- 1Department of Cardiology Center, The First Hospital of Jilin University, Changchun, China
- 2Department of Endocrinology and Metabolism, The First Hospital of Jilin University, Changchun, China
Uromodulin, also referred to as Tamm Horsfall protein (THP), is a renal protein exclusively synthesized by the kidneys and represents the predominant urinary protein under normal physiological conditions. It assumes a pivotal role within the renal system, contributing not only to ion transport and immune modulation but also serving as a critical factor in the prevention of urinary tract infections and kidney stone formation. Emerging evidence indicates that uromodulin may serve as a potential biomarker extending beyond renal function. Recent clinical investigations and Mendelian randomization studies have unveiled a discernible association between urinary regulatory protein levels and cardiovascular events and mortality. This review primarily delineates the intricate relationship between uromodulin and cardiovascular disease, elucidates its predictive utility as a novel biomarker for cardiovascular events, and delves into its involvement in various physiological and pathophysiological facets of the cardiovascular system, incorporating recent advancements in corresponding genetics.
1 Introduction
In 1873, Italian physician Carlo Rovida made the initial observation of a protein present in human urine, forming tubular patterns, later identified as uromodulin(UMOD), also referred to as Tamm-Horsfall protein (THP). This protein is the most abundantly secreted protein in the urine of healthy individuals and is primarily synthesized by the epithelial cells lining the renal tubules. Initially termed “cilindrina” (1), it wasn't until 1950 that Igor Tamman and Frank Horsfall recognized its potential as a mucin in preventing viral blood clotting, leading to the adoption of the name “Tamm-Horsfall protein” (2, 3). Subsequent research by Muchmore and Decker in 1985 revealed its immunosuppressive properties in vitro against T cell proliferation and monocytotoxicity, prompting its renaming as uromodulin (4, 5). Further genomic analysis by Pennica et al. confirmed the equivalence of Tamm Horsfall protein and uromodulin (6). The elucidation of uromodulin's physiology, structure, function, regulation, genomics, and potential clinical applications has gradually unfolded over the past two decades, shedding light on its once-mysterious nature. Extensive research has deepened our understanding of uromodulin's roles in various disease states. While much attention has been paid to its expression as a biomarker for kidney disease, recent years have witnessed a growing body of evidence linking uromodulin to cardiovascular events and mortality through numerous clinical and Mendelian randomization studies. This linkage is comprehensible given the recognized association between chronic kidney disease (CKD) and cardiovascular events (7), alongside the established role of uromodulin in salt-sensitive hypertension (8). Considering hypertension's status as a significant risk factor for various cardiovascular events (9), uromodulin emerges as a potential biomarker extending beyond renal function. This review aims to provide an overview of the current understanding of urinary regulatory proteins, highlighting their newfound implications in cardiovascular diseases such as hypertension and coronary heart disease. It underscores that urinary regulatory proteins serve not only as biomarkers for kidney diseases like CKD but also possess predictive value for cardiovascular events.
2 Overview of the structure and biochemistry of uromodulin
Uromodulin stands as the most prevalent protein in the urine of healthy adults and is exclusively synthesized by epithelial cells lining the renal tubules. Approximately 90% of uromodulin production originates from cells in the thick ascending limb (TAL), with another roughly 10% synthesized by epithelial cells in the early segment of the distal convoluted tubule (DCT). Its normal daily secretion ranges from 50 to 150 mg in the urine (10, 11).Uromodulin is composed of 640 amino acids (12), possesses a half-life of 16 h (13), and boasts a molecular weight of approximately 80–90 kDa (14, 15). Glycosylation, accounting for nearly 30% of its molecular weight, occurs at eight sites (16). The presence of sialic acid residues renders the protein highly acidic (17), with an isoelectric point of about 3.5 (18). Structurally, uromodulin encompasses multiple domains, including four epidermal growth factor (EGF)-like domains, a cysteine-rich domain (D8C) of unclear function, an internal hydrophobic plaque (IHP), and a dichotomous zona pellucida (ZP) domain facilitating protein polymerization (19) (As shown in Figure 1). The zona pellucida is followed by the external hydrophobic sheet (EHP) and the glycophosphatidylinositol(GPI) ancho. Uromodulin undergoes extensive intracellular post-translational modifications, including N-glycosylation of seven of the eight conserved sites (16) formation of 24 disulfide bridges, and cleavage of the serine protease hepsin at the C-terminus (20), with the endoplasmic reticulum playing an important role in uromodulin processing. Uromodulin was further elucidated using cryo-electron microscopy (cryo-EM) (21). The uromodulin polymerises into filaments with a core formed by a unique interlocking structure of the ZP-N and ZP-C structural domains arranged in a helical pattern with a rise of ∼65 Å and a twist of ∼180° (22, 23). The ZP-N and ZP-C domains have an immunoglobulin-like structure and interact with the ZP linker region by forming a β-fold. After Hepsin cleavage and EHP dissociation, uromodulin monomers are incorporated into growing filaments in a head-to-tail fashion as the activated ZP-C terminus interacts with the ZP-N structural domain of the afferent subunit (23). Although the predominant form of urinary uromodulin (uUMOD) is polymerized, new data suggest that non-polymerized forms exist and that retained EHP lacks GPI anchors (24). In addition to urine, non-aggregated forms of basolateral release also result in detectable serum uromodulin (sUMOD), albeit at significantly lower levels than urine concentrations (25).
3 Overview of the physiological functions of uromodulin
Uromodulin serves various physiological functions, encompassing the prevention of urinary tract infections, kidney stone formation, involvement in renal ion transport, and immune regulation, among others (12). Scanning electron microscopy of urine-purified uromodulin reveals a three-dimensional mesh composed of filaments with pore sizes ranging from 0.1 to 1 μm, forming a “fishing net” capable of trapping microorganisms for elimination through urination (26). Imaging studies of uromodulin-urinary pathogen interactions, both in vitro and in patient urine samples, demonstrate that uromodulin filaments associate with uropathogens, facilitating bacterial aggregation and potentially impeding adherence and clearance by urination (27). Numerous animal experiments and clinical investigations corroborate the positive correlation between uromodulin levels and protection against urinary tract infections (28–30). The protective effect of uromodulin against renal stones is believed to occur indirectly by inhibiting fiducial protein-mediated endocytosis and up-regulating the activity of TRPV5 channels to enhance calcium reabsorption in the distal convoluted tubule (DCT) (31–33), thereby reducing luminal calcium concentration. However, the precise mechanism and stages of uromodulin's action in crystal nucleation, growth, and aggregation remain to be fully elucidated. Uromodulin also plays a role in renal ion transport, particularly sodium, calcium, and magnesium. Its modulation of magnesium reabsorption involves regulating the cell surface abundance of the magnesium channel TRPM6 at the apical membrane of DCT cells (34, 35). Additionally, uromodulin exhibits potent immunomodulatory properties, activating various inflammatory cells such as neutrophils (36–38), macrophages (39, 40), and dendritic cells (41, 42). Moreover, it serves as a binding ligand for multiple molecules including serum albumin, immunoglobulin G light chains, complement components C1 and C1q, interleukins (IL)-1β, IL-6, IL-8, tumor necrosis factor (TNF)-α, and interferon-γ, via its carbohydrate side chains, thereby contributing to circulatory and renal immune homeostasis (15). Uromodulins exert distinct immune functions across different diseases and environments. Differences in the structure and regulation of uUMOD and sUMOD play important roles in physiological functions in the kidney, urinary tract, and systemic circulation. A growing number of animal experiments and clinical studies have shown that uUMOD levels are associated with an increased risk of salt-sensitive hypertension, while sUMOD appears to have a protective effect on the vascular system by inhibiting vascular calcification, both of which will be focused on later in this review. As the physiological functions of uromodulins are gradually being explored and recognized, they are increasingly recognized as a novel biological marker suggestive of cardiovascular disease.
4 Relationship between uromodulin and various cardiovascular diseases
The mechanisms by which uromodulin affects hypertension and coronary heart disease are shown in Figures 2, 3.
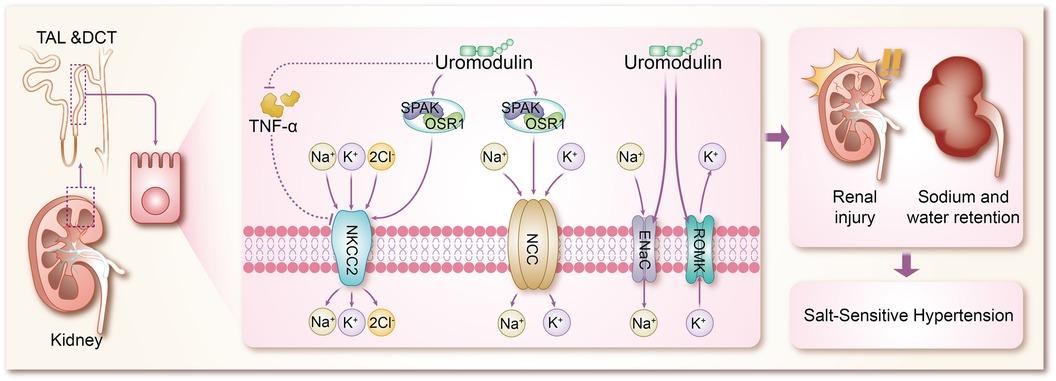
Figure 2 Mechanisms by which uromodulin affects salt-sensitive hypertension. TAL, thick ascending limb; DCT, distal convoluted tubule; TNF-α, tumor necrosis factor-α; SPAK, SPS1-associated proline/alanine-rich kinase; OSR1, oxidative stress-responsive kinase 1; NKCC2, Na + K + -2CI-cotransporter protein; NCC, Na + -CI-cotransporter protein; ENaC, epithelial sodium channel; ROMK, renal outer medullary potassium channel.
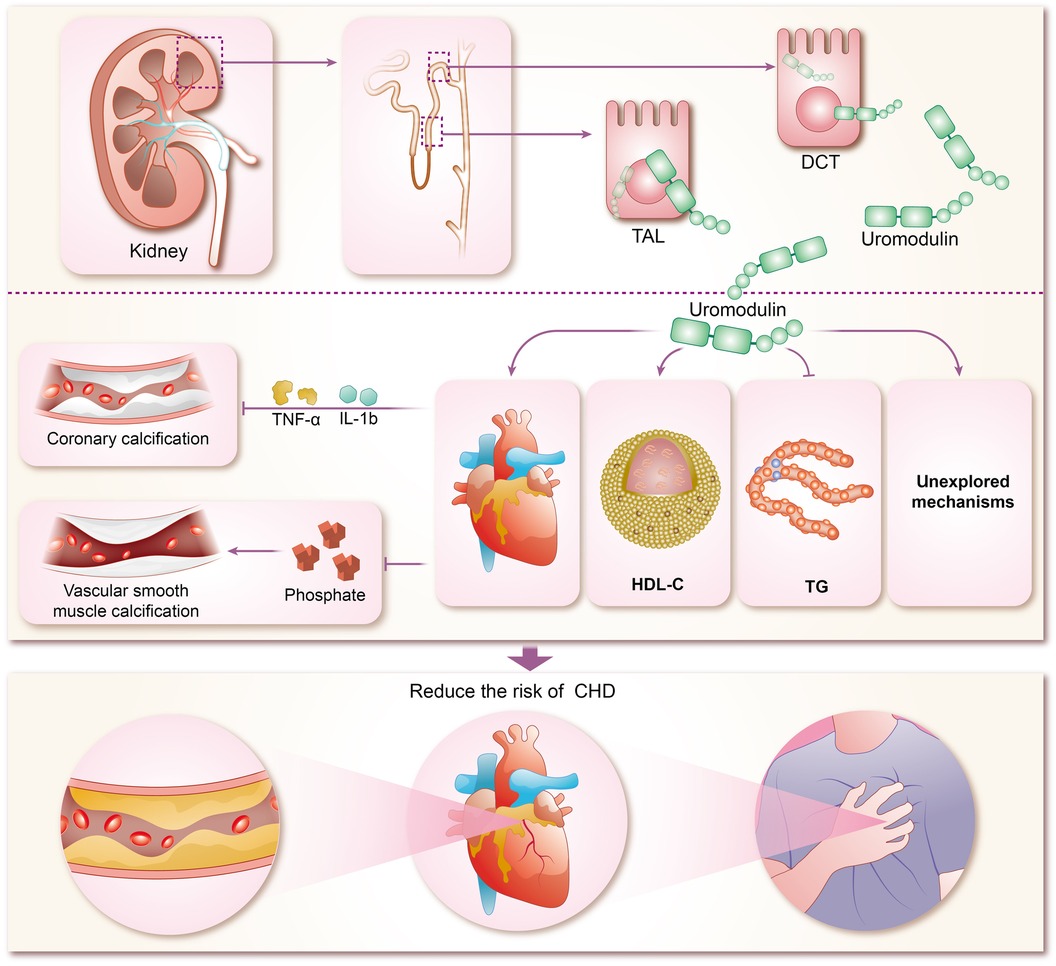
Figure 3 Mechanisms of coronary heart disease risk reduction by uromodulin. TAL, thick ascending limb; DCT, distal convoluted tubule; HDL-C, high-density lipoprotein cholesterol; TG, triglycerides; IL-1b, interleukins-1b; TNF-α, tumor necrosis factor-α; CHD, coronary heart disease.
4.1 Uromodulin and hypertension
Hypertension, a multifaceted chronic clinical syndrome, stands as a leading cause of various cardiovascular diseases including coronary heart disease and stroke (43). Globally, over a quarter of the population is estimated to suffer from hypertension, totaling more than 1 billion individuals. Its prevalence has been on the rise over the past decade, contributing significantly to the global disease burden and accounting for up to 10 million deaths worldwide (44). Hypertension manifests through numerous variable risk factors (43, 45), such as low potassium intake (46), high sodium intake (46), overweight and obesity (47), unhealthy diet (48), lack of physical activity (49), smoking and alcohol consumption (50), and other factors. However, the pathogenesis of hypertension is complex and is not fully understood. Sodium (Na+) homeostasis plays an important role in the regulation of blood pressure, and small changes in its rate of reabsorption may lead to significant changes in Na + excretion, leading to disturbances in Na + homeostasis and extracellular fluid volume, and ultimately to hypertension (51, 52). In a trial based on testing the effects of sodium on blood pressure modulated by uUMOD levels in the general population, it was found that higher sodium intake was associated with higher blood pressure in a stratified group of people with high levels of uUMOD, whereas subjects with low expression of uUMOD did not show a correlation between blood pressure and sodium intake, which confirms the role of uUMOD in the salt-sensitive component of blood pressure regulation (53). Under basal conditions, systolic blood pressure was significantly lower in UMOD knockout mice compared to wild-type (WT) mice (54). In addition in transgenic mice overexpressing UMOD, treatment with furosemide, a labeled diuretic, significantly enhanced urinary sodium excretion and reduced blood pressure levels (55). These studies using knockout and transgenic UMOD mouse models suggest that umod plays an important role in the development of salt-sensitive hypertension, resulting primarily from sodium reabsorption via Na + K + -2Cl- cotransporter protein (NKCC2) and Na + -Cl- cotransporter protein (54–56). The mechanism by which UMOD has been shown to affect blood pressure is mainly the combination of TAL and DCT to modulate the activity of ion transport proteins in epithelial cells, including the renal outer medullary potassium channel (ROMK) (57, 58), epithelial sodium channel (ENaC) (59), NKCC2 (60), and NCC (8, 61, 62), of which NKCC2 and NCC are the major transport proteins responsible for sodium reabsorption (63). Mutations in NKCC2 and malfunctions in its regulators are known to cause Bartter syndrome, a salt-depleting hypotensive disorder with reduced levels of UMOD, in addition to a significant reduction in NKCC2 phosphorylation in Umod(-/-) mice, where NKCC2 expression is not as strong as in WT mice, and conversely, mice with overexpressed UMOD exhibit salt-sensitive hypertension (64–66). Earlier studies confirmed that uromodulin promotes the activation of NKCC2 in a chloride-sensitive manner (66), but did not identify a specific mechanistic process for its activation, and similar to NKCC2, uromodulin also activates NCC in the DCT (67). It was subsequently demonstrated in a growing number of animal studies that uromodulin induces a significant increase in NKCC2 and NCC phosphorylation and its activity, leading to the up-regulation of NKCC2 and NCC through the regulation of the SPS1-associated proline/alanine-rich kinase/oxidative stress-responsive kinase 1 (SPAK-OSR1) (55, 66–70), which are involved in the pathogenesis of salt-sensitive hypertension (71–75). In previous studies of salt-sensitive hypertension, it has been shown that stimulation of SPAK/OSR1 activates renal ion channels including NKCC2, NCC, ENaC, and ROMK (76), so it is likely to be hypothesized that UMOD also promotes the expression of ROMK and ENaC through activation of SPAK-OSR1. The role of ENaC in salt-sensitive hypertension is self-evident, but there are no results on the specific molecular mechanisms by which UMOD affects ENaC, which will be a direction for future research to focus on and expand. Furthermore, WNK kinases are a family of central kinases that regulate the activity of ion channels involved in blood pressure regulation. Mechanistically, WNK kinases regulate the activity of renal cation-chloride transport proteins through the activation of the SGK1-WNK-SPAK/OSR1 phosphorylation cascade (76), and it is not clear whether UMOD stimulates WNK, or stimulates the upstream kinases of the WNK-SPAK/OSR1 axis, such as SGK1 (serum/glucocorticoid-regulated kinase 1) and protein kinase B (also known as AKT), which is not supported by the literature and will be something to explore in the future. ROMK, a voltage-dependent K + channel, increases K + efflux when ROMK activity is enhanced, largely decreasing intracellular K + concentration, at which point cation channels in the epithelial cells of the renal unit (including NKCC2, NCC, and ENaC) increase the uptake of the corresponding cations to compensate for the negative membrane potential resulting from the increased ROMK activity (77–79). In animal experiments, it has been demonstrated that Umod(-/-) mice exhibit a significant accumulation of ROMK in vesicles due to UMOD deletion, which results in delayed or reduced ROMK expression. Furthermore, the co-expression of ROMK with UMOD has been shown to enhance ROMK-mediated K + transporter activity, leading to an enhanced current amplitude. This effect appears to be dependent on the C-terminus of ROMK. Further investigation of the mechanism of the interactions between ROMK and UMOD reveals that UMOD removes the ubiquitylated C-terminus of the targeting ROMK from binding, thereby promoting an increase in the activity and amount of ROMK (80). In addition, tumor necrosis factor-α (TNF-α) has been widely mentioned in various animal experiments and clinical studies, and both protein and mRNA expression of NKCC2 was significantly up-regulated in TAL cells of TNFα-/- mice, suggesting that TNF-α is an endogenous inhibitor of NKCC2 and can affect the bioavailability and function of NKCC2 (81). TNF-α is produced by TAL and downregulates NKCC2 expression in an autocrine manner, reducing NaCl reabsorption at this site. Several studies have shown that UMOD binds several cytokines through its epidermal growth factor (EGF) structural domain, including TNF-α, which has a high affinity for UMOD (65, 82–85). Lesley A et al. went further by finding that in TAL cells, TNF-α stimulation increased the relative levels of UMOD mRNA, resulting in a negative feedback loop in which TNFα-induced reductions in NKCC2 gene expression were counteracted by increased production of cell surface UMOD (54). It can be concluded that UMOD plays a direct role in blood pressure regulation by modulating the effect of TNF-α on NKCC2 expression. Vasopressin plays an important role in water reabsorption by inducing apical expression of the water channel aquaporin-2 (AQP 2) (86), and because vasopressin is a hormone secreted during dehydration or volume deprivation, upregulation of UMOD secretion by vasopressin is considered to be a plausible physiological response. Recently, vasopressin has been shown to increase UMOD secretion by activating PKA (protein kinase A). In addition, it has been shown that UMOD enhances AQP2 phosphorylation and apical transport in mouse collecting duct cells treated with vasopressin analog dDAVP. The uromodulin-induced apicaltrafficking of AQP2 was attenuated via endocytosis inhibitor treatment, suggesting that uromodulin activates AQP2 through the suppression of endocytosis (87). Moreover, NKCC2 phosphorylation and activity are also regulated by the dDAVP-V2R-cAMP-PKA pathway, in which dDAVP promotes salt reabsorption by upregulating NKCC2 (88). However, in Umod(-/-) mice, attenuated phosphorylation of NKCC2 conferred a dDAVP-resistant phenotype for salt reabsorption, suggesting that activation of NKCC2 by urinary regulatory protein-regulated SPAK/OSR1 kinase superimposed on activation of salt regulation by dDAVP ligands (66). These findings suggest that TAL and collecting ducts work synergistically to retain sodium and water by interacting with urinary modification proteins, which in turn leads to hypertension.
In parallel to scientific studies such as animal experiments, advances in UMOD genetics have confirmed that UMOD affects blood pressure. Genome-wide association studies (GWAS) have revealed the existence of variants in the UMOD gene encoding uromodulins that are susceptible to renal function, and hypertension (89), and that non-coding UMOD gene variants induce salt-sensitive hypertension and renal injury through increased expression of uromodulins (55). The relevance of urinary regulators and their role in the regulation of sodium homeostasis is consistent with the results of the human GWAS (90). Single nucleotide polymorphisms in the UMOD gene were associated with hypertension, and conversely, variants with lower UMOD levels were associated with a lower risk of hypertension (91). Mendelian randomization (MR) is an emerging research methodology that allows the use of genetic variation (usually single nucleotide polymorphisms, SNPs) as instrumental variables to simulate randomized controlled trials (92). Using data from more than 750,000 people of European ancestry and applying a two-sample Mendelian randomization method, the researchers found that for every 1-SD increase in uUMOD, SBP increased by 0.06 SDmmHg and DBP increased by 0.08 SDmmHg (93). In another study, a two-sample Mendelian randomization of six datasets of over one million people found that SNPs leading to higher sUMOD and uUMOD were causally related to both SBP and DBP (61, 93). In a study of serum uromodulin and its genetic variants about blood pressure and hypertension in Chinese adults, Chinese researchers found that rs12917707 and rs12708631 in the uromodulin gene were significantly associated with longitudinal blood pressure change over 8 years of follow-up and that rs12708631 was significantly associated with the 8-year incidence of hypertension (94). Mendelian randomization studies using genetic variation as instrumental variables have further substantiated the causal relationship between uromodulin levels and blood pressure. However, these studies are subject to heterogeneity and polyvalence due to environmental factors influencing uromodulin levels, including nutritional intake, volume status, acid-base balance, renal function, and medication use.
The current studies allow us to determine that uUMOD promotes ion channel activity on TAL and DCT to cause sodium and water retention, which in turn leads to hypertension. Since uUMOD levels are associated with an increased risk of salt-sensitive hypertension, measurement of uUMOD is important for the diagnosis of hypertension and already has future clinical significance in guiding the use of diuretics, such as furosemide, in patients with sodium-water retention in this type of hypertension. uUMOD has often been used in various cohort studies of uromodulin and hypertension in populations, however, this does not mean that sUMOD is not associated with hypertension, as sUMOD was found to be significantly associated with a reduced risk of hypertension in a cohort study of adolescents with hypertension in Hanzhong City, China (94). In conclusion, there is a close link between uUMOD and hypertension, and the specific mechanism has not yet been fully explained, which still needs to be supported by continuous exploration of scientific research.
4.2 Uromodulin and coronary heart disease
The concentration of sUMOD has been widely proven to be closely related to renal function, and can even be used as the basis for CKD staging, and sUMOD has become self-evident as a biological marker of renal disease (95–98). In recent years, sUMOD has also been gradually emerging in the field of coronary heart disease. In the community-based KORA F4 study, sUMOD emerged as an independent biomarker of cardiovascular event-related mortality in men aged 62 years or older, even after adjusting for confounding clinical factors. Furthermore, sUMOD displayed a significant negative association with total and cardiovascular mortality in men (99). Studies by Steubl et al. indicated that higher levels of both sUMOD and uUMOD were linked not only to a reduced risk of end-stage renal disease (ESKD) in the elderly (100), but also to a decreased risk of cardiovascular disease, including myocardial infarction, stroke, and coronary artery disease or stroke-induced mortality (101). Delgado et al. investigated the association of sUMOD concentrations with cardiovascular biomarkers and mortality risk in a large cohort referred for coronary angiography, revealing that higher sUMOD levels were associated with favorable metabolic profiles, reduced prevalence of comorbidities, and a lower 10-year mortality risk (102). In addition among among white patients with chronic kidney disease, elevated sUMOD levels were associated with decreased risks of mortality, cardiovascular events, and kidney failure (103). Although most of the current studies on sUMOD and coronary heart disease are clinical observational studies, with few animal experiments and other specific elucidations of the mechanism, there are clues to explore the specific mechanisms involved. Atherosclerosis forms the pathogenetic basis of CHD, characterized by endothelial injury, inflammation, and endothelial cell apoptosis (104). Coronary artery calcification (CAC) typically accompanies advanced atherosclerosis development and serves as a predictor of future cardiovascular events (105). Uromodulin has been shown to be an inhibitor of calcification in blood and urine, reducing the risk of calcification (25, 106). Inflammatory pathway activation and oxidative stress drive vascular smooth muscle calcification (107–109), which uromodulin counteracts by interfering with pro-inflammatory cytokine signaling (TNF-a and IL-1b mediated osteochondral signaling) and reducing phosphate-induced calcification in aortic smooth muscle cells (110). Prospective studies demonstrate that higher baseline sUMOD levels predict reduced odds of CAC progression and diabetic kidney disease (DKD) in adults with type 1 diabetes over 12 years (111). While preliminary evidence suggests uromodulin's potential in reducing CHD risk by inhibiting coronary artery calcification, robust support from extensive animal experiments and clinical studies is lacking and warrants further exploration by researchers. Studies suggest a negative correlation between uromodulins and triglycerides (TG) alongside a positive correlation with high-density lipoprotein cholesterol (HDL-C), indicating their potential in modulating lipid metabolism and reducing CHD risk (112, 113). However, the renal disease may influence this relationship, as severe renal impairment may attenuate the protective effects of uromodulin on calcification and lipid metabolism.
sUMOD appears to have a protective effect on the vascular system by inhibiting vascular calcification, so does uUMOD have no role in this? In the Systolic Blood Pressure Intervention Trial (SPRINT), uUMOD concentration twice the norm was correlated with reduced cardiovascular disease risk in patients with chronic kidney disease (114). Sharma's research indicated reduced levels of uUMOD protein in coronary artery disease (CAD), particularly in individuals with recent myocardial infarction, suggesting its potential as an early diagnostic biomarker (115). However, conflicting evidence suggests that uUMOD may not be associated with risks of end-stage renal disease, cardiovascular disease, or heart failure (116), and baseline uUMOD levels and changes therein may not correlate with mortality (117). The precise relationship between uromodulin and cardiovascular disease is subject to the ongoing investigation and may be influenced by factors such as ethnicity, age, and gender. Genome-wide association studies (GWAS) have shown that common variants in the promoter of the UMOD gene with reduced uUMOD levels result in a reduced risk of CVD in the general population (91). A recent Mendelian randomization study demonstrated an effect of higher uUMOD on elevated blood pressure, which mediates the effect on the risk of myocardial infarction in the general population (118). Genetic and experimental findings were not in complete agreement or even had opposite results, suggesting that further studies are still needed to validate the association between uromodulin and coronary heart disease outcomes.
5 Future and outlook
While significant strides have been made in understanding uromodulin's role in cardiovascular disease, numerous unknown aspects warrant further exploration. Current research primarily focuses on hypertension and coronary artery disease, leaving other cardiac conditions such as atrial fibrillation, valvular disease, cardiomyopathy, myocarditis, and heart failure relatively understudied. Given the interconnected nature of cardiac diseases and their profound impact on overall health, investigating the relationship between uromodulin and these conditions holds promise for advancing our understanding of cardiovascular pathophysiology. Recent findings suggest an association between uromodulin and left atrial remodeling, as well as left atrium size (119), with implications for conditions like atrial fibrillation, stroke, heart failure, and increased cardiovascular disease rates and mortality (120–124). This area presents an exciting avenue for future research into uromodulin's role in cardiovascular disease. Patients with chronic kidney disease (CKD) face elevated cardiovascular risk, with a significant proportion experiencing cardiovascular events (125). Continued exploration of uromodulin's involvement in cardiovascular diseases holds potential benefits for this patient population, given the high prevalence of cardiovascular complications in CKD. Furthermore, the association of uromodulin, exclusively produced in the kidney, and the UMOD gene, exclusively expressed in the kidney, with cardiovascular disease, opens avenues for investigating cardiorenal syndrome and elucidating the intricate link between heart and kidney health.
6 Conclusion
Uromodulins have garnered significant interest among researchers and clinicians in recent years. Progress has been made in understanding their structural characteristics, physiological functions, and clinical relevance. Importantly, their investigation is no longer confined to renal disease, unveiling their enigmatic properties across various body systems. As research advances, uromodulins' distinctive role in the cardiovascular system and beyond is becoming evident. However, further studies are essential to solidify their potential utility as novel biomarkers for cardiovascular disease. Continued exploration holds promise for unlocking the full scope of uromodulins’ impact on human health. Looking ahead, collaborative efforts are needed to explore new avenues of research, such as investigating the mechanisms underlying uromodulins' effects on cardiovascular health and conducting large-scale clinical trials to validate their utility as biomarkers. By doing so, we can harness the diagnostic and therapeutic potential of uromodulin to improve patient outcomes in cardiovascular medicine and beyond. Moreover, considering the multifaceted nature of cardiovascular diseases, future investigations should explore uromodulins' interactions with other biomolecules and their potential modulation of diverse pathological processes. Additionally, elucidating the regulatory pathways governing uromodulins' expression and activity could provide valuable insights into novel therapeutic targets for cardiovascular conditions. Overall, sustained research efforts are crucial to fully comprehend the intricate roles of uromodulins in cardiovascular health and disease. By advancing our understanding in this field, we can pave the way for innovative diagnostic and therapeutic strategies that offer improved outcomes and better quality of life for patients worldwide.
Author contributions
CC: Conceptualization, Writing – original draft. WZ: Methodology, Writing – original draft. HZ: Writing – original draft. GD: Writing – original draft, Formal Analysis. WZ: Writing – original draft. YW: Writing – original draft, Visualization. QD: Visualization, Writing – original draft. BS: Supervision, Writing – review & editing.
Funding
The author(s) declare that no financial support was received for the research, authorship, and/or publication of this article.
Conflict of interest
The authors declare that the research was conducted in the absence of any commercial or financial relationships that could be construed as a potential conflict of interest.
Publisher's note
All claims expressed in this article are solely those of the authors and do not necessarily represent those of their affiliated organizations, or those of the publisher, the editors and the reviewers. Any product that may be evaluated in this article, or claim that may be made by its manufacturer, is not guaranteed or endorsed by the publisher.
References
1. Fogazzi GB, Testanera G. The farsighted studies of the Italian carlo L. Rovida (1844–1877) on the nature of urinary casts. Am J Nephrol. (2002) 22:300–8. doi: 10.1159/000063778
2. Tamm I, Horsfall FL Jr. Characterization and separation of an inhibitor of viral hemagglutination present in urine. Proc Soc Exp Biol Med. (1950) 74:106–8. doi: 10.3181/00379727-74-17825
3. Tamm I, Horsfall FL Jr. A mucoprotein derived from human urine which reacts with influenza, mumps, and Newcastle disease viruses. J Exp Med (1952) 95:71–97. doi: 10.1084/jem.95.1.71
4. Muchmore AV, Decker JM. Uromodulin: a unique 85-kilodalton immunosuppressive glycoprotein isolated from urine of pregnant women. Science. (1985) 229:479–81. doi: 10.1126/science.2409603
5. Muchmore AV. Uromodulin: an immunoregulatory glycoprotein isolated from pregnancy urine that binds to and regulates the activity of interleukin 1. Am J Reprod Immunol Microbiol. (1986) 11:89–93. doi: 10.1111/j.1600-0897.1986.tb00037.x
6. Pennica D, Kohr WJ, Kuang WJ, Glaister D, Aggarwal BB, Chen EY, et al. Identification of human uromodulin as the Tamm-Horsfall urinary glycoprotein. Science. (1987) 236:83–8. doi: 10.1126/science.3453112
7. Buckley LF, Schmidt IM, Verma A, Palsson R, Adam D, Shah AM, et al. Associations between kidney histopathologic lesions and incident cardiovascular disease in adults with chronic kidney disease. JAMA Cardiol. (2023) 8:357–65. doi: 10.1001/jamacardio.2023.0056
8. Mary S, Boder P, Padmanabhan S, McBride MW, Graham D, Delles C, et al. Role of uromodulin in salt-sensitive hypertension. Hypertension. (2022) 79:2419–29. doi: 10.1161/HYPERTENSIONAHA.122.19888
9. Tadic M, Cuspidi C, Marwick TH. Phenotyping the hypertensive heart. Eur Heart J. (2022) 43:3794–810. doi: 10.1093/eurheartj/ehac393
10. Bachmann S, Dawnay AB, Bouby N, Bankir L. Tamm-Horsfall protein excretion during chronic alterations in urinary concentration and protein intake in the rat. Ren Physiol Biochem. (1991) 14:236–45. doi: 10.1159/000173411
11. Goodall AA, Marshall RD. Effects of freezing on the estimated amounts of Tamm–Horsfall glycoprotein in urine, as determined by radioimmunoassay. Biochem J. (1980) 189:533–9. doi: 10.1042/bj1890533
12. Schaeffer C, Devuyst O, Rampoldi L. Uromodulin: roles in health and disease. Annu Rev Physiol. (2021) 83:477–501. doi: 10.1146/annurev-physiol-031620-092817
13. Grant AM, Neuberger A. The turnover rate of rabbit urinary Tamm-Horsfall glycoprotein. Biochem J. (1973) 136:659–68. doi: 10.1042/bj1360659
14. Zimmerhackl LB, Rostasy K, Wiegele G, Rasenack A, Wilhelm C, Lohner M, et al. Tamm-Horsfall protein as a marker of tubular maturation. Pediatr Nephrol. (1996) 10:448–52. doi: 10.1007/s004670050137
15. Wu TH, Li KJ, Yu CL, Tsai CY. Tamm-Horsfall protein is a potent immunomodulatory molecule and a disease biomarker in the urinary system. Molecules. (2018) 23(1):200. doi: 10.3390/molecules23010200
16. van Rooijen JJ, Voskamp AF, Kamerling JP, Vliegenthart JF. Glycosylation sites and site-specific glycosylation in human tamm-horsfall glycoprotein. Glycobiology. (1999) 9:21–30. doi: 10.1093/glycob/9.1.21
17. Parsons CL, Rajasekaran M, Arsanjani AH, Chenoweth M, Stein P. Role of sialic acid in urinary cytoprotective activity of Tamm-Horsfall protein. Urology. (2007) 69:577–81. doi: 10.1016/j.urology.2006.12.026
18. Schnierle P, Hering F, Seiler H. Isoelectric focusing of Tamm-Horsfall glycoproteins: a simple tool for recognizing recurrent calcium oxalate renal stone formers. Urol Res. (1996) 24:79–82. doi: 10.1007/BF00431083
19. Karagiannidis AG, Theodorakopoulou MP, Pella E, Sarafidis PA, Ortiz A. Uromodulin biology. Nephrol Dial Transplant. (2024) 39(7):1073–87. doi: 10.1093/ndt/gfae008
20. Brunati M, Perucca S, Han L, Cattaneo A, Consolato F, Andolfo A, et al. The serine protease hepsin mediates urinary secretion and polymerisation of Zona Pellucida domain protein uromodulin. Elife. (2015) 4:e08887. doi: 10.7554/eLife.08887
21. Stsiapanava A, Xu C, Brunati M, Zamora-Caballero S, Schaeffer C, Bokhove M, et al. Cryo-EM structure of native human uromodulin, a Zona Pellucida module polymer. Embo J. (2020) 39:e106807. doi: 10.15252/embj.2020106807
22. Bokhove M, Nishimura K, Brunati M, Han L, de Sanctis D, Rampoldi L, et al. A structured interdomain linker directs self-polymerization of human uromodulin. Proc Natl Acad Sci U S A. (2016) 113:1552–7. doi: 10.1073/pnas.1519803113
23. Stanisich JJ, Zyla DS, Afanasyev P, Xu J, Kipp A, Olinger E, et al. The cryo-EM structure of the human uromodulin filament core reveals a unique assembly mechanism. Elife. (2020) 9:e60265. doi: 10.7554/eLife.60265
24. Micanovic R, LaFavers KA, Patidar KR, Ghabril MS, Doud EH, Mosley AL, et al. The kidney releases a nonpolymerizing form of uromodulin in the urine and circulation that retains the external hydrophobic patch domain. Am J Physiol Renal Physiol. (2022) 322:F403–18. doi: 10.1152/ajprenal.00322.2021
25. LaFavers K, Garimella PS. Uromodulin: more than a marker for chronic kidney disease progression. Curr Opin Nephrol Hypertens. (2023) 32:271–7. doi: 10.1097/MNH.0000000000000885
26. Bjugn R, Flood PR. Scanning electron microscopy of human urine and purified Tamm-Horsfall’s glycoprotein. Scand J Urol Nephrol. (1988) 22:313–5. doi: 10.3109/00365598809180806
27. Weiss GL, Stanisich JJ, Sauer MM, Lin CW, Eras J, Zyla DS, et al. Architecture and function of human uromodulin filaments in urinary tract infections. Science. (2020) 369:1005–10. doi: 10.1126/science.aaz9866
28. Garimella PS, Bartz TM, Ix JH, Chonchol M, Shlipak MG, Devarajan P, et al. Urinary uromodulin and risk of urinary tract infections: the cardiovascular health study. Am J Kidney Dis. (2017) 69:744–51. doi: 10.1053/j.ajkd.2016.08.022
29. Bates JM, Raffi HM, Prasadan K, Mascarenhas R, Laszik Z, Maeda N, et al. Tamm-Horsfall protein knockout mice are more prone to urinary tract infection: rapid communication. Kidney Int. (2004) 65:791–7. doi: 10.1111/j.1523-1755.2004.00452.x
30. Mo L, Zhu XH, Huang HY, Shapiro E, Hasty DL, Wu XR. Ablation of the Tamm-Horsfall protein gene increases susceptibility of mice to bladder colonization by type 1-fimbriated Escherichia coli. Am J Physiol Renal Physiol. (2004) 286:F795–802. doi: 10.1152/ajprenal.00357.2003
31. Wolf MT, Wu XR, Huang CL. Uromodulin upregulates TRPV5 by impairing caveolin-mediated endocytosis. Kidney Int. (2013) 84:130–7. doi: 10.1038/ki.2013.63
32. Nie M, Bal MS, Yang Z, Liu J, Rivera C, Wenzel A, et al. Mucin-1 increases renal TRPV5 activity in vitro, and urinary level associates with calcium nephrolithiasis in patients. J Am Soc Nephrol. (2016) 27:3447–58. doi: 10.1681/ASN.2015101100
33. Cui Z, Li Y, Liu G, Jiang Y. miR-103a-3p silencing ameliorates calcium oxalate deposition in rat kidney by activating the UMOD/TRPV5 axis. Dis Markers. (2022) 2022:2602717. doi: 10.1155/2022/2602717
34. Nie M, Bal MS, Liu J, Yang Z, Rivera C, Wu XR, et al. Uromodulin regulates renal magnesium homeostasis through the ion channel transient receptor potential melastatin 6 (TRPM6). J Biol Chem. (2018) 293:16488–502. doi: 10.1074/jbc.RA118.003950
35. Maeoka Y, McCormick JA. NaCl cotransporter activity and mg(2+) handling by the distal convoluted tubule. Am J Physiol Renal Physiol. (2020) 319:F1043–53. doi: 10.1152/ajprenal.00463.2020
36. Patras KA, Coady A, Olson J, Ali SR, RamachandraRao SP, Kumar S, et al. Tamm-Horsfall glycoprotein engages human siglec-9 to modulate neutrophil activation in the urinary tract. Immunol Cell Biol. (2017) 95:960–5. doi: 10.1038/icb.2017.63
37. Horton JK, Davies M, Topley N, Thomas D, Williams JD. Activation of the inflammatory response of neutrophils by Tamm-Horsfall glycoprotein. Kidney Int. (1990) 37:717–26. doi: 10.1038/ki.1990.38
38. Micanovic R, Chitteti BR, Dagher PC, Srour EF, Khan S, Hato T, et al. Tamm-Horsfall protein regulates granulopoiesis and systemic neutrophil homeostasis. J Am Soc Nephrol. (2015) 26:2172–82. doi: 10.1681/ASN.2014070664
39. Micanovic R, Khan S, Janosevic D, Lee ME, Hato T, Srour EF, et al. Tamm-Horsfall protein regulates mononuclear phagocytes in the kidney. J Am Soc Nephrol. (2018) 29:841–56. doi: 10.1681/ASN.2017040409
40. Thomas DB, Davies M, Williams JD. Release of gelatinase and superoxide from human mononuclear phagocytes in response to particulate Tamm Horsfall protein. Am J Pathol. (1993) 142(1):249–60. doi: 10.1007/BF01607171
41. Pfistershammer K, Klauser C, Leitner J, Stöckl J, Majdic O, Weichhart T, et al. Identification of the scavenger receptors SREC-I, cla-1 (SR-BI), and SR-AI as cellular receptors for Tamm-Horsfall protein. J Leukoc Biol. (2008) 83:131–8. doi: 10.1189/jlb.0407231
42. Säemann MD, Weichhart T, Zeyda M, Staffler G, Schunn M, Stuhlmeier KM, et al. Tamm-Horsfall glycoprotein links innate immune cell activation with adaptive immunity via a toll-like receptor-4-dependent mechanism. J Clin Invest. (2005) 115:468–75. doi: 10.1172/JCI200522720
43. Charchar FJ, Prestes PR, Mills C, Ching SM, Neupane D, Marques FZ, et al. Lifestyle management of hypertension: international society of hypertension position paper endorsed by the world hypertension league and European society of hypertension. J Hypertens. (2024) 42:23–49. doi: 10.1097/HJH.0000000000003563
44. Hengel FE, Sommer C, Wenzel U. Arterial hypertension. Dtsch Med Wochenschr. (2022) 147:414–28. doi: 10.1055/a-1577-8663
45. Mills KT, Stefanescu A, He J. The global epidemiology of hypertension. Nat Rev Nephrol. (2020) 16:223–37. doi: 10.1038/s41581-019-0244-2
46. Da Costa C, Leszek Paccolat C, Ponte B. Sodium, potassium et hypertension artérielle: quoi de neuf? [Sodium, potassium and arterial hypertension: what's new?]. Rev Med Suisse. (2022) 18(795):1694–7. French. doi: 10.53738/REVMED.2022.18.795.1694
47. Shams E, Kamalumpundi V, Peterson J, Gismondi RA, Oigman W, de Gusmão Correia ML. Highlights of mechanisms and treatment of obesity-related hypertension. J Hum Hypertens. (2022) 36:785–93. doi: 10.1038/s41371-021-00644-y
48. Nepali P, Suresh S, Pikale G, Jhaveri S, Avanthika C, Bansal M, et al. Hypertension and the role of dietary fiber. Curr Probl Cardiol. (2022) 47:101203. doi: 10.1016/j.cpcardiol.2022.101203
49. Booth FW, Roberts CK, Laye MJ. Lack of exercise is a major cause of chronic diseases. Compr Physiol. (2012) 2:1143–211. doi: 10.1002/cphy.c110025
50. Nagao T, Nogawa K, Sakata K, Morimoto H, Morita K, Watanabe Y, et al. Effects of alcohol consumption and smoking on the onset of hypertension in a long-term longitudinal study in a male workers’ cohort. Int J Environ Res Public Health. (2021) 18(22):11781. doi: 10.3390/ijerph182211781
51. Aung K, Ream-Winnick S, Lane M, Akinlusi I, Shi T, Htay T. Sodium homeostasis and hypertension. Curr Cardiol Rep. (2023) 25:1123–9. doi: 10.1007/s11886-023-01931-5
52. Van Beusecum JP, Rianto F, Teakell J, Kon V, Sparks MA, Hoorn EJ, et al. Novel concepts in nephron sodium transport: a physiological and clinical perspective. Adv Kidney Dis Health. (2023) 30:124–36. doi: 10.1053/j.akdh.2022.12.007
53. Ponte B, Pruijm M, Ackermann D, Olinger E, Youhanna S, Vogt B, et al. Uromodulin, salt, and 24-hour blood pressure in the general population. Clin J Am Soc Nephrol. (2021) 16:787–9. doi: 10.2215/CJN.11230720
54. Graham LA, Padmanabhan S, Fraser NJ, Kumar S, Bates JM, Raffi HS, et al. Validation of uromodulin as a candidate gene for human essential hypertension. Hypertension. (2014) 63:551–8. doi: 10.1161/HYPERTENSIONAHA.113.01423
55. Trudu M, Janas S, Lanzani C, Debaix H, Schaeffer C, Ikehata M, et al. Common noncoding UMOD gene variants induce salt-sensitive hypertension and kidney damage by increasing uromodulin expression. Nat Med. (2013) 19:1655–60. doi: 10.1038/nm.3384
56. Liu Y, Goldfarb DS, El-Achkar TM, Lieske JC, Wu XR. Tamm-Horsfall protein/uromodulin deficiency elicits tubular compensatory responses leading to hypertension and hyperuricemia. Am J Physiol Renal Physiol. (2018) 314:F1062–76. doi: 10.1152/ajprenal.00233.2017
57. Aretz CD, Vadukoot AK, Hopkins CR. Discovery of small molecule renal outer medullary potassium (ROMK) channel inhibitors: a brief history of medicinal chemistry approaches to develop novel diuretic therapeutics. J Med Chem. (2019) 62:8682–94. doi: 10.1021/acs.jmedchem.8b01891
58. Schiano G, Glaudemans B, Olinger E, Goelz N, Müller M, Loffing-Cueni D, et al. The urinary excretion of uromodulin is regulated by the potassium channel ROMK. Sci Rep. (2019) 9:19517. doi: 10.1038/s41598-019-55771-x
59. Rossier BC. Epithelial sodium channel (ENaC) and the control of blood pressure. Curr Opin Pharmacol. (2014) 15:33–46. doi: 10.1016/j.coph.2013.11.010
60. Caceres PS, Ortiz PA. Molecular regulation of NKCC2 in blood pressure control and hypertension. Curr Opin Nephrol Hypertens. (2019) 28:474–80. doi: 10.1097/MNH.0000000000000531
61. You R, Chen L, Xu L, Zhang D, Li H, Shi X, et al. High level of uromodulin increases the risk of hypertension: a Mendelian randomization study. Front Cardiovasc Med. (2021) 8:736001. doi: 10.3389/fcvm.2021.736001
62. Meor Azlan NF, Koeners MP, Zhang J. Regulatory control of the Na-Cl co-transporter NCC and its therapeutic potential for hypertension. Acta Pharm Sin B. (2021) 11:1117–28. doi: 10.1016/j.apsb.2020.09.009
63. Kipp A, Olinger E. What does uromodulin do? Clin J Am Soc Nephrol. (2020) 16:150–3. doi: 10.2215/CJN.06390420
64. Adachi M, Asakura Y, Sato Y, Tajima T, Nakajima T, Yamamoto T, et al. Novel SLC12A1 (NKCC2) mutations in two families with Bartter syndrome type 1. Endocr J. (2007) 54:1003–7. doi: 10.1507/endocrj.K06-204
65. Mutig K, Kahl T, Saritas T, Godes M, Persson P, Bates J, et al. Activation of the bumetanide-sensitive na+,K+,2Cl- cotransporter (NKCC2) is facilitated by Tamm-Horsfall protein in a chloride-sensitive manner. J Biol Chem. (2011) 286:30200–10. doi: 10.1074/jbc.M111.222968
66. Lin SC, Ma C, Chang KJ, Cheong HP, Lee MC, Lan YT, et al. The post-translational modification networking in WNK-centric hypertension regulation and electrolyte homeostasis. Biomedicines. (2022) 10(9):2169. doi: 10.3390/biomedicines10092169
67. Tokonami N, Takata T, Beyeler J, Ehrbar I, Yoshifuji A, Christensen EI, et al. Uromodulin is expressed in the distal convoluted tubule, where it is critical for regulation of the sodium chloride cotransporter NCC. Kidney Int. (2018) 94:701–15. doi: 10.1016/j.kint.2018.04.021
68. Richardson C, Sakamoto K, de los Heros P, Deak M, Campbell DG, Prescott AR, et al. Regulation of the NKCC2 ion cotransporter by SPAK-OSR1-dependent and -independent pathways. J Cell Sci. (2011) 124:789–800. doi: 10.1242/jcs.077230
69. Boder P, Mary S, Mark PB, Leiper J, Dominiczak AF, Padmanabhan S, et al. Mechanistic interactions of uromodulin with the thick ascending limb: perspectives in physiology and hypertension. J Hypertens. (2021) 39:1490–504. doi: 10.1097/HJH.0000000000002861
70. Lin SH, Yu IS, Jiang ST, Lin SW, Chu P, Chen A, et al. Impaired phosphorylation of Na(+)-K(+)-2Cl(-) cotransporter by oxidative stress-responsive kinase-1 deficiency manifests hypotension and Bartter-like syndrome. Proc Natl Acad Sci U S A. (2011) 108:17538–43. doi: 10.1073/pnas.1107452108
71. Furusho T, Uchida S, Sohara E. The WNK signaling pathway and salt-sensitive hypertension. Hypertens Res. (2020) 43:733–43. doi: 10.1038/s41440-020-0437-x
72. Hirohama D, Fujita T. Evaluation of the pathophysiological mechanisms of salt-sensitive hypertension. Hypertens Res. (2019) 42:1848–57. doi: 10.1038/s41440-019-0332-5
73. Nomura N, Shoda W, Uchida S. Clinical importance of potassium intake and molecular mechanism of potassium regulation. Clin Exp Nephrol. (2019) 23:1175–80. doi: 10.1007/s10157-019-01766-x
74. McCormick JA, Mutig K, Nelson JH, Saritas T, Hoorn EJ, Yang CL, et al. A SPAK isoform switch modulates renal salt transport and blood pressure. Cell Metab. (2011) 14:352–64. doi: 10.1016/j.cmet.2011.07.009
75. Yang SS, Lo YF, Wu CC, Lin SW, Yeh CJ, Chu P, et al. SPAK-knockout mice manifest Gitelman syndrome and impaired vasoconstriction. J Am Soc Nephrol. (2010) 21:1868–77. doi: 10.1681/ASN.2009121295
76. Richardson C, Rafiqi FH, Karlsson HK, Moleleki N, Vandewalle A, Campbell DG, et al. Activation of the thiazide-sensitive Na+-cl- cotransporter by the WNK-regulated kinases SPAK and OSR1. J Cell Sci. (2008) 121:675–84. doi: 10.1242/jcs.025312
77. Welling PA, Ho K. A comprehensive guide to the ROMK potassium channel: form and function in health and disease. Am J Physiol Renal Physiol. (2009) 297:F849–63. doi: 10.1152/ajprenal.00181.2009
78. Fang L, Li D, Welling PA. Hypertension resistance polymorphisms in ROMK (Kir1.1) alter channel function by different mechanisms. Am J Physiol Renal Physiol. (2010) 299:F1359–64. doi: 10.1152/ajprenal.00257.2010
79. Lu M, Wang T, Yan Q, Yang X, Dong K, Knepper MA, et al. Absence of small conductance K+ channel (SK) activity in apical membranes of thick ascending limb and cortical collecting duct in ROMK (Bartter’s) knockout mice. J Biol Chem. (2002) 277:37881–7. doi: 10.1074/jbc.M206644200
80. Renigunta A, Renigunta V, Saritas T, Decher N, Mutig K, Waldegger S. Tamm-Horsfall glycoprotein interacts with renal outer medullary potassium channel ROMK2 and regulates its function. J Biol Chem. (2011) 286:2224–35. doi: 10.1074/jbc.M110.149880
81. Battula S, Hao S, Pedraza PL, Stier CT, Ferreri NR. Tumor necrosis factor-alpha is an endogenous inhibitor of Na+-K+-2Cl- cotransporter (NKCC2) isoform A in the thick ascending limb. Am J Physiol Renal Physiol. (2011) 301:F94–100. doi: 10.1152/ajprenal.00650.2010
82. Sherblom AP, Decker JM, Muchmore AV. The lectin-like interaction between recombinant tumor necrosis factor and uromodulin. J Biol Chem. (1988) 263:5418–24. doi: 10.1016/S0021-9258(18)60733-1
83. Tanna S, Doshi G, Godad A. siRNA as potential therapeutic strategy for hypertension. Eur J Pharmacol. (2024) 969:176467. doi: 10.1016/j.ejphar.2024.176467
84. Bachmann S, Mutig K, Bates J, Welker P, Geist B, Gross V, et al. Renal effects of Tamm-Horsfall protein (uromodulin) deficiency in mice. Am J Physiol Renal Physiol. (2005) 288:F559–67. doi: 10.1152/ajprenal.00143.2004
85. Hao S, Zhao H, Darzynkiewicz Z, Battula S, Ferreri NR. Differential regulation of NFAT5 by NKCC2 isoforms in medullary thick ascending limb (mTAL) cells. Am J Physiol Renal Physiol. (2011) 300:F966–75. doi: 10.1152/ajprenal.00408.2010
86. Sandoval PC, Claxton JS, Lee JW, Saeed F, Hoffert JD, Knepper MA. Systems-level analysis reveals selective regulation of Aqp2 gene expression by vasopressin. Sci Rep. (2016) 6:34863. doi: 10.1038/srep34863
87. Takata T, Hamada S, Mae Y, Iyama T, Ogihara R, Seno M, et al. Uromodulin regulates murine aquaporin-2 activity via thick ascending limb-collecting duct cross-talk during water deprivation. Int J Mol Sci. (2022) 23(16):9410. doi: 10.3390/ijms23169410
88. Ponce-Coria J, San-Cristobal P, Kahle KT, Vazquez N, Pacheco-Alvarez D, de Los Heros P, et al. Regulation of NKCC2 by a chloride-sensing mechanism involving the WNK3 and SPAK kinases. Proc Natl Acad Sci U S A. (2008) 105:8458–63. doi: 10.1073/pnas.0802966105
89. Devuyst O, Bochud M, Olinger E. UMOD and the architecture of kidney disease. Pflugers Arch. (2022) 474:771–81. doi: 10.1007/s00424-022-02733-4
90. Takata T, Isomoto H. The versatile role of uromodulin in renal homeostasis and its relevance in chronic kidney disease. Intern Med. (2024) 63:17–23. doi: 10.2169/internalmedicine.1342-22
91. Padmanabhan S, Melander O, Johnson T, Di Blasio AM, Lee WK, Gentilini D, et al. Genome-wide association study of blood pressure extremes identifies variant near UMOD associated with hypertension. PLoS Genet. (2010) 6:e1001177. doi: 10.1371/journal.pgen.1001177
92. Birney E. Mendelian randomization. Cold Spring Harb Perspect Med. (2022) 12(4):a041302. doi: 10.1101/cshperspect.a041302
93. Ponte B, Sadler MC, Olinger E, Vollenweider P, Bochud M, Padmanabhan S, et al. Mendelian randomization to assess causality between uromodulin, blood pressure and chronic kidney disease. Kidney Int. (2021) 100:1282–91. doi: 10.1016/j.kint.2021.08.032
94. Wang Y, Du MF, Yao S, Zou T, Zhang XY, Hu GL, et al. Associations of serum uromodulin and its genetic variants with blood pressure and hypertension in Chinese adults. Front Cardiovasc Med. (2021) 8:710023. doi: 10.3389/fcvm.2021.710023
95. Leiherer A, Muendlein A, Saely CH, Brandtner EM, Geiger K, Fraunberger P, et al. The value of uromodulin as a new serum marker to predict decline in renal function. J Hypertens. (2018) 36:110–8. doi: 10.1097/HJH.0000000000001527
96. Steubl D, Block M, Herbst V, Nockher WA, Schlumberger W, Satanovskij R, et al. Plasma uromodulin correlates with kidney function and identifies early stages in chronic kidney disease patients. Medicine (Baltimore). (2016) 95:e3011. doi: 10.1097/MD.0000000000003011
97. Lv L, Wang J, Gao B, Wu L, Wang F, Cui Z, et al. Serum uromodulin and progression of kidney disease in patients with chronic kidney disease. J Transl Med. (2018) 16:316. doi: 10.1186/s12967-018-1693-2
98. Scherberich JE, Gruber R, Nockher WA, Christensen EI, Schmitt H, Herbst V, et al. Serum uromodulin-a marker of kidney function and renal parenchymal integrity. Nephrol Dial Transplant. (2018) 33:284–95. doi: 10.1093/ndt/gfw422
99. Then C, Then HL, Lechner A, Thorand B, Meisinger C, Heier M, et al. Serum uromodulin and risk for cardiovascular morbidity and mortality in the community-based KORA F4 study. Atherosclerosis. (2020) 297:1–7. doi: 10.1016/j.atherosclerosis.2020.01.030
100. Steubl D, Buzkova P, Garimella PS, Ix JH, Devarajan P, Bennett MR, et al. Association of serum uromodulin with ESKD and kidney function decline in the elderly: the cardiovascular health study. Am J Kidney Dis. (2019) 74:501–9. doi: 10.1053/j.ajkd.2019.02.024
101. Steubl D, Buzkova P, Garimella PS, Ix JH, Devarajan P, Bennett MR, et al. Association of serum uromodulin with mortality and cardiovascular disease in the elderly-the cardiovascular health study. Nephrol Dial Transplant. (2020) 35:1399–405. doi: 10.1093/ndt/gfz008
102. Delgado GE, Kleber ME, Scharnagl H, Krämer BK, März W, Scherberich JE. Serum uromodulin and mortality risk in patients undergoing coronary angiography. J Am Soc Nephrol. (2017) 28:2201–10. doi: 10.1681/ASN.2016111162
103. Steubl D, Schneider MP, Meiselbach H, Nadal J, Schmid MC, Saritas T, et al. Association of serum uromodulin with death, cardiovascular events, and kidney failure in CKD. Clin J Am Soc Nephrol. (2020) 15:616–24. doi: 10.2215/CJN.11780919
104. Medina-Leyte DJ, Zepeda-García O, Domínguez-Pérez M, González-Garrido A, Villarreal-Molina T, Jacobo-Albavera L. Endothelial dysfunction, inflammation and coronary artery disease: potential biomarkers and promising therapeutical approaches. Int J Mol Sci. (2021) 22(8):3850. doi: 10.3390/ijms22083850
105. Onnis C, Virmani R, Kawai K, Nardi V, Lerman A, Cademartiri F, et al. Coronary artery calcification: current concepts and clinical implications. Circulation. (2024) 149:251–66. doi: 10.1161/CIRCULATIONAHA.123.065657
106. Schlieper G, Westenfeld R, Brandenburg V, Ketteler M. Inhibitors of calcification in blood and urine. Semin Dial. (2007) 20:113–21. doi: 10.1111/j.1525-139X.2007.00257.x
107. Shao JS, Cheng SL, Sadhu J, Towler DA. Inflammation and the osteogenic regulation of vascular calcification: a review and perspective. Hypertension. (2010) 55:579–92. doi: 10.1161/HYPERTENSIONAHA.109.134205
108. Macrì F, Vigorito I, Castiglione S, Faggiano S, Casaburo M, Fanotti N, et al. High phosphate-induced JAK-STAT signalling sustains vascular smooth muscle cell inflammation and limits calcification. Biomolecules. (2023) 14(1):29. doi: 10.3390/biom14010029
109. Woo SH, Kim DY, Choi JH. Roles of vascular smooth muscle cells in atherosclerotic calcification. J Lipid Atheroscler. (2023) 12:106–18. doi: 10.12997/jla.2023.12.2.106
110. Alesutan I, Luong TTD, Schelski N, Masyout J, Hille S, Schneider MP, et al. Circulating uromodulin inhibits vascular calcification by interfering with pro-inflammatory cytokine signalling. Cardiovasc Res. (2021) 117:930–41. doi: 10.1093/cvr/cvaa081
111. Bjornstad P, Wiromrat P, Johnson RJ, Sippl R, Cherney DZI, Wong R, et al. Serum uromodulin predicts less coronary artery calcification and diabetic kidney disease over 12 years in adults with type 1 diabetes: the CACTI study. Diabetes Care. (2019) 42:297–302. doi: 10.2337/dc18-1527
112. Virani SS, Newby LK, Arnold SV, Bittner V, Brewer LC, Demeter SH, et al. 2023 AHA/ACC/ACCP/ASPC/NLA/PCNA guideline for the management of patients with chronic coronary disease: a report of the American Heart Association/American College of Cardiology joint committee on clinical practice guidelines. Circulation. (2023) 148:e9–119. doi: 10.1161/CIR.0000000000001168
113. Zheng M, Ye S, Chen Y, Chen M. A study of urinary Tamm-Horsfall protein excretion in adult type 2 diabetes mellitus. Clin Nephrol. (2018) 90:40–5. doi: 10.5414/CN109220
114. Garimella PS, Lee AK, Ambrosius WT, Bhatt U, Cheung AK, Chonchol M, et al. Markers of kidney tubule function and risk of cardiovascular disease events and mortality in the SPRINT trial. Eur Heart J. (2019) 40:3486–93. doi: 10.1093/eurheartj/ehz392
115. Sharma P, Roy A, Dhamija RK, Bhushan S, Baswal K, Kulandaisamy R, et al. A comprehensive proteomic profiling of urinary exosomes and the identification of early non-invasive biomarker in patients with coronary artery disease. J Proteomics. (2024) 293:105059. doi: 10.1016/j.jprot.2023.105059
116. Garimella PS, Biggs ML, Katz R, Ix JH, Bennett MR, Devarajan P, et al. Urinary uromodulin, kidney function, and cardiovascular disease in elderly adults. Kidney Int. (2015) 88:1126–34. doi: 10.1038/ki.2015.192
117. Chen TK, Estrella MM, Appel LJ, Surapaneni AL, Köttgen A, Obeid W, et al. Associations of baseline and longitudinal serum uromodulin with kidney failure and mortality: results from the African American study of kidney disease and hypertension (AASK) trial. Am J Kidney Dis. (2024) 83:71–8. doi: 10.1053/j.ajkd.2023.05.017
118. Jian Z, Yuan C, Ma Y. Blood pressure mediated the effects of urinary uromodulin levels on myocardial infarction: a Mendelian randomization study. Hypertension. (2022) 79:2430–8. doi: 10.1161/HYPERTENSIONAHA.122.19670
119. Algharably EAH, Bolbrinker J, Lezius S, Reibis R, Wegscheider K, Völler H, et al. Uromodulin associates with cardiorenal function in patients with hypertension and cardiovascular disease. J Hypertens. (2017) 35:2053–8. doi: 10.1097/HJH.0000000000001432
120. Froehlich L, Meyre P, Aeschbacher S, Blum S, Djokic D, Kuehne M, et al. Left atrial dimension and cardiovascular outcomes in patients with and without atrial fibrillation: a systematic review and meta-analysis. Heart. (2019) 105:1884–91. doi: 10.1136/heartjnl-2019-315174
121. Zhang XF, Xu WD, Wu J. Effect of left atrial enlargement on the prognosis of acute ischemic stroke. Zhonghua Yi Xue Za Zhi. (2022) 102(9):671–4. doi: 10.3760/cma.j.cn112137-20210704-01503
122. Gard EK, Beale AL, Telles F, Silvestry FE, Hanff T, Hummel SL, et al. Left atrial enlargement is associated with pulmonary vascular disease in heart failure with preserved ejection fraction. Eur J Heart Fail. (2023) 25:806–14. doi: 10.1002/ejhf.2805
123. Bayes-Genis A, Vazquez R, Puig T, Fernandez-Palomeque C, Fabregat J, Bardají A, et al. Left atrial enlargement and NT-proBNP as predictors of sudden cardiac death in patients with heart failure. Eur J Heart Fail. (2007) 9:802–7. doi: 10.1016/j.ejheart.2007.05.001
124. Patel DA, Lavie CJ, Milani RV, Shah S, Gilliland Y. Clinical implications of left atrial enlargement: a review. Ochsner J. (2009) 9(4):191–6. doi:10.1043/1524-5012-9.4.19121603443
Keywords: uromodulin, hypertension, coronary heart disease, kidney, biomarker
Citation: Chen C, Zhong W, Zheng H, Dai G, Zhao W, Wang Y, Dong Q and Shen B (2024) The role of uromodulin in cardiovascular disease: a review. Front. Cardiovasc. Med. 11:1417593. doi: 10.3389/fcvm.2024.1417593
Received: 15 April 2024; Accepted: 25 June 2024;
Published: 9 July 2024.
Edited by:
Xiaofeng Yang, Temple University, United StatesReviewed by:
Sheon Mary, University of Glasgow, United KingdomLin Chang, University of Michigan, United States
© 2024 Chen, Zhong, Zheng, Dai, Zhao, Wang, Dong and Shen. This is an open-access article distributed under the terms of the Creative Commons Attribution License (CC BY). The use, distribution or reproduction in other forums is permitted, provided the original author(s) and the copyright owner(s) are credited and that the original publication in this journal is cited, in accordance with accepted academic practice. No use, distribution or reproduction is permitted which does not comply with these terms.
*Correspondence: Botao Shen, shenbt@jlu.edu.cn