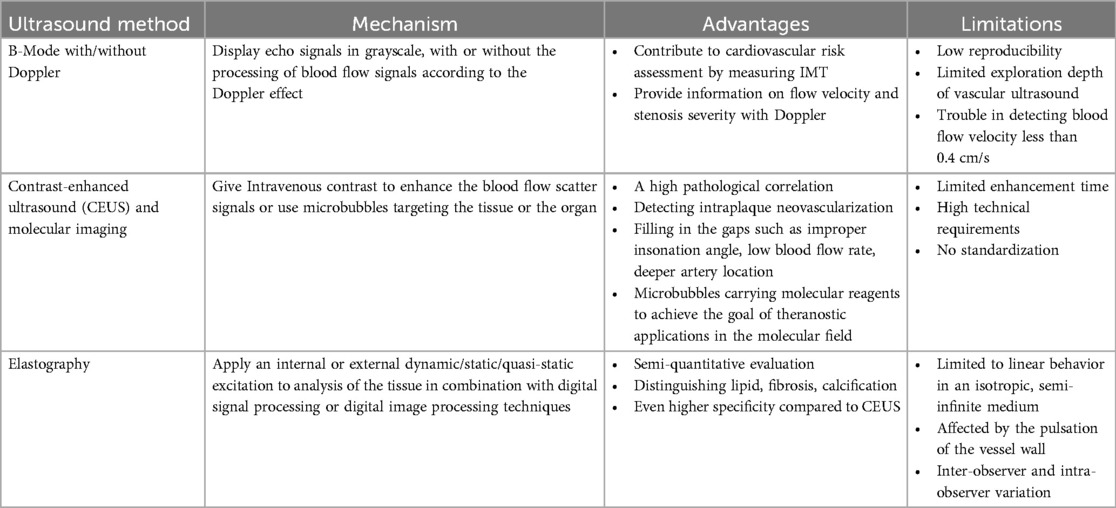
Table 2. An overview of the mechanism, advantages, and limitations of three common types of non-invasive ultrasound in the detection of vulnerable plaques.
Real-time ultrasound (B-Mode, 2D Mode) is the primary imaging modality for initial assessment that can diagnose artery stenosis, as well as identify and locate plaque (25). With Doppler, color flow mode, it provides information on flow velocity, stenosis severity, and plaque surface and composition. There is an ultrasound-based classification, called Gray-Weale–Nicolaides (GWN) classification, distinguishing between five types (classes) of plaques, that is, uniformly echolucent plaque, predominately echolucent plaque, predominantly echogenic plaque, uniformly echogenic plaque, heavy calcification (26). (Figure 2) In addition, carotid intima-media thickness (IMT) is a quantitative parameter measured using high-resolution B-mode ultrasound, which is implicated in cardiovascular risk assessment. (Figure 3) IMT is more considered a surrogate marker of atherosclerosis, and yet its importance is rather debatable (27, 28). Also, considering the asynchronous movement of the plaque from B-mode ultrasound associated with a higher risk of stroke, Golemati S, et al. (29) statistically analyzed 135 plaques in 77 patients (59 men, 18 women) with carotid atherosclerosis and proved the ability to obtain tissue kinematic characteristics. It can be utilized to develop ultrasound-based risk stratification.
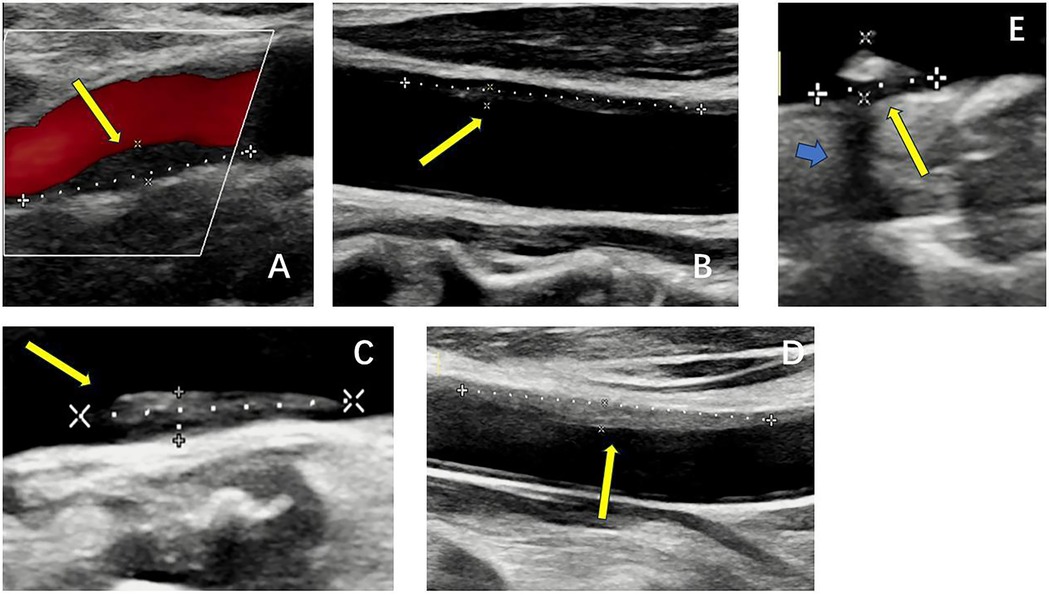
Figure 2. B-mode ultrasound images of five types of plaques distinguished by the gray-weale–nicolaides (GWN) classification including (A) uniformly echolucent plaque, (B) predominately echolucent plaque, (C) predominantly echogenic plaque, (D) uniformly echogenic plaque, and (E) heavy calcification. The yellow arrows indicate plaques. The blue arrow indicates the shadow.
Due to the frequency of the linear-array probe (around 5–10 MHz), nevertheless, the exploration depth of vascular ultrasound is limited. To solve this problem, contrast agents (mostly microbubbles) can be used. At present, contrast-enhanced ultrasound (CEUS) is commonly used in clinical practice for defining endocardial border or for left ventricular opacification. When used to assess atherosclerotic plaques, CEUS compensates for defects such as improper insonation angle, low blood flow rate, deeper artery location, and difficulty in identifying intraplaque neovascularization with conventional B-mode ultrasound, and even allows for better measurement of IMT (30, 31). A study confirmed that CEUS has a high pathological correlation (32). The novel technology is also expected to achieve the goal of theranostic applications in the molecular field. VCAM-1 is a promising target for the detection of early and advanced atherosclerotic lesions as well. The use of microparticles for detecting VCAM-1 in molecular imaging methods has been described (31, 33). Since VCAM-1 is located on the endothelial surface, the blood contrast agent is easily accessible. However, to date, studies have not been tested outside of animal models, and the clinical applicability remains a challenge. Moreover, molecular expression varies at different stages of atherosclerosis development, making it impossible to obtain complete information from a single targeted imaging.
Besides, elastography can translate the obtained elastic information of biological materials into visible images so that we can distinguish the mechanical properties of tissue materials and then determine the possible pathological changes, position, shape, and size of corresponding tissues or organs based on their conditions. Using the principle of elastography, we can distinguish components of plaques like lipid (soft), fibrosis (moderately hard), calcification (hard), etc. Given that stable plaques are fibrocalcific, heterogeneous strain distribution suggests plaque vulnerability. In addition, neovascularization may improve the performance of ultrasound non-invasive vascular elastography, which possibly makes it a valuable alternative to MR for plaque assessment (30). Strain and shear-wave elastography allows for semi-quantitative evaluation of plaques and the characterization of plaque components. Shear-wave elastography, a common measure to quantify elastic modulus distribution in atherosclerotic plaque, seems more unequivocal than strain elastography (34). What's more, Di Leo N, et al.’s (35) research reflected high sensitivity (87.1%) of shear-wave elastography and even higher specificity compared to CEUS (66.7% vs.58.3%) in identifying vulnerable plaques. However, assumptions of linear behavior in an isotropic, semi-infinite medium and the pulsation of the vessel wall limit its application. More extensive and long-term controlled clinical studies are required to further validate the accuracy of elastography in detecting vulnerable plaques.
2.4 Positron emission tomography (PET)
Inflammation is a major factor in the development and progression of atherosclerosis. In recent years, the assessment of plaques has gradually shifted from a single morphological analysis to a comprehensive evaluation of morphological characteristics and inflammatory activity. With molecular imaging being developed, PET imaging, a non-invasive nuclear imaging technique, is at the forefront. Based on the employing intravenous administration of a selective radiotracer, PET imaging can target specific biochemical processes in vivo. Based on its characteristics, PET imaging has been widely used in oncology and neurology. It has recently been considered for clinical applications such as risk stratification and prediction in the cardiovascular field because of its highly sensitive assessment of disease activity. To achieve this goal, the most important thing is to develop the right radiotracer.
The use of glucose analogs like 18F-fluorodeoxyglucose (18F-FDG) is most common, which targets the inflammation that relies on the highly metabolic state of macrophages, and takes advantage of the characteristics of abnormal glucose metabolism in the lesion for localization, diagnosis, and evaluation. Galiuto L, et al. (36) demonstrated for the first time that there is a high correspondence between increased 18F-FDG uptake and the unstable morphology of coronary plaques on intravascular imaging. However, it should be noted that due to its non-specificity, it can also be taken up by adjacent tissues limiting imaging accuracy, especially in the myocardium, which has high glucose metabolism and high affinity for 18F-FDG (37). It is necessary to perform special patient preparation in advance to minimize myocardial glucose uptake (4, 38, 39).
In addition to inflammation, microcalcification, which creates local mechanical stress on the plaque surface leading to plaque rupture, is also associated with vulnerable plaques (40). PET imaging detects microcalcification more reliably than other non-invasive imaging modalities. Therefore, several recent studies are exploring the application of 18F-sodium fluoride (18F-NaF) in detecting vulnerable plaques. 18F-NaF is a positron bone imaging agent. It is concentrated by bone tissue through ion exchange, reflecting changes in bone metabolism. Interestingly, it tends to bind to newly formed hydroxyapatite more than the old crystals, which is more helpful in identifying actively inflamed calcifications (4, 41). What's more, 18F-NaF may detect areas of tissue necrosis at the site of myocardial infarction and even be related to PVAT, another marker of vascular inflammation mentioned above (37). A combination of features suggests that it is likely to be an excellent choice for the detection of vulnerable plaques.
Besides, there are various other targeted tracers for the detection of atherosclerosis. It is generally believed that nicotinic acetylcholine receptors (nAChRs), pentameric ligand-gated cation channels, are mainly found in central and peripheral nervous system diseases. Recently, however, it has been found that nAChRs, especially the α7nAChRs, can also be expressed in endothelial cells and may be an early target for vulnerable atherosclerotic plaques. Wang D, et al. (42) verified the reliability of an 18F-labeled PET molecular probe with high selectivity for α7nAChR in early diagnosis of atherosclerosis, which may play an early warning role in cardiovascular events. Further studies on the radiotracers for early plaque diagnosis are ongoing. Equally, 68Ga-pentixafor is thought that plaque vulnerability can be assessed through cell recruitment, the accumulation of oxidized LDL, and the formation of foam cells, which are related to Chemokine receptor-4, but fail to prove the exact cellular source (43, 44). Increased 68Ga-DOTATATE uptake with specificity for the G-coupled receptor somatostatin receptor subtype-2, whose expression is highly up-regulated in activated macrophages affected by an inflammatory stimulus, is associated with risk factors of cardiovascular disease (45, 46). More information on the different tracers is provided in Table 3.
Though PET has advantages in its excellent sensitivity and quantitative efficiency, disadvantages such as the low spatial resolution (about 6 mm), the effects of heartbeats and respiration on signals, radiation exposure, and high cost cannot be ignored. Since PET signals cannot distinguish between plaques with rupture and plaques with erosion, it may not be suitable to provide adequate indications for individualized treatment. At the same time, due to the shortcomings of PET in anatomical structure, it also needs to be combined with CT or MR to obtain a more accurate diagnosis.
The non-invasive imaging modalities described above have their advantages and disadvantages and are generally less hurt. The comparison among them is summarized in Table 4.
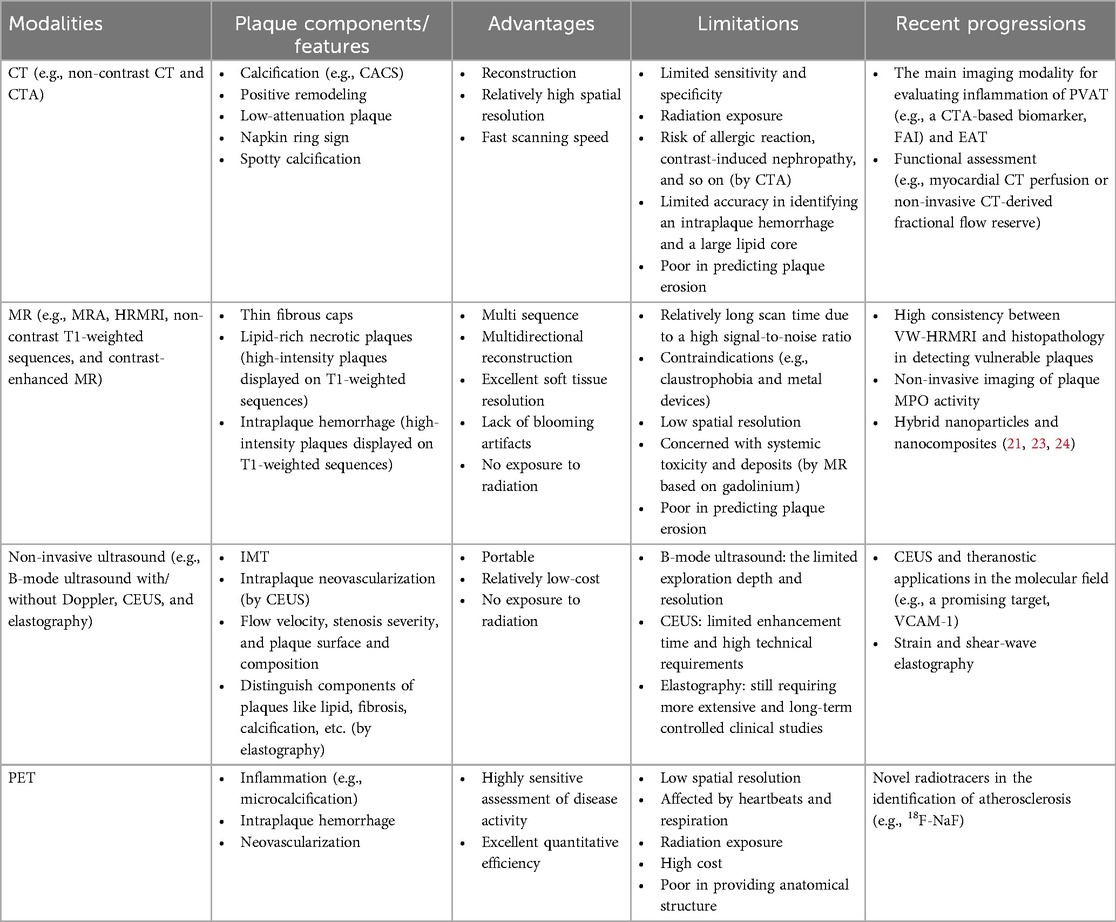
Table 4. Comparison of different non-invasive imaging methods in the detection of vulnerable plaques.
3 Invasive imaging
Invasive imaging, such as optical coherence tomography (OCT), intravascular ultrasound imaging (IVUS), and near-infrared spectroscopy (NIRS), enables the accurate assessment of vulnerable plaques in high-risk patients.
3.1 Optical coherence tomography (OCT) and intravascular ultrasound imaging (IVUS)
Based on the principle of low coherence interference, OCT can reconstruct a two-dimensional or three-dimensional image of the internal structure through scanning. The signal contrast is derived from the spatial variation of the optical reflection/scattering characteristics. In terms of imaging, the fast data acquisition speed and lack of artifacts make it an excellent advantage as a potentially invasive tool for plaque detection. A comparative study has also confirmed the ability of OCT to assess vascular disease (55). Prati F, et al. (56) designed the first OCT study that directly found the morphology of coronary plaques obtained by OCT has the potential to be a powerful tool for predicting the risk of adverse cardiovascular events. The combination of multiple characteristics of OCT high-risk plaques is more useful in screening patients at higher risk of acute coronary events. Similar results were reflected in the Massachusetts General Hospital Optical Coherence Tomography Registry (57) which indicated lipid-rich plaques detected by OCT were at greater risk of non-culprit lesion-related major adverse cardiovascular events. At the same time, lesions of thin-cap fibroatheroma, a precursor lesion for plaque rupture, detected by OCT have also been shown to be associated with an increased risk of major adverse cardiovascular events during long-term follow-up (58). OCT is also the only imaging modality that accurately diagnoses plaque erosion in vivo. OCT-defined plaque erosion is divided into definite erosion and probable erosion (2, 59). The former has a thrombus covering the intact plaque, while the latter may be non-thrombotic but have an irregular luminal surface at the lesion, or the thrombus may attenuate the underlying plaque without superficial lipids or calcifications proximal or distal to the site. Furthermore, Huang M, et al. (60) compared the differences between the multilayer and single-layer 3D thin-layer models and found the single-layer model may overestimate the outer wall stress and underestimate the outer wall strain as well as the risk of plaque rupture. The current literature is affected by the lack of multilayer image segmentation technology and limited resolution of imaging modalities, therefore, the multilayer plaque model based on in vivo images has not been studied. However, the emergence of OCT has brought about an improvement in resolution, making it possible to distinguish the three-layer structure of blood vessels.
When it comes to detecting atherosclerotic plaques, the high resolution (10–20 µm) of OCT allows it to discriminate superficial plaque components at a microscopic level, including quantifying the presence of macrophages and cholesterol crystal, discriminating lipid-rich plaques and visualizing fibrous cup thickness (Figure 4) and calcifications. However, it is limited by the poor penetration (1–2 mm) causing it not suitable for the measurement of plaque load. Unlike OCT, IVUS has greater penetration and can assess the entire arterial wall (38).
IVUS, utilizing a combination of non-invasive ultrasound technology and invasive catheter technology to image, is the first intravascular imaging modality to be applied in clinical practice. It visualizes the cardiovascular cross-sectional morphology and/or blood flow patterns, which can be used to examine the inner wall of the blood vessels. Grayscale IVUS can broadly divide plaques compared to surrounding adventitia echogenicity into four categories: soft plaques, fibrous plaques, calcified plaques, and mixed plaques (31). However, due to the distortion, grayscale IVUS cannot accurately discriminate plaque components. With the advancement of technology, intravascular ultrasound radiofrequency, i.e., virtual histology intravascular ultrasound (VH-IVUS), has emerged. VH-IVUS optimizes the differentiation of plaque components, thereby dividing plaques into fibrous tissue (dark green), fibrofatty tissue (light green), necrotic core (red), and dense calcium (white) (38). Additionally, high-definition IVUS facilitates the analysis of the luminal surface of blood vessels, which is expected to be used to detect plaque erosion (2). Generally, IVUS can provide qualitative assessments including identification of thin fibrous caps and analysis of plaque composition, as well as quantitative assessments such as plaque thickness, cross-sectional area, plaque burden, and remodeling index.
Whereas the insufficient spatial resolution (around 100–150 µm) of IVUS, the accuracy of the assessment of plaque composition is affected. Positive predictive values were found to be low at follow-up (61, 62). In a study of patients with ST-elevation myocardial infarction (61), VH-IVUS was found to be likely to overestimate the presence of thin-cap fibroatheroma probably because of its lack of the resolution to directly image fibrous cap thickness. The natural history Providing Regional Observations to Study Predictors of Events in the Coronary Tree (PROSPECT) study reported that IVUS was not able to visualize the entire coronary tree (63). Its resolution is proportional to the center frequency, but increasing the center frequency decreases the penetration depth, which can be improved if the probe can choose from a variety of frequency options depending on the region of interest (64). Due to the limitations of technology and process, nevertheless, the acoustic stack used for research is too large to perform intravascular application. Therefore, it still cannot be applied in clinical practice today.
The imaging signal of IVUS is susceptible to calcified areas, which makes it difficult to match OCT in terms of calcification. OCT can penetrate the calcified area of plaque, facilitate visualization of the adjacent tissue, and take a detailed measurement, dividing plaque calcifications into macrocalcifications, spotty calcifications, and microcalcifications (4). Unfortunately, OCT is inferior in detecting positive remodeling or plaque burden (61). In addition to the lack of clinical studies to establish a reliable clinical utility for predicting risk stratification, attenuation of light by blood also significantly impacts imaging and assessment. To overcome blood interference, methods such as saline flushes and balloon occlusion can be employed. Optical frequency domain imaging (OFDI) is a next-generation OCT technology that eliminates the need to block blood flow and provides fast imaging. Polarimetric measurements simultaneously with conventional OFDI cross-sectional imaging can obtain birefringence and depolarization of tissue (65). The high birefringence of the tunica media, as well as the significant depolarization of the lipid and necrotic core materials, make it not only provide a reliable assessment of the external elastic membrane but also determine fibrous cap thickness quantitatively and automatically. The first-in-human pilot study of intravascular polarimetry has also shown that caps of vulnerable plaques including ruptured caps exhibited lower birefringence than caps of stable lesions (66).
To sum up, OCT does better in imaging luminal plaque, calcium, and foreign body structures, such as stent struts in summary, while IVUS has a stronger penetrating ability and does not have the problem of being masked by blood flow, which has a greater advantage in vessels with larger luminal diameters.
3.2 Near-infrared spectroscopy (NIRS)
Because different tissues in vivo have different absorption and scattering characteristics of near-infrared light, the light has a strong ability to distinguish them. According to this characteristic, NIRS can be used to measure the optical parameters to obtain the physiological parameters of tissues, establish the relationship between physiological parameters and spectral data, generate two-dimensional images, or conduct clinical analysis. Recently, NIRS has been found to have the ability to detect high-risk plaques (67, 68). Plaques with a large lipid core tend to have a greater plaque burden, which is one of the risk factors for adverse cardiovascular events (69, 70). NIRS can accurately and quantitatively characterize the lipid content of a plaque based on the absorption pattern by cholesterol molecules, which is challenging in IVUS and OCT. Nonetheless, NIRS provides minimal anatomic visualization, that is, it is necessary to combine other modalities like IVUS to get more detailed and complete information on the lumen or the plaque (71). Lipid core burden assessment can be used as a supplement to plaque structure information for future use to refine risk stratification and treatment. On the clinical application of NIRS, there is relatively little clinical evidence, and more data are needed to support it.
3.3 Others
In addition to the invasive imaging modalities mentioned above, there are many other emerging techniques for detecting plaque features. Near-infrared fluorescence (NIRF) is a molecular intravascular imaging modality detecting inflammation, oxidative stress, and abnormal endothelial permeability with the help of fluorescent probes at a cellular and molecular level (72, 73), which may play a role in identifying plaques that are susceptible to erosion (2). Near-infrared autofluorescence can detect intraplaque hemorrhage by autofluorescence within the plaque (74). However, a clear association between NIRAF and cardiovascular disease is still needed through natural history studies with clinical follow-up. Besides, angioscopy visualizes the surface appearance of plaques like substantial information of atherosclerosis as yellow plaques (67), but has not demonstrated the clinical predictive value (74). A study performed survival intravascular photoacoustic imaging (IVPA) for quantitative assessment of lipid-rich atherosclerotic plaques in rabbits and demonstrated the potential of the clinical use of IVPA catheters for the detection of lipid-rich, high-risk plaques (75). Fluorescence lifetime imaging microscopy (FLIM) of plaques can measure the biochemical content near the luminal surface and evaluate the relative concentration of lipids and collagen.
Although the above imaging methods are invasive, the information obtained will be more accurate and intuitive. Table 5 compares the three main invasive imaging modalities (e.g., OCT, IVUS, and NIRS), and the advantages and disadvantages of the eight non-invasive and invasive imaging modalities mentioned in this paper in the visualization of vulnerable plaques are summarized (Table 6).
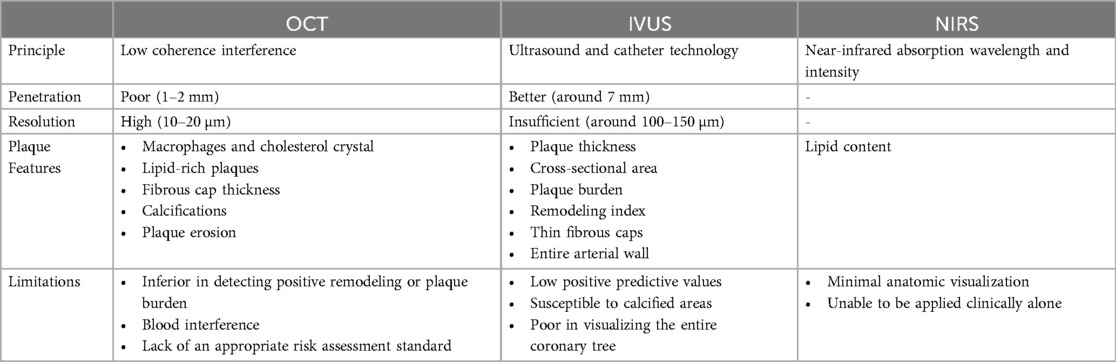
Table 5. Comparison of the three main invasive imaging methods in the detection of vulnerable plaques.
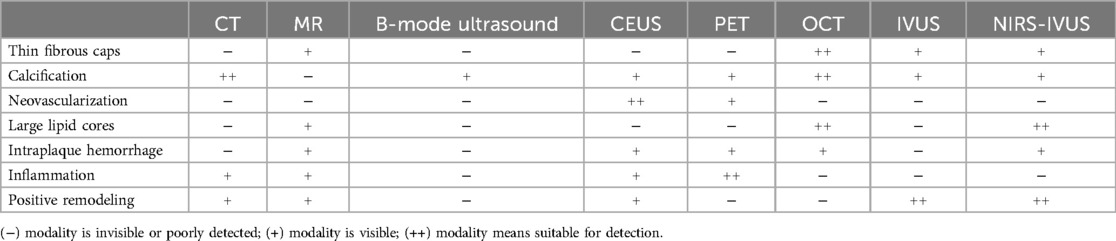
Table 6. Comparison of the eight non-invasive and invasive imaging modalities for visualization of vulnerable plaque. Among the modalities, NIRS needs to combine other modalities clinically, so the hybrid imaging mode of NIRS-IVUS is used here to add to the comparison.
4 Prospect
As the understanding of plaques has deepened, more and more attention has been paid to combining imaging modalities with different strengths to detect multiple features for a more accurate assessment of plaques, known as multimodality imaging. For instance, OCT with high resolution of thin fibrous caps can be fused with IVUS to accurately predict plaque cap thickness and obtain relevant mechanical information (76, 77). Nowadays co-registration of IVUS and OCT slices is performed using fiduciary points such as side branches, bifurcations, and calcifications. However, it is technically difficult to acquire both IVUS and OCT data at the same time. Data acquisition requires two separate catheterizations, both of which are invasive and expensive. The harm to the patient is undoubtedly greater (77). Devices that can perform both IVUS and OCT are under development and may be available shortly. Similarly, an emerging hybrid imaging modality combining IVUS with IVPA provides specific lipid detection and localization, and the dual-frequency IVPA/US catheter has a diameter of 1 mm, making it easy to accommodate current catheterization (78), while the OCT-NIRS catheter with automated co-registration of data contributes to improving stenting, as well as detect and treat vulnerable plaques (79). For the first time, Leng J, et al. (80) have developed a new multispectral intravascular three-modality imaging system integrating OCT, IVUS, and IVPA that can provide macro- and microstructural information of the vessel wall, as well as identify and quantify lipids. A single imaging method cannot provide comprehensive information about plaques, so multimodality imaging will be a promising future development direction, and clinical trials and technological breakthroughs with large samples are still needed.
The main focus of the discussion above is basically around the morphological imaging of vulnerable plaques. Recently, hemodynamic-associated biomechanical forces have also been taken into consideration. More than one study supports the close correlation between the formation and progression of plaques and hemodynamic characteristics, that is, the local hemodynamic parameters are likely to be regarded as prognostic markers for the assessment of plaque vulnerability (81–85). For example, the formation of plaque usually occurs in the low wall shear stress (WSS) area, while plaque instability is more related to high wall shear stress (81). The same results are also reflected in the research by Moerman AM, et al. (82) in which high time-averaged WSS is related to larger macrophage areas and necrotic core sizes. Their study is the first to show relations between the oscillatory shear index (OSI) and characteristics of plaque vulnerability, which supported low OSI is linked to larger macrophage areas as well, and a combination of low time-averaged WSS and low OSI is associated with larger cap thickness. In short, it contributes to the prognostic value of the prediction of plaques at different stages. WSS is currently measured invasively causing trauma to patients. Therefore, future development of similar algorithms in non-invasive imaging is required, like the CT-based measurement of fraction flow reserve. Computational fluid dynamics (CFD) is the most powerful research tool to assess patterns of WSS at present but needs to address many assumptions and procedures with strict requirements, such as three-dimensional reconstruction of the vessel, meshing, and solving the Navier–Stokes equation. Regarding the issue of three-dimensional reconstruction, coupled with a preference for non-invasive imaging, the trend is to combine the imaging system with CFD in the future (81, 83). Combining morphological and biomechanical features to determine plaque instability can improve diagnostic accuracy (86). In conclusion, morphological imaging with biomechanical measurements can better screen patients but still requires more clinical data support, and further improves algorithms as well as provides a set of standards.
5 Conclusion
In non-invasive imaging, CT, a high spatial resolution imaging method, has irreplaceable advantages in the evaluation of calcification and perivascular adipose tissue, but its radiation exposure and poor ability to distinguish between lipid and fiber components need to be paid attention to. In contrast, MR has no radiation and has a strong ability to identify soft tissues with multi-sequence and multi-directional reconstruction, so the plaque morphological information obtained is more comprehensive. However, its scanning time is too long, which affects the clinical application. The development of novel ultrasound techniques makes the assessment of plaque no longer limited to stenosis. Therefore, we can obtain more detailed information from the mechanical and even molecular aspects. PET, as a cutting-edge diagnostic tool for molecular application, can be used in the diagnosis of high-risk plaques as well.
In invasive imaging, the high resolution of OCT makes it accurate for the diagnosis of calcifications and thin fibrous caps and is the only imaging method for accurate diagnosis of plaque erosion in vivo. However, its penetration is not good and the signals are deeply affected by blood. IVUS, a commonly used intravascular imaging tool in clinical practice, can evaluate the lumen-related mechanical information and plaque burden. Different from OCT, its penetration of calcium is not high, and the resolution is still poor. NIRS has an excellent ability to detect lipids, but due to the limited anatomical structure information it provides, it still needs to be combined with other methods like IVUS in clinical use. In addition, there are many other emerging invasive imaging modalities such as NIRF, angioscopy, IVPA, and FLIM.
Despite the variety of imaging modalities available, none of them can be used alone to obtain comprehensive information to determine the vulnerability of plaques. Combining multiple imaging modalities to give full play to their respective advantages will be a significant trend in the future. At the same time, the combination of morphological and biomechanical characteristics of vulnerable plaques is also an important study. It is necessary to provide an evaluation standard for the combination of the two in the future, to make a more accurate early diagnosis of atherosclerotic plaques.
Author contributions
ZW: Writing – original draft, Writing – review & editing. PZ: Writing – review & editing.
Funding
The author(s) declare that no financial support was received for the research, authorship, and/or publication of this article.
Conflict of interest
The authors declare that the research was conducted in the absence of any commercial or financial relationships that could be construed as a potential conflict of interest.
Publisher's note
All claims expressed in this article are solely those of the authors and do not necessarily represent those of their affiliated organizations, or those of the publisher, the editors and the reviewers. Any product that may be evaluated in this article, or claim that may be made by its manufacturer, is not guaranteed or endorsed by the publisher.
References
1. Libby P, Theroux P. Pathophysiology of coronary artery disease. Circulation. (2005) 111(25):3481–8. doi: 10.1161/CIRCULATIONAHA.105.537878
2. Collet C, Conte E, Mushtaq S, Brouwers S, Shinke T, Coskun AU, et al. Reviewing imaging modalities for the assessment of plaque erosion atherosclerosis. Atherosclerosis. (2021) 318:52–9. doi: 10.1016/j.atherosclerosis.2020.10.017
3. Han N, Ma Y, Li Y, Zheng Y, Wu C, Gan T, et al. Imaging and hemodynamic characteristics of vulnerable carotid plaques and artificial intelligence applications in plaque classification and segmentation. Brain Sci. (2023) 13(1):143. doi: 10.3390/brainsci13010143
4. Henein MY, Vancheri S, Bajraktari G, Vancheri F. Coronary atherosclerosis imaging. Diagnostics (Basel). (2020) 10(2):65. doi: 10.3390/diagnostics10020065
5. Seitun S, Clemente A, De Lorenzi C, Benenati S, Chiappino D, Mantini C, et al. Cardiac CT perfusion and FFR(CTA): pathophysiological features in ischemic heart disease. Cardiovasc Diagn Ther. (2020) 10(6):1954–78. doi: 10.21037/cdt-20-414
6. Channon KM, Newby DE, Nicol ED, Deanfield J. Cardiovascular computed tomography imaging for coronary artery disease risk: plaque, flow and fat. Heart. (2022) 108(19):1510–5. doi: 10.1136/heartjnl-2021-320265
7. Osborne-Grinter M, Kwiecinski J, Doris M, McElhinney P, Cadet S, Adamson PD, et al. Association of coronary artery calcium score with qualitatively and quantitatively assessed adverse plaque on coronary CT angiography in the SCOT-HEART trial. Eur Heart J Cardiovasc Imaging. (2022) 23(9):1210–21. doi: 10.1093/ehjci/jeab135
8. Williams MC, Kwiecinski J, Doris M, McElhinney P, D’Souza MS, Cadet S, et al. Low-attenuation noncalcified plaque on coronary computed tomography angiography predicts myocardial infarction. Circulation. (2020) 141(18):1452–62. doi: 10.1161/CIRCULATIONAHA.119.044720
9. Otsuka K, Fukuda S, Tanaka A, Nakanishi K, Taguchi H, Yoshikawa J, et al. Napkin-ring sign on coronary CT angiography for the prediction of acute coronary syndrome. JACC Cardiovasc Imaging. (2013) 6(4):448–57. doi: 10.1016/j.jcmg.2012.09.016
10. Fordyce CB, Newby DE, Douglas PS. Diagnostic strategies for the evaluation of chest pain: clinical implications from SCOT-HEART and PROMISE. J Am Coll Cardiol. (2016) 67(7):843–52. doi: 10.1016/j.jacc.2015.11.055
11. Liu Y, Sun Y, Hu C, Liu J, Gao A, Han H, et al. Perivascular adipose tissue as an indication, contributor to, and therapeutic target for atherosclerosis. Front Physiol. (2020) 11:615503. doi: 10.3389/fphys.2020.615503
12. Klüner LV, Oikonomou EK, Antoniades C. Assessing cardiovascular risk by using the fat attenuation Index in coronary CT angiography. Radiol Cardiothorac Imaging. (2021) 3(1):e200563. doi: 10.1148/ryct.2021200563.3
13. Antonopoulos AS, Angelopoulos A, Tsioufis K, Antoniades C, Tousoulis D. Cardiovascular risk stratification by coronary computed tomography angiography imaging: current state-of-the-art. Eur J Prev Cardiol. (2022) 29(4):608–24. doi: 10.1093/eurjpc/zwab067
14. Sun JT, Sheng XC, Feng Q, Yin Y, Li Z, Ding S, et al. Pericoronary fat attenuation Index is associated with vulnerable plaque components and local immune-inflammatory activation in patients with non-ST elevation acute coronary syndrome. J Am Heart Assoc. (2022) 11(2):e022879. doi: 10.1161/JAHA.121.022879
15. Yamaura H, Otsuka K, Ishikawa H, Shirasawa K, Fukuda D, Kasayuki N. Determinants of non-calcified low-attenuation coronary plaque burden in patients without known coronary artery disease: a coronary CT angiography study. Front Cardiovasc Med. (2022) 9:824470. doi: 10.3389/fcvm.2022.824470
16. Dakis K, Nana P, Athanasios C, Spanos K, Konstantinos B, Giannoukas A, et al. Carotid plaque vulnerability diagnosis by CTA versus MRA: a systematic review. Diagnostics (Basel). (2023) 13(4):646. doi: 10.3390/diagnostics13040646
17. Benson JC, Saba L, Bathla G, Brinjikji W, Nardi V, Lanzino G. MR imaging of carotid artery atherosclerosis: updated evidence on high-risk plaque features and emerging trends. AJNR Am J Neuroradiol. (2023) 44(8):880–8. doi: 10.3174/ajnr.A7921
18. Teng N, Maghzal GJ, Talib J, Rashid I, Lau AK, Stocker R. The roles of myeloperoxidase in coronary artery disease and its potential implication in plaque rupture. Redox Rep. (2017) 22(2):51–73. doi: 10.1080/13510002.2016.1256119
19. Rashid I, Maghzal GJ, Chen YC, Cheng D, Talib J, Newington D, et al. Myeloperoxidase is a potential molecular imaging and therapeutic target for the identification and stabilization of high-risk atherosclerotic plaque. Eur Heart J. (2018) 39(35):3301–10. doi: 10.1093/eurheartj/ehy419
20. Evans RJ, Lavin B, Phinikaridou A, Chooi KY, Mohri Z, Wong E, et al. Targeted molecular iron oxide contrast agents for imaging atherosclerotic plaque. Nanotheranostics. (2020) 4(4):184–94. doi: 10.7150/ntno.44712
21. Bagalkot V, Badgeley MA, Kampfrath T, Deiuliis JA, Rajagopalan S, Maiseyeu A. Hybrid nanoparticles improve targeting to inflammatory macrophages through phagocytic signals. J Control Release. (2015) 217:243–55. doi: 10.1016/j.jconrel.2015.09.027
22. Ferencik M, Mayrhofer T, Lu MT, Bittner DO, Emami H, Puchner SB, et al. Coronary atherosclerosis, cardiac troponin and interleukin-6 in patients with chest pain: results from the PROMISE trial. JACC Cardiovasc Imaging. (2022) 15(8):1427–38. doi: 10.1016/j.jcmg.2022.03.016
23. Mo H, Fu C, Wu Z, Liu P, Wen Z, Hong Q, et al. IL-6-targeted ultrasmall superparamagnetic iron oxide nanoparticles for optimized MRI detection of atherosclerotic vulnerable plaques in rabbits. RSC Adv. (2020) 10(26):15346–53. doi: 10.1039/c9ra10509c
24. Dai Y, Sha X, Song X, Zhang X, Xing M, Liu S, et al. Targeted therapy of atherosclerosis vulnerable plaque by ROS-scavenging nanoparticles and MR/fluorescence dual-modality imaging tracing. Int J Nanomedicine. (2022) 17:5413–29. doi: 10.2147/IJN.S371873
25. Saxena A, Ng EYK, Lim ST. Imaging modalities to diagnose carotid artery stenosis: progress and prospect. Biomed Eng Online. (2019) 18(1):66. doi: 10.1186/s12938-019-0685-7
26. Fedak A, Ciuk K, Urbanik A. Ultrasonography of vulnerable atherosclerotic plaque in the carotid arteries: b-mode imaging. J Ultrason. (2020) 20(81):e135–45. doi: 10.15557/JoU.2020.0022
27. Tschiderer L, Seekircher L, Izzo R, Mancusi C, Manzi MV, Baldassarre D, et al. Association of intima-media thickness measured at the common carotid artery with incident carotid plaque: individual participant data meta-analysis of 20 prospective studies. J Am Heart Assoc. (2023) 12(12):e027657. doi: 10.1161/JAHA.122.027657
28. Willeit P, Tschiderer L, Allara E, Reuber K, Seekircher L, Gao L, et al. Carotid intima-media thickness progression as surrogate marker for cardiovascular risk: meta-analysis of 119 clinical trials involving 100 667 patients. Circulation. (2020) 142(7):621–42. doi: 10.1161/CIRCULATIONAHA.120.046361
29. Golemati S, Patelaki E, Gastounioti A, Andreadis I, Liapis CD, Nikita KS. Motion synchronisation patterns of the carotid atheromatous plaque from B-mode ultrasound. Sci Rep. (2020) 10(1):11221. doi: 10.1038/s41598-020-65340-2
30. Cismaru G, Serban T, Tirpe A. Ultrasound methods in the evaluation of atherosclerosis: from pathophysiology to clinic. Biomedicines. (2021) 9(4):418. doi: 10.3390/biomedicines9040418
31. Yao Y, Zhang P. Novel ultrasound techniques in the identification of vulnerable plaques-an updated review of the literature. Front Cardiovasc Med. (2023) 10:1069745. doi: 10.3389/fcvm.2023.1069745
32. Huang S, Wu X, Zhang L, Wu J, He Y, Lai M, et al. Assessment of carotid plaque stability using contrast-enhanced ultrasound and its correlation with the expression of CD147 and MMP-9 in the plaque. Front Comput Neurosci. (2021) 15:778946. doi: 10.3389/fncom.2021.778946
33. Thayse K, Kindt N, Laurent S, Carlier S. VCAM-1 target in non-invasive imaging for the detection of atherosclerotic plaques. Biology (Basel). (2020) 9(11):368. doi: 10.3390/biology9110368
34. Davidhi A, Rafailidis V, Destanis E, Prassopoulos P, Foinitsis S. Ultrasound elastography: another piece in the puzzle of carotid plaque vulnerability? Med Ultrason. (2022) 24(3):356–63. doi: 10.11152/mu-3190
35. Di Leo N, Venturini L, de Soccio V, Forte V, Lucchetti P, Cerone G, et al. Multiparametric ultrasound evaluation with CEUS and shear wave elastography for carotid plaque risk stratification. J Ultrasound. (2018) 21(4):293–300. doi: 10.1007/s40477-018-0320-7
36. Galiuto L, Leccisotti L, Locorotondo G, Porto I, Burzotta F, Trani C, et al. Coronary plaque instability assessed by positron emission tomography and optical coherence tomography. Ann Nucl Med. (2021) 35(10):1136–46. doi: 10.1007/s12149-021-01651-2
37. MacAskill MG, Newby DE, Tavares AAS. Frontiers in positron emission tomography imaging of the vulnerable atherosclerotic plaque. Cardiovasc Res. (2019) 115(14):1952–62. doi: 10.1093/cvr/cvz162
38. Mushenkova NV, Summerhill VI, Zhang D, Romanenko EB, Grechko AV, Orekhov AN. Current advances in the diagnostic imaging of atherosclerosis: insights into the pathophysiology of vulnerable plaque. Int J Mol Sci. (2020) 21(8):2992. doi: 10.3390/ijms21082992
39. Bartlett B, Ludewick HP, Lee S, Verma S, Francis RJ, Dwivedi G. Imaging inflammation in patients and animals: focus on PET imaging the vulnerable plaque. Cells. (2021) 10(10):2573. doi: 10.3390/cells10102573
40. Vancheri F, Longo G, Vancheri S, Danial JSH, Henein MY. Coronary artery microcalcification: imaging and clinical implications. Diagnostics (Basel). (2019) 9(4):125. doi: 10.3390/diagnostics9040125
41. Fiz F, Morbelli S, Piccardo A, Bauckneht M, Ferrarazzo G, Pestarino E, et al. 18F-NaF uptake by atherosclerotic plaque on PET/CT imaging: inverse correlation between calcification density and mineral metabolic activity. J Nucl Med. (2015) 56(7):1019–23. doi: 10.2967/jnumed.115.154229
42. Wang D, Yao Y, Wang S, Zhang H, He ZX. The availability of the α7-nicotinic acetylcholine receptor in early identification of vulnerable atherosclerotic plaques: a study using a novel 18F-label radioligand PET. Front Bioeng Biotechnol. (2021) 9:640037. doi: 10.3389/fbioe.2021.640037
43. Derlin T, Sedding DG, Dutzmann J, Haghikia A, König T, Napp LC, et al. Imaging of chemokine receptor CXCR4 expression in culprit and nonculprit coronary atherosclerotic plaque using motion-corrected [68 ga]pentixafor PET/CT. Eur J Nucl Med Mol Imaging. (2018) 45(11):1934–44. doi: 10.1007/s00259-018-4076-2
44. Kircher M, Tran-Gia J, Kemmer L, Zhang X, Schirbel A, Werner RA, et al. Imaging inflammation in atherosclerosis with CXCR4-directed 68Ga-pentixafor PET/CT: correlation with 18F-FDG PET/CT. J Nucl Med. (2020) 61(5):751–6. doi: 10.2967/jnumed.119.234484
45. Li X, Samnick S, Lapa C, Israel I, Buck AK, Kreissl MC, et al. 68Ga-DOTATATE PET/CT for the detection of inflammation of large arteries: correlation with18F-FDG, calcium burden and risk factors. EJNMMI Res. (2012) 2(1):52. doi: 10.1186/2191-219X-2-52
46. Tarkin JM, Joshi FR, Evans NR, Chowdhury MM, Figg NL, Shah AV, et al. Detection of atherosclerotic inflammation by 68Ga-DOTATATE PET compared to [18F]FDG PET imaging. J Am Coll Cardiol. (2017) 69(14):1774–91. doi: 10.1016/j.jacc.2017.01.060
47. Rudd JH, Warburton EA, Fryer TD, Jones HA, Clark JC, Antoun N, et al. Imaging atherosclerotic plaque inflammation with [18F]-fluorodeoxyglucose positron emission tomography. Circulation. (2002) 105(23):2708–11. doi: 10.1161/01.cir.0000020548.60110.76
48. Gaemperli O, Shalhoub J, Owen DR, Lamare F, Johansson S, Fouladi N, et al. Imaging intraplaque inflammation in carotid atherosclerosis with 11C-PK11195 positron emission tomography/computed tomography. Eur Heart J. (2012) 33(15):1902–10. doi: 10.1093/eurheartj/ehr367
49. Dweck MR, Chow MW, Joshi NV, Williams MC, Jones C, Fletcher AM, et al. Coronary arterial 18F-sodium fluoride uptake: a novel marker of plaque biology. J Am Coll Cardiol. (2012) 59(17):1539–48. doi: 10.1016/j.jacc.2011.12.037
50. Silvola JMU, Li XG, Virta J, Marjamäki P, Liljenbäck H, Hytönen JP, et al. Aluminum fluoride-18 labeled folate enables in vivo detection of atherosclerotic plaque inflammation by positron emission tomography. Sci Rep. (2018) 8(1):9720. doi: 10.1038/s41598-018-27618-4
51. Beer AJ, Pelisek J, Heider P, Saraste A, Reeps C, Metz S, et al. PET/CT imaging of integrin αvβ3 expression in human carotid atherosclerosis. JACC Cardiovasc Imaging. (2014) 7(2):178–87. doi: 10.1016/j.jcmg.2013.12.003
52. Boswijk E, Bauwens M, Mottaghy FM, Wildberger JE, Bucerius J. Potential of α7 nicotinic acetylcholine receptor PET imaging in atherosclerosis. Methods. (2017) 130:90–104. doi: 10.1016/j.ymeth.2017.06.008
53. Chae SY, Kwon TW, Jin S, Kwon SU, Sung C, Oh SJ, et al. A phase 1, first-in-human study of 18F-GP1 positron emission tomography for imaging acute arterial thrombosis. EJNMMI Res. (2019) 9(1):3. doi: 10.1186/s13550-018-0471-8
54. Joshi FR, Manavaki R, Fryer TD, Figg NL, Sluimer JC, Aigbirhio FI, et al. Vascular imaging with 18F-fluorodeoxyglucose positron emission tomography is influenced by hypoxia. J Am Coll Cardiol. (2017) 69(14):1873–4. doi: 10.1016/j.jacc.2017.01.050
55. Pavillard E, Sewall L. A post-market, multi-vessel evaluation of the imaging of peripheral arteries for diagnostic purposeS comparing optical coherence tomogrApy and iNtravascular ultrasound imaging (SCAN). BMC Med Imaging. (2020) 20(1):18. doi: 10.1186/s12880-020-0420-7
56. Prati F, Romagnoli E, Gatto L, La Manna A, Burzotta F, Ozaki Y, et al. Relationship between coronary plaque morphology of the left anterior descending artery and 12 months clinical outcome: the CLIMA study. Eur Heart J. (2020) 41(3):383–91. doi: 10.1093/eurheartj/ehz520
57. Xing L, Higuma T, Wang Z, Aguirre AD, Mizuno K, Takano M, et al. Clinical significance of lipid-rich plaque detected by optical coherence tomography: a 4-year follow-up study. J Am Coll Cardiol. (2017) 69(20):2502–13. doi: 10.1016/j.jacc.2017.03.556
58. Fabris E, Berta B, Hommels T, Roleder T, Hermanides RS, Rivero F, et al. Long-term outcomes of patients with normal fractional flow reserve and thin-cap fibroatheroma. EuroIntervention. (2023) 18(13):e1099–107. doi: 10.4244/EIJ-D-22-00306
59. Luo X, Lv Y, Bai X, Qi J, Weng X, Liu S, et al. Plaque erosion: a distinctive pathological mechanism of acute coronary syndrome. Front Cardiovasc Med. (2021) 8:711453. doi: 10.3389/fcvm.2021.711453
60. Huang M, Maehara A, Tang D, Zhu J, Wang L, Lv R, et al. Comparison of multilayer and single-layer coronary plaque models on stress/strain calculations based on optical coherence tomography images. Front Physiol. (2023) 14:1251401. doi: 10.3389/fphys.2023.1251401
61. Ueki Y, Yamaji K, Losdat S, Karagiannis A, Taniwaki M, Roffi M, et al. Discordance in the diagnostic assessment of vulnerable plaques between radiofrequency intravascular ultrasound versus optical coherence tomography among patients with acute myocardial infarction: insights from the IBIS-4 study. Int J Cardiovasc Imaging. (2021) 37(10):2839–47. doi: 10.1007/s10554-021-02272-6
62. Calvert PA, Obaid DR, O’Sullivan M, Shapiro LM, McNab D, Densem CG, et al. Association between IVUS findings and adverse outcomes in patients with coronary artery disease: the VIVA (VH-IVUS in vulnerable atherosclerosis) study. JACC Cardiovasc Imaging. (2011) 4(8):894–901. doi: 10.1016/j.jcmg.2011.05.005
63. Stone GW, Maehara A, Lansky AJ, de Bruyne B, Cristea E, Mintz GS, et al. A prospective natural-history study of coronary atherosclerosis. N Engl J Med. (2011) 364(3):226–35. doi: 10.1056/NEJMoa1002358
64. Lee HS, Jeong JS. Triple-element back-to-back transducer with 3D printed housing for intravascular ultrasound imaging: a feasibility study. IEEE ACCESS. (2022) 10:9287–97. doi: 10.1109/ACCESS.2022.3144604
65. Villiger M, Otsuka K, Karanasos A, Doradla P, Ren J, Lippok N, et al. Coronary plaque microstructure and composition modify optical polarization: a new endogenous contrast mechanism for optical frequency domain imaging. JACC Cardiovasc Imaging. (2018) 11(11):1666–76. doi: 10.1016/j.jcmg.2017.09.023
66. Otsuka K, Villiger M, Karanasos A, van Zandvoort LJC, Doradla P, Ren J, et al. Intravascular polarimetry in patients with coronary artery disease. JACC Cardiovasc Imaging. (2020) 13(3):790–801. doi: 10.1016/j.jcmg.2019.06.015
67. Omatsu T, Sotomi Y, Kobayashi T, Hamanaka Y, Hirata A, Hirayama A, et al. Quantitative validation of the coronary angioscopic yellow plaque with lipid core burden Index assessed by intracoronary near-infrared spectroscopy. J Atheroscler Thromb. (2022) 29(3):362–9. doi: 10.5551/jat.60566
68. Waksman R, Di Mario C, Torguson R, Ali ZA, Singh V, Skinner WH, et al. Identification of patients and plaques vulnerable to future coronary events with near-infrared spectroscopy intravascular ultrasound imaging: a prospective, cohort study. Lancet. (2019) 394(10209):1629–37. doi: 10.1016/S0140-6736(19)31794-5
69. Dohi T, Maehara A, Moreno PR, Baber U, Kovacic JC, Limaye AM, et al. The relationship among extent of lipid-rich plaque, lesion characteristics, and plaque progression/regression in patients with coronary artery disease: a serial near-infrared spectroscopy and intravascular ultrasound study. Eur Heart J Cardiovasc Imaging. (2015) 16(1):81–7. doi: 10.1093/ehjci/jeu169
70. Trusinskis K, Knoka E, Caunite L, Karantajere M, Lapsovs M, Paeglite S, et al. What is hidden behind yellow pixels: from pathology to intravascular imaging of atherosclerotic plaque. Curr Atheroscler Rep. (2022) 24(2):97–108. doi: 10.1007/s11883-022-00990-3
71. Brugaletta S, Sabaté M. Assessment of plaque composition by intravascular ultrasound and near-infrared spectroscopy: from PROSPECT I to PROSPECT II. Circ J. (2014) 78(7):1531–9. doi: 10.1253/circj.cj-14-0496
72. Verjans JW, Osborn EA, Ughi GJ, Calfon Press MA, Hamidi E, Antoniadis AP, et al. Targeted near-infrared fluorescence imaging of atherosclerosis: clinical and intracoronary evaluation of indocyanine green. JACC Cardiovasc Imaging. (2016) 9(9):1087–95. doi: 10.1016/j.jcmg.2016.01.034
73. Calfon MA, Rosenthal A, Mallas G, Mauskapf A, Nudelman RN, Ntziachristos V, et al. In vivo near infrared fluorescence (NIRF) intravascular molecular imaging of inflammatory plaque, a multimodal approach to imaging of atherosclerosis. J Vis Exp. (2011) 54:2257. doi: 10.3791/2257
74. Htun NM, Chen YC, Lim B, Schiller T, Maghzal GJ, Huang AL, et al. Near-infrared autofluorescence induced by intraplaque hemorrhage and heme degradation as marker for high-risk atherosclerotic plaques. Nat Commun. (2017) 8(1):75. doi: 10.1038/s41467-017-00138-x
75. Zhang Y, Taylor E, Huang N, Hamilton J, Cheng JX. Survival intravascular photoacoustic imaging of lipid-rich plaque in cholesterol fed rabbits. Transl Biophotonics. (2022) 4(4):e202200012. doi: 10.1002/tbio.202200012
76. Lv R, Wang L, Maehara A, Matsumura M, Guo X, Samady H, et al. Combining IVUS+OCT data, biomechanical models and machine learning method for accurate coronary plaque morphology quantification and cap thickness and stress/strain Index predictions. J Funct Biomater. (2023) 14(1):41. doi: 10.3390/jfb14010041
77. Lv R, Maehara A, Matsumura M, Wang L, Wang Q, Zhang C, et al. Using optical coherence tomography and intravascular ultrasound imaging to quantify coronary plaque cap thickness and vulnerability: a pilot study. Biomed Eng Online. (2020) 19(1):90. doi: 10.1186/s12938-020-00832-w
78. Cao Y, Alloosh M, Sturek M, Cheng JX. Highly sensitive lipid detection and localization in atherosclerotic plaque with a dual-frequency intravascular photoacoustic/ultrasound catheter. Transl Biophotonics. (2020) 2(3):e202000004. doi: 10.1002/tbio.202000004
79. Muller J, Madder R. OCT-NIRS imaging for detection of coronary plaque structure and vulnerability. Front Cardiovasc Med. (2020) 7:90. doi: 10.3389/fcvm.2020.00090
80. Leng J, Zhang J, Li C, Shu C, Wang B, Lin R, et al. Multi-spectral intravascular photoacoustic/ultrasound/optical coherence tomography tri-modality system with a fully-integrated 0.9-mm full field-of-view catheter for plaque vulnerability imaging. Biomed Opt Express. (2021) 12(4):1934–46. doi: 10.1364/BOE.420724
81. Zhou M, Yu Y, Chen R, Liu X, Hu Y, Ma Z, et al. Wall shear stress and its role in atherosclerosis. Front Cardiovasc Med. (2023) 10:1083547. doi: 10.3389/fcvm.2023.1083547
82. Moerman AM, Korteland S, Dilba K, van Gaalen K, Poot DHJ, van Der Lugt A, et al. The correlation between wall shear stress and plaque composition in advanced human carotid atherosclerosis. Front Bioeng Biotechnol. (2022) 9:828577. doi: 10.3389/fbioe.2021.828577
83. Tomaniak M, Katagiri Y, Modolo R, de Silva R, Khamis RY, Bourantas CV, et al. Vulnerable plaques and patients: state-of-the-art. Eur Heart J. (2020) 41(31):2997–3004. doi: 10.1093/eurheartj/ehaa227
84. Bajraktari A, Bytyçi I, Henein MY. The relationship between coronary artery wall shear strain and plaque morphology: a systematic review and meta-analysis. Diagnostics (Basel). (2020) 10(2):91. doi: 10.3390/diagnostics10020091
85. Xu LS, Zhang YL, Meng L, Li S, Niu LL, Greenwald SE, et al. Ultrasound assessment of the relation between local hemodynamic parameters and plaque morphology. IEEE ACCESS. (2020) 8:145149–58. doi: 10.1109/ACCESS.2020.3014814
Keywords: atherosclerosis, vulnerable plaque, computed tomography, magnetic resonance, ultrasound, positron emission tomography, optical coherence tomography
Citation: Wang Z and Zhang P (2024) Novel imaging modalities for the identification of vulnerable plaques. Front. Cardiovasc. Med. 11:1450252. doi: 10.3389/fcvm.2024.1450252
Received: 17 June 2024; Accepted: 26 August 2024;
Published: 12 September 2024.
Edited by:
Kenichiro Otsuka, Osaka City University Graduate School of Medicine, JapanReviewed by:
Hirotoshi Ishikawa, Kashibaseiki Hospital, JapanYung-Chih Chen, Monash Medical Centre, Australia
Copyright: © 2024 Wang and Zhang. This is an open-access article distributed under the terms of the Creative Commons Attribution License (CC BY). The use, distribution or reproduction in other forums is permitted, provided the original author(s) and the copyright owner(s) are credited and that the original publication in this journal is cited, in accordance with accepted academic practice. No use, distribution or reproduction is permitted which does not comply with these terms.
*Correspondence: Pingyang Zhang, emhweTI4QDEyNi5jb20=
†These authors have contributed equally to this work