- 1Department of Cardiology, The First Affiliated Hospital of Guangxi Medical University, Nanning, Guangxi, China
- 2Second Department of Orthopedic Rehabilitation, Taihe Hospital, Hubei University of Medicine, Shiyan, China
- 3Guangxi Key Laboratory Base of Precision Medicine in Cardio-Cerebrovascular Diseases Control and Prevention & Guangxi Clinical Research Center for Cardio-Cerebrovascular Diseases, Nanning, Guangxi, China
- 4School of Basic Medical Sciences, Guangxi Medical University, Nanning, Guangxi, China
Background: Emerging evidence indicates a significant link between various immune cell types and the development of heart valve disorders. Mitral valve prolapse (MVP) is a common condition that can lead to heart failure, arrhythmias, and even sudden death. Currently, the role of immune cells in MVP is not well understood. Thus, this study aimed to explore the causal relationship between immunophenotypes and the risk of MVP.
Methods: This study conducted a two-sample Mendelian randomization (MR) analysis to examine the link between 731 immunophenotypes and MVP. Publicly available data from genome-wide association studies were used for both the exposures and outcomes. The primary method for assessing the causal relationship between mitral valve prolapse and the 731 immunophenotypes was the inverse variance weighted method. Additionally, to ensure the MR results were reliable and valid, sensitivity analyses, including leave-one-out analysis, the Cochran Q-test, and the Egger intercept test, were conducted.
Results: The findings indicated that multiple immune cell phenotypes potentially cause changes in the risk of developing MVP. After adjusting for the false discovery rate, nine immune phenotypes were found to increase the risk of MVP, while nine others appeared to decrease it. In addition, reverse MR analysis found no causal relationship between MVP and these eighteen immunophenotypes.
Conclusion: Through genetic analyses, this research demonstrated a significant causal relationship between certain immune cells and MVP, providing new insights for future basic and clinical research.
1 Introduction
Mitral valve prolapse (MVP) is the most common form of valvular heart disease, affecting approximately 2%–3% of the general population in Western countries (1, 2). It occurs when one or both mitral valve leaflets bulge into the left atrium by at least 2 mm above the mitral annulus during systole, as observed in an echocardiogram (3, 4). Although often benign, MVP can lead to severe complications such as significant mitral regurgitation and heart failure and is a primary cause of valve repair surgery. In some cases, it may cause life-threatening ventricular arrhythmias and sudden cardiac death, alongside severe mitral regurgitation and left ventricular dysfunction (5, 6). Presently, because of a limited understanding of the mechanisms underlying MVP, only a few known preventive or recommended treatment strategies are available currently, leaving surgery as the only option once the disease advances.
For decades, clinicians have learned from infectious diseases and the immune activation that affects heart function (7, 8). Immunotherapy for cardiovascular diseases has emerged as a significant focus within the scientific community. In mouse models of myocardial infarction, transverse aortic constriction, and hypertension, mice with adoptive T cell transfers that express a chimeric antigen receptor against fibroblast activation protein showed a marked decrease in cardiac fibrosis and a restoration of function (9). Delivery of therapeutic mRNA into the body via lipid nanoparticles to form transient anti-fibrotic chimeric antigen receptor T cells also improved cardiac function in mouse models of heart failure (10). Research highlights the critical role of the immune system not only in cardiac repair but also in MVP development (11). The two primary causes identified are myxomatous MVP and fibroelastic deficiency, with various cytokines and chemokines playing a role in the disease (12). By utilizing a mouse model of Marfan syndrome (MFS) defined by a mutation in the Fibrillin-1 (FBN1) gene, researchers have discovered a connection between mitral valve pathology and the enhanced activity of transforming growth factor-β (TGF-β) (13), which plays a significant fibrotic role in MVP development. Treatment with angiotensin II blockers has been shown to reduce TGF-β activity and mitigate MVP by affecting extracellular matrix production (13, 14). MFS mice with myxomatous valve degeneration showed a notable increase in infiltrating cells expressing MHCII and CCR2, along with increased chemokine activity and changes in the inflammatory extracellular matrix were noted (15), correlating with findings in human and mouse tissues of myxomatous disease (16). A notable link between immune response and MVP was also observed in patients with inflammatory bowel disease (IBD), who had a high incidence of MVP and mitral leaflet thickening in patients with IBD (17). These findings suggest that abnormal immune responses and inflammation may be key factors in MVP pathogenesis. However, current research is limited by small sample sizes, study design issues, potential reverse causality, and unaddressed confounding factors.
Mendelian randomization (MR) is an analytical technique founded on the principle of Mendelian inheritance, predominantly used in the inference of epidemiological etiology (18). This method employs genetic variants, usually single-nucleotide polymorphisms (SNPs), as proxies for clinical interventions to assess their association with outcomes following exposure (19). MR thus enables causal inference, effectively bridging the divide between observational epidemiology and interventional trials (20). It is critical for the causal sequence in MR to be logical, as an individual's genetic code is fixed at birth. A detailed two-sample MR analysis was conducted in our study to establish the causal link between immune cell signatures and MVP.
2 Materials and methods
2.1 Study design
This study conducted a two-sample MR analysis to explore the potential causal relationship between 731 immune cell signatures (across 7 panels) and MVP. Our study design is illustrated in Figure 1. MR utilizes genetic variations to estimate the effect of exposure, relying on three critical assumptions for valid instrumental variables (IVs): (1) the genetic variation is strongly associated with the exposure; (2) the genetic variants are not associated with any confounders of the exposure-outcome relationship; (3) the genetic variation affects the outcome solely through the exposure. This study was exempt from further ethical approval or informed consent due to the use of publicly available data (21).
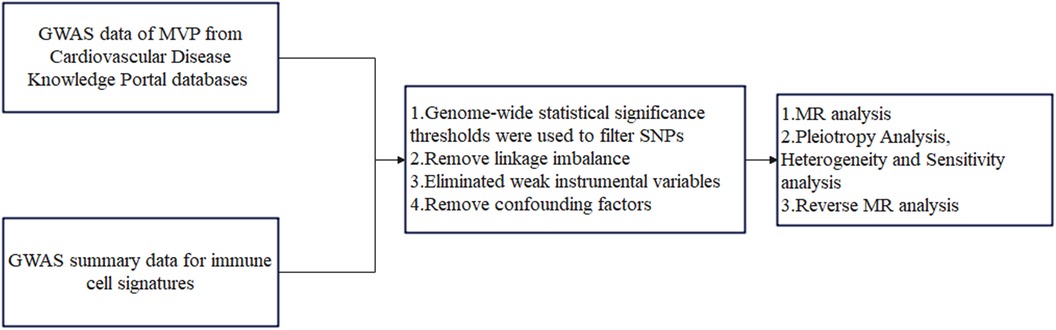
Figure 1. The flowchart of the study. MVP, mitral valve prolapse; SNPs, single-nucleotide polymorphisms; MR, Mendelian randomization.
2.2 Genome-wide association study (GWAS) summary data for MVP
Data for MVP were extracted from genome-wide association studies (GWAS) from the Cardiovascular Disease Knowledge Portal databases (https://cvd.hugeamp.org/). The data included contributions from the Framingham Heart Study (FHS), the Mass General Brigham (MGB) Biobank, the UK Biobank, the Broad Cardiovascular Disease Initiative (Broad CVDi), the MVP-FRANCE/Three-City (3C), and the MVP-NANTES/D.E.S.I.R. The GWAS involved a total of 439,533 individuals from European and American populations (4,884 cases and 434,649 controls) and approximately 7.5 million SNPs (22), leading to the identification of 14 significant (P < 5 × 10−8) SNPs associated with MVP.
2.3 Immunity-wide GWAS data sources
The GWAS Catalog (accession numbers: GCST0001391 to GCST0002121) provides public access to summary statistics for immune traits (23), encompassing a combined total of 731 immunophenotypes. These phenotypes include absolute cell counts (AC, n = 118), median fluorescence intensity (MFI, n = 389) for surface antigen levels (n = 389), morphological parameters (MP, n = 32), and relative cell counts (RC, n = 192). These measurements span various cell types and stages, including B cells, conventional dendritic cells (DCs), T-cell maturation stages, monocytes, myeloid cells, TBNK (T cells, B cells, natural killer cells), and Treg panels, with MPs focusing on cDC and TBNK panels. The immunophenotype GWAS, involving 3,757 Europeans from non-overlapping cohorts, accounted for sex, age, and the square of age as covariates. Approximately 22 million SNPs were analyzed using high-density arrays and imputed with a reference panel based on the Sardinian genome (24).
2.4 Selection of instrumental variables (IVs)
In the research (23, 25, 26), IVs were selected based on variants with P < 1 × 10−5. These SNPs were then pruned using the clumping procedure in PLINK software (version 1.90), setting the linkage disequilibrium (LD) r2 threshold to below 0.1 within a 500 kb distance. The 1,000 Genomes Projects were used as a reference panel for calculating LD r2. The proportion of phenotypic variation was explained, and the F statistic was calculated for each IV to evaluate IV strength and avoid weak instrumental bias. The equation R2 was computed using R2 = [2 × EAF (1- EAF) × β2], with EAF representing the effect allele frequency and β representing the estimated genetic influence on immunophenotypes. To assess the strength of each SNP, we calculated the F-statistic using the following equation: F = [R2 × (n−1−k)]/[(1−R2) × k]. In this equation, R2 measures how much of the phenotypic variation can be attributed to the genetic variants, k represents the total number of SNPs, and n is the sample size (27). To prevent bias from weak independent variables (IVs), only IVs with an F-value greater than 10 were retained (28). Fourteen identified specific loci were used as instrumental variables for reverse Mendelian randomization of MVP and immunophenotypes (22).
2.5 Statistical analysis
All analyses were conducted using R software version 4.3.2 (http://www.Rproject.org). The causal relationship between 731 immunophenotypes and MVP was assessed using the “TwoSampleMR” package (version 0.5.8), employing methods such as inverse variance weighted (IVW), weighted median (WM), simple mode, weighted mode, and MR Egger. The IVW method was the primary estimator for causal effects in MR analysis (29). Scatter plots confirmed that outliers did not skew the results. Given the risk of type 1 errors from multiplex testing, false discovery rate (FDR) correction was applied. To evaluate heterogeneity among chosen variables, leave-one-out analysis, funnel plots, Cochran's Q statistic, and their respective p-values were employed. The Egger intercept was used to identify the influence of horizontal pleiotropy, indicating horizontal multiplicity when statistically significant.
3 Results
3.1 Exploration of the causal effect of immunophenotypes on MVP
Two-sample MR analysis was conducted to investigate the causal effect of immunophenotypes on MVP. To correct for multiple comparisons, the FDR method was applied, setting the significance threshold at an FDR-adjusted P-value (PFDR) of 0.1. The study identified eighteen immunophenotypes across various cell panels, including five from the TBKN panel, four from the Treg panel, and two each from the B cell, T-cell maturation stages, DC panel, and monocyte cell panels, with one from the myeloid cell panel. According to Table 1, comprehensive GWAS details on eighteen immunophenotypes can be obtained. The involvement of immune cells in MVP development, as demonstrated through the IVW method, is illustrated in Figures 2, 3.
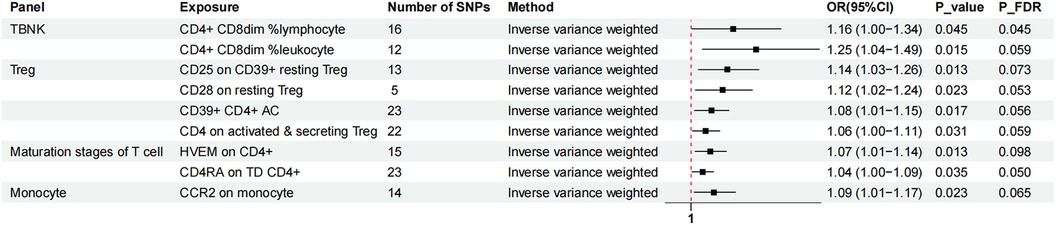
Figure 2. The forest plot showed the causal relationship in which nine immunophenotypes promoted MVP development by the IVW method. TBNK, T cells, B cells, Natural killer cells; AC, absolute cell; TD, terminally differentiated.
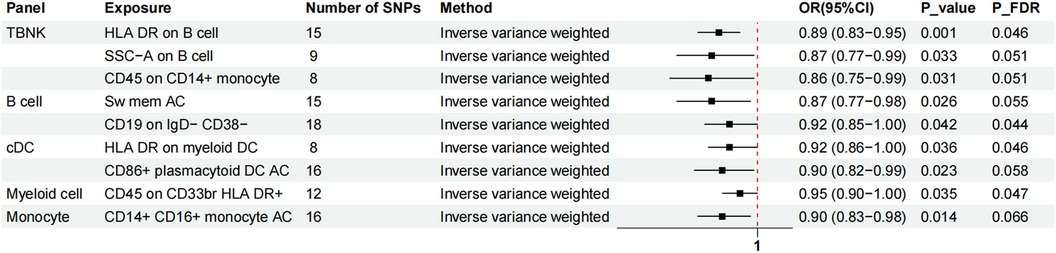
Figure 3. The forest plot showed the causal relationship in which nine additional immunophenotypes protected the development of MVP by the IVW method. TBNK, T cells, B cells, natural killer cells; AC, absolute cell; Sw mem, switched memory; DC, dendritic cell; HLA, human leucocyte antigen; br, bright.
Nine immunophenotypes were associated with increased MVP risk: CD4+ CD8dim% lymphocytes, CD4+ CD8dim% leukocytes, CCR2 on monocytes, CD25 on CD39+ resting Treg cells, CD28 on resting Treg cells, CD39+ CD4+ AC, CD4 on activated and secreting Treg cells, herpesvirus entry mediator (HVEM) on CD4+ cells, and CD4 RA on TD CD4+ cells (Figure 2).
The study utilized the IVW method to estimate the odds ratios (ORs) for various immunophenotypes and their effect on the risk of MVP. A significant association was observed for CD4+ CD8dim% lymphocytes, with an OR of 1.16 [95% confidence interval (CI) = 1.00–1.34, P = 0.045, PFDR = 0.045]. Similarly, CD4+ CD8dim% leukocytes showed an OR of 1.25 (95% CI = 1.04–1.49, P = 0.015, PFDR = 0.059). CCR2 expression on monocytes was associated with an OR of 1.09 (95% CI = 1.01–1.17, P = 0.023, PFDR = 0.065), and simple mode analysis provided consistent results (OR = 1.24, 95% CI = 1.05–1.47, P = 0.028). CD25 expression on CD39+ resting Treg cells was linked to an OR of 1.14 (95% CI = 1.03–1.26, P = 0.013, PFDR = 0.073), with MR Egger analysis indicating an OR of 1.33 (95% CI = 1.12–1.58, P = 0.008). The influence of CD28 on resting Treg cells resulted in an OR of 1.12 (95% CI = 1.02–1.24, P = 0.023, PFDR = 0.053). The OR for CD39+ CD4 + AC was 1.08 (95% CI = 1.01–1.15, P = 0.017, PFDR = 0.056), with both MR Egger (OR = 1.12, 95% CI = 1.01–1.23, P = 0.038) and WM (OR = 1.08, 95% CI = 1.00–1.16, P = 0.039) analyses supporting these findings. The OR for CD4 on activated and secreting Treg cells was 1.06 (95% CI = 1.00–1.11, P = 0.031, PFDR = 0.059). HVEM expression on CD4+ cells showed an OR of 1.07 (95% CI = 1.01–1.14, P = 0.013, PFDR = 0.098), confirmed by MR Egger (OR = 1.16, 95% CI = 1.04–1.30, P = 0.018) and WM (OR = 1.12, 95% CI = 1.03–1.21, P = 0.008) analyses. CD4 RA expression on TD CD4+ cells was associated with an OR of 1.04 (95% CI = 1.00–1.09, P = 0.035, PFDR = 0.050).
Nine immunophenotypes have been identified that provide protection against the development of MVP, including the expression of CD45 on CD33 bright HLA DR+, HLA DR on myeloid DC, CD19 on IgD− CD38−, CD14+ CD16+ monocyte ACs, CD86+ plasmacytoid DCs ACs, HLA DR on B cells, Sw mem, SSC-A on B cells, and CD45 on CD14+ monocytes (Figure 3).
The OR for CD45 on CD33br HLA DR+ in relation to MVP risk was estimated at 0.95 (95% CI = 0.90–1.00, P = 0.035, PFDR = 0.047) using the IVW method. Similarly, the OR for HLA DR on myeloid DCs and MVP risk was calculated to be 0.92 (95% CI = 0.86–1.00, P = 0.036, PFDR = 0.046) using the IVW method. For CD19 on IgD− CD38− cells, the OR was found to be 0.92 (95% CI = 0.85–1.00, P = 0.042, PFDR = 0.044), and for CD14+ CD16+ monocyte antigen-presenting cells (AC), the OR was 0.90 (95% CI = 0.83–0.98, P = 0.014, PFDR = 0.066), both estimated with the IVW method. The OR for CD86+ plasmacytoid DCs ACs on MVP risk was determined to be 0.90 (95% CI = 0.82–0.99, P = 0.023, PFDR = 0.058) using the IVW method. Similar results were observed by using two more methods: Weighted mode (OR = 1.16, 95% CI = 1.00–1.30, P = 0.018) and WM (OR = 0.85, 95% CI = 0.75–0.96, P = 0.019). For HLA DR on B cells, the OR was 0.89 (95% CI = 0.83–0.95, P = 0.001, PFDR = 0.046), and for switched memory AC, the OR was 0.87 (95% CI = 0.77–0.98, P = 0.026, PFDR = 0.055), both estimated with the IVW method. The OR for SSC-A on B cells was 0.87 (95% CI = 0.77–0.99, P = 0.033, PFDR = 0.051), and for CD45 on CD14+ monocytes, it was 0.86 (95% CI = 0.75–0.99, P = 0.031, PFDR = 0.051), also estimated using the IVW method.
To facilitate the presentation of MR analysis for these eighteen immunophenotypes, we used a circos plot for data visualization (Figure 4 and Supplementary Table S1).
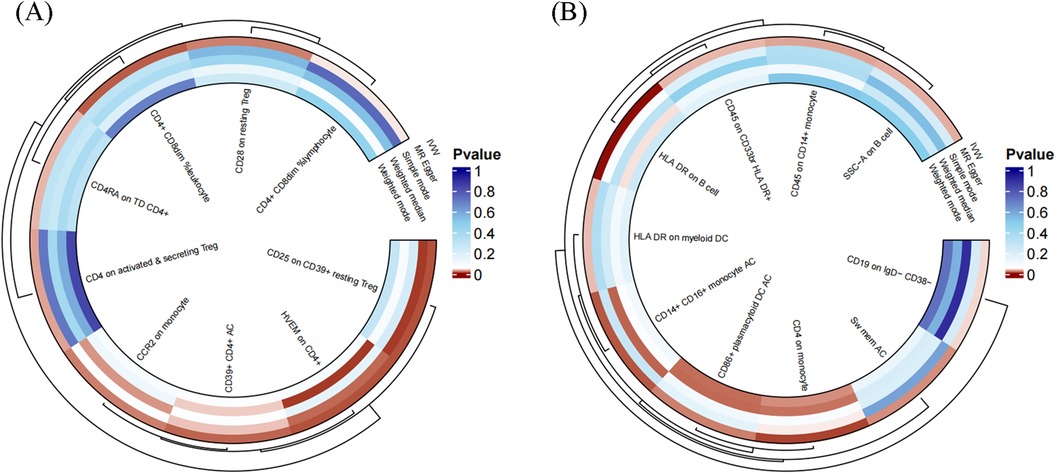
Figure 4. The MR analysis of immunophenotypes to MVP was shown by a circos plot. (A), The circos plot displayed the results of MR analysis about nine immunophenotypes promoting MVP, including inverse variance weighted, MR Egger, simple mode, weighted median, and weighted mode. (B), The circos plot displayed the results of sensitivity analyses about nine immunophenotypes protecting MVP, including inverse variance weighted, MR Egger, simple mode, weighted median, and weighted mode.
Additionally, the study utilized scatter plots, forest plots, funnel plots, and leave-one-out plots to demonstrate the robustness of these findings (Supplementary Figures S1 – S4). The assessment of diversity and horizontal pleiotropy among the eighteen immune cell types indicated no signs of horizontal pleiotropy (Table 2). However, significant heterogeneity was observed in CD4+ CD8dim% lymphocytes and leukocytes, whereas other immunophenotypes exhibited no significant heterogeneity (Table 2).
3.2 Exploration of the causal effect of MVP on immunophenotypes
We again used IVW as the primary result of reverse MR on the previous eighteen immunophenotypes. After FDR adjustment significance of 0.10, MVP had no causal relationship for any immune cell phenotype (Figure 5).
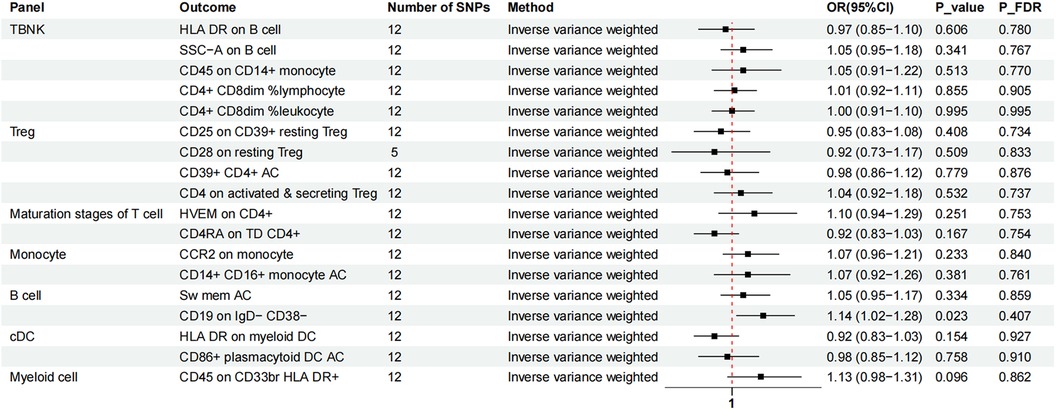
Figure 5. The forest plot showed the causal association between MVP and immunophenotypes by the IVW method. TBNK, T cells, B cells, natural killer cells; AC, absolute cell; Sw mem, switched memory; DC, dendritic cell; HLA, human leucocyte antigen; br, bright; TD, terminally differentiated.
4 Discussion
The research examined the causal relationship between 731 immunophenotypes and MVP using publicly available genetic data. Currently, limited studies focus on the association between immunophenotypes and MVP. To the best of our knowledge, this is the inaugural study to assess the connection between immunophenotypes and MVP through MR. Our results revealed a significant causal relationship between MVP and 18 immunophenotypes (PFDR < 0.1). Conversely, reverse MR analysis showed that MVP does not causally influence these 18 crucial immunophenotypes, suggesting that MVP does not interact with immunophenotypes during the disease progression. This finding preliminarily ruled out the possibility that MVP affected these 18 immunophenotypes during the disease process and reverse causation.
The findings highlighted the role of T cells (CD4+ CD8dim% lymphocyte, CD4+ CD8dim% leukocyte, HVEM on CD4+, and CD4 RA on TD CD4+) in the development of MVP. CD4+ T cells are implicated in inflammation and fibrosis during cardiac remodeling (30). In dogs with myxomatous mitral valve disease (MMVD), an increase in CD4+ CD3+ T cell counts was noted in severely affected animals, along with elevated levels of inflammatory cytokines [tumor necrosis actor-α, interleukin (IL)-1β, and IL-6] (31). Additionally, CD4+ T cells and the TGFβ1/MAPK pathway have been identified as contributors to valvular hyperplasia and fibrosis in individuals with rheumatic heart disease (32). Adult T-cell leukemia/lymphoma (ATLL), a severe mature T-cell tumor, has been observed to involve the mitral valve, showing CD4+ T-cell infiltration in chronic cases (33, 34). TNFSF14, also known as LIGHT or CD258, is crucial in immune responses, primarily through its interaction with the lymphotoxin β receptor (LTβR) and the HVEM (or TNFRSF14), both prevalent in T lymphocytes and other immune cells (35). The association between MVP and HVEM has yet to be explored. However, HVEM is known to enhance TGF-β expression and has a pro-inflammatory and pro-fibrotic role in atrial fibrillation (36), and is associated with poor prognosis in patients with heart failure (37).
Despite the anti-inflammatory role of regulatory T cells (Tregs), our findings indicate an association between Tregs (CD25 on CD39+ resting Tregs, CD28 on resting Tregs, CD4 on activated and secreting Tregs, CD39+ CD4+ AC) and an increased risk of MVP. Notably, Treg levels were higher in dogs with MMVD than in older, healthy dogs (31). Similar to Marfan syndrome, Loeys-Dietz syndrome is an autosomal dominant disorder caused by mutations in the gene encoding the TGF-β receptor subunit, which is also a cause of mitral valve prolapse. The dysregulation of TGF-β and the promotion of Smad2 and Smad3 protein phosphorylation and nuclear translocation are linked to modifications in the frequencies and functions of Tregs and treatment with losartan rescued this phenotype (38). Tregs were also found to be elevated in aortic stenosis patients, and following aortic valve intervention, the total number of Tregs decreased (39). Aligning with our analysis, these studies insinuate potential involvement of Tregs in MVP pathogenesis.
CCR2+ monocytes are involved in the myxomatous valve disease (15, 16). Notably, Na Xu et al. have proved targeting CCR2 may be a potential medical therapy in both the early and late phases of MVD in MFS by using C-C chemokine receptor type 2 genetic knockout mice and C-C chemokine receptor type 2 antagonist RS504393 (40).
The MR analysis suggested that HLA DR+ expression on myeloid cells, DCs, and B cells might offer protection against MVP. Decreased HLA DR expression on monocytes and B cells post-cardiac surgery indicates immune suppression (41). An inverse relationship between HLA DR5 and rheumatic valvular heart disease, as reported by Ozkan et al., suggests a protective role (42).
The risk of MVP may be reduced by memory B cells. Autoimmune retinopathy (AIR) is linked to autoimmunity, with primary abnormalities found in B lymphocyte differentiation. Elena Stansky and colleagues showed that patients with AIR had an increased frequency of naive B cells, while switch memory B cells and plasmablasts were significantly decreased (43). Elevated levels of (un)switched and unswitched memory B cells have been correlated with improved outcomes after carotid artery endarterectomy, suggesting that specific B-cell subgroups could act as predictors and preventers of cardiovascular events in patients with atherosclerosis (44).
Compared with CCR2+ monocytes, CD16+ monocytes, alongside CD86+ plasmacytoid DCs (pDC), may offer a protective effect on MVP. In patients with rheumatoid arthritis, the induction of CD14+ CD16+ monocytes and the differentiation of M2 macrophages, which play an anti-inflammatory role, were facilitated by platelet-derived TGF-β and monocyte-derived IL-6 (45). Moreover, non-classical monocytes may contribute to anti-atherosclerosis efforts and promote myocardial injury healing (46). Recent research has shown that in patients with heart failure before cardiac resynchronization therapy (CRT), there is a lower frequency of pDC, which increases CD86 expression post-CRT. This study observed that classical monocyte levels (associated with pro-inflammatory effects) decreased, whereas intermediate monocytes (beneficial and anti-inflammatory) and nonclassical monocytes (important for wound healing) increased post-CRT. It suggests that pDC and monocytes play roles in cardiac remodeling and respond positively to CRT (47).
This study utilized data from large GWAS cohorts to conduct a highly statistically efficient two-sample MR analysis. Various MR analysis techniques were applied to infer causality based on genetic IVs, with the results showing robustness unaffected by horizontal pleiotropy. However, several limitations were acknowledged. First, horizontal pleiotropy could not be fully assessed through multiple sensitivity analyses. Heterogeneity was observed in two immunophenotypes, attributed to differences in populations and experimental conditions. The generalizability of the results is limited to European descent, restricting applicability to other ethnic groups. Additionally, the lack of clinical and basic experiments to support the data's reliability highlights the need for various types of experiments to confirm these conclusions. Lastly, a broader threshold for data analysis was applied, potentially increasing false positives but allowing a more comprehensive evaluation of the robust correlation between the immune profile and MVP.
5 Conclusion
This MR study is believed to be the first to investigate the causal relationship between immunophenotypes and MVP, significantly reducing reverse causality and other confounding factors. Thus, it may offer new insights into basic and clinical research of MVP from an immunity standpoint.
Data availability statement
Publicly available datasets were analyzed in this study. This data can be found here: https://cvd.hugeamp.org/; https://www.ebi.ac.uk/gwas/home.
Author contributions
YW: Writing – original draft, Writing – review & editing. YS: Software, Writing – review & editing, Writing – original draft. LT: Writing – review & editing, Writing – original draft. LH: Writing – original draft, Writing – review & editing. MH: Writing – original draft, Writing – review & editing, Methodology. XZ: Writing – original draft, Writing – review & editing.
Funding
The author(s) declare financial support was received for the research, authorship, and/or publication of this article. The grants for this study were supported stage-wise by National Natural Science Foundation of China (grant no. 82260069), the China Postdoctoral Science Foundation (Grant no. 2021MD703817).
Acknowledgments
Thanks to all authors for their contributions. And we thank Bullet Edits Limited for the linguistic editing and proofreading of the manuscript.
Conflict of interest
The authors declare that the research was conducted in the absence of any commercial or financial relationships that could be construed as a potential conflict of interest.
Publisher's note
All claims expressed in this article are solely those of the authors and do not necessarily represent those of their affiliated organizations, or those of the publisher, the editors and the reviewers. Any product that may be evaluated in this article, or claim that may be made by its manufacturer, is not guaranteed or endorsed by the publisher.
Supplementary material
The Supplementary Material for this article can be found online at: https://www.frontiersin.org/articles/10.3389/fcvm.2024.1404284/full#supplementary-material
Abbreviations
AC, absolute cell; AIR, autoimmune retinopathy; ATLL, adult T-cell leukemia/lymphoma; CRT, cardiac resynchronization therapy; cDC, conventional dendritic cells; FBN1, fibrillin-1; FDR, false discovery rate; GWAS, genome-wide association study; IBD, inflammatory bowel disease; IVs, instrumental variables; IVW, inverse variance weighted; MFI, median fluorescence intensity; MFS, marfan syndrome; MMVD, myxomatous mitral valve disease; MR, Mendelian randomization; MP, morphological parameter; MVP, mitral valve prolapse; pDC, plasmacytoid dendritic cells; RC, relative cell; RHD, rheumatic heart disease; SNPs, single-nucleotide polymorphisms; TBNK, T cells, B cells, natural killer cells; TD, terminally differentiated.; TGF-β, transforming growth factor-β; WM, weighted median.
References
1. Freed LA, Levy D, Levine RA, Larson MG, Evans JC, Fuller DL, et al. Prevalence and clinical outcome of mitral-valve prolapse. N Engl J Med. (1999) 341(1):1–7. doi: 10.1056/NEJM199907013410101s
2. Caselli S, Mango F, Clark J, Pandian NG, Corrado D, Autore C, et al. Prevalence and clinical outcome of athletes with mitral valve prolapse. Circulation. (2018) 137(19):2080–2. doi: 10.1161/CIRCULATIONAHA.117.033395
3. Levine RA, Stathogiannis E, Newell JB, Harrigan P, Weyman AE. Reconsideration of echocardiographic standards for mitral valve prolapse: lack of association between leaflet displacement isolated to the apical four chamber view and independent echocardiographic evidence of abnormality. J Am Coll Cardiol. (1988) 11(5):1010–9. doi: 10.1016/s0735-1097(98)90059-6
4. Fulton BL, Liang JJ, Enriquez A, Garcia FC, Supple GE, Riley MP, et al. Imaging characteristics of papillary muscle site of origin of ventricular arrhythmias in patients with mitral valve prolapse. J Cardiovasc Electrophysiol. (2018) 29(1):146–53. doi: 10.1111/jce.13374
5. Narayanan K, Uy-Evanado A, Teodorescu C, Reinier K, Nichols GA, Gunson K, et al. Mitral valve prolapse and sudden cardiac arrest in the community. Heart Rhythm. (2016) 13(2):498–503. doi: 10.1016/j.hrthm.2015.09.026
6. Nalliah CJ, Mahajan R, Elliott AD, Haqqani H, Lau DH, Vohra JK, et al. Mitral valve prolapse and sudden cardiac death: a systematic review and meta-analysis. Heart. (2019) 105(2):144–51. doi: 10.1136/heartjnl-2017-312932
7. Corrales-Medina VF, Alvarez KN, Weissfeld LA, Angus DC, Chirinos JA, Chang CC, et al. Association between hospitalization for pneumonia and subsequent risk of cardiovascular disease. JAMA. (2015) 313(3):264–74. doi: 10.1001/jama.2014.18229
8. Knowlton KU, Badorff C. The immune system in viral myocarditis: maintaining the balance. Circ Res. (1999) 85(6):559–61. doi: 10.1161/01.res.85.6.559
9. Aghajanian H, Kimura T, Rurik JG, Hancock AS, Leibowitz MS, Li L, et al. Targeting cardiac fibrosis with engineered T cells. Nature. (2019) 573(7774):430–3. doi: 10.1038/s41586-019-1546-z
10. Rurik JG, Tombacz I, Yadegari A, Mendez Fernandez PO, Shewale SV, Li L, et al. Car T cells produced in vivo to treat cardiac injury. Science. (2022) 375(6576):91–6. doi: 10.1126/science.abm0594
11. Epelman S, Liu PP, Mann DL. Role of innate and adaptive immune mechanisms in cardiac injury and repair. Nat Rev Immunol. (2015) 15(2):117–29. doi: 10.1038/nri3800
12. van Wijngaarden SE, Abou R, Hiemstra YL, Ajmone Marsan N, Bax JJ, Delgado V. Regional left ventricular myocardial mechanics in degenerative myxomatous mitral valve disease: a comparison between fibroelastic deficiency and barlow’s disease. JACC Cardiovasc Imaging. (2018) 11(9):1362–4. doi: 10.1016/j.jcmg.2017.11.012
13. Ng CM, Cheng A, Myers LA, Martinez-Murillo F, Jie C, Bedja D, et al. Tgf-beta-dependent pathogenesis of mitral valve prolapse in a mouse model of marfan syndrome. J Clin Invest. (2004) 114(11):1586–92. doi: 10.1172/JCI22715
14. Geirsson A, Singh M, Ali R, Abbas H, Li W, Sanchez JA, et al. Modulation of transforming growth factor-Beta signaling and extracellular matrix production in myxomatous mitral valves by angiotensin ii receptor blockers. Circulation. (2012) 126(11 Suppl 1):S189–97. doi: 10.1161/CIRCULATIONAHA.111.082610
15. Kim AJ, Xu N, Umeyama K, Hulin A, Ponny SR, Vagnozzi RJ, et al. Deficiency of circulating monocytes ameliorates the progression of myxomatous valve degeneration in marfan syndrome. Circulation. (2020) 141(2):132–46. doi: 10.1161/CIRCULATIONAHA.119.042391
16. Hulin A, Anstine LJ, Kim AJ, Potter SJ, DeFalco T, Lincoln J, et al. Macrophage transitions in heart valve development and myxomatous valve disease. Arterioscler Thromb Vasc Biol. (2018) 38(3):636–44. doi: 10.1161/ATVBAHA.117.310667
17. Vizzardi E, Sciatti E, Bonadei I, Bordonali T, Ricci C, Lanzarotto F, et al. Subclinical cardiac involvement in crohn’s disease and ulcerative colitis: an echocardiographic case-control study. Panminerva Med. (2016) 58(2):115–20.26964076
18. Davey Smith G, Hemani G. Mendelian Randomization: genetic anchors for causal inference in epidemiological studies. Hum Mol Genet. (2014) 23(R1):R89–98. doi: 10.1093/hmg/ddu328
19. O'Donnell CJ, Sabatine MS. Opportunities and challenges in Mendelian randomization studies to guide trial design. JAMA Cardiol. (2018) 3(10):967. doi: 10.1001/jamacardio.2018.2863
20. Davies NM, Holmes MV, Davey Smith G. Reading Mendelian randomisation studies: a guide, glossary, and checklist for clinicians. Br Med J. (2018) 362:k601. doi: 10.1136/bmj.k601
21. Sanderson E, Glymour MM, Holmes MV, Kang H, Morrison J, Munafo MR, et al. Mendelian Randomization. Nat Rev Methods Primers. (2022) 2(1):6. doi: 10.1038/s43586-021-00092-5
22. Roselli C, Yu M, Nauffal V, Georges A, Yang Q, Love K, et al. Genome-wide association study reveals novel genetic loci: a new polygenic risk score for mitral valve prolapse. Eur Heart J. (2022) 43(17):1668–80. doi: 10.1093/eurheartj/ehac049
23. Orru V, Steri M, Sidore C, Marongiu M, Serra V, Olla S, et al. Complex genetic signatures in immune cells underlie autoimmunity and inform therapy. Nat Genet. (2020) 52(10):1036–45. doi: 10.1038/s41588-020-0684-4
24. Sidore C, Busonero F, Maschio A, Porcu E, Naitza S, Zoledziewska M, et al. Genome sequencing elucidates sardinian genetic architecture and augments association analyses for lipid and blood inflammatory markers. Nat Genet. (2015) 47(11):1272–81. doi: 10.1038/ng.3368
25. Wang C, Zhu D, Zhang D, Zuo X, Yao L, Liu T, et al. Causal role of immune cells in schizophrenia: Mendelian randomization (mr) study. BMC Psychiatry. (2023) 23(1):590. doi: 10.1186/s12888-023-05081-4
26. Ran B, Qin J, Wu Y, Wen F. Causal role of immune cells in chronic obstructive pulmonary disease: Mendelian randomization study. Expert Rev Clin Immunol. (2024) 20(4):413–21. doi: 10.1080/1744666X.2023.2295987
27. Burgess S, Davies NM, Thompson SG. Bias due to participant overlap in two-sample Mendelian randomization. Genet Epidemiol. (2016) 40(7):597–608. doi: 10.1002/gepi.21998
28. Pierce BL, Ahsan H, Vanderweele TJ. Power and instrument strength requirements for Mendelian randomization studies using multiple genetic variants. Int J Epidemiol. (2011) 40(3):740–52. doi: 10.1093/ije/dyq151
29. Bowden J, Davey Smith G, Burgess S. Mendelian Randomization with invalid instruments: effect estimation and bias detection through egger regression. Int J Epidemiol. (2015) 44(2):512–25. doi: 10.1093/ije/dyv080
30. Wei L. Immunological aspect of cardiac remodeling: t lymphocyte subsets in inflammation-mediated cardiac fibrosis. Exp Mol Pathol. (2011) 90(1):74–8. doi: 10.1016/j.yexmp.2010.10.004
31. Piantedosi D, Musco N, Palatucci AT, Carriero F, Rubino V, Pizzo F, et al. Pro-Inflammatory and immunological profile of dogs with myxomatous mitral valve disease. Vet Sci. (2022) 9(7):326. doi: 10.3390/vetsci9070326
32. Zhao Z, He D, Ling F, Chu T, Huang D, Wu H, et al. Cd4(+) T cells and Tgfbeta1/mapk signal pathway involved in the valvular hyperblastosis and fibrosis in patients with rheumatic heart disease. Exp Mol Pathol. (2020) 114:104402. doi: 10.1016/j.yexmp.2020.104402
33. Sunagawa G, Kato S, Sukehiro Y, Minematsu N, Nagatomo D, Nozoe M, et al. Severe mitral regurgitation in chronic adult T-cell leukemia/lymphoma with granulomatous valvular inflammation. J Cardiol Cases. (2023) 27(4):137–40. doi: 10.1016/j.jccase.2022.12.001
34. Aguilar C, Beltran BE, Morales D, Gutierrez-Garibay M, Villela L, Marques-Piubelli ML, et al. Isolated cardiac valve involvement in smoldering adult T-cell leukemia/lymphoma. Cardiovasc Pathol. (2023) 64:107513. doi: 10.1016/j.carpath.2022.107513
35. Ware CF, Croft M, Neil GA. Realigning the light signaling network to control dysregulated inflammation. J Exp Med. (2022) 219(7):e20220236. doi: 10.1084/jem.20220236
36. Wu Y, Zhan S, Chen L, Sun M, Li M, Mou X, et al. Tnfsf14/light promotes cardiac fibrosis and atrial fibrillation vulnerability via Pi3kgamma/Sgk1 pathway-dependent M2 macrophage polarisation. J Transl Med. (2023) 21(1):544. doi: 10.1186/s12967-023-04381-3
37. Markousis-Mavrogenis G, Tromp J, Ouwerkerk W, Ferreira JP, Anker SD, Cleland JG, et al. Multimarker profiling identifies protective and harmful immune processes in heart failure: findings from biostat-chf. Cardiovasc Res. (2022) 118(8):1964–77. doi: 10.1093/cvr/cvab235
38. Frischmeyer-Guerrerio PA, Guerrerio AL, Oswald G, Chichester K, Myers L, Halushka MK, et al. Tgfbeta receptor mutations impose a strong predisposition for human allergic disease. Sci Transl Med. (2013) 5(195):195ra94. doi: 10.1126/scitranslmed.3006448
39. Shimoni S, Bar I, Meledin V, Gandelman G, George J. Circulating regulatory T cells in patients with aortic valve stenosis: association with disease progression and aortic valve intervention. Int J Cardiol. (2016) 218:181–7. doi: 10.1016/j.ijcard.2016.05.039
40. Xu N, Yutzey KE. Therapeutic Ccr2 blockade prevents inflammation and alleviates myxomatous valve disease in marfan syndrome. JACC Basic Transl Sci. (2022) 7(11):1143–57. doi: 10.1016/j.jacbts.2022.06.001
41. Borgermann J, Friedrich I, Scheubel R, Kuss O, Lendemans S, Silber RE, et al. Granulocyte-Macrophage colony-stimulating factor (gm-csf) restores decreased monocyte hla-dr expression after cardiopulmonary bypass. Thorac Cardiovasc Surg. (2007) 55(1):24–31. doi: 10.1055/s-2006-924621
42. Ozkan M, Carin M, Sonmez G, Senocak M, Ozdemir M, Yakut C. Hla antigens in turkish race with rheumatic heart disease [see comment]. Circulation. (1993) 87(6):1974–8. doi: 10.1161/01.cir.87.6.1974
43. Stansky E, Biancotto A, Dagur PK, Gangaputra S, Chaigne-Delalande B, Nussenblatt RB, et al. B cell anomalies in autoimmune retinopathy (air). Invest Ophthalmol Vis Sci. (2017) 58(9):3600–7. doi: 10.1167/iovs.17-21704
44. Meeuwsen JAL, van Duijvenvoorde A, Gohar A, Kozma MO, van de Weg SM, Gijsberts CM, et al. High levels of (un)Switched memory B cells are associated with better outcome in patients with advanced atherosclerotic disease. J Am Heart Assoc. (2017) 6(9):e005747. doi: 10.1161/JAHA.117.005747
45. Lee SJ, Yoon BR, Kim HY, Yoo SJ, Kang SW, Lee WW. Activated platelets convert Cd14(+)Cd16(-) into Cd14(+)Cd16(+) monocytes with enhanced fcgammar-mediated phagocytosis and skewed M2 polarization. Front Immunol. (2020) 11:611133. doi: 10.3389/fimmu.2020.611133
46. Tahir S, Steffens S. Nonclassical monocytes in cardiovascular physiology and disease. Am J Physiol Cell Physiol. (2021) 320(5):C761–C70. doi: 10.1152/ajpcell.00326.2020
Keywords: Mendelian randomization analysis, mitral valve prolapse, genetic approaches, immune cells, immunophenotypes
Citation: Wang Y, Shen Y, Tan L, Hu L, He M and Zeng X (2024) Causal relationship between immunophenotypes and mitral valve prolapse: a bidirectional Mendelian randomization study. Front. Cardiovasc. Med. 11:1404284. doi: 10.3389/fcvm.2024.1404284
Received: 20 March 2024; Accepted: 23 September 2024;
Published: 3 October 2024.
Edited by:
Yufeng Yao, Huazhong University of Science and Technology, ChinaReviewed by:
Qianqian Li, First Affiliated Hospital of Zhengzhou University, ChinaMeng Yang, Huazhong University of Science and Technology, China
Kelvin Chua, The Heart and Vascular Centre, Singapore
Copyright: © 2024 Wang, Shen, Tan, Hu, He and Zeng. This is an open-access article distributed under the terms of the Creative Commons Attribution License (CC BY). The use, distribution or reproduction in other forums is permitted, provided the original author(s) and the copyright owner(s) are credited and that the original publication in this journal is cited, in accordance with accepted academic practice. No use, distribution or reproduction is permitted which does not comply with these terms.
*Correspondence: Xiaocong Zeng, aban829@163.com