- Department of Cardiovascular Medicine, Wenzhou Central Hospital, Wenzhou, China
Matrine (MT) and Oxymatrine (OMT) are two natural alkaloids derived from plants. These bioactive compounds are notable for their diverse pharmacological effects and have been extensively studied and recognized in the treatment of cardiovascular diseases in recent years. The cardioprotective effects of MT and OMT involve multiple aspects, primarily including antioxidative stress, anti-inflammatory actions, anti-atherosclerosis, restoration of vascular function, and inhibition of cardiac remodeling and failure. Clinical pharmacology research has identified numerous novel molecular mechanisms of OMT and MT, such as JAK/STAT, Nrf2/HO-1, PI3 K/AKT, TGF-β1/Smad, and Notch pathways, providing new evidence supporting their promising therapeutic potential against cardiovascular diseases. Thus, this review aims to investigate the potential applications of MT and OMT in treating cardiovascular diseases, encompassing their mechanisms, efficacy, and safety, confirming their promise as lead compounds in anti-cardiovascular disease drug development.
1 Introduction
Cardiovascular diseases (CVDs) encompass a range of conditions affecting the heart and vessels (arteries, veins, and capillaries), often manifesting clinically as atherosclerosis, hypertension, heart failure, cardiomyopathy, arrhythmias, and myocardial infarction. With ongoing socioeconomic growth and population ageing, risk factors such as smoking, unhealthy diets, hypertension, dyslipidemia, diabetes, chronic kidney disease, metabolic syndrome, and high stress levels are poised to elevate the risk of CVDs (1, 2). CVDs are among the leading causes of mortality worldwide, with ischemic heart disease being the primary reason for cardiovascular-related deaths in both men and women (3).
To date, research into the mechanisms of cardiac diseases has been ongoing. The mechanisms are complex and interrelated, with different types of heart conditions involving varied pathological processes. Here are some of the primary heart diseases and their associated mechanisms. Atherosclerosis is a fundamental cause of many cardiac conditions. When ox-LDL enters the arteries, its oxidation triggers an inflammatory response, attracting immune cells like macrophages to accumulate and form plaques, causing narrowing or blockage of vessels and limiting blood flow to the myocardium (4, 5). Plaque rupture under strain can initiate the coagulation process, leading to thrombosis, which may rapidly obstruct the supplying arteries, causing myocardial ischemia and, in severe cases, myocardial infarction (6). Reperfusion following ischemia induces oxidative stress and inflammatory responses in cardiac cells, resulting in myocardial cell apoptosis (7). Myocardial ischemia, hypertension, and excessive death of cardiac cells alter the heart's composition and morphology, inducing ventricular remodeling, which may ultimately lead to heart failure (8). These mechanisms may manifest differently across various types of cardiovascular diseases and may interact, contributing to the development and progression of these conditions. Despite numerous therapeutic strategies for cardiovascular diseases, mortality rates remain high. Western medicines, such as statins, anticoagulants, beta-blockers, nitrates, and antithrombotic drugs, are the mainstay treatments for these patients (9). Despite substantial evidence of their efficacy in treating cardiovascular diseases, the potential for serious adverse effects limits the use of these drugs (10, 11). Consequently, there is an urgent need to develop alternative and complementary therapies for better management of cardiovascular diseases.
MT and OMT are natural alkaloids primarily extracted from the Fabaceae family, such as Sophora flavescens Ait., S. tonkinensis Gagnep, S. alopecuroides L., and S. viciifolia Hance (12). These medicinal plants have a long history of use in China and many East Asian countries. MT and OMT, due to their clinical efficacy and safety, have been developed into various formulations for clinical use in Asian countries (13, 14). Pharmacological research has identified MT and OMT as some of the most promising natural compounds for treating cardiovascular diseases. To date, there is no comprehensive review of MT and OMT's cardioprotective effects in PubMed or other relevant databases. Therefore, this article provides a timely and in-depth review of the therapeutic potential and molecular targets of MT and OMT in the treatment of cardiovascular diseases, aiming to further advance their development and application.
2 The basic characteristics of MT and OMT
MT (Figure 1A) is a quinolizidine alkaloid, characterized by two piperidine rings fused by sharing a nitrogen atom. There are two N atoms in the structure, one is tertiary amine N and the other is amide N. The molecular formula of the substance is C15H24N2O, and the molar mass is 248.37. MT pure product is a white powder with a unique bitter taste of alkaloids. The boiling point of the substance is 396.7°C, the melting point is 77°C, it can be dissolved in water, methanol, ethanol, benzene, chloroform and other polar solvents, and is slightly soluble in petroleum ether (15). This compound can be extracted from the roots, fruits, and other parts of its source plants through methods such as extraction and reflux. Notably, it cannot coexist with other alkaline substances; mixing them may trigger chemical reactions, leading to reduced or lost medicinal activity, or even the formation of unstable compounds or hazardous substances (16) (http://www.baidu.com, last accessed on 03/26/2024).
OMT (Figure 1B) is also a quinolizidine alkaloid, with the molecular formula C15H24N2O2 and a molar mass of 264.36. It has a boiling point of 497.19°C and a melting point of 197°C (17). Structurally, OMT is the N-oxide of MT, featuring semi-polar coordination bonds. Consequently, OMT has increased polarity, is more soluble in water and dissolves in chloroform, but is less soluble in solutions like ether and petroleum ether (18). Pure OMT appears brown or deep yellow, with its color deepening upon further oxidation. The extraction method of OMT is the same as that of MT. According to the different polarity, the extract can be extracted by ether, and MT is dissolved in the ether solution to achieve the separation of the two (http://www.baidu.com, last accessed on 03/26/2024).
3 Pharmacokinetics of MT and OMT
Pharmacokinetics is pivotal in drug development by elucidating a drug's absorption, distribution, metabolism, and excretion. Clinicians can choose the appropriate formulation, administration route, and dosing frequency based on pharmacokinetic outcomes to ensure maximal therapeutic efficacy. Furthermore, pharmacokinetics can assess metabolic pathways and byproducts, unveiling the drug's mechanism of action. Therefore, to enhance the development potential of MT and OMT as medicinal formulations, it is essential to further explore their absorption and clearance characteristics both in vivo and in vitro. Current studies suggested that the bioavailability of MT and OMT was relatively low, primarily due to their poor solubility and stability in the gastrointestinal tract, leading to low oral absorption rates. Moreover, they may undergo significant first-pass metabolism in the liver (i.e., extensive metabolism before reaching systemic circulation), further reducing their bioavailability (19, 20).
When MT soft capsules (100, 200, 400 mg) were orally administered in humans, the Tmax (time to reach maximum plasma concentration) was approximately 1.5 h, and the T1/2β (elimination half-life) was around 8 h, with neither Tmax nor t1/2β showing significant variation with dose changes (21). During intravenous infusion (6 mg/ml), the Tmax of MT was approximately 5.6 h, and the T1/2β was 9.5 h (22). When administered orally to SD rats, the absolute bioavailability of MT was approximately 17.1%, with tissue distribution in descending order of liver, spleen, lung, brain, and heart. The concentration in the liver was about 5 times that in plasma. However, MT metabolism did not occur through the liver's most common metabolic pathways, CYP and UGT (23). MT was absorbed through intestinal cells by passive diffusion, showing significant regional differences within the gut. Intestinal permeability was highest in the ileum (Pw = 6.18), followed by the colon (Pw = 2.07), duodenum (Pw = 0.61), and jejunum (Pw = 0.52). MT exhibited linear pharmacokinetics in humans (21, 24). A study indicated that MT inhibited HOCT1-mediated transport by 88% but did not affect hOCT3-mediated transport. This suggested potential drug interactions during hepatic and renal uptake mediated by HOCT1, as well as intestinal absorption through hOCT3 (25). Drug co-administration could alter MT's pharmacokinetics. ACAPHA, an anticancer drug composed of various herbs including Sophora flavescens Ait., showed markedly different pharmacokinetic properties compared to the same dose of pure MT. Initially, MT concentrations in ACAPHA were lower than in pure MT; however, 2 h post-administration, MT levels from ACAPHA surpassed those of pure MT in the kidneys, and after 4 h, in plasma and other tissues. The matrix effect and solubility differences within ACAPHA modified MT absorption (26).
Zhang et al. (27) observed that the Tmax of oral OMT (300, 400, 600 mg) in humans was approximately 1.2 h, with a T1/2β around 2.3 h. Additionally, during intravenous infusion (6 mg/ml), OMT's Tmax was 0.5 h, with a T1/2β of 2.2 h. Furthermore, the pharmacokinetic parameters of capsule and dispersible tablet formulations were similar, indicating that the dispersible tablet did not enhance the diffusion rate of OMT (28). After intravenous administration, the concentration-time distribution of OMT followed a two-compartment pharmacokinetic model. Furthermore, both OMT and MT exhibited low plasma protein binding rates (OMT: 4.80%–8.95%, MT: 5.10%–10.55%), ensuring their efficient permeability (22). P-glycoprotein (P-gp) was located on the epithelial cell membrane and was involved in the transport of many drugs. However, numerous absorption experiments based on Caco-2 cells indicated that P-gp may not play a significant role in the transport of OMT. The apparent permeability coefficient (Papp) of OMT in the absorptive direction was 6.39 × 10−6 cm/s, which was lower than its Papp value of 11.28 × 10−6 cm/s in the secretory direction, with a permeation rate constant of approximately 0.8. Rat intestinal perfusion experiments reveal that the absorption of OMT and MT did not exhibit concentration saturation, and inhibitors of P-glycoprotein, endocytosis, and metabolism did not affect their absorption (29, 30). These findings suggested that P-gp did not participate in the transport of OMT and MT, indicating that their absorption primarily involved passive diffusion mechanisms. The distribution of OMT in tissues followed the order of kidney/lung>muscle>intestine/stomach>heart/spleen>uterus>adipose/testes>brain/liver, which differs from that of MT (31). When OMT was used as a topical formulation, the method of local external application to the skin may not be suitable. Studies showed that during topical application, OMT and MT account for only 1% in the dermis, indicating that the low enzyme content in dermal cells hindered the decomposition of OMT. Therefore, although the skin barrier system impeded the penetration of OMT, the lack of enzymes in dermal cells may be a key factor limiting the efficacy of OMT (32). The primary metabolic pathways for OMT and MT involved renal metabolism, with approximately 50% of the parent drugs excreted in the urine (27). The main metabolites include oxysophocarpine, sophocarpine, 9,10-dihydro-sophocarpine, 3,4,7,8-dihydro-sophocarpine, and 14-hydroxy-matrine (33).
4 Application of MT and OMT in cardiovascular diseases
MT and OMT exhibited strong anti-cardiovascular disease effects in both in vivo and in vitro studies (Tables 1, 2). MT and OMT's cardioprotective effects involved multiple mechanisms. Firstly, they exerted antioxidant actions to reduce oxidative stress, lowering the risk of cardiovascular diseases. Secondly, they had significant anti-inflammatory properties, inhibiting the production of inflammation-related cytokines and mitigating the inflammatory response in cardiovascular conditions. Additionally, they offered protection to patients with cardiovascular diseases by improving vascular function, reducing blood pressure, and inhibiting cardiac remodeling.
4.1 Ischemic injury
Myocardial ischemia, caused by coronary artery narrowing, blockage, and its complications, can lead to severe conditions such as angina, myocardial infarction, and even sudden death. Arteriosclerosis and diabetes are among the factors that can induce myocardial ischemia. It is a leading cause of death and disability worldwide (90). During myocardial ischemia, the excitatory reflex of the sympathetic nervous system further increases myocardial oxygen consumption, exacerbating the adverse effects of myocardial ischemia (91). The primary regulatory neuroarc in nociceptive transmission during myocardial ischemia involved the SCG and DRG, marked by the overexpression of P2X3 receptors in sympathetic neurons (92). OMT reduced the expression of P2X3 receptors, inhibiting the exacerbation of sympathetic excitatory reflexes caused by P2X3 receptor-mediated nociceptive transmission in rat SCG and DRG neurons during myocardial ischemic injury (93, 94).
Ischemia-reperfusion (I/R) was a common intervention for myocardial ischemia, yet it could exacerbate cardiomyocyte death through various mechanisms, including oxidative stress, calcium overload, activation of pro-apoptotic pathways, and inflammatory responses (95, 96). However, clinically, few drugs effectively protected cardiomyocytes from I/R injury. Thus, investigating the potential mechanisms of MT and OMT on I/R could aid in mitigating its damage. During I/R, myocardial membrane integrity was compromised, resulting in the release of cardiac enzymes such as cTnI, CTnT, LDH, and CK-MB. OMT treatment significantly reversed these effects (97). I/R induced autophagy in cardiomyocytes, characterized by increased expression of autophagic markers LC-3II/LC-3I, Beclin-1, and Atg5. OMT reduced these responses and suppressed levels of inflammatory cytokines TNF-α, IL-6, and IL-8, maintaining autophagic homeostasis in cardiomyocytes (98). Another manifestation of I/R was the induction of cellular endoplasmic reticulum stress (ERS). Under ERS conditions, the expression of PERK, IRE1α, and ATF6 significantly increased, contributing to ROS production and apoptosis. Treatment with MT notably reduced the levels of these three markers in the peripheral blood of rats (97).
Furthermore, the JAK/STAT pathway was closely associated with I/R, where STAT family proteins were directly linked to apoptotic behavior (99). Downstream factor HSP70, proved to be a cardioprotective agent, could inhibit cardiomyocyte autophagy and prevent apoptosis upon activation (100, 101). MT, acting as a JAK/STAT agonist, simultaneously activated this pathway and enhanced HSP70 expression in I/R cardiomyocytes, reducing lactate dehydrogenase release, creatine kinase activity, and in vitro cardiomyocyte apoptosis. These effects are abrogated by the JAK2 inhibitor AG490 and HSP70 siRNA (34). Zhou et al. (102) identified high affinity and stable hydrogen bonding between MT and JAK2 through bioinformatics. Further investigations revealed that MT promotes the phosphorylation of JAK2 and STAT3 and the expression of anti-apoptotic proteins Bcl-xL and Bcl-2 in I/R cells. Additionally, activation of the AMPK/Sirt3 pathway constituted one of MT's mechanisms influencing I/R, where pathway upregulation revitalized cardiomyocytes, stabilized mitochondrial membrane potential, suppressed excessive oxidative reactions, and mitigated I/R-induced cardiac injury (35). Figure 2 shows the pharmacological mechanism of MT and OMT against myocardial ischemia.
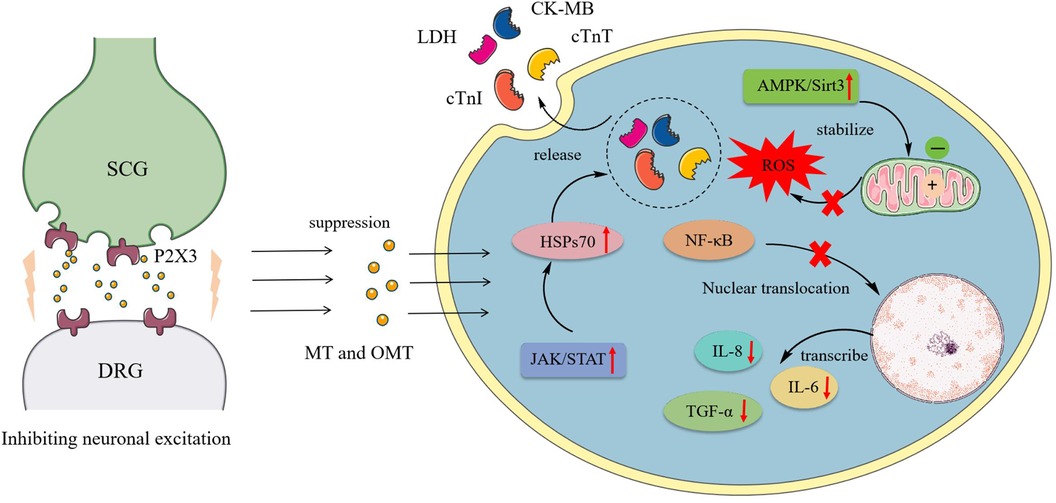
Figure 2 Pharmacological mechanism of MT and OMT against myocardial ischemia. SCG, Superior cervical ganglia; DRG, Dorsal root ganglia; LDH, Lactate dehydrogenase; CK-MB, Creatine kinase-MB; CTnT, Cardiac troponin T; CTnI, Cardiac troponin I; HSPs70, Heat shock proteins 70.
4.2 Cell damage
Cardiomyocytes encompass various functional cells such as myocardial cells, endothelial cells, and fibroblasts, whose synergistic actions sustain cardiac function. As non-regenerable terminally differentiated cells, their damage leads to irreversible cardiac dysfunction, thus triggering a range of cardiovascular diseases including angina, arrhythmias, and heart failure (103). Investigating the mechanisms of cardiomyocyte damage and identifying effective therapeutic drugs are crucial for both the theoretical and clinical management of cardiovascular diseases. Figure 3 shows the protective mechanism of MT and OMT on cardiac cells.
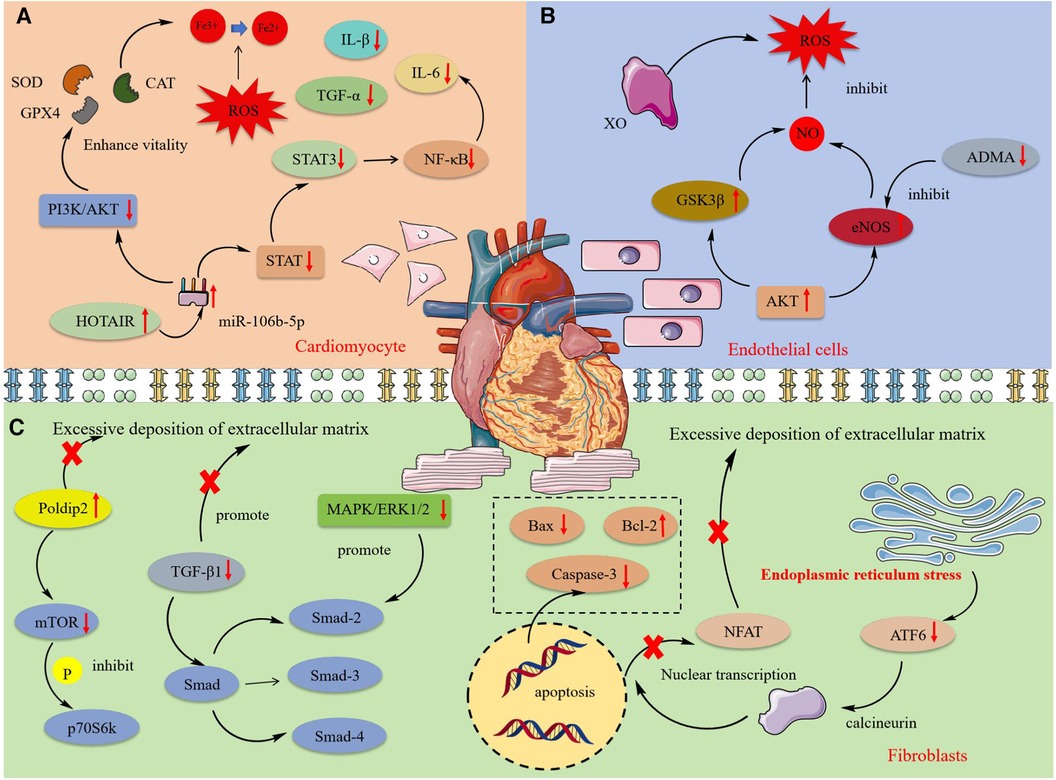
Figure 3 The mechanism of protective effect of MT and OMT on cardiac cells. SOD, Superoxide dismutase; CAT, Catalase; GPX4, Glutathione peroxidase 4; STAT, Signal transducer and activator of transcription; HOTAIR, HOX transcript antisense RNA; XO, Xanthine oxidase; Gsk3β, glycogen synthase kinase 3β; ADMA. Asymmetric dimethylarginine; eNOS, Endothelial nitric oxide synthase; Poldip2, polymerase delta interacting protein 2; p70S6 K, 70 kDa Ribosomal Protein S6 Kinase 2; ATF6, Activating transcription factor 6.
4.2.1 Myocardial cell injury
Myocardial cell damage was closely associated with oxidative reactions and inflammation. High glucose levels could lead to myocardial cell death, with oxidative stress and inflammatory response being key processes in its damage (104, 105). MT enhanced GSH-PX and CAT activity in H9C2 cardiomyocytes under high glucose or H2O2 conditions and inhibited the activation of inflammatory factors IL-1β, IL-6, and TNF-α mediated by the NF-κB inflammatory pathway, thereby suppressing oxidative and inflammatory responses in cardiomyocytes (48, 106). Experimental evidence suggested this effect may be related to the intervention in the expression of long non-coding RNA HOTAIR. In H9C2 cells under oxidative conditions, HOTAIR expression was significantly reduced, which was effectively reversed by MT treatment. HOTAIR negatively regulated the synthesis of miR-106b-5p, further inhibiting the activation of the PI3 K/AKT and STAT3 pathways, and thus preventing the production of reactive oxygen species and inflammatory factors (107). Additionally, the production of peroxides and lipid peroxidation led to the accumulation of iron ions, further stimulating the generation of reactive oxygen species and resulting in ferroptosis. This regulatable form of cell death was primarily influenced by GPX4 (108). Interestingly, recent studies increasingly report that ferroptosis played a significant role in the onset and progression of cardiovascular diseases. MT could reverse the peroxidation phenomenon in the process of cardiomyocyte ferroptosis, enhance SOD activity in cells, and promote GPX4 expression. This process coincided with the phosphorylation of p-PI3 K, PI3 K, p-AKT, AKT proteins and a decrease in the Bax/Bcl-2 ratio, confirming the PI3 K/AKT pathway as the upstream pathway through which MT inhibited ferroptosis and apoptosis (44). Additionally, OMT could improve mitochondrial membrane potential, inhibit the expression of pro-apoptotic proteins Caspase-3 and Bax, reduce inflammatory cell infiltration in cardiac tissue, and alleviate cardiomyocyte pyroptosis induced by high glucose and lipopolysaccharides (77, 78).
4.2.2 Cardiac endothelial cells
Cardiac endothelial cell damage was associated with excessive oxidative reactions. The production of reactive oxygen species mediated by the myocardial defense system, depletion of antioxidants, and imbalance of nitric oxide often led to oxidative stress responses in endothelial cells, further inducing mitochondrial dysfunction and damage to functional macromolecules, ultimately resulting in cell death (109). Increased XO activity was a significant factor in endothelial oxidative stress, with overexpression triggering the release of ROS (110). Studies confirmed that MT could improve endothelial dysfunction by regulating the ROS/NO balance. MT treatment restored the expression of Akt and eNOS in damaged ventricular cells induced by isoproterenol (ISO), reactivated glycogen synthase kinase 3b, reduced the levels of the eNOS inhibitor ADMA in serum, and maintained NO homeostasis (46).
4.2.3 Cardiac fibroblasts and fibrosis
Cardiac fibroblasts play a crucial role in maintaining the heart's basic structure and function and regulating cardiac remodeling under pathological conditions. During cardiovascular diseases, cardiac fibroblasts proliferate, are activated, and differentiate into contractile myofibroblasts. This differentiation leads to functional changes, including increased proliferation, the release of signaling molecules, and excessive accumulation of the extracellular matrix (ECM), ultimately resulting in cardiac fibrosis (111). Studies had demonstrated that MT and OMT significantly inhibited the malignant proliferation of cardiac fibroblasts. MT exhibited a dose-dependent inhibition of fibroblast proliferation induced by AngⅡ, with most cells arrested in the G1 phase of the cell cycle, a decrease in the Bcl-2/Bax ratio, and an increase in cleaved-caspase-3 activity, further promoting apoptosis of cardiac fibroblasts (55). TGF-β1 was a potent fibrogenic factor critical for the deposition of the extracellular matrix, particularly in the accumulation of type I and III collagen (112). Evidence indicated that MT and OMT significantly reduced the expression of TGF-β1 mRNA, preventing the entry of its downstream target Smad transcription complex (Smad-2, Smad-3, and Smad-4) into the nucleus to bind with specific target genes. This action inhibited collagen deposition in cardiac tissue, restored left ventricular function and compliance, and markedly improved ventricular remodeling in rats (57, 72, 73). Xu et al. (74) discovered that TGF-β1-induced hyperproliferation of cardiac myofibroblasts was associated with significant increases in phosphorylation of the MAPK/ERK1/2 pathway. Interestingly, this effect could be notably suppressed by both MAPK/ERK1/2 inhibitors and OMT. The MAPK/ERK1/2 pathway may be one of the mechanisms through which MT treats fibrosis. Additionally, experiments confirmed that activation of p38 in fibroblasts was sufficient to drive myofibroblast formation and collagen synthesis (113). MT could inhibit p38 activation by upregulating RPS5 expression, mitigating fibrosis remodeling and cardiac dysfunction induced by AB operation or ISO (58).
Studies had linked ERS to diabetic-induced cardiomyopathy fibrosis (114). Inducing ERS activated ATF6, which in turn activated calcineurin. This activated calcineurin engages in the nuclear transcription of the ECM gene expression inducer NFAT, leading to ECM build-up (115). Western blotting revealed that MT attenuated fibroblast calcium accumulation and NFAT activation by inhibiting the ATF6 signaling pathway, suppressing ECM synthesis, and restoring impaired cardiac compliance and function (56). Human coronary artery smooth muscle cells; (HCSMCs) exposed to advanced glycation end products (AGEs) were prone to phenotypic transformation, leading to increased ECM deposition (116). This mechanism was primarily characterized by reductions in MYH11 and ACTA2. Treatment with MT inhibited Notch signaling activation by suppressing the expression of Notch ligand DLL4, increased MYH11 and ACTA2 in HCSMCs, and reversed the AGEs-induced contractile-synthetic phenotypic transformation in HCSMCs (59). Additionally, Ma et al. (60) demonstrated that MT inhibited ECM accumulation by promoting Poldip2 expression, suppressing downstream mTOR protein expression, preventing p70S6k phosphorylation and nuclear translocation, a mechanism confirmed through Poldip2-specific inhibitory siRNA. Overall, MT and OMT effectively inhibited cardiac fibrosis, importantly involving multiple targets and pathways, highlighting their broad and diverse potential in fibrosis treatment. In summary, MT and OMT represent promising natural lead compounds for treating cardiac fibrosis.
4.3 Vascular cell injury and atherosclerosis
Atherosclerosis is an inflammatory disease and a leading cause of CVDs and stroke. The primary pathology occurs in blood vessels, characterized by the prolonged accumulation and transformation of lipids, inflammatory cells, smooth muscle cells, and necrotic cell debris beneath the endothelial monolayer in the intimal space, ultimately leading to cell damage (117). Figure 4 shows the mechanism of protective effect of MT and OMT on vascular cells.
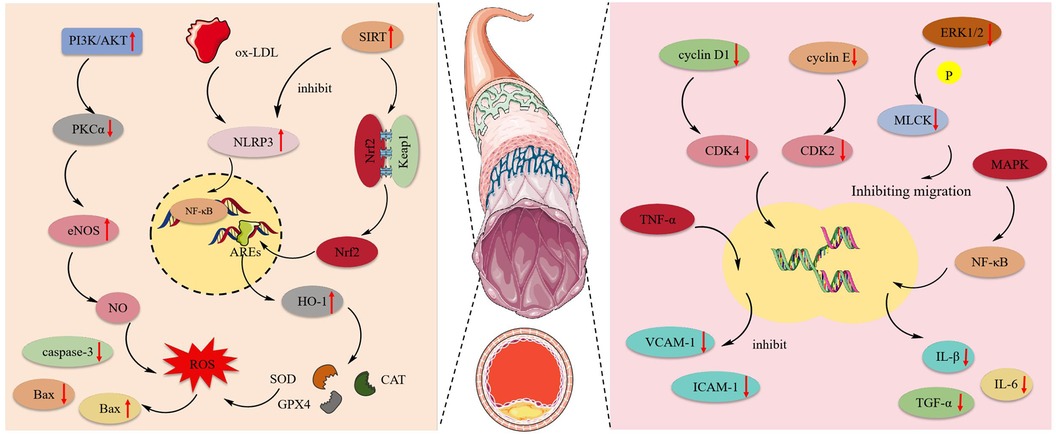
Figure 4 The mechanism of protective effect of MT and OMT on vascular cells. eNOS, Endothelial nitric oxide synthase; NLRP3, NLR family pyrin domain containing 3; ox-LDL, Low-density lipoprotein; HO-1, heme oxygenase-1; Nrf2, Transcription of nuclear factor erythroid 2-like 2; SOD, Superoxide dismutase; CAT, Catalase; GPX4, Glutathione peroxidase 4; MAPK, mitogen-activated protein kinase.
4.3.1 Vascular endothelium injury
MT had been shown to possess anti-apoptotic effects. By activating the JAK2/STAT3 pathway, it could reduce the expression of caspase-3 and Bax while increasing Bcl-2 expression in cardiac microvascular endothelial cells (CMECs). This promoted cell entry into the S phase and accelerated maturation (36). Human umbilical vein endothelial cells (HUVEC) exposed to ox-LDL were prone to activating NLRP3 inflammasome expression, inducing inflammation and oxidative reactions, leading to focal death. Notably, OMT could activate the SIRT pathway, inhibiting the expression of NLRP3, IL-1β, and IL-18. Additionally, the expression of the downstream target Nrf2 was also enhanced, which could suppress NLRP3-mediated focal death (118). Additionally, this target acts as a critical antioxidant factor, particularly in regulating HO-1 activation. It enhances the activity of SOD, CAT, and GSH-Px in HUVEC while suppressing the production of ROS and MDA (119). This suggests that the SIRT1/Nrf2 signaling pathway is an effective route through which OMT inhibits NLRP3 inflammasome-mediated pyroptosis (81). Furthermore, Zhang et al. (66) observed that ox-LDL disrupts the NO balance in HUVEC, leading to oxidative stress-induced endothelial cell death. MT, by activating the PI3 K/AKT pathway, stimulated the expression of downstream substrate eNOS and inversely inhibits the activation of the eNOS negative regulator PKCα, ultimately increasing NO synthesis. In the aortic endothelial cell apoptosis model induced by AGEs, MT activates the MAPK enzymes MKK3 and MKK6, promoting MAPK phosphorylation. This leads to the nuclear translocation of Nrf2 and its binding to ARE, triggering the transcription of antioxidant enzyme genes. Consequently, it suppresses the production of reactive oxygen species and inhibits endothelial cell apoptosis (65).
4.3.2 Aortic smooth muscle cell injury
Post-aortic injury commonly presented with intimal hyperplasia and luminal stenosis. This occurs due to the pathological stimulation or damage of blood vessels, where Vascular Smooth Muscle Cells (VSMCs) become abnormally activated. The expression of their differentiation marker genes was downregulated, leading to a de-differentiated state with enhanced proliferation and migration capabilities. This resulted in abnormal intimal proliferation, a key process contributing to the development and progression of various cardiovascular diseases such as atherosclerosis and pulmonary arterial hypertension (120, 121). MT could block the cell division cycle, which required the expression and activation of cyclins and CDK complexes. Briefly, the activation of cyclin D1/CDK4 and cyclin E/CDK2 drove cells into the G1 phase and phosphorylated the Rb complex, facilitating entry into the S phase (122). MT inhibited the expression of p53 and p21 in VSMCs, downregulated the levels of cyclin D1/CDK4, cyclin E/CDK2, and the phosphorylated Rb protein, thereby blocking the VSMC G1 phase (62). In addition to its anti-proliferative effects, MT also inhibited the migration of vascular smooth muscle cells under conditions of disturbed blood flow by suppressing the phosphorylation of ERK1/2 and MLCK (123). Atherosclerosis is a chronic inflammatory disease where the overexpression of adhesion molecules in vascular smooth muscle cells can lead to its onset (124). MT inhibited the expression of VCAM-1 and ICAM-1 in human aortic smooth muscle cells (HASMCs) induced by TNF-α. Moreover, post-MT treatment, there was significant suppression of the NF-κB inflammatory pathway and the MAPK pathway, along with a notable decrease in the levels of IL-1β, IL-6, TNF-α, and ROS. This indicated that MT mitigated atherosclerosis by reducing the expression of adhesion molecules in HASMCs through the inhibition of the NF-κB inflammatory pathway, MAPK pathway, and ROS production (64).
4.4 Ion balance and arrhythmias
Action potential duration (APD) was a key determinant of arrhythmias, influenced by various ion channel currents and transporters. The transient outward potassium current (Ito) serves as the primary repolarizing current in cardiac cells, and its amplitude reduction could lead to APD prolongation (125). The cardiac IK1 current was a strongly inwardly rectifying K+ selective current crucial for shaping the normal action potential, responsible for initial depolarization and final repolarization (126). Prolongation of APD further triggered Ca2+ influx and sarcoplasmic reticulum (SR) Ca2+ release, leading to cytotoxic Ca2+ overload. In response, cardiomyocytes may initiate protective mechanisms by downregulating mRNA and proteins associated with Ca2+ channels and accelerating their inactivation to reduce Ca2+ influx (127, 128). However, excessive activation of this response led to the clustered downregulation of L-type Ca2+ channels (ICa-L) in the atria, paradoxically increasing the risk of ventricular arrhythmias without showing beneficial effects on atrial fibrillation (129). Long-term administration of oral MT in rats with arrhythmias had demonstrated a reduced likelihood of myocardial infarction, restoring the expression of Ito/Kv4.2 and IK1/Kir2.1 proteins in K + channels. Moreover, there was a significant decrease in IKM3 density and an increase in ICa-L density, suggesting that MT ensured normal action potential duration in cardiomyocytes by maintaining the stability of myocardial K+ and Ca2+ channels, thereby alleviating arrhythmias (39, 40, 130). Ventricular remodeling following myocardial infarction often triggered ventricular arrhythmias, with reduced expression of Cx43 being a primary cause. Studies indicated that post-OMT treatment, the expression and phosphorylation levels of Cx43 in rat myocardial tissue significantly increased, effectively treating myocardial injury and arrhythmias (82).
The balance of intracellular Ca2+ concentration was vital for maintaining cardiac viability, affecting not only the rhythm balance as previously discussed but also closely associated with mitochondrial function, oxidative reactions, and apoptosis. Detrimental stimuli induced Ca2+ influx, altering mitochondrial membrane potential (MMP) and triggering caspase cascade activation, leading to cell death (131). Overexpression of RyR2 led to the efflux of endoplasmic calcium. MT maintained MMP stability and mitigated mitochondrial damage by enhancing the binding of FKBP12.6 to RyR2, reducing RyR2 activity (49). OMT reduced calpain activity and the content of its target tBID, diminished cytosolic Ca2+ concentration, inhibited cytochrome c release from mitochondria to the cytosol and caspase-3 activation, and prevented AIF translocation to the nucleus, thereby decreasing apoptosis (80).
4.5 Relieving heart failure
Heart failure represents the end stage of cardiac disease, with nearly all cardiovascular conditions ultimately leading to its onset. Clinically, heart failure is categorized into acute and chronic forms based on the rate of onset (132). Multiple studies have demonstrated the therapeutic potential of MT in animal models of heart failure. In a rat model of chronic heart failure established by ligation of the coronary artery, MT was found to enhance myocardial contractile function by reducing serum levels of AST, CPK, and LDH, and by inhibiting the β3-AR/eNOS pathway (68). In a rat model of heart failure induced by ISO, treatment with OMT and MT effectively improved ventricular dysfunction and myocardial hypertrophy, demonstrating inhibition of ventricular remodeling, oxidative stress, and inflammation biomarkers. Specifically, MT reduced serum levels of BNP (a heart failure biomarker), and facilitated COX-2 and Prostacyclin synthase coupling, thereby converting arachidonic acid into PGI2 (a cardioprotective factor) (88, 133, 134). Sui et al. (70) suggested that the cardioprotective effect of MT against heart failure might be associated with the inhibition of the RhoA/ROCK1 signaling pathway. Inhibition of this pathway significantly improved cardiac function and reduced myocardial fibrosis in rats. OMT attenuated inflammation by suppressing TLR4 levels, inhibiting the nuclear transcription activity of NF-κB, and deactivating the MAPK pathway, thereby reducing serum levels of TNF-α and IL-6 (89). ADMA, an inhibitor of NOS, promoted apoptosis, oxidative stress, and inflammation, and was a significant biomarker of heart failure (135). Post-treatment with OMT (25, 50, and 100 mg/kg for 14 days) in ISO-administered rats enhanced the expression of DDAH2, facilitating the degradation of ADMA, thereby reducing the levels of cardiac biomarkers BNP and cTn-I (69, 87). Additionally, OMT dose-dependently decreased the expression levels of neutrophil chemoattractant factors (CINC-2, CINC-3), thereby reducing inflammation (86).
4.6 Inhibiting ventricular remodeling
Ventricular remodeling refers to the pathological and physiological response involving changes in size, shape, wall thickness, and tissue structure of the ventricles, secondary to myocardial injury or increased load. This process is primarily associated with excessive ventricular pressure load, cardiomyocyte hypertrophy, myocardial thickening, fibrosis, and hemodynamics (136). In the aforementioned section, we discussed the therapeutic effects of MT and OMT on myocardial fibrosis, which further prevents the occurrence of ventricular hypertrophy. In animal models, MT and OMT have been shown to reduce the degree of ventricular remodeling. For instance, in acute myocardial infarction rabbit models, MT and OMT (1 mg/100 g/d, for 4 weeks) increased cardiac output, peak rate of left ventricular pressure change (dp/dtmax), and left ventricular end-systolic pressure (LVESP), while decreasing left ventricular end-diastolic pressure (LVEDP), thus stabilizing ventricular pressure (84). OMT (200 mg/kg/d) inhibited the decrease in dP/dtmax and the weight of the heart and left ventricle in rats undergoing cardiac remodeling, protecting the mitochondrial structure. Mechanistic studies revealed that MT suppressed the expression of IGF-1, TGF-β1 proteins, and mRNA, decreased the expression of FFA, LAC, Col I, Col III, GRP78, CHOP, and osteopontin, increased the ATP/AMP ratio and calreticulin expression, thereby reducing myocardial energy metabolism and decreasing cardiomyocyte apoptosis (67, 85). Existing research indicated that Angiotensin II (Ang II), the final active product of the Renin-angiotensin-aldosterone system (RAAS), was involved in cardiac remodeling by regulating cardiac contractility, cellular coupling, and electrical impulse propagation (137). Post-OMT treatment, there was a reduction in ACE expression in the myocardial tissue of hypertensive rats, thus decreasing the conversion of Ang I to Ang II and the deposition of type I and III collagen, alleviating ventricular remodeling (138). Phosphorylation of RhoA GTPase and its downstream ROCKs played a role in processes such as vascular smooth muscle contraction, stress fibre formation, cell migration, and blood pressure regulation (139, 140). OMT exerted an inhibitory effect on the RhoA/ROCK pathway, reducing the formation of adhesion factors and tension fibres, thus alleviating increased cardiac hypertrophy index and right ventricular remodeling in rats with monocrotaline-induced pulmonary arterial hypertension (83, 141).
4.7 Diabetic cardiomyopathy
The maintenance of blood glucose homeostasis relies on hepatic gluconeogenesis and glycogenolysis, as well as glucose uptake and utilization by peripheral tissues, facilitated by insulin. Insufficient insulin secretion can lead to hyperglycemia, resulting in diabetes (142). The global mortality rate of diabetes has rapidly increased in recent years, primarily due to its severe cardiovascular complications (114). Recently, natural compounds extracted from herbs have been found to have significant anti-diabetic cardiomyopathy effects, gaining widespread attention for their efficacy, lower side effects, and low cost (143). Recent studies showed that MT and OMT modulated various signaling pathways in diabetic heart disease, making them promising drugs for treating diabetic cardiomyopathy. In in vivo models, MT and OMT regulated oxidative stress and inflammation in DCM rats, offering protection. OMT reduces fasting blood glucose, GHb, NEFA, TC, TG, and LDL-c levels in diabetic rats while increasing serum insulin, liver and muscle glycogen, HDL-c, GLP-1, and muscle GLUT-4 content. Histological examination of the pancreas and liver shows that OMT protects islet structure and prevents liver disorganization (144). MT treatment inhibited the expression of two peroxisome proliferator-activated receptors (PPARβ, PPARγ1), and reduced ROS and MDA levels in cardiomyocytes of DCM rats, thus suppressing the activation of the TLR-4/MyD-88 pathway induced by oxidative stress, and decreased the expression of pro-apoptotic factors caspase-8 and caspase-3 (50, 51). MT attenuated oxidative stress by upregulating the expression of Nrf2 and HO-1 (52). Additionally, the peroxidation reaction in DCM rat cells played a critical role in the formation of atherosclerotic plaques by mediating pathological processes in related cell types such as vascular smooth muscle cells, endothelial cells, and macrophages (145). Oxidative stress was a primary trigger for the release of pro-inflammatory cytokines (146). MT inhibited the activation of the TLR4/STAT1 signaling pathway induced by AGEs/RAGE-mediated oxidative stress, promoted the expression of GPX1, and thereby suppressed M1 macrophage polarization (53). Furthermore, MT exerted potential anti-inflammatory effects by downregulating the TGF-β-induced PERK signaling pathway and suppressing levels of inflammatory factors TNF-α and IL-6 (51). Mitochondrial fission observed in DCM rat cardiomyocytes, which accelerated mitochondrial autophagy, was mitigated by MT through activation of Mfn2, facilitating the fusion of damaged mitochondria to preserve function (52). Furthermore, OMT, by activating the Nrf2/HO-1 pathway and inhibiting the JAK/STAT pathway, enhanced the activity of antioxidant enzymes such as glutathione, SOD, and catalase, and modulating the expression of inflammatory factors, thereby alleviating changes in blood glucose levels and left ventricular systolic pressure in type 2 diabetic rats (71).
4.8 Drug-induced cardiotoxicity
Isoproterenol (ISO), a β-adrenergic agonist used clinically for the treatment of conditions such as bronchial asthma, cardiac arrest, shock, and hypotension, can lead to myocardial energy depletion, increased myocardial oxygen consumption, and excessive release of oxygen free radicals, ultimately resulting in ischemic necrosis of the myocardium when administered in excess (147, 148). In the aforementioned sections, we discussed the therapeutic effects of MT and OMT on ISO-induced heart failure (68, 133). Clinical pharmacological research indicated that MT and OMT were effective in treating ISO-induced myocardial injury in rats. Oral administration of MT in rats increased the activity of SOD, CAT, and GSH-Px in plasma and myocardial tissue, reduced the content of the lipid peroxidation product malondialdehyde, inhibited the expression of the eNOS inhibitor ADMA, restored the expression of damaged ventricular Akt and eNOS proteins, and also restored Gsk3β activity (46, 47).
Doxorubicin (DOX), a broad-spectrum antitumor agent clinically utilized for various cancers, is substantially limited in its clinical application due to pronounced cardiotoxic side effects. It is reported that DOX's cardiotoxicity commonly manifests as dilated cardiomyopathy, accompanied by increased cardiomyocyte apoptosis, which can lead to severe heart failure (149). Studies indicated that DOX induced myocardial cell oxidative stress, characterized by increased CK-MB and LDH activities and ROS levels, and decreased SOD, CAT, and GSH-Px levels, resulting in myocardial tissue damage and apoptosis. These effects could be effectively reversed by MT and OMT (43, 150). Additionally, Hu et al. (43) found that the antioxidative effects of MT were associated with activating the AMPKα/UCP2 signaling pathway. Activation of this pathway reduced mitochondrial Ca2 + influx, thereby inhibiting ROS synthesis and mitochondrial death. In DOX-treated H9c2 cardiomyocytes, MT enhanced mitochondrial Na + -K + -ATPase and Ca2 + ATPase activities, improved mitochondrial membrane potential (MMP), and reduced apoptosis rates (42).
Aldosterone (ALD) induced myocardial cell injury through various mechanisms, including the phosphorylation of the MAPK signaling pathway (151). JNK protein, as a member of the MAPK family, played a crucial role in cellular stress responses. OMT restored normal morphology in cardiomyocytes by inhibiting the increase in ALD-induced p-JNK expression levels, without suppressing JNK mRNA expression. This suggested that OMT may only inhibit JNK phosphorylation without preventing its synthesis (76, 152). Moreover, OMT mitigated ALD-induced proliferation and migration of cardiac fibroblasts, and reduced levels of α-SMA, type I collagen, type III collagen, and CTGF, thereby attenuating cardiac fibrosis. Mechanistically, this regulatory effect relied on the dissociation of Nrf2-Keap1 and the nuclear translocation of Nrf2, a key modulator of antioxidant action (153).
Viral and bacterial infections can lead to shock and infectious myocarditis, ultimately causing symptoms such as difficulty breathing and acute heart failure (154). Sepsis, a systemic inflammatory response syndrome caused by the invasion of pathogens such as bacteria, identifies the heart as a primary target organ for induced organ dysfunction (155). OMT exhibited anti-inflammatory properties, attenuating the expression of NF-κB, TNF-α, and lipopolysaccharide-binding protein in the myocardial tissue of septic rats, as well as the activation of the upstream regulatory MAPK pathway, thereby reducing heart rate and improving ventricular pressure changes in infected rats (79). Reports suggested that miR-106b-5p could alleviate inflammation-induced cardiac dysfunction (156). With MT administration, the expression of miR-106b-5p was upregulated in cardiomyocytes, and the expression of its sponge protein PTENP1 was downregulated (45).
5 Clinical trials and safety
Presently, there is a scarcity of clinical application reports for MT and OMT in cardiovascular and cerebrovascular diseases. Sophora flavescens Ait. (SFA) is the principal source of MT and OMT. Formulations containing SFA, as well as traditional Chinese medicine compounds, have demonstrated beneficial effects in treating cardiovascular diseases. Clinical data from 59 breast cancer patients, showing cardiotoxicity due to chemotherapy, demonstrated that adjunct treatment with Compound SFA Injection effectively reduced myocardial enzyme levels and the incidence of electrocardiogram abnormalities (157). Modified Huanglian Wendan Decoction, comprising SFA and 12 additional Chinese herbs, had been clinically proven to effectively alleviate clinical symptoms in patients with ventricular premature contractions caused by hypertensive left ventricular hypertrophy, reducing the frequency of such contractions. It also normalized heart rate variability, thereby improving cardiac autonomic dysfunction (158). Mengshi Kushen Huanglian Decoction. a traditional Chinese medicine formula consisting of lapis chloriti, SFA, and Coptis chinensis Franch., had demonstrated significant efficacy in reducing the occurrence of ventricular premature beats and alleviating associated symptoms, as shown in a clinical trial (159). Furthermore, another clinical study had shown that the traditional Chinese medicine formula Sanshen Gansong Decoction, containing SFA among other herbs, could reduce triglyceride, total cholesterol, and ox-LDL levels in patients with coronary heart disease experiencing ventricular premature beats. It also revealed no adverse effects on liver function, renal function, or blood pressure during clinical use (160).
The survey indicated that there were 138 marketed drugs based on MT and OMT, primarily available as injectables. These were generally safe for clinical use, with toxicity manifesting only at elevated concentrations (http://www.da.yaozh.com, last accessed on 26/03/2024). MT at a concentration of 140 mg/ml could reduce cell viability, enhance the protein expression of CYP2A6, CYP2B6, and CYP3A4, and decrease levels of LDH and AST, indicating hepatotoxicity (161). MT (50, 100, 150, 200, and 250 mg/L) exhibited teratogenic and lethal effects on zebrafish embryos in a concentration-dependent manner, with EC50 and LC50 values of 145 and 240 mg/L, respectively (162). Additionally, various acute toxicity tests involving intravenous and oral administration in mice indicated that the LD50 of OMT was approximately 200 mg/kg. Symptoms in mice included increased respiration, reduced mobility, decreased activity, reduced levels of hepatic glutathione, and elevated hepatocyte apoptosis rates (163–165).
6 Discussion
Synthesizing past research, MT and OMT, as natural medicinal components, exert cardioprotective effects through various pharmacological actions. These include treating myocardial ischemia, cardiomyocyte injury, cardiac fibrosis, ventricular remodeling, heart failure, atherosclerosis, and diabetic cardiomyopathy, among others. MT and OMT feature multi-target, multi-pathway characteristics in cardiovascular disease treatment. MT mitigates cardiomyocyte apoptosis or autophagy by activating the JAK/STAT pathway, regulating the imbalance of pro-apoptotic and anti-apoptotic proteins, as well as inflammatory factors. Both MT and OMT reduce oxidative stress-induced cardiac cell dysfunction, primarily through the mitigation of excessive ROS production or accumulation, involving endoplasmic reticulum stress and ferroptosis responses. The expression of the Nrf2/HO-1 and PI3 K/AKT pathways has been commonly observed during pharmacological interventions. In the treatment of cardiac fibrosis, MT and OMT primarily exhibit an inhibitory effect on the TGF-β1/Smad pathway, thereby preventing the formation and deposition of the extracellular matrix (ECM) and the expression of inflammatory factors. The MAPK/ERK1/2 and Notch signaling pathways also play significant roles in this context. Additionally, they prevent atherosclerosis by eliminating inflammation, oxidative responses, and excessive proliferation in vascular cells. They also maintain normal action potential changes by regulating intracellular ion channels (K+, Ca2+) in cardiomyocytes, thus normalizing abnormal heart rates.
In current research, MT and OMT exhibit low bioavailability. Studies on modern formulations offer numerous benefits. Developing new formulations of MT and OMT can enhance their solubility and stability in the gastrointestinal tract. For instance, encapsulation in nano-carriers such as Covalent Organic Frameworks (166), liposomes (167), chitosan (168, 169), poly (lactide-co-glycolide) microspheres (170), microemulsions (171), and polymersomes (172) can effectively overcome their limitations. Additionally, the combination of MT and OMT with other drugs may improve their pharmacokinetic parameters (26).
Current research indicates that MT and OMT are generally considered safe at recommended doses. However, high doses may lead to adverse reactions such as indigestion and liver damage. Therefore, patients should consult healthcare professionals prior to using MT or OMT for cardiovascular diseases to ensure safety and optimal therapeutic outcomes.
In summary, MT and OMT are two promising natural alkaloid compounds for treating cardiac diseases, owing to their multifactorial actions such as anti-inflammatory, antioxidant, anti-apoptotic, and ion balance regulation. However, the discovery of their cardioprotective effects is primarily based on animal or cellular experimental models, with most corresponding clinical studies focusing on herbal formulations containing these components. This inevitably introduces certain objective limitations, insufficient to demonstrate their practicality and efficacy in clinical applications. Therefore, further research and clinical trials are necessary to better understand their mechanisms of action, optimize treatment regimens, and ensure safety. Future studies should also focus on exploring the optimal dosage of MT and OMT, their long-term effects, and the potential for combination with other therapeutic approaches.
Author contributions
SC: Writing – original draft, Writing – review & editing. SW: Data curation, Investigation, Writing – review & editing. BL: Funding acquisition, Supervision, Writing – review & editing.
Funding
The author(s) declare financial support was received for the research, authorship, and/or publication of this article.
This research was funded by Wenzhou Central Hospital.
Acknowledgments
All the authors of the manuscript thank and acknowledge their respective Universities and Institutes.
Conflict of interest
The authors declare that the research was conducted in the absence of any commercial or financial relationships that could be construed as a potential conflict of interest.
Publisher's note
All claims expressed in this article are solely those of the authors and do not necessarily represent those of their affiliated organizations, or those of the publisher, the editors and the reviewers. Any product that may be evaluated in this article, or claim that may be made by its manufacturer, is not guaranteed or endorsed by the publisher.
References
1. Yusuf S, Joseph P, Rangarajan S, Islam S, Mente A, Hystad P, et al. Modifiable risk factors, cardiovascular disease, and mortality in 155 722 individuals from 21 high-income, middle-income, and low-income countries (PURE): a prospective cohort study. Lancet. (2020) 395:795–808. doi: 10.1016/S0140-6736(19)32008-2
2. O’Sullivan JW, Raghavan S, Marquez-Luna C, Luzum JA, Damrauer SM, Ashley EA, et al. Polygenic risk scores for cardiovascular disease: a scientific statement from the American heart association. Circulation. (2022) 146:93–118. doi: 10.1161/CIR.0000000000001077
3. Eckle T, Bertazzo J, Khatua TN, Fatemi Tabatabaei SR, Moori Bakhtiari N, Walker LA, et al. Circadian influences on myocardial ischemia-reperfusion injury and heart failure. Circ Res. (2024) 134(6):675–94. doi: 10.1161/CIRCRESAHA.123.323522
4. Libby P. The changing landscape of atherosclerosis. Nature. (2021) 592:524–33. doi: 10.1038/s41586-021-03392-8
5. Frostegård J. Immunity, atherosclerosis and cardiovascular disease. BMC Med. (2013) 11:117. doi: 10.1186/1741-7015-11-117
6. Gibbons RJ. Myocardial ischemia in the management of chronic coronary artery disease: past and present. Circ Cardiovasc Imaging. (2021) 14:e011615. doi: 10.1161/CIRCIMAGING.120.011615
7. Zhao T, Wu W, Sui L, Huang Q, Nan Y, Liu J, et al. Reactive oxygen species-based nanomaterials for the treatment of myocardial ischemia reperfusion injuries. Bioact Mater. (2021) 7:47–72. doi: 10.1016/j.bioactmat.2021.06.006
8. Frantz S, Hundertmark MJ, Schulz-Menger J, Bengel FM, Bauersachs J. Left ventricular remodelling post-myocardial infarction: pathophysiology, imaging, and novel therapies. Eur Heart J. (2022) 43:2549–61. doi: 10.1093/eurheartj/ehac223
9. Leong DP, Joseph PG, McKee M, Anand SS, Teo KK, Schwalm JD, et al. Reducing the global burden of cardiovascular disease, part 2: prevention and treatment of cardiovascular disease. Circ Res. (2017) 121:695–710. doi: 10.1161/CIRCRESAHA.117.311849
10. Squizzato A, Bellesini M, Takeda A, Middeldorp S, Donadini MP. Clopidogrel plus aspirin versus aspirin alone for preventing cardiovascular events. Cochrane Database Syst Rev. (2017) 12:CD005158. doi: 10.1002/14651858.CD005158.pub4
11. Blessberger H, Kammler J, Domanovits H, Schlager O, Wildner B, Azar D, et al. Perioperative beta-blockers for preventing surgery-related mortality and morbidity. Cochrane Database Syst Rev. (2018) 3:CD004476. doi: 10.1002/14651858.CD004476.pub3
12. Sun XY, Jia LY, Rong Z, Zhou X, Cao LQ, Li AH, et al. Research advances on matrine. Front Chem. (2022) 10:867318. doi: 10.3389/fchem.2022.867318
13. Huan DQ, Hop NQ, Son NT. Oxymatrine: a current overview of its health benefits. Fitoterapia. (2023) 168:105565. doi: 10.1016/j.fitote.2023.105565
14. Long Y, Lin XT, Zeng KL, Zhang L. Efficacy of intramuscular matrine in the treatment of chronic hepatitis B. Hepatobiliary Pancreat Dis Int. (2004) 3:69–72.14969841
15. Chen X, Liu P, Mao Y, Wang G. Research advancements in pharmacological activities and mechanisms of matrine. Pharmacogn Mag. (2024) 20:189–205. doi: 10.1177/09731296231203458
16. Zhang C, Niu H, Wei M, Men X, Zhang Z. Study on the identification of sanweiquanshen koufuye. Chin J Ver Drug. (2023) 57:54–9.
17. Bai GY, Wang DQ, Ye CH, Liu ML. 1H And13c chemical shift assignments and stereochemistry of matrine and oxymatrine. Appl Magn Reson. (2002) 23:113–21. doi: 10.1007/BF03166189
18. Zhang L, Wei Y, Cao Z, Hui D, Yang F. Simultaneous determination of matrine and oxymatrine in danggui kushen pills by HPLC. Northwest Pharm J. (2008) 4:199–200.
19. Wu B. Modification Synthesis, Crystal Structure, and Insecticidal Activity of Matrine Derivatives. Guangzhou, Guangdong: Zhongkai College of Agricultural Engineering (2018).
20. Guo J, Zeng H, Chen Y. Research progress on the pharmacological effects of oxymatrine and its novel drug delivery system. Modern Trad Chin Med Mat Medica-World Sci Technol. (2022) 24:4633–43.
21. Zhang XL, Xu HR, Chen WL, Chu NN, Li XN, Liu GY, et al. Matrine determination and pharmacokinetics in human plasma using LC/MS/MS. J Chromatogr B Analyt Technol Biomed Life Sci. (2009) 877:3253–6. doi: 10.1016/j.jchromb.2009.08.026
22. Wu XL, Hang TJ, Shen JP, Zhang YD. Determination and pharmacokinetic study of oxymatrine and its metabolite matrine in human plasma by liquid chromatography tandem mass spectrometry. J Pharm Biomed Anal. (2006) 41:918–24. doi: 10.1016/j.jpba.2006.01.029
23. Yang Z, Gao S, Yin T, Kulkarni KH, Teng Y, You M, et al. Biopharmaceutical and pharmacokinetic characterization of matrine as determined by a sensitive and robust UPLC-MS/MS method. J Pharm Biomed Anal. (2010) 51:1120–7. doi: 10.1016/j.jpba.2009.11.020
24. Luo XJ, Xia BN. Study on the metabolic kinetics of matrine. J Guizho Med Univ. (1991) 2:180–3. doi: 10.19367/j.cnki.1000-2707.1991.02.026
25. Pan X, Wang L, Gründemann D, Sweet DH. Inhibition of human organic cation transporters by the alkaloids matrine and oxymatrine. Fitoterapia. (2014) 92:206–10. doi: 10.1016/j.fitote.2013.11.009
26. Gao G, Law FC. Physiologically based pharmacokinetics of matrine in the rat after oral administration of pure chemical and ACAPHA. Drug Metab Dispos. (2009) 37:884–91. doi: 10.1124/dmd.108.023788
27. Zhang MF, Shen YQ. Research advance on pharmacokinetics of oxymatrine. Drug Eval Res. (2020) 43:1903–11.
28. Wang C, Zhang MF, Shen YQ. Research progress on vascular pharmacologic effect of oxymatrine. Drug Eval Res. (2021) 44:1555–61.
29. Zhang H, Zhang W, Sun F, Yu J, Chai Y, Zhang G. Three alkaloids in radix so phorae flavescentis: metabolic rate in rat liver microsomes and absorption behavior in caco-2 cells. Acad J Nav Med Univ. (2015) 36:1167–72. doi: 10.3724/SP.J.1008.2015.1167
30. Zhang L, Feng ZQ, Chen XJ. Absorption characteristic of total alkaloids from Sophora flavescens and their monomers in caco-2 cell model. Chin Tradit Herb Drugs. (2012) 43:2464–7.
31. Liu J, Huang X, Xue H, Jiang Z, Zhang L, Ma S, et al. Effect of urea nitrogen cleaning fluid on renal function and expression of nephrin, MCP-1 and PAI-1 in rats with chronic renal failure. Pharmacol Clin Chin Mater Med. (2011) 27:74–8. doi: 10.13412/j.cnki.zyyl.2011.04.006
32. Zheng H, Chen G, Shi L, Lou Z, Chen F, Hu J. Determination of oxymatrine and its metabolite matrine in rat blood and dermal microdialysates by high throughput liquid chromatography/tandem mass spectrometry. J Pharm Biomed Anal. (2009) 49:427–33. doi: 10.1016/j.jpba.2008.11.032
33. Liu XH. Basic Study on Biopharmaceutics and Pharmacokinetics of Oxymatrine. Shenyang, Liaoning: Shenyang pharmaceutical university (2007).
34. Guo S, Gao C, Xiao W, Zhang J, Qu Y, Li J, et al. Matrine protects cardiomyocytes from ischemia/reperfusion injury by regulating HSP70 expression via activation of the JAK2/STAT3 pathway. Shock. (2018) 50:664–70. doi: 10.1097/SHK.0000000000001108
35. Lu Q, Lin X, Wu J, Wang B. Matrine attenuates cardiomyocyte ischemia-reperfusion injury through activating AMPK/Sirt3 signaling pathway. J Recept Signal Transduct Res. (2021) 41:488–93. doi: 10.1080/10799893.2020.1828914
36. Zhao XB, Qin Y, Niu YL, Yang J. Retraction notice to “matrine inhibits hypoxia/reoxygenation-induced apoptosis of cardiac microvascular endothelial cells in rats via the JAK2/STAT3 signaling pathway”. Biomed Pharmacother. (2023) 162:114721. doi: 10.1016/j.biopha.2023.114721
37. Cai B, Gong D, Chen N, Li J, Wang G, Lu Y, et al. The negative inotropic effects of homocysteine were prevented by matrine via the regulating intracellular calcium level. Int J Cardiol. (2011) 150:113–5. doi: 10.1016/j.ijcard.2011.04.031
38. Zhou Y, Wu Y, Deng L, Chen L, Zhao D, Lv L, et al. The alkaloid matrine of the root of Sophora flavescens prevents arrhythmogenic effect of ouabain. Phytomedicine. (2014) 21:931–5. doi: 10.1016/j.phymed.2014.02.008
39. Li X, Chu W, Liu J, Xue X, Lu Y, Shan H, et al. Antiarrhythmic properties of long-term treatment with matrine in arrhythmic rat induced by coronary ligation. Biol Pharm Bull. (2009) 32:1521–6. doi: 10.1248/bpb.32.1521
40. Zhou Y, Xu W, Han R, Zhou J, Pan Z, Rong H, et al. Matrine inhibits pacing induced atrial fibrillation by modulating I(KM3) and I(ca-L). Int J Biol Sci. (2012) 8:150–8. doi: 10.7150/ijbs.8.150
41. Tu YP, Hou YM, Jin XY, Ou YS, Zhang XY. Effect of matrine on left atrium high-frequency pacing rabbit atrial fibrillation model. Chin J Gerontol. (2019) 39:368–71.
42. Xu JY, Gu JL, Wang YQ, Wang Q, Lu JK. Protective effect of matrine on H9c2 cardiomyocyte injury induced by Adriamycin and its relationship with mi-tochondrial na+-K+-ATPase and Ca2+-ATPase. Chin J Hosp Pharm. (2019) 39:941–4. doi: 10.13286/j.cnki.chinhosppharmacyj.2019.09.12
43. Hu C, Zhang X, Wei W, Zhang N, Wu H, Ma Z, et al. Matrine attenuates oxidative stress and cardiomyocyte apoptosis in doxorubicin-induced cardiotoxicity via maintaining AMPKα/UCP2 pathway. Acta Pharm Sin B. (2019) 9:690–701. doi: 10.1016/j.apsb.2019.03.003
44. Xiao Y, Yu Y, Hu L, Yang Y, Yuan Y, Zhang W, et al. Matrine alleviates sepsis-induced myocardial injury by inhibiting ferroptosis and apoptosi. Inflammation. (2023) 46:1684–96. doi: 10.1007/s10753-023-01833-2
45. Liu Y, Liu L, Zhang J. Protective role of matrine in sepsis-associated cardiac dysfunction through regulating the lncRNA PTENP1/miR-106b-5p axis. Biomed Pharmacother. (2021) 134:111112. doi: 10.1016/j.biopha.2020.111112
46. Li X, Wang X, Guo Y, Deng N, Zheng P, Xu Q, et al. Regulation of endothelial nitric oxide synthase and asymmetric dimethylarginine by matrine attenuates isoproterenol-induced acute myocardial injury in rats. J Pharm Pharmacol. (2012) 64:1107–18. doi: 10.1111/j.2042-7158.2012.01502.x
47. Li X, Zhou R, Zheng P, Yan L, Wu Y, Xiao X, et al. Cardioprotective effect of matrine on isoproterenol-induced cardiotoxicity in rats. J Pharm Pharmacol. (2010) 62:514–20. doi: 10.1211/jpp.62.04.001
48. Hu C, Tang Q. Effect of matrine on high glucose induced injury of H9C2 cardiomyocytes. Chin J Geriatr Hear. (2020) 22:180–4.
49. Wang J, Tang Z, Zhang Y, Qiu C, Zhu L, Zhao N, et al. Matrine alleviates AGEs- induced cardiac dysfunctions by attenuating calcium overload via reducing ryanodine receptor 2 activity. Eur J Pharmacol. (2019) 842:118–24. doi: 10.1016/j.ejphar.2018.10.010
50. Liu ZW, Wang JK, Qiu C, Guan GC, Liu XH, Li SJ, et al. Matrine pretreatment improves cardiac function in rats with diabetic cardiomyopathy via suppressing ROS/TLR-4 signaling pathway. Acta Pharmacol Sin. (2015) 36:323–33. doi: 10.1038/aps.2014.127
51. Hou H, Zhang Q, Dong H, Ge Z. Matrine improves diabetic cardiomyopathy through TGF-β-induced protein kinase RNA-like endoplasmic reticulum kinase signaling pathway. J Cell Biochem. (2019) 120:13573–82. doi: 10.1002/jcb.28632
52. Xiao T, Huang J, Liu Y, Zhao Y, Wei M. Matrine protects cardiomyocytes against hyperglycemic stress by promoting mitofusin 2-induced mitochondrial fusion. Front Physiol. (2021) 11:597429. doi: 10.3389/fphys.2020.597429
53. Cui Q, Du H, Ma Y, Wang T, Zhu H, Zhu L, et al. Matrine inhibits advanced glycation end products-induced macrophage M1 polarization by reducing DNMT3a/b-mediated DNA methylation of GPX1 promoter. Eur J Pharmacol. (2022) 926:175039. doi: 10.1016/j.ejphar.2022.175039
54. Zhang S, Gui X, Ding Y, Tong H, Ju W, Li Y, et al. Matrine impairs platelet function and thrombosis and inhibits ROS production. Front Pharmacol. (2021) 12:717725. doi: 10.3389/fphar.2021.717725
55. Li Y, Wang B, Zhou C, Bi Y. Matrine induces apoptosis in angiotensin II-stimulated hyperplasia of cardiac fibroblasts: effects on bcl-2/bax expression and caspase-3 activation. Basic Clin Pharmacol Toxicol. (2007) 101:1–8. doi: 10.1111/j.1742-7843.2006.00040.x
56. Liu Z, Zhang Y, Tang Z, Xu J, Ma M, Pan S, et al. Matrine attenuates cardiac fibrosis by affecting ATF6 signaling pathway in diabetic cardiomyopathy. Eur J Pharmacol. (2017) 804:21–30. doi: 10.1016/j.ejphar.2017.03.061
57. Zhang Y, Cui L, Guan G, Wang J, Qiu C, Yang T, et al. Matrine suppresses cardiac fibrosis by inhibiting the TGF-β/smad pathway in experimental diabetic cardiomyopathy. Mol Med Rep. (2018) 17:1775–81. doi: 10.3892/mmr.2017.8054
58. Zhang X, Hu C, Zhang N, Wei WY, Li LL, Wu HM, et al. Matrine attenuates pathological cardiac fibrosis via RPS5/p38 in mice. Acta Pharmacol Sin. (2021) 42(4):573–84. doi: 10.1038/s41401-020-0473-8
59. Liu Z, Wang Y, Zhu H, Qiu C, Guan G, Wang J, et al. Matrine blocks AGEs- induced HCSMCs phenotypic conversion via suppressing Dll4-notch pathway. Eur J Pharmacol. (2018) 835:126–31. doi: 10.1016/j.ejphar.2018.07.051
60. Ma W, Xu J, Zhang Y, Zhang H, Zhang Z, Zhou L, et al. Matrine pre-treatment suppresses AGEs- induced HCSMCs fibrotic responses by regulating Poldip2/mTOR pathway. Eur J Pharmacol. (2019) 865:172746. doi: 10.1016/j.ejphar.2019.172746
61. Zhao L, Cai H, Tang Z, Cui Q, Liu Z, Lu S. Matrine suppresses advanced glycation end products-induced human coronary smooth muscle cells phenotype conversion by regulating endoplasmic reticulum stress-dependent notch signaling. Eur J Pharmacol. (2020) 882:173257. doi: 10.1016/j.ejphar.2020.173257
62. Zhu P, Chen JM, Chen SZ, Zhang C, Zheng SY, Long G, et al. Matrine inhibits vascular smooth muscle cell proliferation by modulating the expression of cell cycle regulatory genes. Acta Pharmacol Sin. (2010) 31:1329–35. doi: 10.1038/aps.2010.145
63. Zhu P, Chen JM, Guo HM, Fan XP, Zhang XS, Fan RX, et al. Matrine inhibits disturbed flow-enhanced migration via downregulation of ERK1/2-MLCK signaling vascular smooth muscle cells. Ann Vasc Surg. (2012) 26:268–75. doi: 10.1016/j.avsg.2011.10.006
64. Liu J, Zhang L, Ren Y, Gao Y, Kang L, Lu S. Matrine inhibits the expression of adhesion molecules in activated vascular smooth muscle cells. Mol Med Rep. (2016) 13:2313–9. doi: 10.3892/mmr.2016.4767
65. Liu Z, Lv Y, Zhang Y, Liu F, Zhu L, Pan S, et al. Matrine-type alkaloids inhibit advanced glycation End products induced reactive oxygen Species-mediated apoptosis of aortic endothelial cells in vivo and in vitro by targeting MKK3 and p38MAPK signaling. J Am Heart Assoc. (2017) 6:e007441. doi: 10.1161/JAHA.117.007441
66. Zhang S, Guo S, Gao XB, Liu A, Jiang W, Chen X, et al. Matrine attenuates high-fat diet-induced in vivo and ox-LDL-induced in vitro vascular injury by regulating the PKCα/eNOS and PI3K/akt/eNOS pathways. J Cell Mol Med. (2019) 23:2731–43. doi: 10.1111/jcmm.14180
67. Wei SJ, Zhou JW, Gan MY, Ma H, Wen EH, Dang HW. Improvement of matrine on the cardiac function and left ventricular remodeling in cardiac hypertrophy of rats. Chin J Clin Pharmacol Ther. (2017) 33:338–42. doi: 10.13699/j.cnki.1001-6821.2017.04.014
68. Yu J, Yang S, Wang X, Gan R. Matrine improved the function of heart failure in rats via inhibiting apoptosis and blocking β3-adrenoreceptor/endothelial nitric oxide synthase pathway. Mol Med Rep. (2014) 10:3199–204. doi: 10.3892/mmr.2014.2642
69. Zhang W, Qi X, Dai G, Wang T, Chang R, Xu X, et al. Matrine inhibits isoproterenol by regulating ADMA metabolic pathway. J Shaanxi Uni Chin Med. (2022) 45:109–13. doi: 10.13424/j.cnki.jsctcm.2022.05.021
70. Sun ZR, Shen YX, Ren YY. Effect of matrine on cardiac function in rats with heart failure. Chin J Clin Pharmacol Ther. (2021) 37:2003–200. doi: 10.13699/j.cnki.1001-6821.2021.15.014
71. Huang Y, He B, Song C, Long X, He J, Huang Y, et al. Oxymatrine ameliorates myocardial injury by inhibiting oxidative stress and apoptosis via the Nrf2/HO-1 and JAK/STAT pathways in type 2 diabetic rats. BMC Complement Med Ther. (2023) 23:2. doi: 10.1186/s12906-022-03818-4
72. Shen XC, Yang YP, Xiao TT, Peng J, Liu XD. Protective effect of oxymatrine on myocardial fibrosis induced by acute myocardial infarction in rats involved in TGF-β₁-smads signal pathway. J Asian Nat Prod Res. (2011) 13:215–24. doi: 10.1080/10286020.2010.550883
73. Fu L, Xu Y, Tu L, Huang H, Zhang Y, Chen Y, et al. Oxymatrine inhibits aldosterone-induced rat cardiac fibroblast proliferation and differentiation by attenuating smad-2,-3 and-4 expression: an in vitro study. BMC Complement Altern Med. (2016) 16:241. doi: 10.1186/s12906-016-1231-9
74. Xu Y, Xiao H, Luo H, Chen Y, Zhang Y, Tao L, et al. Inhibitory effects of oxymatrine on TGF-β1-induced proliferation and abnormal differentiation in rat cardiac fibroblasts via the p38MAPK and ERK1/2 signaling pathways. Mol Med Rep. (2017) 16:5354–62. doi: 10.3892/mmr.2017.7277
75. Ma J, Wu G, Xu J, Chang L, Li J. Effects of oxymatrine on myocardial repair in rats with acute myocardial infarction after adipose-derived mesenchymal stem cell transplantation. Chin J Immunol. (2020) 36:299–304.
76. Yang LQ, Sun AH, Xu YN, Zhang Y, Pan D, Chen Y, et al. Protective effect of oxymatrine against cardiac myocytes injury induced by aldosterone via MAPK signaling pathway. Chin J Exp Tradit Med Formulae. (2017) 23:130–5. doi: 10.13422/j.cnki.syfjx.2017150130
77. Yang X, Han X, Shen X. Effect of oxymatrine on oxidative stress injury of H9C2 cells induced by high glucose. Chin J Exp Tradit Med Formulae. (2020) 26:113–8. doi: 10.13422/j.cnki.syfjx.20200804
78. Gao G, Fang G, Fu L, Xu Y, Tao L, Liu T, et al. Improvement of oxymatrine on myocardial injury induced by lipopolysaccharide through inhibiting cardiomyocyte pyroptosis. J Chin Med Mater. (2022) 45:2961–7. doi: 10.13863/j.issn1001-4454.2022.12.031
79. Zhang M, Wang X, Bai B, Zhang R, Li Y, Wang Y. Oxymatrine protects against sepsis-induced myocardial injury via inhibition of the TNF-α/p38-MAPK/caspase-3 signaling pathway. Mol Med Rep. (2016) 14:551–9. doi: 10.3892/mmr.2016.5250
80. Xiao TT, Wang YY, Zhang Y, Bai CH, Shen XC. Similar to spironolactone, oxymatrine is protective in aldosterone-induced cardiomyocyte injury via inhibition of calpain and apoptosis-inducing factor signaling. PLoS One. (2014) 9:e88856. doi: 10.1371/journal.pone.0088856
81. Jin X, Fu W, Zhou J, Shuai N, Yang Y, Wang B. Oxymatrine attenuates oxidized low-density lipoprotein-induced HUVEC injury by inhibiting NLRP3 inflammasome-mediated pyroptosis via the activation of the SIRT1/Nrf2 signaling pathway. Int J Mol Med. (2021) 48:187. doi: 10.3892/ijmm.2021.5020
82. Qin GW, Li SN, Zhou T. Effect of oxymatrine on ventricular arrhythmia induced by acute myocardial infarction in rats. Chin J Clin. (2020) 14:917–21.
83. Li BP, Xu YP, Zeng ZL, Zeng ZL, Dai GD. Effects of oxymatrine combined with abesentan on right ventricular remodeling in rats with monocrotaline-induced pulmonary hypertension. Chin Tradit Pat Med. (2022) 44:223–6.
84. Wang XF, Liu XM. Effect of oxymatrine on cardiac function and left ventricular remodeling in rabbits after acute myocardial infarction. Chin J Appl Physiol. (2015) 31:123–6. doi: 10.13459/j.cnki.cjap.2015.02.008
85. Wang YS, Pan YF, Lv RF, Cui SL, Chen SH. Effect of oxymatrine on ventricular remodeling in mice with viral myocarditis. China J Mod Med. (2019) 29:1–7.
86. Xu QB, Xiong AQ, Yang YL, Xu YH, Zhang BT, Ma P. Effects of oxymatrine on the expression of myocardial tissue related factors in rats with congestive heart failure. J Huazhong Univ Sci Technol. (2018) 47:568–57.
87. Zhang W, Zhang J, Liu YK, Liu J, Wang X, Xu Q, et al. Cardioprotective effects of oxymatrine on isoproterenol-induced heart failure via regulation of DDAH/ADMA metabolism pathway in rats. Eur J Pharmacol. (2014) 745:29–35. doi: 10.1016/j.ejphar.2014.10.001
88. Zhou R, Xu Q, Xu Y, Xiong A, Wang Y, Ma P. Oxymatrine attenuated isoproterenol-induced heart failure in rats via regulation of COX-2/PGI2 pathway. Biomed Pharmacother. (2016) 84:1359–66. doi: 10.1016/j.biopha.2016.10.070
89. Sun H, Bai J, Sun Y, Zhen D, Fu D, Wang Y, et al. Oxymatrine attenuated isoproterenol-induced heart failure via the TLR4/NF-κB and MAPK pathways in vivo and in vitro. Eur J Pharmacol. (2023) 941:175500. doi: 10.1016/j.ejphar.2023.17550
90. Pagliaro BR, Cannata F, Stefanini GG, Bolognese L. Myocardial ischemia and coronary disease in heart failure. Heart Fail Rev. (2020) 25:53–65. doi: 10.1007/s10741-019-09831-z
91. Armour JA. Myocardial ischaemia and the cardiac nervous system. Cardiovasc Res. (1999) 4:41–54. doi: 10.1016/s0008-6363(98)00252-1
92. Huang MH, Horackova M, Negoescu RM, Wolf S, Armour JA. Polysensory response characteristics of dorsal root ganglion neurones that may serve sensory functions during myocardial ischaemia. Cardiovasc Res. (1996) 32:503–15. doi: 10.1016/S0008-6363(96)00108-3
93. Liang S, Xu C, Li G, Gao Y. P2x receptors and modulation of pain transmission: focus on effects of drugs and compounds used in traditional Chinese medicine. Neurochem Int. (2010) 57:705–12. doi: 10.1016/j.neuint.2010.09.004
94. Li G, Liu S, Zhang J, Yu K, Xu C, Lin J, et al. Increased sympathoexcitatory reflex induced by myocardial ischemic nociceptive signaling via P2X2/3 receptor in rat superior cervical ganglia. Neurochem Int. (2010) 56:984–90. doi: 10.1016/j.neuint.2010.04.010
95. Zhou H, Toan S. Pathological roles of mitochondrial oxidative stress and mitochondrial dynamics in cardiac microvascular ischemia/reperfusion injury. Biomolecules. (2020) 10:85. doi: 10.3390/biom10010085
96. Wang J, Toan S, Zhou H. Mitochondrial quality control in cardiac microvascular ischemia-reperfusion injury: new insights into the mechanisms and therapeutic potentials. Pharmacol Res. (2020) 156:104771. doi: 10.1016/j.phrs.2020.104771
97. Huang Y, Long X, Li X, Li S, He J. The role of oxymatrine in amelioration of acute lung injury subjected to myocardial I/R by inhibiting endoplasmic Reticulum stress in diabetic rats. Evid Based Complement Alternat Med. (2020) 2020:8836904. doi: 10.1155/2020/8836904
98. Xiong Z, Xu J, Liu X. Oxymatrine exerts a protective effect in myocardial ischemia/reperfusion-induced acute lung injury by inhibiting autophagy in diabetic rats. Mol Med Rep. (2021) 23:183. doi: 10.3892/mmr.2021.11822
99. McCormick J, Barry SP, Sivarajah A, Stefanutti G, Townsend PA, Lawrence KM, et al. Free radical scavenging inhibits STAT phosphorylation following in vivo ischemia/reperfusion injury. FASEB J. (2006) 20:2115–7. doi: 10.1096/fj.06-6188fje
100. Boengler K, Hilfiker-Kleiner D, Drexler H, Heusch G, Schulz R. The myocardial JAK/STAT pathway: from protection to failure. Pharmacol Ther. (2008) 120:172–85. doi: 10.1016/j.pharmthera.2008.08.002
101. Peng W, Zhang Y, Zheng M, Cheng H, Zhu W, Cao CM, et al. Cardioprotection by CaMKII-deltaB is mediated by phosphorylation of heat shock factor 1 and subsequent expression of inducible heat shock protein 70. Circ Res. (2010) 106:102–10. doi: 10.1161/CIRCRESAHA.109.210914
102. Zhou K, Chen H, Wang XY, Xu YM, Liao YF, Qin YY, et al. Targeted pharmacokinetics and bioinformatics screening strategy reveals JAK2 as the main target for Xin-ji-er-kang in treatment of MIR injury. Biomed Pharmacother. (2022) 155:113792. doi: 10.1016/j.biopha.2022.113792
103. Messaoudi S, Gravez B, Tarjus A, Pelloux V, Ouvrard-Pascaud A, Delcayre C, et al. Aldosterone-specific activation of cardiomyocyte mineralocorticoid receptor in vivo. Hypertension. (2013) 61:361–7. doi: 10.1161/HYPERTENSIONAHA.112.198986
104. Ruegsegger GN, Creo AL, Cortes TM, Dasari S, Nair KS. Altered mitochondrial function in insulin-deficient and insulin-resistant states. J Clin Invest. (2018) 128:3671–81. doi: 10.1172/JCI120843
105. Jia G, Hill MA, Sowers JR. Diabetic cardiomyopathy: an update of mechanisms contributing to this clinical entity. Circ Res. (2018) 122:624–38. doi: 10.1161/CIRCRESAHA.117.311586
106. Zhang Z, Qin X, Wang Z, Li Y, Chen F, Chen R, et al. Oxymatrine pretreatment protects H9c2 cardiomyocytes from hypoxia/reoxygenation injury by modulating the PI3K/akt pathway. Exp Ther Med. (2021) 21:556. doi: 10.3892/etm.2021.9988
107. Xu G, Zhang W, Wang Z, Chen M, Shi B. Matrine regulates H2O2-induced oxidative stress through long non-coding RNA HOTAIR/miR-106b-5p axis via AKT and STAT3 pathways. Biosci Rep. (2020) 40:BSR20192560. doi: 10.1042/BSR20192560
108. Zhou B, Zhang J, Chen Y, Liu Y, Tang X, Xia P, et al. Puerarin protects against sepsis-induced myocardial injury through AMPK-mediated ferroptosis signaling. Aging (Albany NY). (2022) 14:3617–32. doi: 10.18632/aging.204033
109. Nafisa A, Gray SG, Cao Y, Wang T, Xu S, Wattoo FH, et al. Endothelial function and dysfunction: impact of metformin. Pharmacol Ther. (2018) 192:150–62. doi: 10.1016/j.pharmthera.2018.07.007
110. Zhang Y, Hu S, Chen Y. Hepatocyte growth factor suppresses hypoxia/reoxygenation-induced XO activation in cardiac microvascular endothelial cells. Heart Vessels. (2015) 30:534–44. doi: 10.1007/s00380-014-0547-y
111. Travers JG, Kamal FA, Robbins J, Yutzey KE, Blaxall BC. Cardiac fibrosis: the fibroblast awakens. Circ Res. (2016) 118:1021–40. doi: 10.1161/CIRCRESAHA.115.306565
112. Kim KK, Sheppard D, Chapman HA. TGF-β1 Signaling and tissue fibrosis. Cold Spring Harb Perspect Biol. (2018) 10:a022293. doi: 10.1101/cshperspect.a022293
113. Molkentin JD, Bugg D, Ghearing N, Dorn LE, Kim P, Sargent MA, et al. Fibroblast-specific genetic manipulation of p38 mitogen-activated protein kinase in vivo reveals its central regulatory role in fibrosis. Circulation. (2017) 136:549–61. doi: 10.1161/CIRCULATIONAHA.116.026238
114. Lotfy M, Adeghate J, Kalasz H, Singh J, Adeghate E. Chronic complications of diabetes Mellitus: a Mini review. Curr Diabetes Rev. (2017) 13:3–10. doi: 10.2174/157339981266615101610162
115. Asadi F, Razmi A, Dehpour AR, Shafiei M. Tropisetron inhibits high glucose-induced calcineurin/NFAT hypertrophic pathway in H9c2 myocardial cells. J Pharm Pharmacol. (2016) 68:485–93. doi: 10.1111/jphp.12522
116. Tang Y, Yu S, Liu Y, Zhang J, Han L, Xu Z. MicroRNA-124 controls human vascular smooth muscle cell phenotypic switch via Sp1. Am J Physiol Heart Circ Physiol. (2017) 313:H641–9. doi: 10.1152/ajpheart.00660.2016
117. Duan H, Zhang Q, Liu J, Li R, Wang D, Peng W, et al. Suppression of apoptosis in vascular endothelial cell, the promising way for natural medicines to treat atherosclerosis. Pharmacol Res. (2021) 168:105599. doi: 10.1016/j.phrs.2021.105599
118. Gao Z, Sui J, Fan R, Qu W, Dong X, Sun D. Emodin protects against acute pancreatitis-associated lung injury by inhibiting NLPR3 inflammasome activation via Nrf2/HO-1 signaling. Drug Des Devel Ther. (2020) 14:1971–82. doi: 10.2147/DDDT.S247103
119. Zhang Q, Liu J, Duan H, Li R, Peng W, Wu C. Activation of Nrf2/HO-1 signaling: an important molecular mechanism of herbal medicine in the treatment of atherosclerosis via the protection of vascular endothelial cells from oxidative stress. J Adv Res. (2021) 34:43–63. doi: 10.1016/j.jare.2021.06.023
120. Alexander MR, Owens GK. Epigenetic control of smooth muscle cell differentiation and phenotypic switching in vascular development and disease. Annu Rev Physiol. (2012) 74:13–40. doi: 10.1146/annurev-physiol-012110-142315
121. Welt FG, Rogers C. Inflammation and restenosis in the stent era. Arterioscler Thromb Vasc Biol. (2002) 22:1769–76. doi: 10.1161/01.atv.0000037100.44766.5b
122. Engeland K. Cell cycle regulation: p53-p21-RB signaling. Cell Death Differ. (2022) 29:946–60. doi: 10.1038/s41418-022-00988-z
123. Feng X, Sureda A, Jafari S, Memariani Z, Tewari D, Annunziata G, et al. Berberine in cardiovascular and metabolic diseases: from mechanisms to therapeutics. Theranostics. (2019) 9:1923–51. doi: 10.7150/thno.30787
124. Bäck M, Hansson GK. Anti-inflammatory therapies for atherosclerosis. Nat Rev Cardiol. (2015) 12:199–211. doi: 10.1038/nrcardio.2015.5
125. Cordeiro JM, Calloe K, Aschar-Sobbi R, Kim KH, Korogyi A, Occhipinti D, et al. Physiological roles of the transient outward current ito in normal and diseased hearts. Front Biosci (Schol Ed). (2016) 8(1):143–59. doi: 10.2741/s454
126. Dhamoon AS, Jalife J. The inward rectifier current (IK1) controls cardiac excitability and is involved in arrhythmogenesis. Heart Rhythm. (2005) 2:316–24. doi: 10.1016/j.hrthm.2004.11.012
127. van der Velden HMW, van der Zee L, Wijffels MC, van Leuven C, Dorland R, Vos MA, et al. Atrial fibrillation in the goat induces changes in monophasic action potential and mRNA expression of ion channels involved in repolarization. J Cardiovasc Electrophysiol. (2000) 11:1262–9. doi: 10.1046/j.1540-8167.2000.01262.x
128. Yue L, Melnyk P, Gaspo R, Wang Z, Nattel S. Molecular mechanisms underlying ionic remodeling in a dog model of atrial fibrillation. Circ Res. (1999) 84:776–84. doi: 10.1161/01.res.84.7.776
129. Fareh S, Bénardeau A, Nattel S. Differential efficacy of L- and T-type calcium channel blockers in preventing tachycardia-induced atrial remodeling in dogs. Cardiovasc Res. (2001) 49:762–70. doi: 10.1016/s0008-6363(00)00288-1
130. Cao YG, Jing S, Li L, Gao JQ, Shen ZY, Liu Y, et al. Antiarrhythmic effects and ionic mechanisms of oxymatrine from Sophora flavescens. Phytother Res. (2010) 24:1844–9. doi: 10.1002/ptr.3206
131. Rodrigues FP, Pestana CR, Dos Santos GA, Pardo-Andreu GL, Santos AC, Uyemura SA, et al. Characterization of the stimulus for reactive oxygen species generation in calcium-overloaded mitochondria. Redox Rep. (2011) 16:108–13. doi: 10.1179/1351000211Y.0000000001
132. McDonagh TA, Metra M, Adamo M, Gardner RS, Baumbach A, Böhm M, et al. 2021 ESC guidelines for the diagnosis and treatment of acute and chronic heart failure: developed by the task force for the diagnosis and treatment of acute and chronic heart failure of the European Society of Cardiology (ESC) with the special contribution of the heart failure association (HFA) of the ESC. Rev Esp Cardiol (Engl Ed). (2022) 75:523. doi: 10.1016/j.rec.2022.05.005 35636830
133. Francois H, Athirakul K, Howell D, Dash R, Mao L, Kim HS, et al. Prostacyclin protects against elevated blood pressure and cardiac fibrosis. Cell Metab. (2005) 2:201–7. doi: 10.1016/j.cmet.2005.08.005
134. Xiao CY, Hara A, Yuhki K, Fujino T, Ma H, Okada Y, et al. Roles of prostaglandin I (2) and thromboxane A(2) in cardiac ischemia-reperfusion injury: a study using mice lacking their respective receptors. Circulation. (2001) 104:2210–5. doi: 10.1161/hc4301.098058
135. De Gennaro, Colonna V, Bianchi M, Pascale V, Ferrario P, Morelli F, Pascale W, et al. Asymmetric dimethylarginine (ADMA): an endogenous inhibitor of nitric oxide synthase and a novel cardiovascular risk molecule. Med Sci Monit. (2009) 15:91–101.
136. Hill BG, Schulze PC. Insights into metabolic remodeling of the hypertrophic and failing myocardium. Circ Heart Fail. (2014) 7:874–6. doi: 10.1161/CIRCHEARTFAILURE.114.001803
137. Wang PZ, Wen SN, Bai R. Research progress of myocardial renin-angiotensin-aldosterone system. Zhonghua Xin Xue Guan Bing Za Zhi. (2019) 47:585–9. doi: 10.3760/cma.j.issn.0253-3758.2019.07.016
138. Huang XY, Chen CX. Effect of oxymatrine, the active component from radix Sophorae flavescentis (kushen), on ventricular remodeling in spontaneously hypertensive rats. Phytomedicine. (2013) 20:202–12. doi: 10.1016/j.phymed.2012.10.012
139. Tang L, Dai F, Liu Y, Yu X, Huang C, Wang Y, et al. Rhoa/ROCK signaling regulates smooth muscle phenotypic modulation and vascular remodeling via the JNK pathway and vimentin cytoskeleton. Pharmacol Res. (2018) 133:201–12. doi: 10.1016/j.phrs.2018.05.011
140. Ikeda S, Satoh K, Kikuchi N, Miyata S, Suzuki K, Omura J, et al. Crucial role of rho-kinase in pressure overload-induced right ventricular hypertrophy and dysfunction in mice. Arterioscler Thromb Vasc Biol. (2014) 34:1260–71. doi: 10.1161/ATVBAHA.114.303320
141. Li YP, Xu YP, Wei CQ, Li ZJ, Dai GD. Oxymatrine inhibits monocrotaline-induced right ventricular hypertrophy in rats by regulating RhoA/ROCK1/COX2/PGIS signaling pathway. Mod Tradit Chin Med Mat Med-World Sci Technology. (2022) 24:4031–7.
142. Mayfield J. Diagnosis and classification of diabetes mellitus: new criteria. Am Fam Physician. (1998) 58:1355–70.9803200
143. Grover JK, Yadav S, Vats V. Medicinal plants of India with anti-diabetic potential. J Ethnopharmacol. (2002) 81:81–100. doi: 10.1016/s0378-8741(02)00059-4
144. Guo C, Zhang C, Li L, Wang Z, Xiao W, Yang Z. Hypoglycemic and hypolipidemic effects of oxymatrine in high-fat diet and streptozotocin-induced diabetic rats. Phytomedicine. (2014) 21:807–14. doi: 10.1016/j.phymed.2014.02.007
145. Nowak WN, Deng J, Ruan XZ, Xu Q. Reactive oxygen Species generation and atherosclerosis. Arterioscler Thromb Vasc Biol. (2017) 37:41–52. doi: 10.1161/ATVBAHA.117.309228
146. Sharma A, Tate M, Mathew G, Vince JE, Ritchie RH, de Haan JB. Oxidative stress and NLRP3-inflammasome activity as significant drivers of diabetic cardiovascular complications: therapeutic implications. Front Physiol. (2018) 9:114. doi: 10.3389/fphys.2018.00114
147. Kossack M, Hein S, Juergensen L, Siragusa M, Benz A, Katus HA, et al. Induction of cardiac dysfunction in developing and adult zebrafish by chronic isoproterenol stimulation. J Mol Cell Cardiol. (2017) 108:95–105. doi: 10.1016/j.yjmcc.2017.05.011
148. Zhang N, Zhang Y, Qian H, Wu S, Cao L, Sun Y. Selective targeting of ubiquitination and degradation of PARP1 by E3 ubiquitin ligase WWP2 regulates isoproterenol-induced cardiac remodeling. Cell Death Differ. (2020) 27:2605–19. doi: 10.1038/s41418-020-0523-2
149. Carvalho FS, Burgeiro A, Garcia R, Moreno AJ, Carvalho RA, Oliveira PJ. Doxorubicin-induced cardiotoxicity: from bioenergetic failure and cell death to cardiomyopathy. Med Res Rev. (2014) 34:106–35. doi: 10.1002/med.21280
150. Zhang YY, Yi M, Huang YP. Oxymatrine ameliorates doxorubicin-induced cardiotoxicity in rats. Cell Physiol Biochem. (2017) 43:626–35. doi: 10.1159/000480471
151. Kojonazarov B, Novoyatleva T, Boehm M, Happe C, Sibinska Z, Tian X, et al. P38 MAPK inhibition improves heart function in pressure-loaded right ventricular hypertrophy. Am J Respir Cell Mol Biol. (2017) 57:603–14. doi: 10.1165/rcmb.2016-0374OC
152. Chen Y, Wen JM, Xu YN, Zhang YY, Luo H, Tao L, et al. Protective effects of oxymatrine on aldosterone-induced cardiomyocyte injury. Chin J Exp Tradit Med Formulae. (2016) 22(19):76–80. doi: 10.13422/j.cnki.syfjx.2016190076
153. Yang Y, Chen S, Tao L, Gan S, Luo H, Xu Y, et al. Inhibitory effects of oxymatrine on transdifferentiation of neonatal rat cardiac fibroblasts to myofibroblasts induced by aldosterone via Keap1/Nrf2 signaling pathways in vitro. Med Sci Monit. (2019) 25:5375–88. doi: 10.12659/MSM.915542
154. Lasrado N, Reddy J. An overview of the immune mechanisms of viral myocarditis. Rev Med Virol. (2020) 30:1–14. doi: 10.1002/rmv.2131
155. Nowak RM, Nanayakkara P, DiSomma S, Levy P, Schrijver E, Huyghe R, et al. Noninvasive hemodynamic monitoring in emergency patients with suspected heart failure, sepsis and stroke: the PREMIUM registry. West J Emerg Med. (2014) 15:786–94. doi: 10.5811/westjem.2014.8.21357
156. An Z, Yang G, Nie W, Ren J, Wang D. MicroRNA-106b overexpression alleviates inflammation injury of cardiac endothelial cells by targeting BLNK via the NF-κB signaling pathway. J Cell Biochem. (2018) 119:3451–63. doi: 10.1002/jcb.26517
157. Xu Y, Liu Y, Sun A, Huang F, Gao X, Xu W. Preventive effect of compound matrine injection on Adriamycin-induced cardiotoxicity. China Pharm. (2015) 24:25–6.
158. Cao J. The Clinical Efficacy of Jiawei Huanglian Wendan Decoction in the Treatment of Patients with Ventricular Premature Contraction (Phlegm-Fire Disturbing Heart Syndrome) Caused by Hypertensive Left Ventricular Hypertrophy and its Effect on Heart Rate Turbulence. Chengdu, Sichuan: Chengdu university of traditional chinese medicine (2018).
159. Xia Z. The Clinical Observation of Mengshi Kusheng Huanglian Decoction by Enema the Patients of Premature Ventricular Complexes of Phlegm-Fire Disturbing Heart Syndrome. Harbin, Heilongjiang: Heilongjiang Academy of Chinese Medical Sciences (2018).
160. Cheng A. Clinical Observation of Sanshen Gansong Decoction in the Treatment of Ventricular Premature Beat of Coronary Heart Disease (Qi and Yin Deficiency Syndrome). Harbin, Heilongjiang: Heilongjiang university of Chinese medicine (2022). doi: 10.27127/d.cnki.ghlzu.2022.000284
161. Gong X, Gao Y, Guo G, Vondran FW, Schwartlander R, Efimova E, et al. Effect of matrine on primary human hepatocytes in vitro. Cytotechnology. (2015) 67:255–65. doi: 10.1007/s10616-013-9680-1
162. Lu ZG, Li MH, Wang JS, Wei DD, Liu QW, Kong LY. Developmental toxicity and neurotoxicity of two matrine-type alkaloids, matrine and sophocarpine, in Zebrafish (Danio Rerio) embryos/larvae. Reprod Toxicol. (2014) 47:33–41. doi: 10.1016/j.reprotox.2014.05.015
163. Dai WH, Qian LW, Wang LL, Yang SY, Zhou GQ. Toxicity studies in mice of matrine and oxymatrine. Anhui Med Pharm J. (2012) 16:904–5.
164. Jin SJ, Jiang YS, Zhou R, Liu LJ. Acute toxicity of sodium carbenoxolone and kushenin inclusion complex and its protective effect on CCl4 liver injury in mice. J Ningxia Med Univ. (2005) 4:272–3.
165. Guo QP, Jin RM. Comparison of hepatotoxicity induced by matrine and oxymatrine in mice. Chin J Pharmacol Toxicol. (2016) 30:736–40.
166. Huang C, Zhou S, Chen C, Wang X, Ding R, Xu Y, et al. Biodegradable redox-responsive AIEgen-based-covalent organic framework nanocarriers for long-term treatment of myocardial ischemia/reperfusion injury. Small. (2022) 18:e2205062. doi: 10.1002/smll.202205062
167. Liu M, Jin S, Yan H, Du S. Effect of oxymatrine HSPC liposomes on improving bioavailability, liver target distribution and hepatoprotective activity of oxymatrine. Eur J Pharm Sci. (2017) 104:212–20. doi: 10.1016/j.ejps.2017.03.048
168. Liu Y, Chen L, Zhou C, Yang J, Hou Y, Wang W. Development and evaluation of alginate-chitosan gastric floating beads loading with oxymatrine solid dispersion. Drug Dev Ind Pharm. (2016) 42:456–63. doi: 10.3109/03639045.2015.1088866
169. Zhang LL, Li P, Li YM, Wang AQ. Preparation and characterization of magnetic alginate-chitosan hydrogel beads loaded matrine. Drug Dev Ind Pharm. (2012) 3:872–82. doi: 10.3109/03639045.2011.630397
170. Wang W, Cai Y, Zhang G, Liu Y, Sui H, Park K, et al. Sophoridine-loaded PLGA microspheres for lung targeting: preparation, in vitro, and in vivo evaluation. Drug Deliv. (2016) 23:3674–80. doi: 10.1080/10717544.2016.1223210
171. Cao FH, OuYang WQ, Wang YP, Yue PF, Li SP. A combination of a microemulsion and a phospholipid complex for topical delivery of oxymatrine. Arch Pharm Res. (2011) 34:551–62. doi: 10.1007/s12272-011-0405-8
172. Yang J, Hou Y, Ji G, Song Z, Liu Y, Dai G, et al. Targeted delivery of the RGD-labeled biodegradable polymersomes loaded with the hydrophilic drug oxymatrine on cultured hepatic stellate cells and liver fibrosis in rats. Eur J Pharm Sci. (2014) 52:180–90. doi: 10.1016/j.ejps.2013.11.017
Glossary
ADMA, Asymmetric dimethylarginine; ATF6, Activating transcription factor 6; Atg5, Autophagy protein 5; AngⅡ, Angiotensin II; AB, Aortic banding; AGEs, advanced glycation end products; ACTA2, actin alpha 2; ARE, Activation of antioxidant response element; APD, Action potential duration; AIF, Apoptosis-inducing factor; AST, Aspartate amino transferase; ATP, adenosine triphosphate; AMP, adenine nucleotides; ACE, Angiotensin converting enzyme; Bcl-xl, B cell lymphoma extra-large; Bcl-2, B cell lymphoma-2; BNP, Brain natruretic peptide; CK-MB, Creatine kinase-MB; CTnT, Cardiac troponin T; CTnI, Cardiac troponin I; CVDs, Cardiovascular diseases; CAT, Catalase; CMECs, Cardiac microvascular endothelial cells; Cx43, connexin 43; CPK, creatine phosphokinase; COX-2, Cyclooxygenase-2; cTn-I, cardiac troponin I; CHOP, C/EBP-homologous protein; CTGF, Connective tissue growth factor; DRG, Dorsal root ganglia; DDAH2, Dimethylarginine dimethyllaminohydrolase 2; DOX, Doxorubicin; ERS, Endoplasmic reticulum stress; eNOS, Endothelial nitric oxide synthase; ECM, Extracellular matrix; FKBP12.6, FK506-binding protein 12.6; GSH-Px, Glutathione peroxidase; GPX4, Glutathione peroxidase 4; GRP78, Glucose-regulated protein; GHb, Glycosylated hemoglobin; GLP-1, Glucagon-like peptide-1; GLUT-4, Glucose transporter-4; Gsk3β, glycogen synthase kinase 3β; HSPs70, Heat shock proteins 70; HOTAIR, HOX transcript antisense RNA; HCSMCs, Human coronary artery smooth muscle cells; HUVEC, Human umbilical vein endothelial cell; HASMCs, Human aortic smooth muscle cells, HDL-c, High density lipoprotein cholesterol; HO-1, heme oxygenase-1; IRE1α, Inositol-requiring enzyme 1α; I/R, Ischemia-reperfusion; ISO, Isoprenaline; ICAM-1, Intercellular adhesion molecule-1; Ito, Transient outward potassium Current; ICa-L, L-type Ca2 + channels; IK1, Inwardly rectifying potassium current; IKM3, M3-receptor-activated delayed rectifier K + current; JAK, Janus kinase; LDH, Lactate dehydrogenase; LDL-c, Low density lipoprotein cholesterol; MT, Matrine; MYH11, Mooth muscle myosin heavy chain; Mfn2, Mitofusin 2; MAPK, Mitogen-activated protein kinase; NLRP3, NLR family pyrin domain containing 3; NEFA, Non-esterified fatty acid; Nrf2, Transcription of nuclear factor erythroid 2-like 2; OMT, Oxymatrine; ox-LDL, Low-density lipoprotein; Papp, Apparent permeability; P-gp, P-glycoprotein; PERK, protein kinase RNA-like endoplasmic reticulum kinase; Poldip2, polymerase delta interacting protein 2; RPS5, Ribosomal protein S5; p70S6 K, 70 kDa Ribosomal Protein S6 Kinase 2; PTENP1, pseudogene of PTEN tumor suppressor; RyR-2, Ryanodine receptor type 2; RAAS, Renin-angiotensin-aldosterone system; SCG, Superior cervical ganglia; STAT, Signal transducer and activator of transcription; SOD, Superoxide dismutase; TGF-β1, Transforming growth factor beta 1; TC, Total cholesterol; TG, Triglyceride; TLR-4, Toll-like receptor-4; UCP2, Uncoupling protein 2; VSMC, Vascular Smooth Muscle Cell; VCAM-1, vascular cell adhesion molecule-1; VASP, Vasodilator-Stimulated Phosphoprotein; XO, Xanthine oxidase; α-SMA, α-smooth muscle actin; β3-AR, β3-adrenoreceptor.
Keywords: matrine, oxymatrine, cardiovascular disease, pharmacological mechanism, pharmacokinetics
Citation: Chen S, Wu S and Lin B (2024) The potential therapeutic value of the natural plant compounds matrine and oxymatrine in cardiovascular diseases. Front. Cardiovasc. Med. 11:1417672. doi: 10.3389/fcvm.2024.1417672
Received: 15 April 2024; Accepted: 17 June 2024;
Published: 8 July 2024.
Edited by:
Hong Wang, Temple University, United StatesReviewed by:
Renyikun Yuan, Guangxi Traditional Chinese Medical University, ChinaJianli jimmy Zhao, University of Alabama at Birmingham, United States
© 2024 Chen, Wu and Lin. This is an open-access article distributed under the terms of the Creative Commons Attribution License (CC BY). The use, distribution or reproduction in other forums is permitted, provided the original author(s) and the copyright owner(s) are credited and that the original publication in this journal is cited, in accordance with accepted academic practice. No use, distribution or reproduction is permitted which does not comply with these terms.
*Correspondence: Bin Lin, d4c3b2a@126.com