- 1Department of Critical Care Medicine, Affiliated Hospital of Guangdong Medical University, Zhanjiang, China
- 2Outpatient Appointment Center, Affiliated Hospital of Guangdong Medical University, Zhanjiang, China
Septic cardiomyopathy, a life-threatening complication of sepsis, can cause acute heart failure and carry a high mortality risk. Current treatments have limitations. Fortunately, engineered exosomes, created through bioengineering technology, may represent a potential new treatment method. These exosomes can both diagnose and treat septic cardiomyopathy, playing a crucial role in its development and progression. This article examines the strategies for using engineered exosomes to protect cardiac function and treat septic cardiomyopathy. It covers three innovative aspects: exosome surface modification technology, the use of exosomes as a multifunctional drug delivery platform, and plant exosome-like nanoparticle carriers. The article highlights the ability of exosomes to deliver small molecules, proteins, and drugs, summarizing several RNA molecules, proteins, and drugs beneficial for treating septic cardiomyopathy. Although engineered exosomes are a promising biotherapeutic carrier, they face challenges in clinical application, such as understanding the interaction mechanism with host cells, distribution within the body, metabolism, and long-term safety. Further research is essential, but engineered exosomes hold promise as an effective treatment for septic cardiomyopathy.
1 Introduction
Sepsis is a life-threatening organ dysfunction caused by a dysregulated host response to infection (1). Septic cardiomyopathy is a prevalent complication of sepsis unrelated to ischemia, and has a high fatality rate (2). It commonly presents with symptoms such as ventricular dilation, reduced contractility and/or ventricular dysfunction, coupled with a diminished responsiveness to volume infusion (3, 4). Current treatment strategies primarily involve supportive care, including antibiotics and hemodynamic improvement (5, 6). Potential risk factors for septic cardiomyopathy encompass pathogen-associated molecular patterns (PAMPs), cytokines, and nitric oxide (7). The exact pathogenesis remains unclear, highlighting the need for more effective and safer therapeutic approaches for septic cardiomyopathy.
Extracellular vesicles (EVs), which are nanoscale lipid bilayer membrane structures secreted by cells (8), can be divided into two main categories based on their generation mechanisms: endosome-origin exosomes and plasma membrane-derived ectosomes (9, 10). Exosomes, as naturally occurring extracellular vesicles with diameters ranging from 30 to 200 nm, have garnered considerable interest in recent years. Found in various biological fluids, almost all eukaryotes or prokaryotes can release, these vesicles that carry a range of cellular components, including lipids, proteins, DNA, and RNA (11–14). They facilitate intercellular communication by being taken up by distant cells, influencing the function and behavior of recipient cells (15). Given their excellent biocompatibility, inherent stability, low-immunogenicity, and capacity for targeted delivery and immunomodulation (16, 17), exosomes have been as therapeutic agents for various diseases' studies and treatment (18–20).
However, naturally occurring exosomes carry different cargos, and not all of them are effective in treating diseases. For instance, certain cargoes like miR-1249-3p are known to alleviate insulin resistance and inflammation in a type 2 diabetes mouse model (21). Similarly, miR-144-3p can inhibit the growth, migration, and invasion of osteosarcoma cells (22), while miR-150-5p serves as a negative regulator of disease severity (23), thereby slowing disease progression. Conversely, some cargoes, such as miR-155-5p, contribute to severe acute pancreatitis-related intestinal barrier damage (24). miR-30d-5p induces macrophage M1 polarization and triggers macrophage pyroptosis, playing a significant role in sepsis-related acute lung injury (25). Additionally, miR-423-5p promotes cancer growth and metastasis and can be a potential diagnostic and prognostic marker for gastric cancer, posing potential risks to the body (26). Therefore, it is necessary to carry out certain modifications to exosomes to weaken their side effects and enhance the therapeutic function. At the same time, we can also modify the surface of exosomes so that they can target the heart and stay in the damaged heart for a longer period, making their therapeutic effect more significant. Research on engineered exosomes has shown their critical role in modulating the immune response to inflammation (27, 28). Furthermore, they contribute to cardiac protection by preventing cardiomyocyte apoptosis (29, 30), boosting mitochondrial function, and preserving myocardial contractility (31). Engineered exosomes hold considerable promise as a novel treatment strategy for septic cardiomyopathy, highlighting the need for comprehensive investigative efforts in this area.
This article systematically reviews the pathophysiology of septic cardiomyopathy, the characteristics of engineered exosomes, and the connection between them. The article also discusses different construction strategies of engineered exosomes in protecting cardiac function and treating septic cardiomyopathy, and analyzes the challenges faced in the development and utilization of engineered exosomes, aiming to provide reference and inspiration for future research.
2 Materials and methods
We systematically searched the PUBMED database and manually scanned the reference lists of articles. We conducted a search of the PubMed database for the most relevant articles regarding engineering exosomes, sepsis, septic cardiomyopathy, and the relationship between septic cardiomyopathy and engineering exosomes. For the search formula, we used the following terms: “(((exosomes)OR (extracellular vesicle) OR (EVs))) AND ((sepsis)OR (septic))”, “engineering exosomes”, “sepsis and EVs”, “miRNA and exosomes”, “septic cardiomyopathy”, “plant exosomes”. Initially, the PubMed database showed 594 results. In this study, a thorough evaluation of article titles was conducted to ascertain the inclusion of at least one relevant search term. Articles that did not satisfy the inclusion criteria or focused on subjects divergent from the treatment of engineering exosomes in septic cardiomyopathy were methodically excluded. Consequently, the final analysis incorporated 148 studies. The schematic diagram of the article was produced using Microsoft Office PowerPoint software. The research was conducted from a holistic viewpoint, concentrating on the treatment of engineering exosomes in sepsis.
3 Pathophysiology of septic cardiomyopathy
Septic cardiomyopathy develops from a disordered immune response to infection, a process that involves pathogen-associated molecular patterns (PAMPs) and injury-associated molecular patterns (DAMPs) that activate pattern recognition receptors and triggers a variety of intracellular pathways, such as NF-κB and mitogen-activated protein kinase pathways. Septic cardiomyopathy presents as an inflammatory state, with evidence of inflammatory cell infiltration in affected organs (32).
The field of septic cardiomyopathy research has some puzzling phenomena. Earlier studies indicated increased cardiac output in sepsis patients, suggesting that cardiac systolic function remained intact. Yet, further research revealed a reduction in left ventricular ejection fraction (EF) in these individuals (33). Intriguingly, having a reversible decrease in EF correlates with improved prognoses in contrast to maintaining stable EF levels (34). Numerous studies are exploring the mechanisms behind myocardial dysfunction in sepsis. These studies cover various aspects, such as the emergence of circulating myocardial inhibitory substances, the weakening of adrenergic pathways, the production of nitric oxide and reactive oxygen species, abnormal calcium regulation, mitochondrial dysfunction, disturbances in the coronary microvasculature, and the suppression of genetic expression for sarcomeric and mitochondrial proteins.
Patients with sepsis exhibit a non-ischemic myocardium, supported by elevated plasma troponin levels (35, 36). Studies have identified “myocardial inhibitory substances” in circulation, such as tumor necrosis factor and IL-1β, which can suppress cardiomyocyte function (37, 38). The formation of S-nitroso albumin from DAMPs and nitric oxide (NO), along with various other circulating mediators, may also contribute to the pathogenesis of septic cardiomyopathy. Sepsis-induced PAMP and DAMP signaling can initiate an inflammatory cascade via Toll-like receptor activation (39). This activation may enhance cytokine production, which can directly inhibit cardiomyocyte contraction (40). These cytokines activate inducible nitric oxide synthase (iNOS), resulting in an overproduction of NO that causes vasodilation and hypotension (41). Oxidative stress, implicated in septic cardiomyopathy, can damage myocardial cell membrane lipids, proteins, and DNA, leading to cellular dysfunction and death. Free radical scavengers have been found to improve cardiac function in sepsis mouse models (42). Overstimulation of sympathetic nerves negatively impacts myocardial performance and contractility. Inflammation-related calcium responsiveness impairment may result in myocardial contractile dysfunction (43). Severe mitochondrial dysfunction in sepsis is strongly associated with poor outcomes (44). During sepsis, local disturbances of the cardiac microcirculation may trigger a compensatory metabolic closure in the region of hypoperfusion, leading to abnormal cardiac function and energy (45). Although septic cardiomyopathy may be very severe, it is usually reversible for surviving patients (46).
Treatment of septic cardiomyopathy faces limitations, primarily focusing on managing sepsis itself through infection control, fluid resuscitation, and vasoactive drugs to maintain hemodynamic stability. Therefore, we still need the development of more comprehensive, safe, and effective treatment strategies for septic cardiomyopathy.
4 Overview of exosomes
4.1 Exosome biogenesis
Cellular secretion includes a spectrum of extracellular vesicles, with exosomes and ectosomes, each characterized by their unique origins. Exosomes are extracellular vesicles derived from endosomes with a diameter between 30 and 200 nm. The genesis of endosomes is traced back to the plasma membrane's internal budding. Exosomes are composed of proteins from the plasma membrane and Golgi apparatus, along with lipid and nucleic acid contents, encompassing cytokines, molecular patterns linked to pathogens and damage, and autoantigens. The process of segregating proteins and RNA into exosomes is stringently controlled, enabling cells to emit exosomes with varied properties based on the molecular cues triggering their synthesis. Late-stage endosomes within multivesicular bodies fuse with the cell's outer membrane, releasing their cargo, termed exosomes. Lysosomes can lead to the breakdown of multivesicular bodies. Subsequently, recipient cells internalize exosomes, which then move the activated receptors to the cell's surface or attach to these receptors to initiate signaling pathways (47). Exosome-transported mRNA can be converted into protein, while exosome-delivered miRNA can specifically target mRNA expression in the recipient cells (48).
4.2 Overview of the engineered exosomes
Engineered exosomes preserve the inherent properties of natural exosomes while acquiring additional or enhanced functions through bioengineering. These enhancements may involve targeting particular diseased tissues or cells or encapsulating specific drugs or functional RNA (49). Exosomes are primarily derived from animals, plants, and artificial synthesis. The modification of exosomes can occur via genetic engineering, chemical modifications (both covalent and non-covalent), alterations to the cell membrane, and encapsulation with biomaterials (50, 51) (Figure 1). For instance, autologous exosomes can be engineered to carry specific ligands, enabling the stable delivery of therapeutic agents (52). Exosome-loaded drugs mainly include cell transfection, direct co-incubation, sonication, electroporation, freezing and thawing, and extrusion. When compared to other biological treatments like cell and viral therapies, exosomes have the advantage of not being able to divide or replicate. This characteristic could make engineered exosome therapies comparatively safer in terms of tumorigenicity and infectivity (53–55). Furthermore, engineered exosomes have been demonstrated and well tolerated without significant side effects. Furthermore, engineered exosomes are well tolerated in without significant side effects. For instance, the study conducted by Bellavia et al. demonstrated the ability of exosomes released by HEK293T cells (Exo or IL3l-Exo) loaded with or without Imatinib to reduce tumor growth tested in an in vivo tumor xenograft model (56). Another toxicological study of MSC-Exo showed that MSC-Exo were safe without significant side effects when topical treatment on skin (57). In addition, the result of a phase I study showed that autologous dendritic cell (DC)-derived exosomes (DEX) loaded with MAGE tumor antigen were well tolerated in patients with non-small cell lung cancer (NSCLC) without evidence of severe toxicity (58).
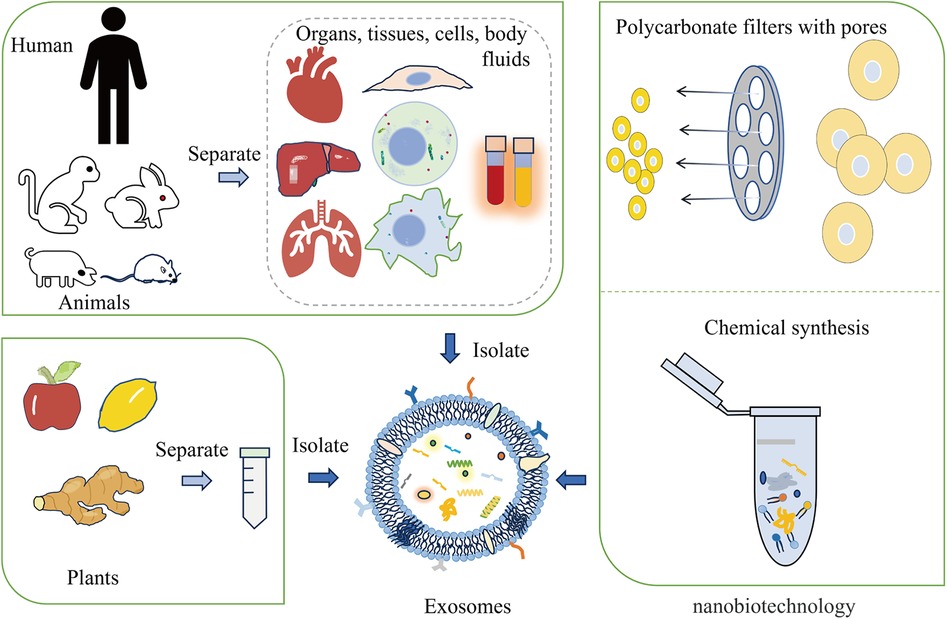
Figure 1 The main origins of exosomes include animals, plants, and nanobiotechnology techniques. Exosomes can be derived from animal organs, tissues, cells, and body fluids. Additionally, plants like apples, lemons, and ginger are capable of providing exosomes. Nanobiotechnology techniques, such as forcing cells through membrane pores or using supramolecular chemistry methods, can also generate synthetic exosomes.
5 The link between exosomes and septic cardiomyopathy
Exosomes may be linked to septic cardiomyopathy. Initially considered mere cellular waste disposal entities, they are now recognized as nanoscale intercellular communication carriers. Janiszewski et al. observed a 60% increase in platelet-derived exosomes in the plasma of septic patients compared to healthy controls. These exosomes exhibit pro-apoptotic NAD(P)H oxidase activity and can produce reactive oxygen species (ROS) via NADPH oxidase. An abundance of exosomes can lead to endothelial damage and potentially affect nearby cardiomyocytes, resulting in cardiac dysfunction (59).
Azevedo et al. analyzed blood samples from 55 septic shock patients and 12 healthy individuals. The research revealed that exosomes derived from platelets in patients with sepsis markedly reduced the myocardium contractility and hindered its function in isolated rabbit hearts (60). The NLRP3 inflammasome, activated by TXNIP, has been studied by Wang et al. They demonstrated that TXNIP-NLRP3 complexes embed in CD63 exosomes and transfer from monocytes to resident cardiac macrophages. These complexes trigger the activation of caspase-1, leading to the cleavage of IL-1β and IL-18 precursors. This process results in the secretion of the active forms of IL-1β and IL-18, contributing to the dysfunction of the inflammatory response observed in sepsis (61). Moreover, exosomes play additional roles. Zhou et al. proposed that exosomes of human mesenchymal stem cells (MSC) carry large amounts of mRNA that provides the protein PINK 1 needed to avoid calcium overload. Upon intraperitoneal injection of human MSC-derived exosomes, these mRNAs can be transported to cardiomyocytes, enhancing PINK1 expression, restoring calcium regulation, and improving myocardial function (62).
Exosomes demonstrate considerable potential in disease diagnosis (63–66). Released by nearly every cell type, these entities transport numerous molecules and are found in all types of bodily fluids. Exosomes are detectable in liquid biopsy samples, such as blood, urine, and cerebrospinal fluid. Undoubtfully, exosomes for diagnostic purposes have attracted widespread attention. Some of the identified miRNAs, for instance, miR-150-5p, miR-125b, and miR-495, may be potential markers of cardiomyopathy (23, 67, 68), and their aberrant expression is closely associated with cardiac inflammation and injury (69). Wang's study involving 214 sepsis patients assessed serum miRNAs, identifying six with significant expression differences between survivors and non-survivors: miR-223, miR-15a, miR-16, miR-122, miR-193b*, and miR-483-5p. It was observed that miR-15a, miR-122, miR-193b*, and miR-483-5p were expressed at significantly higher levels in non-survivors, while the expression of miR-223 and miR-16 decreased. Notably, miR-193b* showed a higher predictive value for sepsis mortality than SOFA scores and APACHE II scores (70). Currently, liquid biopsy methods for detecting extracellular vesicle contents are utilized in prostate cancer diagnosis (71). Similarly, studies on sepsis have demonstrated that mRNA, miRNA, or proteins found in exosomes can be potential biomarkers (Supplementary Table S1). Consequently, the detection of these miRNA can augment the specificity of early septic cardiomyopathy diagnosis.
6 Exosomes for the treatment of septic cardiomyopathy
Engineered exosomes offer significant potential for treating septic cardiomyopathy (Table 1). Firstly, they can home in on the heart, delivering drugs directly to affected areas, and enhancing therapeutic efficacy while minimizing systemic side effects (82–85). Secondly, engineered exosomes can modulate immune responses, potentially inhibiting the cardiac inflammatory response and reducing myocardial damage (86). Moreover, they possess cell-protective and repair capabilities, which could improve cardiac function by promoting cardiomyocyte survival and regeneration (87–89).
6.1 Exosome surface modification methods
Exosomes can be genetically engineered to display specific surface markers by modifying proteins or peptides (Figure 2). For instance, exosomes modified with myocardial-targeting peptides or antibodies can enhance uptake by cardiomyocytes in vitro, reduce apoptosis, and increase aggregation in myocardial tissue, thereby improving treatment outcomes (83, 90). Myocardial-targeting peptides such as WLSEAGPVVTARALRGTGSW (91, 92), APWHLSSQYSRT (93, 94), STSMLKA (95, 96), CSKTSMLKAC (84, 85, 97, 98), have specific targeted effects for cardiovascular conditions. Yang et al. discovered that STSMLKA was preserved in the ischemic myocardium after intravenous delivery. This result suggests that the engineered exosome can target the infarcted hearts after non-invasive intravenous injection, which may help with recovery after myocardial infarction (96). In addition, CDCs-EVs were engineered using a DOPE-NHS linker paired with CSTSMLKAC. in one study. By targeting exosomes to the infarcted heart, it could improve fibrosis and increase cell proliferation and angiogenesis (84). Although the exact mechanism of the interaction between CHP and myocardium is unclear, it can amplify the role of exosomes by combining CHP with exosomes. The comparison of the administration methods of engineered exosomes are listed in Supplementary Table S2.
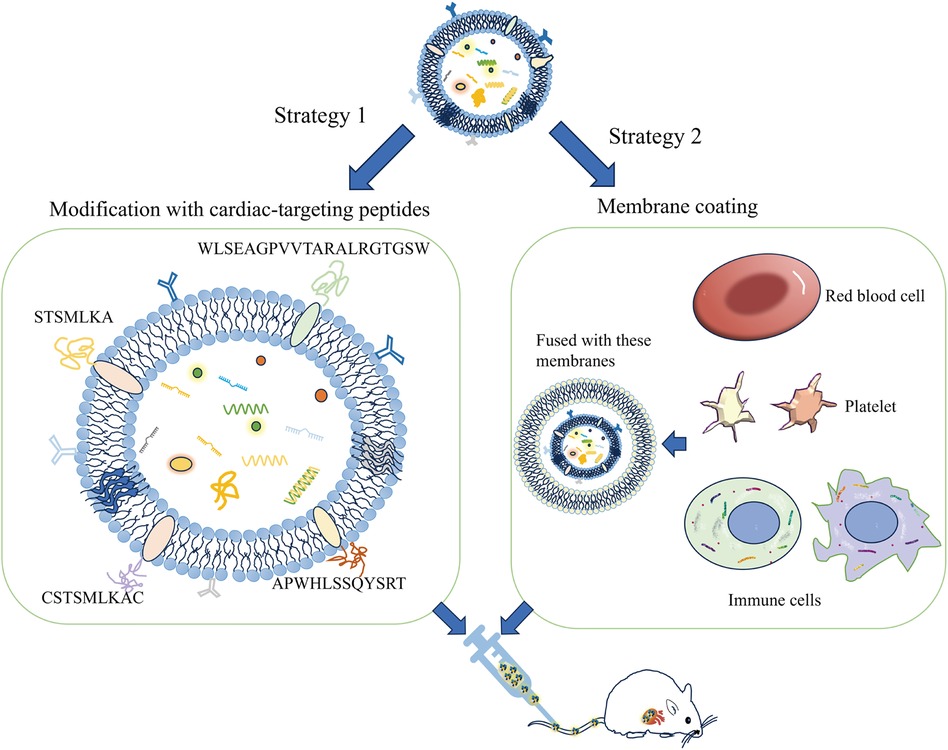
Figure 2 A schematic diagram of the construction of the exosome cardiac targeting system modified with targeting peptides and cell membranes. The cardiac targeting peptides include sequences like WLSEAGPVVTARALRGTGSW, APWHLSSQYSRT, STSMLKA, and CSKTSMLKAC. When these sequences are attached to the exosome membrane, the exosomes can be delivered to cardiomyocytes through intravenous injection. Additionally, exosomes that have fused with the membranes of red blood cells, platelets, or immune cells show a greater tendency to adhere to damaged cardiomyocytes.
In research by Gupta et al., expressing tumor necrosis factor receptor 1 (TNFR1) and interleukin 6 signaling protein (IL-6ST) on extracellular vesicles significantly mitigated systemic inflammation induced by lipopolysaccharide (LPS). These vesicles with cytokine decoys outperformed clinically approved drugs targeting TNF-α and IL-6 pathways in terms of therapeutic effects (99). Experimental evidence confirms that the CD47-SIRPα combination can activate the “don't eat me” signal, thereby inhibiting monocyte phagocytosis. Additionally, exosomes with high CD47 expression can promote immune evasion and extend their circulation half-life in mice (100, 101). MiR21-loaded CD47-EVs were efficiently delivered to cardiomyocytes, resulting in notable anti-apoptotic effects, and reduced cardiac inflammation (79). Since proteins on exosome surfaces are pivotal for biodistribution and cell targeting (102), their modification remains a promising area for ongoing investigation.
Biomimetic nanocarriers coated with cell membranes, or exosomes, leverage the native properties of the cell membrane to achieve targeted localization with remarkable efficiency (103, 104). Sources of cell membranes include red blood cells, platelets, immune cells, cancer cells, and bacterial membranes. Studies indicate that endothelial cell uptake of extracellular vesicles fused with cell membranes is increased by 2–3 times, and by 5 to 8 times in cardiomyocytes, compared to unmodified vesicles (105). Platelet membrane-modified extracellular vesicles not only mimic the binding properties of platelets and monocytes but also facilitate endosomal escape following macrophage endocytosis. They deliver miRNAs into the cytoplasm and induce a shift from the M1 to the M2 macrophage phenotype. This transition decreases the production of inflammatory factors and consequently aids in cardiac repair (106). Monocyte membrane-decorated MSC also significantly increases homing efficiency to the injured heart and improves treatment outcomes (107).
6.2 Engineered exosomes as therapeutic carriers for drug delivery
Traditional drug carriers include synthetic lipid nanoparticles and virus vectors, but their targeting ability and loading capability are relatively limited. As a source of cell nanovesicles, exosome has several merits, including good biocompatibility, stability, targeting, low immunogenicity, which make it a rare natural carrier in the field of drug delivery (16, 17). The comparison of engineered exosomes as therapeutic carriers for drug delivery is provided in Table 2.
Engineered exosomes can traverse biological barriers and transport bioactive components (Figure 3). These exosomes are capable of ferrying poorly characterized molecules and drugs, while also evading the P-glycoprotein drug efflux mechanism, which can reduce issues with drug resistance (114, 115). Thus, they are considered natural drug delivery vehicles (116–119).
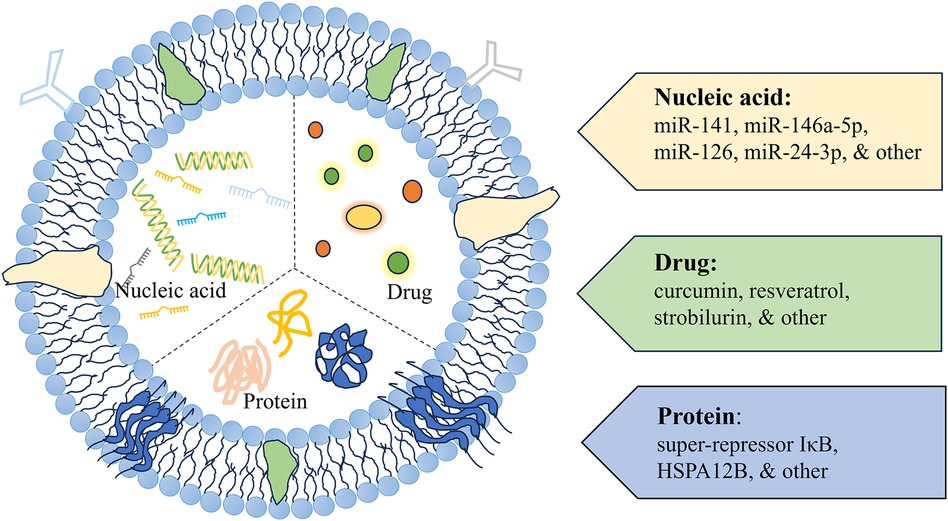
Figure 3 A schematic diagram of exosomes as delivery vehicles. Exosome delivery systems designed for cardiac therapy typically incorporate modifications with nucleic acids, drugs, and proteins.
6.2.1 Using exosomes to load nucleic acid substances such as miRNAs
Research increasingly indicates that microRNAs (miRNAs) are key in driving the healing properties of exosomes (120). The dysregulation of miRNAs is linked to a variety of illnesses, including but not limited to cancer, diabetes, obesity, viral infections, and diseases of the cardiovascular system (121–123). Real and co-authors observed a stark contrast in the miRNA content of exosomes from those with septic shock compared to healthy volunteers. For example, miR-27a levels in exosomes from sepsis survivors were sixfold higher than in controls, suggesting a possible role for exosomal miRNAs in sepsis pathogenesis, including the inflammatory response, oxidative stress, and cell cycle regulation (124). MiRNA-21 has been shown to protect against sepsis-induced cardiac dysfunction, and its upregulation could be a potential strategy for treating septic cardiomyopathy (125). Exosomes can shield miR-21 from RNase degradation and deliver it effectively to target cells, thus decreasing PDCD4 protein levels, reducing apoptosis, and promoting cardiac recovery (80).
Wang et al. demonstrated that miR-223-KO mesenchymal stem cell exosome injection did not improve cardiac function or survival rates in septic mice, highlighting the importance of miR-223 in mediating the protective effects of mesenchymal stem cells on septic cardiomyopathy by downregulating Sema3A and Stat proteins (72). Pei et al. discovered that pre-injecting miR-141-enriched mesenchymal stem cell exosomes could restore myocardial function in a CLP sepsis mouse model, as evidenced by improvements in left ventricular ejection fraction and short-axis shortening (73). Additionally, Liu C et al. confirmed that miR-146a-5p, carried by mesenchymal cell exosomes, has protective effects in cardiomyocytes and myocardial tissue in sepsis models by negatively regulating MYBL1 (74). Zhang et al. also discovered that overexpression of miR-146 in exosomes from bone marrow stromal cells reduced the expression of adhesion molecules during sepsis. This led to a decrease in macrophage and neutrophil accumulation in the myocardium and ameliorated septic cardiomyopathy (75).
Moreover, overexpression of miR-181b has been found to downregulate HMGB1 expression in septic rats, leading to reduced inflammatory factors and myocardial damage, and inhibited cardiomyocyte apoptosis (126). In another study, the cardioprotective effects were attributed to the transfer of miR-181b via exosomes from cardiosphere-derived cells (CDCs) to macrophages, which subsequently reduced PKCδ transcript levels. Notably, while exosomes from fibroblasts alone did not confer protection in this model, those loaded with miR-181b were capable of altering the macrophage phenotype and providing cardioprotection. Conversely, inhibiting miR-181b in CDC exosomes diminishes their cardioprotective properties (81, 127).
In addition, mRNA and other nucleic acid drugs have great potential in the treatment of diseases. However, some characteristics of RNA molecules, such as instability in the body and difficulty in crossing cell membranes and blood-brain barriers, hinder the further application of nucleic acid drugs. Therefore, there are now studies on using exosomes to load mRNA to treat diseases. In innovative research, the LiuM team developed inhalable IL-12mRNA-loaded exosomes IL-12-Exo (128); Kojima et al. focused on the treatment of Parkinson's disease and attempted to deliver catalase mRNA through designed exosomes. to the brain (129); while Wang et al. used exosomes to deliver HChrR6-encoding mRNA to HER2+ cells (130); Similarly, Usman et al. experimented with red blood cell extracellular vesicles filled with Cas9 mRNA and gRNA, targeting the mir-125b-2 site to combat acute myeloid leukemia in MOLM13 cells (131). These pioneering studies highlight the versatility and potential of exosomes as carriers for mRNA delivery in various therapeutic contexts.
Overall, these studies collectively illustrate the transformative potential of exosome-based nucleic acid delivery systems in various medical fields. Continued research and development in this area could lead to significant breakthroughs in the treatment of septic cardiomyopathy, offering new hope for patients. The challenge now lies in optimizing these delivery systems for clinical use, ensuring their safety, efficacy, and scalability for widespread application.
6.2.2 Using exosomes to load proteins
Engineered exosomes can be utilized to deliver proteins, such as HSP60, which function as cytoprotective molecules to alleviate cell damage caused by oxidative stress and inflammatory agents. The study revealed that endothelial heat shock protein A12B (HSPA12B) ameliorates cardiac dysfunction in sepsis and decreases mortality (75, 76). Tu F et al. discovered that exosomes enriched with endothelial cell-derived HSPA12B were shown to inhibit NF-κB activation in LPS-stimulated macrophages. Choi H et al. engineered exosomes (EXPLOR) carrying srIκB, a stable form of IκBα, to create immunosuppressive exosomes. These exosomes blocked NF-κB-mediated gene transcription in the nucleus. In a sepsis mouse model, the application of Exo-srIκB effectively decreased levels of inflammatory markers such as TNF-α, IL-1β, and IL-6, be-sides reducing organ damage (77). Additionally, delivering HSP60 and antioxidant enzymes via exosomes has shown promise in preventing cardiomyocyte damage (86).
6.2.3 Using exosomes to load chemical drugs
In treating septic cardiomyopathy, exosomes can be engineered to transport anti-inflammatory drugs or antioxidants directly to the damaged myocardium, thereby reducing the effects of inflammatory mediators and protecting cardiac cells from further injury (16). Kang JY and his colleagues genetically modified the parent cells of extracellular vesicles (EVs) to display a cardiac targeting peptide (CTP) on the exosome surface. They loaded curcumin into CTP-modified EVs, which delivered curcumin specifically to the heart. These curcumin-loaded EVs exhibited increased bioavailability and enhanced cardio-protection (94). They also co-delivered curcumin and miR-144-3p using CTP-EVs, which not only preserved cardiac targeting capabilities but also significantly improved therapeutic outcomes both in vitro and in vivo. In another study, Zheng et al. used folic acid-functionalized macrophage-derived exosomes to co-load two anti-inflammatory drugs (resveratrol, strobilurin) to inhibit LPS-induced sepsis and protect against Lung function, exosomes showed strong anti-inflammatory and immunosuppressive activities, and multiple administrations significantly enhanced the protective effect and resisted the second hit of LPS (132).
Several drugs have been identified to ameliorate septic cardiomyopathy at the molecular level. Puerarin mitigates inflammation and oxidative stress in myocardial tissues, and inhibits apoptosis and ferroptosis in cardiomyocytes (133). Emodin reduces the inflammatory response and pyroptosis in cardiomyocytes by suppressing the activation of the NLRP3 inflammasome (134). Capsaicin enhances 14-3-3γ-mediated autophagy, alleviating LPS-induced myocardial injury and dysfunction (135). Therefore, we have high hopes for the treatment of septic cardiomyopathy using drugs that are loaded with these beneficial effects on cardiac function.
6.3 Using plant-derived exosome-like nanoparticles as therapeutic vehicles
The source of the exosomes greatly influences their effectiveness. Exosomes from inflammatory cells have different biological functions compared to those from mesenchymal stem cells (MSCs). Research has revealed that MSC-derived exosomes have immunomodulatory and regenerative properties akin to MSCs themselves. They can improve the viability of cardiomyocytes post-ischemia/reperfusion injury and exhibit low immunogenicity (78). Numerous studies are currently developing therapeutic drugs based on MSC-EVs (124, 136).
Due to the limited production of exosomes from mammalian cells, researchers have begun to isolate exosomes from fruits or vegetables, such as grapefruits, broccoli, and ginger. These plant-derived exosomes are being explored for treatment of diagnosed diseases (137–139). While the application of plant exosomes for septic cardiomyopathy treatment remains unexplored, current research highlights their ability to deliver a wide range of therapeutic agents—including chemotherapy drugs, siRNA, DNA expression vectors, and proteins—to target cells, demonstrating therapeutic benefits in mouse models (140). Xu XH and his colleagues discovered that exosome nanovesicles derived from ginseng (G-Exos) can serve as efficient and safe carriers for delivering active miRNAs to BMSCs and inducing their differentiation into neural cells. This study has shown promising results in promoting nerve regeneration and repairing conduction function both in vitro and in vivo (141). Additionally, according to Teng et al., ginger-derived exocrine-like particles contain small RNA that can affect the intestinal microflora of mice, thereby improving intestinal barrier function and reducing the incidence of colitis (138). Ju et al. pointed out that exocrine-like nanoparticles extracted from grape dregs can avoid the degradation of digestive enzymes in mice and promote the proliferation of intestinal epithelial cells after entering the intestine, thus accelerating the recovery of colitis (142).
In conclusion, plant-derived exosome-like nanoparticles may hold therapeutic potential for septic cardiomyopathy. Therefore, further exploration and validation of the role of plant exosomes in septic cardiomyopathy treatment are warranted.
7 Discussion
Exosomes, either inherently or as drug delivery carriers, have garnered wide-spread attention in the diagnosis and treatment of sepsis and cardiovascular diseases (25, 143–145), spearheading a new trend in the biopharmaceutical industry. Engineered exosomes, modified through biotechnological techniques, not only retain their original biological properties but are also endowed with specific functions, enhancing their original capabilities. This positions them as potentially valuable in treating septic cardiomyopathy. This review systematically summarizes the research progress of engineered exosomes in septic cardiomyopathy, innovatively discussing their applications in exosome surface modification techniques, as multi-functional drug delivery platforms, and plant exosome-like nanoparticle carriers. It emphasizes the potential of exosomes in delivering small molecules, proteins, and drugs, and summarizes a collection of RNA, proteins, and drugs beneficial for treating septic cardiomyopathy.
However, despite advances in developing engineered exosomes, multiple challenges remain in their clinical application (112). Enhancing the efficiency and purity of exosome culture, isolation, and purification processes is critical. Large-scale production, quality control, improved targeting, and increased drug loading efficiency must be addressed to meet clinical standards (146). Additionally, standardizing production and storage conditions is essential to maintain exosome stability and biological function. Determining suitable delivery methods and ensuring the biocompatibility and safety of these biomaterials in the human body is also required (147).
Further investigative work is crucial to uncover the interaction mechanisms between exosomes and host cells, their distribution, metabolism, and long-term safety (148). Current knowledge gaps regarding exosome dynamics and targeting in different organisms add to the uncertainty of their clinical translation. Researchers are actively seeking solutions to develop engineered exosomes into effective therapeutic tools.
Engineered exosomes are an innovative biotherapeutic carrier with significant potential in septic cardiomyopathy treatment research. They offer modulation of inflammatory responses, enhancement of myocardial repair, and targeted drug delivery. These capabilities suggest engineered exosomes as a promising direction for new therapeutic strategy development.
Author contributions
LM: Conceptualization, Investigation, Writing – original draft. SL: Data curation, Visualization, Writing – original draft. YC: Validation, Writing – original draft. HH: Investigation, Writing – original draft. FD: Writing – review & editing. LD: Funding acquisition, Writing – review & editing.
Funding
The author(s) declare financial support was received for the research, authorship, and/or publication of this article.
This work was supported by the grants from National Natural Science Foundation of China (82172148 and 81974298 to LD).
Conflict of interest
The authors declare that the research was conducted in the absence of any commercial or financial relationships that could be construed as a potential conflict of interest.
Publisher's note
All claims expressed in this article are solely those of the authors and do not necessarily represent those of their affiliated organizations, or those of the publisher, the editors and the reviewers. Any product that may be evaluated in this article, or claim that may be made by its manufacturer, is not guaranteed or endorsed by the publisher.
Supplementary material
The Supplementary Material for this article can be found online at: https://www.frontiersin.org/articles/10.3389/fcvm.2024.1399738/full#supplementary-material
References
1. Singer M, Deutschman CS, Seymour CW, Shankar-Hari M, Annane D, Bauer M, et al. The third international consensus definitions for sepsis and septic shock (sepsis-3). JAMA. (2016) 315(8):801–10. doi: 10.1001/jama.2016.0287
2. Carbone F, Liberale L, Preda A, Schindler TH, Montecucco F. Septic cardiomyopathy: from pathophysiology to the clinical setting. Cells. (2022) 11:18. doi: 10.3390/cells11182833
3. Beesley SJ, Weber G, Sarge T, Nikravan S, Grissom CK, Lanspa MJ, et al. Septic cardiomyopathy. Crit Care Med. (2018) 46(4):625–34. doi: 10.1097/CCM.0000000000002851
4. Martin L, Derwall M, Al Zoubi S, Zechendorf E, Reuter DA, Thiemermann C, et al. The septic heart: current understanding of molecular mechanisms and clinical implications. Chest. (2019) 155(2):427–37. doi: 10.1016/j.chest.2018.08.1037
5. Rivers E, Nguyen B, Havstad S, Ressler J, Muzzin A, Knoblich B, et al. Early goal-directed therapy in the treatment of severe sepsis and septic shock. N Engl J Med. (2001) 345(19):1368–77. doi: 10.1056/NEJMoa010307
6. Evans L, Rhodes A, Alhazzani W, Antonelli M, Coopersmith CM, French C, et al. Surviving sepsis campaign: international guidelines for management of sepsis and septic shock 2021. Crit Care Med. (2021) 49(11):e1063–143. doi: 10.1097/CCM.0000000000005337
7. Hollenberg SM, Singer M. Pathophysiology of sepsis-induced cardiomyopathy. Nat Rev Cardiol. (2021) 18(6):424–34. doi: 10.1038/s41569-020-00492-2
8. Debbi L, Guo S, Safina D, Levenberg S. Boosting extracellular vesicle secretion. Biotechnol Adv. (2022) 59:107983. doi: 10.1016/j.biotechadv.2022.107983
9. van Niel G, D'Angelo G, Raposo G. Shedding light on the cell biology of extracellular vesicles. Nat Rev Mol Cell Biol. (2018) 19(4):213–28. doi: 10.1038/nrm.2017.125
10. Dixson AC, Dawson TR, Di Vizio D, Weaver AM. Context-specific regulation of extracellular vesicle biogenesis and cargo selection. Nat Rev Mol Cell Biol. (2023) 24(7):454–76. doi: 10.1038/s41580-023-00576-0
11. Thakur A, Qiu G, Ng S-P, Guan J, Yue J, Lee Y, et al. Direct detection of two different tumor-derived extracellular vesicles by SAM-AuNIs LSPR biosensor. Biosens Bioelectron. (2017) 94:400–7. doi: 10.1016/j.bios.2017.03.036
12. Thakur A, Qiu G, Xu C, Han X, Yang T, Ng SP, et al. Label-free sensing of exosomal MCT1 and CD147 for tracking metabolic reprogramming and malignant progression in glioma. Sci Adv. (2020) 6(26):eaaz6119. doi: 10.1126/sciadv.aaz6119
13. Thakur A, Sidu RK, Zou H, Alam MK, Yang M, Lee Y. Inhibition of glioma cells’ proliferation by doxorubicin-loaded exosomes via microfluidics. Int J Nanomed. (2020) 15:8331–43. doi: 10.2147/IJN.S263956
14. Iyaswamy A, Thakur A, Guan X-J, Krishnamoorthi S, Fung TY, Lu K, et al. Fe65-engineered neuronal exosomes encapsulating corynoxine-B ameliorate cognition and pathology of Alzheimer’s disease. Signal Transduct Target Ther. (2023) 8(1):404. doi: 10.1038/s41392-023-01657-4
15. van Niel G, Carter DRF, Clayton A, Lambert DW, Raposo G, Vader P. Challenges and directions in studying cell-cell communication by extracellular vesicles. Nat Rev Mol Cell Biol. (2022) 23(5):369–82. doi: 10.1038/s41580-022-00460-3
16. Lener T, Gimona M, Aigner L, Börger V, Buzas E, Camussi G, et al. Applying extracellular vesicles based therapeutics in clinical trials—an ISEV position paper. J Extracell Vesicles. (2015) 4:30087. doi: 10.3402/jev.v4.30087
17. Fais S, O'Driscoll L, Borras FE, Buzas E, Camussi G, Cappello F, et al. Evidence-based clinical use of nanoscale extracellular vesicles in nanomedicine. ACS Nano. (2016) 10(4):3886–99. doi: 10.1021/acsnano.5b08015
18. Dong S, Liu X, Bi Y, Wang Y, Antony A, Lee D, et al. Adaptive design of mRNA-loaded extracellular vesicles for targeted immunotherapy of cancer. Nat Commun. (2023) 14(1):6610. doi: 10.1038/s41467-023-42365-5
19. Gong L, Tian L, Cui K, Chen Y, Liu B, Li D, et al. An off-the-shelf small extracellular vesicle nanomedicine for tumor targeting therapy. J Control Release. (2023) 364:672–86. doi: 10.1016/j.jconrel.2023.11.013
20. Liu J, Ren H, Zhang C, Li J, Qiu Q, Zhang N, et al. Orally-delivered, cytokine-engineered extracellular vesicles for targeted treatment of inflammatory bowel disease. Small. (2023) 19(50):e2304023. doi: 10.1002/smll.202304023
21. Wang Y, Li M, Chen L, Bian H, Chen X, Zheng H, et al. Natural killer cell-derived exosomal miR-1249-3p attenuates insulin resistance and inflammation in mouse models of type 2 diabetes. Signal Transduct Target Ther. (2021) 6(1):409. doi: 10.1038/s41392-021-00805-y
22. Jiang M, Jike Y, Liu K, Gan F, Zhang K, Xie M, et al. Exosome-mediated miR-144-3p promotes ferroptosis to inhibit osteosarcoma proliferation, migration, and invasion through regulating ZEB1. Mol Cancer. (2023) 22(1):113. doi: 10.1186/s12943-023-01804-z
23. Ye R, Lin Q, Xiao W, Mao L, Zhang P, Zhou L, et al. miR-150-5p in neutrophil-derived extracellular vesicles associated with sepsis-induced cardiomyopathy in septic patients. Cell Death Discov. (2023) 9(1):19. doi: 10.1038/s41420-023-01328-x
24. Shao Y, Li Y, Jiang Y, Li H, Wang J, Zhang D. Circulating exosomal miR-155-5p contributes to severe acute pancreatitis-associated intestinal barrier injury by targeting SOCS1 to activate NLRP3 inflammasome-mediated pyroptosis. FASEB J. (2023) 37(6):e23003. doi: 10.1096/fj.202300237R
25. Jiao Y, Zhang T, Zhang C, Ji H, Tong X, Xia R, et al. Exosomal miR-30d-5p of neutrophils induces M1 macrophage polarization and primes macrophage pyroptosis in sepsis-related acute lung injury. Crit Care. (2021) 25(1):356. doi: 10.1186/s13054-021-03775-3
26. Yang H, Fu H, Wang B, Zhang X, Mao J, Li X, et al. Exosomal miR-423-5p targets SUFU to promote cancer growth and metastasis and serves as a novel marker for gastric cancer. Mol Carcinog. (2018) 57(9):1223–36. doi: 10.1002/mc.22838
27. Robbins PD, Morelli AE. Regulation of immune responses by extracellular vesicles. Nat Rev Immunol. (2014) 14(3):195–208. doi: 10.1038/nri3622
28. Gao K, Jin J, Huang C, Li J, Luo H, Li L, et al. Exosomes derived from septic mouse serum modulate immune responses via exosome-associated cytokines. Front Immunol. (2019) 10:1560. doi: 10.3389/fimmu.2019.01560
29. Xiao J, Pan Y, Li XH, Yang XY, Feng YL, Tan HH, et al. Cardiac progenitor cell-derived exosomes prevent cardiomyocytes apoptosis through exosomal miR-21 by targeting PDCD4. Cell Death Dis. (2016) 7(6):e2277. doi: 10.1038/cddis.2016.181
30. Sun X, Liu Y, Wang J, Zhang M, Wang M. Cardioprotection of M2 macrophages-derived exosomal microRNA-24-3p/Tnfsf10 axis against myocardial injury after sepsis. Mol Immunol. (2022) 141:309–17. doi: 10.1016/j.molimm.2021.11.003
31. Mayourian J, Ceholski DK, Gorski PA, Mathiyalagan P, Murphy JF, Salazar SI, et al. Exosomal microRNA-21-5p mediates mesenchymal stem cell paracrine effects on human cardiac tissue contractility. Circ Res. (2018) 122(7):933–44. doi: 10.1161/CIRCRESAHA.118.312420
32. Rossi MA, Celes MRN, Prado CM, Saggioro FP. Myocardial structural changes in long-term human severe sepsis/septic shock may be responsible for cardiac dysfunction. Shock (Augusta, Ga.). (2007) 27(1):10–8. doi: 10.1097/01.shk.0000235141.05528.47
33. Parker MM, Shelhamer JH, Bacharach SL, Green MV, Natanson C, Frederick TM, et al. Profound but reversible myocardial depression in patients with septic shock. Ann Intern Med. (1984) 100(4):483–90. doi: 10.7326/0003-4819-100-4-483
34. Parker MM, Suffredini AF, Natanson C, Ognibene FP, Shelhamer JH, Parrillo JE. Responses of left ventricular function in survivors and nonsurvivors of septic shock. J Crit Care. (1989) 4(1):19–25. doi: 10.1016/0883-9441(89)90087-7
35. Hollenberg SM, Ahrens TS, Annane D, Astiz ME, Chalfin DB, Dasta JF, et al. Practice parameters for hemodynamic support of sepsis in adult patients: 2004 update. Crit Care Med. (2004) 32(9):1928–48. doi: 10.1097/01.CCM.0000139761.05492.D6
36. Oh JK, Park S-J, Nagueh SF. Established and novel clinical applications of diastolic function assessment by echocardiography. Circ Cardiovasc Imaging. (2011) 4(4):444–55. doi: 10.1161/CIRCIMAGING.110.961623
37. Singer M. Catecholamine treatment for shock–equally good or bad? Lancet (London, England). (2007) 370(9588):636–7. doi: 10.1016/S0140-6736(07)61317-8
38. Landesberg G, Gilon D, Meroz Y, Georgieva M, Levin PD, Goodman S, et al. Diastolic dysfunction and mortality in severe sepsis and septic shock. Eur Heart J. (2012) 33(7):895–903. doi: 10.1093/eurheartj/ehr351
39. Carluccio E, Biagioli P, Alunni G, Murrone A, Zuchi C, Coiro S, et al. Prognostic value of right ventricular dysfunction in heart failure with reduced ejection fraction: superiority of longitudinal strain over tricuspid annular plane systolic excursion. Circ Cardiovasc Imaging. (2018) 11(1):e006894. doi: 10.1161/CIRCIMAGING.117.006894
40. Kumar A, Thota V, Dee L, Olson J, Uretz E, Parrillo JE. Tumor necrosis factor alpha and interleukin 1beta are responsible for in vitro myocardial cell depression induced by human septic shock serum. J Exp Med. (1996) 183(3):949–58. doi: 10.1084/jem.183.3.949
41. Kakihana Y, Ito T, Nakahara M, Yamaguchi K, Yasuda T. Sepsis-induced myocardial dysfunction: pathophysiology and management. J Intensive Care. (2016) 4:22. doi: 10.1186/s40560-016-0148-1
42. Haileselassie B, Su E, Pozios I, Niño DF, Liu H, Lu D-Y, et al. Myocardial oxidative stress correlates with left ventricular dysfunction on strain echocardiography in a rodent model of sepsis. Intensive Care Med Exp. (2017) 5(1):21. doi: 10.1186/s40635-017-0134-5
43. Zhang C, Mo M, Ding W, Liu W, Yan D, Deng J, et al. High-mobility group box 1 (HMGB1) impaired cardiac excitation-contraction coupling by enhancing the sarcoplasmic reticulum (SR) ca(2+) leak through TLR4-ROS signaling in cardiomyocytes. J Mol Cell Cardiol. (2014) 74:260–73. doi: 10.1016/j.yjmcc.2014.06.003
44. Pinto BB, Dyson A, Umbrello M, Carré JE, Ritter C, Clatworthy I, et al. Improved survival in a long-term rat model of sepsis is associated with reduced mitochondrial calcium uptake despite increased energetic demand. Crit Care Med. (2017) 45(8):e840–8. doi: 10.1097/CCM.0000000000002448
45. Hollenberg SM. Think locally: evaluation of the microcirculation in sepsis. Intensive Care Med. (2010) 36(11):1807–9. doi: 10.1007/s00134-010-1973-7
46. Bouhemad B, Nicolas-Robin A, Arbelot C, Arthaud M, Féger F, Rouby J-J. Isolated and reversible impairment of ventricular relaxation in patients with septic shock. Crit Care Med. (2008) 36(3):766–74. doi: 10.1097/CCM.0B013E31816596BC
47. Barile L, Moccetti T, Marbán E, Vassalli G. Roles of exosomes in cardioprotection. Eur Heart J. (2017) 38(18):1372–9. doi: 10.1093/eurheartj/ehw304
48. Koppers-Lalic D, Hackenberg M, Bijnsdorp IV, van Eijndhoven MAJ, Sadek P, Sie D, et al. Nontemplated nucleotide additions distinguish the small RNA composition in cells from exosomes. Cell Rep. (2014) 8(6):1649–58. doi: 10.1016/j.celrep.2014.08.027
49. Vader P, Mol EA, Pasterkamp G, Schiffelers RM. Extracellular vesicles for drug delivery. Adv Drug Delivery Rev. (2016) 106(Pt A):148–56. doi: 10.1016/j.addr.2016.02.006
50. Li Y-J, Wu J-Y, Liu J, Xu W, Qiu X, Huang S, et al. Artificial exosomes for translational nanomedicine. J Nanobiotechnol. (2021) 19(1):242. doi: 10.1186/s12951-021-00986-2
51. Jiang J, Zhang X, Wang H, Spanos M, Jiang F, Ni L, et al. Closer to the heart: harnessing the power of targeted extracellular vesicle therapies. Adv Biol. (2023) 8(2):e2300141. doi: 10.1002/adbi.202300141
52. Santiard-Baron D, Langui D, Delehedde M, Delatour B, Schombert B, Touchet N, et al. Expression of human FE65 in amyloid precursor protein transgenic mice is associated with a reduction in beta-amyloid load. J Neurochem. (2005) 93(2):330–8. doi: 10.1111/j.1471-4159.2005.03026.x
53. Khan M, Nickoloff E, Abramova T, Johnson J, Verma SK, Krishnamurthy P, et al. Embryonic stem cell-derived exosomes promote endogenous repair mechanisms and enhance cardiac function following myocardial infarction. Circ Res. (2015) 117(1):52–64. doi: 10.1161/CIRCRESAHA.117.305990
54. Conlan RS, Pisano S, Oliveira MI, Ferrari M, Mendes Pinto I. Exosomes as reconfigurable therapeutic systems. Trends Mol Med. (2017) 23(7):636–50. doi: 10.1016/j.molmed.2017.05.003
55. Wiklander OPB, Brennan MÁ, Lötvall J, Breakefield XO, El Andaloussi S. Advances in therapeutic applications of extracellular vesicles. Sci Transl Med. (2019) 11(492):eaav8521. doi: 10.1126/scitranslmed.aav8521
56. Bellavia D, Raimondo S, Calabrese G, Forte S, Cristaldi M, Patinella A, et al. Interleukin 3- receptor targeted exosomes inhibit in vitro and in vivo chronic myelogenous leukemia cell growth. Theranostics. (2017) 7(5):1333–45. doi: 10.7150/thno.17092
57. Lee J, Ha D, Cho B, Yi Y. A toxicity study of exosomes derived from mesenchymal stem cells. Cytotherapy. (2020) 22(5, Supplement):S189. doi: 10.1016/j.jcyt.2020.04.044
58. Morse MA, Garst J, Osada T, Khan S, Hobeika A, Clay TM, et al. A phase I study of dexosome immunotherapy in patients with advanced non-small cell lung cancer. J Transl Med. (2005) 3(1):9. doi: 10.1186/1479-5876-3-9
59. Janiszewski M, Do Carmo AO, Pedro MA, Silva E, Knobel E, Laurindo FRM. Platelet-derived exosomes of septic individuals possess proapoptotic NAD(P)H oxidase activity: a novel vascular redox pathway. Crit Care Med. (2004) 32(3):818–25. doi: 10.1097/01.CCM.0000114829.17746.19
60. Azevedo LCP, Janiszewski M, Pontieri V, d M, Pedro A, Bassi E, et al. Platelet-derived exosomes from septic shock patients induce myocardial dysfunction. Critical Care (London, England). (2007) 11(6):R120. doi: 10.1186/cc6176
61. Wang L, Zhao H, Xu H, Liu X, Chen X, Peng Q, et al. Targeting the TXNIP-NLRP3 interaction with PSSM1443 to suppress inflammation in sepsis-induced myocardial dysfunction. J Cell Physiol. (2021) 236(6):4625–39. doi: 10.1002/jcp.30186
62. Zhou Q, Xie M, Zhu J, Yi Q, Tan B, Li Y, et al. PINK1 contained in huMSC-derived exosomes prevents cardiomyocyte mitochondrial calcium overload in sepsis via recovery of mitochondrial Ca2+ efflux. Stem Cell Res Ther. (2021) 12(1):269. doi: 10.1186/s13287-021-02325-6
63. Valadi H, Ekström K, Bossios A, Sjöstrand M, Lee JJ, Lötvall JO. Exosome-mediated transfer of mRNAs and microRNAs is a novel mechanism of genetic exchange between cells. Nat Cell Biol. (2007) 9(6):654–9. doi: 10.1038/ncb1596
64. Rabinowits G, Gerçel-Taylor C, Day JM, Taylor DD, Kloecker GH. Exosomal microRNA: a diagnostic marker for lung cancer. Clin Lung Cancer. (2009) 10(1):42–6. doi: 10.3816/CLC.2009.n.006
65. Jansen F, Yang X, Proebsting S, Hoelscher M, Przybilla D, Baumann K, et al. MicroRNA expression in circulating microvesicles predicts cardiovascular events in patients with coronary artery disease. J Am Heart Assoc. (2014) 3(6):e001249. doi: 10.1161/JAHA.114.001249
66. Guo T, Tang X-H, Gao X-Y, Zhou Y, Jin B, Deng Z-Q, et al. A liquid biopsy signature of circulating exosome-derived mRNAs, miRNAs and lncRNAs predict therapeutic efficacy to neoadjuvant chemotherapy in patients with advanced gastric cancer. Mol Cancer. (2022) 21(1):216. doi: 10.1186/s12943-022-01684-9
67. Ma H, Wang X, Ha T, Gao M, Liu L, Wang R, et al. MicroRNA-125b prevents cardiac dysfunction in polymicrobial sepsis by targeting TRAF6-mediated nuclear factor κB activation and p53-mediated apoptotic signaling. J Infect Dis. (2016) 214(11):1773–83. doi: 10.1093/infdis/jiw449
68. Guo H, Tang L, Xu J, Lin C, Ling X, Lu C, et al. MicroRNA-495 serves as a diagnostic biomarker in patients with sepsis and regulates sepsis-induced inflammation and cardiac dysfunction. Eur J Med Res. (2019) 24(1):37. doi: 10.1186/s40001-019-0396-3
69. Halkein J, Tabruyn SP, Ricke-Hoch M, Haghikia A, Nguyen N-Q-N, Scherr M, et al. MicroRNA-146a is a therapeutic target and biomarker for peripartum cardiomyopathy. J Clin Invest. (2013) 123(5):2143–54. doi: 10.1172/JCI64365
70. Wang H, Zhang P, Chen W, Feng D, Jia Y, Xie L. Serum microRNA signatures identified by solexa sequencing predict sepsis patients’ mortality: a prospective observational study. PLoS One. (2012) 7(6):e38885. doi: 10.1371/journal.pone.0038885
71. Pang B, Zhu Y, Ni J, Thompson J, Malouf D, Bucci J, et al. Extracellular vesicles: the next generation of biomarkers for liquid biopsy-based prostate cancer diagnosis. Theranostics. (2020) 10(5):2309–26. doi: 10.7150/thno.39486
72. Wang X, Gu H, Qin D, Yang L, Huang W, Essandoh K, et al. Exosomal miR-223 contributes to mesenchymal stem cell-elicited cardioprotection in polymicrobial sepsis. Sci Rep. (2015) 5:13721. doi: 10.1038/srep13721
73. Pei Y, Xie S, Li J, Jia B. Bone marrow-mesenchymal stem cell-derived exosomal microRNA-141 targets PTEN and activates β-catenin to alleviate myocardial injury in septic mice. Immunopharmacol Immunotoxicol. (2021) 43(5):584–93. doi: 10.1080/08923973.2021.1955920
74. Liu C, Xue J, Xu B, Zhang A, Qin L, Liu J, et al. Exosomes derived from miR-146a-5p-enriched mesenchymal stem cells protect the cardiomyocytes and myocardial tissues in the polymicrobial sepsis through regulating MYBL1. Stem Cells Int. (2021) 2021:1530445. doi: 10.1155/2021/1530445
75. Zhang X, Wang X, Fan M, Tu F, Yang K, Ha T, et al. Endothelial HSPA12B exerts protection against sepsis-induced severe cardiomyopathy via suppression of adhesion molecule expression by miR-126. Front Immunol. (2020) 11:566. doi: 10.3389/fimmu.2020.00566
76. Tu F, Wang X, Zhang X, Ha T, Wang Y, Fan M, et al. Novel role of endothelial derived exosomal HSPA12B in regulating macrophage inflammatory responses in polymicrobial sepsis. Front Immunol. (2020) 11:825. doi: 10.3389/fimmu.2020.00825
77. Choi H, Kim Y, Mirzaaghasi A, Heo J, Kim YN, Shin JH, et al. Exosome-based delivery of super-repressor IκBα relieves sepsis-associated organ damage and mortality. Sci Adv. (2020) 6(15):eaaz6980. doi: 10.1126/sciadv.aaz6980
78. Feng Y, Huang W, Wani M, Yu X, Ashraf M. Ischemic preconditioning potentiates the protective effect of stem cells through secretion of exosomes by targeting Mecp2 via miR-22. PLoS One. (2014) 9(2):e88685. doi: 10.1371/journal.pone.0088685
79. Wei Z, Chen Z, Zhao Y, Fan F, Xiong W, Song S, et al. Mononuclear phagocyte system blockade using extracellular vesicles modified with CD47 on membrane surface for myocardial infarction reperfusion injury treatment. Biomaterials. (2021) 275:121000. doi: 10.1016/j.biomaterials.2021.121000
80. Song Y, Zhang C, Zhang J, Jiao Z, Dong N, Wang G, et al. Localized injection of miRNA-21-enriched extracellular vesicles effectively restores cardiac function after myocardial infarction. Theranostics. (2019) 9(8):2346–60. doi: 10.7150/thno.29945
81. de Couto G, Gallet R, Cambier L, Jaghatspanyan E, Makkar N, Dawkins JF, et al. Exosomal MicroRNA transfer into macrophages mediates cellular postconditioning. Circulation. (2017) 136(2):200–14. doi: 10.1161/CIRCULATIONAHA.116.024590
82. Kanki S, Jaalouk DE, Lee S, Yu AYC, Gannon J, Lee RT. Identification of targeting peptides for ischemic myocardium by in vivo phage display. J Mol Cell Cardiol. (2011) 50(5):841–8. doi: 10.1016/j.yjmcc.2011.02.003
83. Tian Y, Li S, Song J, Ji T, Zhu M, Anderson GJ, et al. A doxorubicin delivery platform using engineered natural membrane vesicle exosomes for targeted tumor therapy. Biomaterials. (2014) 35(7):2383–90. doi: 10.1016/j.biomaterials.2013.11.083
84. Vandergriff A, Huang K, Shen D, Hu S, Hensley MT, Caranasos TG, et al. Targeting regenerative exosomes to myocardial infarction using cardiac homing peptide. Theranostics. (2018) 8(7):1869–78. doi: 10.7150/thno.20524
85. Wang X, Chen Y, Zhao Z, Meng Q, Yu Y, Sun J, et al. Engineered exosomes with ischemic myocardium-targeting peptide for targeted therapy in myocardial infarction. J Am Heart Assoc. (2018b) 7(15):e008737. doi: 10.1161/JAHA.118.008737
86. Gupta S, Knowlton AA. HSP60 Trafficking in adult cardiac myocytes: role of the exosomal pathway. Am J Physiol Heart Circ Physiol. (2007) 292(6):H3052–6. doi: 10.1152/ajpheart.01355.2006
87. Ibrahim AG-E, Cheng K, Marbán E. Exosomes as critical agents of cardiac regeneration triggered by cell therapy. Stem Cell Rep. (2014) 2(5):606–19. doi: 10.1016/j.stemcr.2014.04.006
88. Gallet R, Dawkins J, Valle J, Simsolo E, de Couto G, Middleton R, et al. Exosomes secreted by cardiosphere-derived cells reduce scarring, attenuate adverse remodelling, and improve function in acute and chronic porcine myocardial infarction. Eur Heart J. (2017) 38(3):201–11. doi: 10.1093/eurheartj/ehw240
89. Zhu L-P, Tian T, Wang J-Y, He J-N, Chen T, Pan M, et al. Hypoxia-elicited mesenchymal stem cell-derived exosomes facilitates cardiac repair through miR-125b-mediated prevention of cell death in myocardial infarction. Theranostics. (2018) 8(22):6163–77. doi: 10.7150/thno.28021
90. Mentkowski KI, Lang JK. Exosomes engineered to express a cardiomyocyte binding peptide demonstrate improved cardiac retention in vivo. Sci Rep. (2019) 9(1):10041. doi: 10.1038/s41598-019-46407-1
91. Smothers JF, Henikoff S, Carter P. Tech.sight. Phage display. Affinity selection from biological libraries. Science (New York, N.Y.). (2002) 298(5593):621–2. doi: 10.1126/science.298.5593.621
92. McGuire MJ, Samli KN, Johnston SA, Brown KC. In vitro selection of a peptide with high selectivity for cardiomyocytes in vivo. J Mol Biol. (2004) 342(1):171–82. doi: 10.1016/j.jmb.2004.06.029
93. Zahid M, Phillips BE, Albers SM, Giannoukakis N, Watkins SC, Robbins PD. Identification of a cardiac specific protein transduction domain by in vivo biopanning using a M13 phage peptide display library in mice. PLoS One. (2010) 5(8):e12252. doi: 10.1371/journal.pone.0012252
94. Kang J-Y, Kim H, Mun D, Yun N, Joung B. Co-delivery of curcumin and miRNA-144-3p using heart-targeted extracellular vesicles enhances the therapeutic efficacy for myocardial infarction. J Control Release. (2021) 331:62–73. doi: 10.1016/j.jconrel.2021.01.018
95. Streng AS, Jacobs LHJ, Schwenk RW, Cardinaels EPM, Meex SJR, Glatz JFC, et al. Cardiac troponin in ischemic cardiomyocytes: intracellular decrease before onset of cell death. Exp Mol Pathol. (2014) 96(3):339–45. doi: 10.1016/j.yexmp.2014.02.012
96. Yang Y, Shi C, Hou X, Zhao Y, Chen B, Tan B, et al. Modified VEGF targets the ischemic myocardium and promotes functional recovery after myocardial infarction. J Control Release. (2015) 213:27–35. doi: 10.1016/j.jconrel.2015.06.036
97. Antes TJ, Middleton RC, Luther KM, Ijichi T, Peck KA, Liu WJ, et al. Targeting extracellular vesicles to injured tissue using membrane cloaking and surface display. J Nanobiotechnol. (2018) 16(1):61. doi: 10.1186/s12951-018-0388-4
98. Wang H, Maimaitiaili R, Yao J, Xie Y, Qiang S, Hu F, et al. Percutaneous intracoronary delivery of plasma extracellular vesicles protects the myocardium against ischemia-reperfusion injury in Canis. Hypertension (Dallas, Tex.: 1979). (2021) 78(5):1541–54. doi: 10.1161/HYPERTENSIONAHA.121.17574
99. Gupta D, Wiklander OPB, Görgens A, Conceição M, Corso G, Liang X, et al. Amelioration of systemic inflammation via the display of two different decoy protein receptors on extracellular vesicles. Nat Biomed Eng. (2021) 5(9):1084–98. doi: 10.1038/s41551-021-00792-z
100. Kamerkar S, LeBleu VS, Sugimoto H, Yang S, Ruivo CF, Melo SA, et al. Exosomes facilitate therapeutic targeting of oncogenic KRAS in pancreatic cancer. Nature. (2017) 546(7659):498–503. doi: 10.1038/nature22341
101. Matlung HL, Szilagyi K, Barclay NA, van den Berg TK. The CD47-SIRPα signaling axis as an innate immune checkpoint in cancer. Immunol Rev. (2017) 276(1):145–64. doi: 10.1111/imr.12527
102. Rana S, Yue S, Stadel D, Zöller M. Toward tailored exosomes: the exosomal tetraspanin web contributes to target cell selection. Int J Biochem Cell Biol. (2012) 44(9):1574–84. doi: 10.1016/j.biocel.2012.06.018
103. Ma C, Liu K, Wang F, Fei X, Niu C, Li T, et al. Neutrophil membrane engineered Panax ginseng root derived exosomes loaded miRNA 182-5p targets NOX4/drp-1/NLRP3 signal pathway to alleviate acute lung injury in sepsis: experimental studies. Int J Surg. (2023) 110(1):72–86. doi: 10.1097/JS9.0000000000000789
104. Wu R, Hu X, Wang JA. Current optimized strategies for stem cell-derived extracellular vesicle/exosomes in cardiac repair. J Mol Cell Cardiol. (2023) 184:13–25. doi: 10.1016/j.yjmcc.2023.09.006
105. Hu S, Wang X, Li Z, Zhu D, Cores J, Wang Z, et al. Platelet membrane and stem cell exosome hybrid enhances cellular uptake and targeting to heart injury. Nano Today. (2021) 39:101210. doi: 10.1016/j.nantod.2021.101210
106. Li Q, Huang Z, Wang Q, Gao J, Chen J, Tan H, et al. Targeted immunomodulation therapy for cardiac repair by platelet membrane engineering extracellular vesicles via hitching peripheral monocytes. Biomaterials. (2022) 284:121529. doi: 10.1016/j.biomaterials.2022.121529
107. Zhang N, Song Y, Huang Z, Chen J, Tan H, Yang H, et al. Monocyte mimics improve mesenchymal stem cell-derived extracellular vesicle homing in a mouse MI/RI model. Biomaterials. (2020) 255:120168. doi: 10.1016/j.biomaterials.2020.120168
108. Shao Q, Ding T, Pan F, Li G, Shen S, Qian J, et al. Protein corona mediated liposomal drug delivery for bacterial infection management. Asian J Pharm Sci. (2022) 17(6):855–66. doi: 10.1016/j.ajps.2022.10.003
109. Zheng L-J, Hu B, Zhao D, Liu W, Liu Q, Huang Y, et al. Recent progresses of exosome–liposome fusions in drug delivery. Chin Chem Lett. (2023) 35(2):108647. doi: 10.1016/j.cclet.2023.108647
110. Kotterman MA, Chalberg TW, Schaffer DV. Viral vectors for gene therapy: translational and clinical outlook. Annu Rev Biomed Eng. (2015) 17:63–89. doi: 10.1146/annurev-bioeng-071813-104938
111. Parodi A, Molinaro R, Sushnitha M, Evangelopoulos M, Martinez JO, Arrighetti N, et al. Bio-inspired engineering of cell- and virus-like nanoparticles for drug delivery. Biomaterials. (2017) 147:155–68. doi: 10.1016/j.biomaterials.2017.09.020
112. Yim N, Choi C. Extracellular vesicles as novel carriers for therapeutic molecules. BMB Rep. (2016) 49(11):585–6. doi: 10.5483/BMBRep.2016.49.11.174
113. Almeida B, Nag OK, Rogers KE, Delehanty JB. Recent progress in bioconjugation strategies for liposome-mediated drug delivery. Molecules (Basel, Switzerland). (2020) 25(23):5672. doi: 10.3390/molecules25235672
114. Kim MS, Haney MJ, Zhao Y, Mahajan V, Deygen I, Klyachko NL, et al. Development of exosome-encapsulated paclitaxel to overcome MDR in cancer cells. Nanomed Nanotechnol Biol Med. (2016) 12(3):655–64. doi: 10.1016/j.nano.2015.10.012
115. Luan X, Sansanaphongpricha K, Myers I, Chen H, Yuan H, Sun D. Engineering exosomes as refined biological nanoplatforms for drug delivery. Acta Pharmacol Sin. (2017) 38(6):754–63. doi: 10.1038/aps.2017.12
116. Batrakova EV, Kim MS. Using exosomes, naturally-equipped nanocarriers, for drug delivery. J Control Release. (2015) 219:396–405. doi: 10.1016/j.jconrel.2015.07.030
117. Bunggulawa EJ, Wang W, Yin T, Wang N, Durkan C, Wang Y, et al. Recent advancements in the use of exosomes as drug delivery systems. J Nanobiotechnol. (2018) 16(1):81. doi: 10.1186/s12951-018-0403-9
118. Liang Y, Duan L, Lu J, Xia J. Engineering exosomes for targeted drug delivery. Theranostics. (2021) 11(7):3183–95. doi: 10.7150/thno.52570
119. Meng W, Wang L, Du X, Xie M, Yang F, Li F, et al. Engineered mesenchymal stem cell-derived extracellular vesicles constitute a versatile platform for targeted drug delivery. J Control Release. (2023) 363:235–52. doi: 10.1016/j.jconrel.2023.09.037
120. Collino F, Bruno S, Incarnato D, Dettori D, Neri F, Provero P, et al. AKI recovery induced by mesenchymal stromal cell-derived extracellular vesicles carrying MicroRNAs. J Am Soc Nephrol. (2015) 26(10):2349–60. doi: 10.1681/ASN.2014070710
121. Sayed D, Abdellatif M. MicroRNAs in development and disease. Physiol Rev. (2011) 91(3):827–87. doi: 10.1152/physrev.00006.2010
122. Zhu H, Fan G-C. Role of microRNAs in the reperfused myocardium towards post-infarct remodelling. Cardiovasc Res. (2012) 94(2):284–92. doi: 10.1093/cvr/cvr291
123. Benz F, Roy S, Trautwein C, Roderburg C, Luedde T. Circulating MicroRNAs as biomarkers for sepsis. Int J Mol Sci. (2016) 17(1):78. doi: 10.3390/ijms17010078
124. Real JM, Ferreira LRP, Esteves GH, Koyama FC, Dias MVS, Bezerra-Neto JE, et al. Exosomes from patients with septic shock convey miRNAs related to inflammation and cell cycle regulation: new signaling pathways in sepsis? Critical Care (London, England). (2018) 22(1):68. doi: 10.1186/s13054-018-2003-3
125. Jia P, Wu X, Dai Y, Teng J, Fang Y, Hu J, et al. MicroRNA-21 is required for local and remote ischemic preconditioning in multiple organ protection against sepsis. Crit Care Med. (2017) 45(7):e703–10. doi: 10.1097/CCM.0000000000002363
126. Ling L, Zhi L, Wang H, Deng Y, Gu C. MicroRNA-181b inhibits inflammatory response and reduces myocardial injury in sepsis by downregulating HMGB1. Inflammation. (2021) 44(4):1263–73. doi: 10.1007/s10753-020-01411-w
127. Davidson SM, Yellon DM. Exosomes and cardioprotection—a critical analysis. Mol Asp Med. (2018) 60:104–14. doi: 10.1016/j.mam.2017.11.004
128. Liu M, Hu S, Yan N, Popowski KD, Cheng K. Inhalable extracellular vesicle delivery of IL-12 mRNA to treat lung cancer and promote systemic immunity. Nat Nanotechnol. (2024) 19(4):565–75. doi: 10.1038/s41565-023-01580-3
129. Kojima R, Bojar D, Rizzi G, Hamri GC-E, El-Baba MD, Saxena P, et al. Designer exosomes produced by implanted cells intracerebrally deliver therapeutic cargo for Parkinson’s disease treatment. Nat Commun. (2018) 9(1):1305. doi: 10.1038/s41467-018-03733-8
130. Wang J-H, Forterre AV, Zhao J, Frimannsson DO, Delcayre A, Antes TJ, et al. Anti-HER2 scFv-directed extracellular vesicle-mediated mRNA-based gene delivery inhibits growth of HER2-positive human breast tumor Xenografts by prodrug activation. Mol Cancer Ther. (2018) 17(5):1133–42. doi: 10.1158/1535-7163.MCT-17-0827
131. Usman WM, Pham TC, Kwok YY, Vu LT, Ma V, Peng B, et al. Efficient RNA drug delivery using red blood cell extracellular vesicles. Nat Commun. (2018) 9(1):2359. doi: 10.1038/s41467-018-04791-8
132. Zheng X, Xing Y, Sun K, Jin H, Zhao W, Yu F. Combination therapy with resveratrol and celastrol using folic acid-functionalized exosomes enhances the therapeutic efficacy of sepsis. Adv Healthcare Mater. (2023) 12(29):e2301325. doi: 10.1002/adhm.202301325
133. Zhou B, Zhang J, Chen Y, Liu Y, Tang X, Xia P, et al. Puerarin protects against sepsis-induced myocardial injury through AMPK-mediated ferroptosis signaling. Aging. (2022) 14(8):3617–32. doi: 10.18632/aging.204033
134. Dai S, Ye B, Chen L, Hong G, Zhao G, Lu Z. Emodin alleviates LPS-induced myocardial injury through inhibition of NLRP3 inflammasome activation. Phytother Res. (2021) 35(9):5203–13. doi: 10.1002/ptr.7191
135. Qiao Y, Wang L, Hu T, Yin D, He H, He M. Capsaicin protects cardiomyocytes against lipopolysaccharide-induced damage via 14-3-3γ-mediated autophagy augmentation. Front Pharmacol. (2021) 12:659015. doi: 10.3389/fphar.2021.659015
136. Yi X, Wei X, Lv H, An Y, Li L, Lu P, et al. Exosomes derived from microRNA-30b-3p-overexpressing mesenchymal stem cells protect against lipopolysaccharide-induced acute lung injury by inhibiting SAA3. Exp Cell Res. (2019) 383(2):111454. doi: 10.1016/j.yexcr.2019.05.035
137. Deng Z, Rong Y, Teng Y, Mu J, Zhuang X, Tseng M, et al. Broccoli-Derived nanoparticle inhibits mouse colitis by activating dendritic cell AMP-activated protein kinase. Mol Ther. (2017) 25(7):1641–54. doi: 10.1016/j.ymthe.2017.01.025
138. Teng Y, Ren Y, Sayed M, Hu X, Lei C, Kumar A, et al. Plant-derived exosomal MicroRNAs shape the gut Microbiota. Cell Host Microbe. (2018) 24(5):637–652.e8. doi: 10.1016/j.chom.2018.10.001
139. Niu W, Xiao Q, Wang X, Zhu J, Li J, Liang X, et al. A biomimetic drug delivery system by integrating grapefruit extracellular vesicles and doxorubicin-loaded heparin-based nanoparticles for glioma therapy. Nano Lett. (2021) 21(3):1484–92. doi: 10.1021/acs.nanolett.0c04753
140. Wang Q, Zhuang X, Mu J, Deng Z-B, Jiang H, Zhang L, et al. Delivery of therapeutic agents by nanoparticles made of grapefruit-derived lipids. Nat Commun. (2013) 4:1867. doi: 10.1038/ncomms2886
141. Xu X-H, Yuan T-J, Dad HA, Shi M-Y, Huang Y-Y, Jiang Z-H, et al. Plant exosomes as novel nanoplatforms for MicroRNA transfer stimulate neural differentiation of stem cells in vitro and in vivo. Nano Lett. (2021) 21(19):8151–9. doi: 10.1021/acs.nanolett.1c02530
142. Ju S, Mu J, Dokland T, Zhuang X, Wang Q, Jiang H, et al. Grape exosome-like nanoparticles induce intestinal stem cells and protect mice from DSS-induced colitis. Mol Ther. (2013) 21(7):1345–57. doi: 10.1038/mt.2013.64
143. Yuan Y, Du W, Liu J, Ma W, Zhang L, Du Z, et al. Stem cell-derived exosome in cardiovascular diseases: macro roles of micro particles. Front Pharmacol. (2018) 9:547. doi: 10.3389/fphar.2018.00547
144. Dawkins JF, Ehdaie A, Rogers R, Soetkamp D, Valle J, Holm K, et al. Biological substrate modification suppresses ventricular arrhythmias in a porcine model of chronic ischaemic cardiomyopathy. Eur Heart J. (2022) 43(22):2139–56. doi: 10.1093/eurheartj/ehac042
145. Li Y, Zhang H, Chen C, Qiao K, Li Z, Han J, et al. Biomimetic immunosuppressive exosomes that inhibit cytokine storms contribute to the alleviation of sepsis. Adv Mater (Deerfield Beach, Fla.). (2022) 34(19):e2108476. doi: 10.1002/adma.202108476
146. Xie Q, Hao Y, Li N, Song H, Chen X, Zhou Z, et al. Cellular uptake of engineered extracellular vesicles: biomechanisms, engineered strategies, and disease treatment. Adv Healthcare Mater. (2024) 13(2):e2302280. doi: 10.1002/adhm.202302280
147. Reiner AT, Witwer KW, van Balkom BWM, de Beer J, Brodie C, Corteling RL, et al. Concise review: developing best-practice models for the therapeutic use of extracellular vesicles. Stem Cells Transl Med. (2017) 6(8):1730–9. doi: 10.1002/sctm.17-0055
Keywords: sepsis, septic cardiomyopathy, engineered exosomes, extracellular vesicles, drug delivery
Citation: Mao L, Liu S, Chen Y, Huang H, Ding F and Deng L (2024) Engineered exosomes: a potential therapeutic strategy for septic cardiomyopathy. Front. Cardiovasc. Med. 11:1399738. doi: 10.3389/fcvm.2024.1399738
Received: 13 March 2024; Accepted: 14 June 2024;
Published: 28 June 2024.
Edited by:
Christoph Thiemermann, Queen Mary University of London, United KingdomReviewed by:
Gentaro Ikeda, Stanford University, United StatesLucy V. Norling, Queen Mary University of London, United Kingdom
Basilia Zingarelli, Cincinnati Children’s Hospital Medical Center, United States
© 2024 Mao, Liu, Chen, Huang, Ding and Deng. This is an open-access article distributed under the terms of the Creative Commons Attribution License (CC BY). The use, distribution or reproduction in other forums is permitted, provided the original author(s) and the copyright owner(s) are credited and that the original publication in this journal is cited, in accordance with accepted academic practice. No use, distribution or reproduction is permitted which does not comply with these terms.
*Correspondence: Liehua Deng, Z2xpbnNvbkAxMjYuY29t