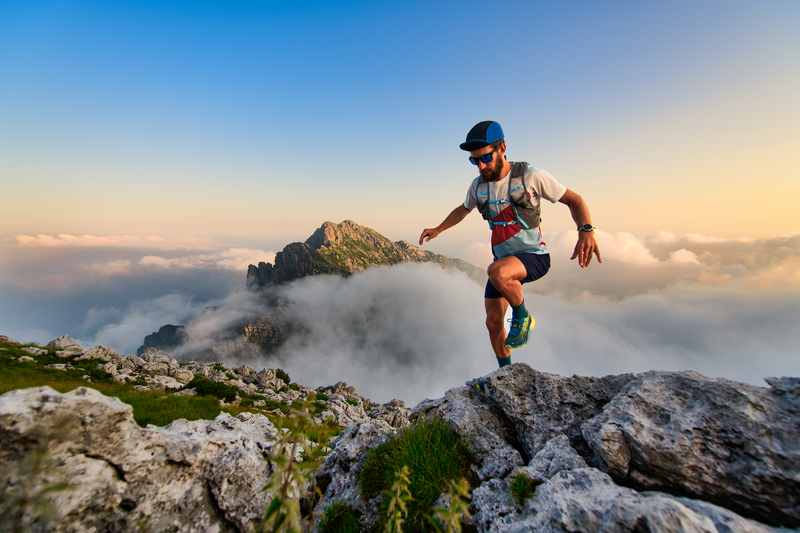
95% of researchers rate our articles as excellent or good
Learn more about the work of our research integrity team to safeguard the quality of each article we publish.
Find out more
MINI REVIEW article
Front. Cardiovasc. Med. , 27 March 2024
Sec. Cardioneurology
Volume 11 - 2024 | https://doi.org/10.3389/fcvm.2024.1384637
This article is part of the Research Topic The Non-Neuronal Cholinergic System in the Cardiovascular System: Its Influence on the Heart, Vasculature, and the Central Nervous System View all 5 articles
The non-neuronal cholinergic system of the cardiovascular system has recently gained attention because of its origin. The final product of this system is acetylcholine (ACh) not derived from the parasympathetic nervous system but from cardiomyocytes, endothelial cells, and immune cells. Accordingly, it is defined as an ACh synthesis system by non-neuronal cells. This system plays a dispensable role in the heart and cardiomyocytes, which is confirmed by pharmacological and genetic studies using murine models, such as models with the deletion of vesicular ACh transporter gene and modulation of the choline acetyltransferase (ChAT) gene. In these models, this system sustained the physiological function of the heart, prevented the development of cardiac hypertrophy, and negatively regulated the cardiac metabolism and reactive oxygen species production, resulting in sustained cardiac homeostasis. Further, it regulated extra-cardiac organs, as revealed by heart-specific ChAT transgenic (hChAT tg) mice. They showed enhanced functions of the blood-brain barrier (BBB), indicating that the augmented system influences the BBB through the vagus nerve. Therefore, the non-neuronal cardiac cholinergic system indirectly influences brain function. This mini-review summarizes the critical cardiac phenotypes of hChAT tg mice and focuses on the effect of the system on BBB functions. We discuss the possibility that a cholinergic signal or vagus nerve influences the expression of BBB component proteins to consolidate the barrier, leading to the downregulation of inflammatory responses in the brain, and the modulation of cardiac dysfunction-related effects on the brain. This also discusses the possible interventions using the non-neuronal cardiac cholinergic system.
The final product of the non-neuronal cholinergic system of the cardiovascular system is acetylcholine (ACh), which is not derived from the parasympathetic nervous system but from cardiomyocytes and endothelial cells. This system plays a protective and physiologically dispensable role specifically in the heart and cardiomyocytes and the system in the heart is named as the non-neuronal cardiac cholinergic system (NNCCS). This system sustains the physiological function of the heart and prevents the development of cardiac dysfunction. Moreover, the system regulates brain functions via modulating the blood-brain barrier (BBB). Therefore, NNCCS influences the heart and brain because each organ is innervated by the vagus nerve. Although the NNCCS is equipped by the heart, the physiological functions are reported to be more crucial than expected by many studies.
A pioneering study revealed that cardiomyocytes or myocardium derived from 1-day-old Xenopus embryos release acetylcholine (ACh), which is upregulated by acetylcholinesterase inhibitor, and the cells express the immunoreactivity of choline acetyltransferase (ChAT) (1). Another study demonstrated that ChAT activity is highest in the sinoatrial node, followed by the atrioventricular node and conduction bundles. However, the activity was one-third of the conduction system in the right and left ventricles of the guinea pig heart (2). Moreover, a transplanted rat heart without nerve innervation maintained an ACh level in each cardiac ventricle and atrium comparable to that of a control heart (3). Therefore, these previous studies strongly suggest that cardiomyocytes are capable of synthesizing ACh, which differs from the ACh released from the vagus nerve ends.
Since the immunohistochemical study by Fu et al. in 1998 and the study by Oda et al. in 1987 measuring regional ACh contents in the heart, the evidence of the presence of ACh in cardiomyocytes has accumulated. However, the significance and biological roles of cardiomyocyte-derived ACh remained unclear until 2009, when it was demonstrated that cardiomyocyte-derived ACh plays an important role in negatively regulating the overshoot of oxygen consumption, probably through mitochondria (4). This study revealed the biological significance of the system by establishing the link between the cardiomyocytes-derived ACh synthesis system and their energy metabolism. Simultaneously, another study by Rana et al. revealed that ACh is synthesized in the heart, both in the atrium and ventricle, and that ACh levels decrease in an age-dependent manner (5), indicating that this system is influenced by aging.
Various studies have established that cardiomyocytes possess the machinery to synthesize ACh through a system different from the parasympathetic nervous system (PNS), such as the vagus nerve. Therefore, ACh is derived from the PNS and ACh synthesis system in the heart. The latter system has been referred to as the non-neuronal ACh in the heart, also referred to as the non-neuronal cholinergic system in the heart, the cardiac non-neuronal cholinergic system, or non-neuronal cardiac cholinergic system (NNCCS). In our review, NNCCS is mainly used.
Prado et al. investigated the significance of this system using the acetylcholinesterase inhibitor pyridostigmine in rat cardiomyocytes. Their results indicated the treated cardiomyocytes increased nitric oxide (NO) production and suppressed isoproterenol-induced hypertrophy as well as the alteration of calcium responses induced by adrenergic overstimulation (6). Further, cardiomyocytes from knockout mice lacking the vesicular ACh transporter (VAChT) gene (VAChT KO mice) had disturbed cardiac activity, including exaggerated exercise-induced elevation of heart rate, poor recovery of heart rate after exercise, increased exposure to mitochondrial reactive oxygen species (ROS) stress, and increased peak calcium transients (7). Notably, these dysregulated cardiac functions contributed to cardiac hypertrophy and disturbed responses to a β-stimulant, isoproterenol (7). These characteristics of VAChT KO mice revealed that cardiomyocyte-secreted ACh is indispensable for sustaining the physiological homeostasis of the heart.
In 2013, two different types of mice−hChAT tg (8) and VAChT KO (7)—were studied, and their effects on the heart were compared. hChAT tg mice overexpressing the ChAT gene showed enhanced NNCCS function because of extremely high cardiac ACh levels (8). Cardiomyocytes from hChAT tg mice were less dependent on oxygen, and the hearts from hChAT tg mice were able to utilize glucose more efficiently as a fuel source than wild-type (WT) mice, as more GLUT4 was expressed in the hearts of hChAT tg mice. Therefore, hChAT tg hearts were more resilient to hypoxia, ischemia, or myocardial infarction (MI) due to enhanced protein expression levels of hypoxia-inducible factor (HIF)-1α, even under normoxic conditions. The MI-subjected hearts of ChAT tg mice contained more new capillaries and intact cardiomyocytes. Despite comparable hemodynamic and cardiac parameters, the ChAT tg mouse heart, which was connected to a Langendorf apparatus, continued to beat for a more prolonged period, even after the perfusion had stopped. Further, the heartbeat started more rapidly than in the WT mouse heart after reperfusion. Consequently, we attempted to explore and consider the critical biological role of the NNCCS. For example, the NNCCS regulates cardiac energy metabolism, and the augmentation of this system increases glucose uptake as a fuel substrate and enhances angiogenesis, resulting in enhanced resistance to hypoxia and ischemia in the heart. The same research group developed a different murine NNCCS dysfunction model (9) from VAChT KO and heart-specific ChAT KO mice (7). Heart-specific ChAT knockdown (hChAT KD) mice, developed by simultaneously overexpressing three ChAT mRNA-specific microRNAs in the heart, showed typical cardiac dysfunction with enhanced expression of heart failure markers, dysregulated expression of glucose metabolism-related molecules, increased ROS exposure in the heart, impaired cardiac NO production, poor angiogenesis, blunted vagus nerve activity, and disturbed association between NO synthase 1 and SERCA2 protein. Therefore, hChAT KD mice shared the phenotypes induced by impaired NNCCS functions with VAChT KO mice.
In addition, Roy et al. extensively examined the influence on cardiac function in angiotensin II-treated VAChT KO mice and heart-specific ChAT KO mice using the Cre-loxP system (10) and revealed that either KO mice comparably showed impaired cardiac functions, especially when challenged by hemodynamic stress. However, the precise underlying mechanisms and targets by which cardiac function is impaired remain unclear.
The following section summarizes the results of various previous studies that focused on the physiological functions of the NNCCS.
First, the NNCCS protects the heart from various stressors such as hypoxia, ischemia, ROS, and inotropic agents (6–8, 10, 11). Notably, the induction of HIF-1α protein through the non-hypoxic pathway is responsible for this resilience (12). HIF-1α, a crucial transcription factor for an anti-hypoxic or anti-ischemic response, shifts cellular energy metabolism predominantly to glucose metabolism.
Second, the NNCCS plays an inhibitory role in conditions with the enhanced sympathetic nervous system where cardiac function is activated for compensation. The NNCCS also attenuates cardiac hypertrophy or cardiac remodeling and efficiently decreases heart rate in response to stimuli (6–8, 10, 11).
Third, the NNCCS positively regulates cell−cell interactions in the heart by sustaining gap junction function by stabilizing connexin and β-catenin localization in cardiomyocytes. Downregulation of the NNCCS causes mislocalization of connexin and β-catenin from the plasma membrane of cardiomyocytes, leading to decreased immunoreactivity (11). The localization of gap junction proteins at the proper site in a cardiomyocyte indicates that the NNCCS plays a role in sustaining electrophysiological properties in the heart and inhibits the genesis of an arrhythmogenic reentry circuit.
Fourth, the NNCCS regulates angiogenesis in the heart, which is closely related to heart protection. Without the NNCCS, angiogenesis impairment may blunt compensatory adaptation of the heart and aggravate cardiac functions, especially in a post-myocardial infarction state (8).
These four characteristics are novel physiological findings related to the role of NNCCS in the heart. Among the previous studies dealing with cardiogenic factors synthesized by cardiomyocytes, ACh, to the best of my knowledge, may be one of the factors exerting pleiotropic effects on the heart, independent of its already known negative inotropy and chronotropic effects.
Additionally, based on previous studies using hChAT tg mice (13, 14), the NNCCS plays a significant role in regulating higher brain functions, including mood or stress (13), and the blood-brain barrier (BBB) (14). hChAT tg mice showed anti-stress and anti-depressive phenotypes because the immobility duration was more shortened in the tail suspension and forced swimming tests than in WT mice. Further, the peak corticosterone levels in hChAT tg mice during restraint stress were also lower than those in WT mice, and hChAT tg mice stayed for a longer time in the open space of the elevated plus maze. In addition, they were more resistant to convulsant inducers and less susceptible to status epileptics that causes death. Furthermore, these specific central nervous system (CNS) phenotypes were dependent on the vagus nerve because the vagus nerve activity of hChAT tg mice was significantly enhanced, and the phenotypes almost overlapped with those of vagus nerve-stimulated WT mice. However, left vagotomy or treatment with L-NAME, an NOS inhibitor, clearly reversed almost all specific phenotypes of hChAT tg mice (13). Therefore, CNS functions in hChAT tg mice may be modulated by enhancing vagus nerve activity and increasing cardiac NO production. Alternatively, hChAT tg mice exhibited enhanced vagus nerve activity like a mouse subjected to the intrinsic vagus nerve stimulation (VNS).
As one of the clues as to the CNS phenotypes of ChAT tg mice, claudin-5, a BBB protein, was significantly expressed in their brains compared with WT mice, and the function of BBB was more consolidated under both physiological and pathological conditions (14). Under physiological conditions, without any manipulation of ChAT tg mice, basal claudin-5 protein expression levels were significantly elevated in the whole brain, whose immunoreactive signals were evident in the hippocampus. Therefore, the ChAT tg mouse brain significantly attenuated Evans blue leakage in a cold injury model, which is a well-known method to evaluate BBB integrity. Furthermore, in the 1-methyl-4-phenyl-1, 2, 3, 6-tetrahydropyridine (MPTP)-induced Parkinson's disease murine model, the disruption of BBB was significantly attenuated in hChAT tg mice, and MPTP-induced neuronal loss in the substantia nigra was significantly suppressed. This indicates that consolidated BBB function via claudin-5 is a key molecular phenotype in augmented NNCCS. However, the link between the claudin-5 protein and the NNCCS or precise mechanism by which VNS regulates BBB function remains to be fully elucidated.
VNS exerts beneficial effects on remote organs innervated by the vagus nerves. Notably, VNS exerts anti-inflammatory (suppression of cytokine levels and inflammasome formation) and anti-apoptotic effects. An α7 nicotinic receptor stimulated by ACh is responsible for anti-inflammation. Here, we would like to discuss results from several animal studies using VNS and speculate the underlying mechanisms for VNS effects on BBB from several VNS aspects.
First, VNS negatively regulates the progression of inflammation in the brain. This cholinergic anti-inflammatory pathway, represented by ACh released from VNS, was initially reported by Borovikova et al. in an LPS-induced endotoxemia model (15). Thereafter, in brain injury models, VNS has been reported to exert anti-inflammatory responses, including a reduction of local responses or systemic levels of pro-inflammatory cytokines. Conversely, VNS also enhanced anti-inflammatory cytokines, including IL-10 (16–18).
Second, VNS upregulates the norepinephrine system in the brain. Upregulation of the norepinephrine levels in the brain leads to functional improvement in animals post-brain injury. In contrast, blockade of the norepinephrine pathway, including lesions of the locus coeruleus (LC), depletes norepinephrine levels in the brain, leading to poor brain functional recovery post-injury (19–22).
Third, VNS decreases brain edema, probably through the modulation of aquaporin (AQP)-4 (23). In a rat model of unilateral fluid percussion brain injury, VNS improved brain injury-induced locomotor dysfunction, probably by reducing the injury-related brain edema that appeared following injury (24). Brain injury-induced brain edema is a post-injury event that increases intracerebral pressure, compromising brain perfusion. Therefore, the suppressive effects of VNS on brain edema are promising. Edema occurs because of increased interstitial fluid storage in the brain vasculature through AQP-4, an intracellular water transport channel. As reported by Lopez, the down-regulation of AQP-4 immunoreactivity in the perivascular regions of the injured brain is one of the underlying mechanisms for VNS-induced attenuation of brain edema (23).
Fourth, several animal and preclinical studies have reported that VNS influences BBB function to prevent the disruption of BBB in a pathological model (25–27). This effect of VNS is closely linked to the reduction of edema, as the reduced BBB function directly causes brain edema. Moreover, VNS-induced attenuation of proinflammatory cytokines also conserves BBB, as these cytokines produced in the brain inhibit expression of tight junction components, including claudin-5 and occludin. Therefore, BBB function is protected by VNS. Notably, VNS also attenuated intestinal permeability regulated by tight junctions in the epithelia in a brain injury model as well as in other inflammatory models (28, 29). The ends of the vagus nerve are not only distributed in the gastrointestinal system as a peripheral organ but also to the brain as a central organ, both of which are influenced by VNS as the vagus nerve includes afferent and efferent fibers. Therefore, the effects of VNS on tight junctions in the BBB or intestine may be mediated by similar mechanisms.
Moreover, an α7 nicotinic receptor agonist, PNU-282987, strengthened the evidence that the VNS modality on BBB function is mediated by α7 nicotinic receptor-mediated signaling (30). Further, PNU-120596, a selective type-II positive allosteric modulator (α7-PAM) of α7 nicotinic receptor, protected the rat brain from traumatic brain injury (31). Moreover, Kimura et al. using rat brain endothelial cells reported that an α7 nicotinic receptor agonist, PHA543613, upregulated the protein expression of claudin-5 and occludin, strengthening BBB function (32). However, several other studies have reported the opposite conclusion regarding an α7 nicotinic receptor; therefore, it remains controversial (33). The Figure shows crucial aspects of the NNCCS in terms of its action mechanisms and relationship between the NNCCS and BBB Figure 1.
Figure 1. Activation of NNCCS can regulate functions of the heart and brain because the ascending pathway of the vagus nerve transduces the enhanced signal from the NNCCS to the brain.
These aforementioned studies strongly suggest that the VNS, or vagus nerve, regulates BBB function. However, the mechanisms by which VNS transduces its signals directly or indirectly to the BBB remain unresolved. First, the anatomical pathway, through which the nerve ends projecting from the main nucleus organizing visceral information connect to the brain vasculature, remains under-studied. Second, which receptor−muscarinic or adrenergic−on brain endothelial cells are directly stimulated, remains unknown. Further, mechanisms that indirectly influence the BBB via VNS also remain unexplored.
The predominant afferent pathway of the vagus nerve transduces signals from visceral organs to the solitary tract nucleus (NTS). The information received by NTS is partly transferred into the LC (34, 35), from which the information is propagated through the noradrenergic pathway into the whole brain. Moreover, information from the NTS is projected to other regions, including the hypothalamus, especially the paraventricular hypothalamic nucleus (PVN) (36, 37), the dorsal nucleus of the vagus nerve (38), and the forebrain (39) or telencephalon (40). Although projection pathways from the NTS or LC have been examined using antero- or retro-grade tracers, the precise anatomical or histological evidence remains lacking. Nevertheless, brain vasculature or capillaries possessing BBB properties are influenced or modulated by noradrenergic or cholinergic neurons, as the nerve end of each nervous system may be distributed near the vasculature to regulate vascular tone through each receptor (41). The next sections discuss neurotransmitter-mediated effects on BBB function.
The stimulation of the LC with carbachol, a muscarinic receptor agonist, reduced hemispheric cerebral blood flow and increased vascular permeability in the brain (41). Moreover, another study reported that α-adrenergic receptors stimulated brain permeability, attenuating BBB function, whereas β-adrenergic receptors strengthened BBB function (42). Several other studies have also supported these findings using noradrenaline or its antagonists (43–45). Notably, noradrenergic neurons modulate BBB functions, depending on α- or β-receptors expressed on brain capillaries. Further, an in vitro study using human pulmonary endothelial cells reported that β1- and β2- adrenergic receptors are involved in the stabilization of endothelial barrier permeability and influence BBB integrity (46).
The transgenic mice with enhanced NNCCS function showed increased claudin-5 protein expression in the brain, leading to more consolidated BBB function and preventing brain pathology in injury models (14). Accordingly, the consolidation of BBB is dependent on the vagus nerve, as the neuroactivity of the vagus nerve of the mice was activated, the lateral vagotomy attenuated claudin-5 protein expression (14), and c-fos signals in the NTS was increased with increased NE content in the hypothalamus (13), suggesting that the NTS of the mice is activated. However, these typical phenotypes were not compatible with increased brain permeability during LC stimulation (41). Therefore, it may be hypothesized that β-receptors, not α-receptors, in brain capillaries are involved in the upregulation of claudin-5-mediated BBB consolidation in NNCCS-enhanced mice.
In addition to α-adrenergic receptor-mediated mechanisms that aggravate BBB permeability, cholinergic neurons may regulate BBB function. Although muscarinic and nicotinic receptors are found in brain capillaries, cholinergic nerves do not terminate on brain capillaries (47, 48), raising a discrepancy between the presence of these receptors and undetectable cholinergic nerve ends in capillaries (49). This missing link may be explained by the fact that a non-neuronal ACh system that exists in the endothelial cells of the vasculatures can synthesize and secrete ACh (50, 51); however, the amount of ACh secreted by endothelial cells is predicted to be low compared with that of systemically administered ACh.
However, in contrast to the reports that α-adrenergic receptors are responsible for brain edema, direct evidence suggesting that ACh or cholinergic receptors, not VNS, play a role in sustaining BBB functions is limited. Notably, one study showed that a systemic infusion of ACh decreased blood pressure and increased BBB permeability in normal rats (52). However, this result using a dose affecting blood pressure does not necessarily exclude the possibility that ACh may upregulate BBB function, as demonstrated in our transgenic mice (14), because the underlying mechanism may be different for bolus-injected ACh and constitutively released ACh from endothelial cells.
Several studies have demonstrated the beneficial effects of nicotine in alleviating acute ischemic stroke-induced BBB damage (53). Further, α7 nicotinic ACh receptor agonists prevented BBB disruption in stroke models (54–56). Another study reported that an α7 nicotinic ACh receptor agonist increased the expression of claudin-5 and occludin in endothelial cells in vitro (32), suggesting a possible direct link between nicotinic receptor activation and the upregulation of BBB function. Therefore, beneficial effects of VNS on BBB function in vivo probably may be mediated by ACh, a transmitter from the cholinergic system, or the synthesis system in endothelial cells. However, evidence of very few cholinergic nerves innervating the microvasculature in the brain may raises issues about the ACh origin.
Considering the modulation of BBB function by adrenergic and cholinergic nervous systems, β-adrenergic and nicotinic receptors may be suitable candidates for augmenting BBB function. Moreover, BBB function is influenced by the upregulated expression of BBB proteins and the anti-inflammatory actions of the cholinergic system. Therefore, BBB function is integratively regulated by both of the aspects.
Interestingly, NNCCS activation causes activation of the afferent fibers of the vagus nerve, as observed in the VNS, leading to increased consolidation of BBB functions through the upregulation of claudin-5 protein. This critical and specific result may suggest re-considering the link between BBB function and the cholinergic system, including the NNCCS, endothelial ACh synthesis system, and the classic cholinergic nervous system. Moreover, the above studies provide us with a possible modality to positively regulate BBB function. Interventions in the BBB may suppress the progression of the decline in higher brain functions. Therefore, intervention in those cholinergic systems may help elucidate a precise mechanism and modality to regulate the BBB and higher brain functions.
YK: Writing – original draft, Writing – review & editing.
The author(s) declare financial support was received for the research, authorship, and/or publication of this article.
All results derived from our studies mentioned in this mini-review were supported by the Japan Society for the Promotion of Science Grants-in-Aid for Scientific Research (grant numbers: 21590283, 23590678, 25460333, and 16K08560), by the Smoking Research Foundation, Japan (2018–2020), and by the Vehicle Racing Commemorative Foundation (2020–2022).
This manuscript has been checked and edited for language by Editage, Cactus Communications Co. Ltd, Tokyo, Japan. The project of developing transgenic mice including hChAT tg and hChAT KD mice were supported by Professor Masayuki Tsuda, Institute for Laboratory Animal Research, and Professor Takayuki Sato, Department of Cardiovascular Control, Kochi Medical School, Kochi, Japan. The evaluation of extra-cardiac phenotypes in those transgenic mice was supported by Professor Shuei Sugama, Research Center for Basic Medicine, International University of Health and Welfare, Tochigi, Japan, and Professor Kazuyo Muramoto, Department of Physiology, School of Dentistry, Meikai University, Saitama, Japan, and all members in Department of Bioregulatory Science, Nippon Medical School, Tokyo, Japan.
The author declares that the research was conducted in the absence of any commercial or financial relationships that could be construed as a potential conflict of interest.
The author declared that they were an editorial board member of Frontiers, at the time of submission. This had no impact on the peer review process and the final decision.
All claims expressed in this article are solely those of the authors and do not necessarily represent those of their affiliated organizations, or those of the publisher, the editors and the reviewers. Any product that may be evaluated in this article, or claim that may be made by its manufacturer, is not guaranteed or endorsed by the publisher.
1. Fu WM, Liou HC, Chen YH, Wang SM. Release of acetylcholine from embryonic myocytes in Xenopus cell cultures. J Physiol. (1998) 509:497–506. doi: 10.1111/j.1469-7793.1998.497bn.x
2. Schmid PG, Greif BJ, Lund DD, Roskoski R Jr. Regional choline acetyltransferase activity in the Guinea pig heart. Circ Res. (1978) 42:657–60. doi: 10.1161/01.res.42.5.657
3. Oda RP, Whiteis CA, Schmid PG, Lund DD. Choline and acetylcholine concentration in transplanted rat heart. Am J Physiol. (1987) 252:H125–30. doi: 10.1152/ajpheart.1987.252.1.H125
4. Kakinuma Y, Akiyama T, Sato T. Cholinoceptive and cholinergic properties of cardiomyocytes involving an amplification mechanism for vagal efferent effects in sparsely innervated ventricular myocardium. FEBS J. (2009) 276:5111–25. doi: 10.1111/j.1742-4658.2009.07208.x
5. Rana OR, Schauerte P, Kluttig R, Schröder JW, Koenen RR, Weber C, et al. Acetylcholine as an age-dependent non-neuronal source in the heart. Auton Neurosci. (2010) 156:82–9. doi: 10.1016/j.autneu.2010.04.011
6. Rocha-Resende C, Roy A, Resende R, Ladeira MS, Lara A, de Morais Gomes ER, et al. Non-neuronal cholinergic machinery present in cardiomyocytes offsets hypertrophic signals. J Mol Cell Cardiol. (2012) 53:206–16. doi: 10.1016/j.yjmcc.2012.05.003
7. Roy A, Fields WC, Rocha-Resende C, Resende RR, Guatimosim S, Prado VF, et al. Cardiomyocyte-secreted acetylcholine is required for maintenance of homeostasis in the heart. FASEB J. (2013) 27:5072–82. doi: 10.1096/fj.13-238279
8. Kakinuma Y, Tsuda M, Okazaki K, Akiyama T, Arikawa M, Noguchi T, et al. Heart-specific overexpression of choline acetyltransferase gene protects murine heart against ischemia through hypoxia-inducible factor-1α-related defense mechanisms. J Am Heart Assoc. (2013) 2:e004887. doi: 10.1161/JAHA.112.004887
9. Oikawa S, Kai Y, Mano A, Ohata H, Kurabayashi A, Tsuda M, et al. Non-neuronal cardiac acetylcholine system playing indispensable roles in cardiac homeostasis confers resiliency to the heart. J Physiol Sci. (2021) 71:2. doi: 10.1186/s12576-020-00787-6
10. Roy A, Dakroub M, Tezini GC, Liu Y, Guatimosim S, Feng Q, et al. Cardiac acetylcholine inhibits ventricular remodeling and dysfunction under pathologic conditions. FASEB J. (2016) 30:688–701. doi: 10.1096/fj.15-277046
11. Kakinuma Y, Akiyama T, Okazaki K, Arikawa M, Noguchi T, Sato T. A non-neuronal cardiac cholinergic system plays a protective role in myocardium salvage during ischemic insults. PLoS One. (2012) 7:e50761. doi: 10.1371/journal.pone.0050761
12. Kakinuma Y, Ando M, Kuwabara M, Katare RG, Okudela K, Kobayashi M, et al. Acetylcholine from vagal stimulation protects cardiomyocytes against ischemia and hypoxia involving additive non-hypoxic induction of HIF-1alpha. FEBS Lett. (2005) 579:2111–8. doi: 10.1016/j.febslet.2005.02.065
13. Oikawa S, Kai Y, Tsuda M, Ohata H, Mano A, Mizoguchi N, et al. Non-neuronal cardiac cholinergic system influences CNS via the vagus nerve to acquire a stress-refractory propensity. Clin Sci (Lond). (2016) 130:1913–28. doi: 10.1042/CS20160277
14. Oikawa S, Kai Y, Mano A, Sugama S, Mizoguchi N, Tsuda M, et al. Potentiating a non-neuronal cardiac cholinergic system reinforces the functional integrity of the blood brain barrier associated with systemic anti-inflammatory responses. Brain Behav Immun. (2019) 81:122–37. doi: 10.1016/j.bbi.2019.06.005
15. Borovikova LV, Ivanova S, Zhang M, Yang H, Botchkina GI, Watkins LR, et al. Vagus nerve stimulation attenuates the systemic inflammatory response to endotoxin. Nature. (2000) 405:458–62. doi: 10.1038/35013070
16. Srihagulang C, Vongsfak J, Vaniyapong T, Chattipakorn N, Chattipakorn SC. Potential roles of vagus nerve stimulation on traumatic brain injury: evidence from in vivo and clinical studies. Exp Neurol. (2022) 347:113887. doi: 10.1016/j.expneurol.2021.113887
17. Neren D, Johnson MD, Legon W, Bachour SP, Ling G, Divani AA. Vagus nerve stimulation and other neuromodulation methods for treatment of traumatic brain injury. Neurocrit Care. (2016) 24:308–19. doi: 10.1007/s12028-015-0203-0
18. Zhou L, Lin J, Kui G, Zhang J, Yu Y. Neuroprotective effects of vagus nerve stimulation on traumatic brain injury. Neural Regen Res. (2014) 9:1585–91. doi: 10.4103/1673-5374.141783
19. Boyeson MG, Feeney DM. Intraventricular norepinephrine facilitates motor recovery following sensorimotor cortex injury. Pharmacol Biochem Behav. (1990) 35:497–501. doi: 10.1016/0091-3057(90)90279-q
20. Sutton RL, Hovda DA, Feeney DM. Amphetamine accelerates recovery of locomotor function following bilateral frontal cortex ablation in cats. Behav Neurosci. (1989) 103:837–41. doi: 10.1037/0735-7044.103.4.837
21. Follesa P, Biggio F, Gorini G, Caria S, Talani G, Dazzi L, et al. Vagus nerve stimulation increases norepinephrine concentration and the gene expression of BDNF and bFGF in the rat brain. Brain Res. (2007) 1179:28–34. doi: 10.1016/j.brainres.2007.08.045
22. Roosevelt RW, Smith DC, Clough RW, Jensen RA, Browning RA. Increased extracellular concentrations of norepinephrine in cortex and hippocampus following vagus nerve stimulation in the rat. Brain Res. (2006) 1119:124–32. doi: 10.1016/j.brainres.2006.08.048
23. Lopez NE, Krzyzaniak MJ, Costantini TW, Putnam J, Hageny AM, Eliceiri B, et al. Vagal nerve stimulation decreases blood-brain barrier disruption after traumatic brain injury. J Trauma Acute Care Surg. (2012) 72:1562–6. doi: 10.1097/TA.0b013e3182569875
24. Clough RW, Neese SL, Sherill LK, Tan AA, Duke A, Roosevelt RW, et al. Cortical edema in moderate fluid percussion brain injury is attenuated by vagus nerve stimulation. Neuroscience. (2007) 147:286–93. doi: 10.1016/j.neuroscience.2007.04.043
25. Yang Y, Yang LY, Orban L, Cuylear D, Thompson J, Simon B, et al. Non-invasive vagus nerve stimulation reduces blood-brain barrier disruption in a rat model of ischemic stroke. Brain Stimul. (2018) 11:689–98. doi: 10.1016/j.brs.2018.01.034
26. Kaya M, Orhan N, Karabacak E, Bahceci MB, Arican N, Ahishali B, et al. Vagus nerve stimulation inhibits seizure activity and protects blood-brain barrier integrity in kindled rats with cortical dysplasia. Life Sci. (2013) 92:289–97. doi: 10.1016/j.lfs.2013.01.009
27. Jin Z, Dong J, Wang Y, Liu Y. Exploring the potential of vagus nerve stimulation in treating brain diseases: a review of immunologic benefits and neuroprotective efficacy. Eur J Med Res. (2023) 28:444. doi: 10.1186/s40001-023-01439-2
28. Bansal V, Costantini T, Kroll L, Peterson C, Loomis W, Eliceiri B, et al. Traumatic brain injury and intestinal dysfunction: uncovering the neuro-enteric axis. J Neurotrauma. (2009) 26:1353–9. doi: 10.1089/neu.2008.0858
29. Zhou H, Liang H, Li ZF, Xiang H, Liu W, Li JG. Vagus nerve stimulation attenuates intestinal epithelial tight junctions disruption in endotoxemic mice through α7 nicotinic acetylcholine receptors. Shock. (2013) 40:144–51. doi: 10.1097/SHK.0b013e318299e9c0
30. Dash PK, Zhao J, Kobori N, Redell JB, Hylin MJ, Hood KN, et al. Activation of alpha 7 cholinergic nicotinic receptors reduce blood-brain barrier permeability following experimental traumatic brain injury. J Neurosci. (2016) 36:2809–18. doi: 10.1523/JNEUROSCI.3197-15.2016
31. Gatsona JW, Simpkins JW, Uteshevc VV. High therapeutic potential of positive allosteric modulation of (7 nAChRs in a rat model of traumatic brain injury: proof-of-concept. Brain Res Bull. (2015) 112:35–41. doi: 10.1016/j.brainresbull.2015.01.008
32. Kimura I, Dohgu S, Takata F, Matsumoto J, Kawahara Y, Nishihira M, et al. Activation of the α7 nicotinic acetylcholine receptor upregulates blood-brain barrier function through increased claudin-5 and occludin expression in rat brain endothelial cells. Neurosci Lett. (2019) 694:9–13. doi: 10.1016/j.neulet.2018.11.022
33. Hammarlund ME, Darsalia V, Mjörnstedt F, Pattanaik B, Mallard C, Rocha-Ferreira E. The selective alpha7 nicotinic acetylcholine receptor agonist AR-R17779 does not affect ischemia-reperfusion brain injury in mice. Biosci Rep. (2021) 41:BSR20210736. doi: 10.1042/BSR20210736
34. Mello-Carpes PB, Izquierdo I. The nucleus of the solitary tract → nucleus paragigantocellularis → locus Coeruleus → CA1 region of dorsal hippocampus pathway is important for consolidation of object recognition memory. Neurobiol Learn Mem. (2013) 100:56–63. doi: 10.1016/j.nlm.2012.12.002
35. Lopes LT, Patrone LG, Li KY, Imber AN, Graham CD, Gargaglioni LH, et al. Anatomical and functional connections between the locus coeruleus and the nucleus tractus solitarius in neonatal rats. Neuroscience. (2016) 324:446–68. doi: 10.1016/j.neuroscience.2016.03.036
36. Kawano H, Masuko S. Neurons in the caudal ventrolateral medulla projecting to the paraventricular hypothalamic nucleus receive synaptic inputs from the nucleus of the solitary tract: a light and electron microscopic double-labeling study in the rat. Neurosci Lett. (1996) 218:33–6. doi: 10.1016/0304-3940(96)13115-3
37. Valentine JD, Matta SG, Sharp BM. Nicotine-induced cFos expression in the hypothalamic paraventricular nucleus is dependent on brainstem effects: correlations with cFos in catecholaminergic and noncatecholaminergic neurons in the nucleus tractus solitarius. Endocrinology. (1996) 137:622–30. doi: 10.1210/endo.137.2.8593811
38. Rogers RC, Kita H, Butcher LL, Novin D. Afferent projections to the dorsal motor nucleus of the vagus. Brain Res Bull. (1980) 5:365–73. doi: 10.1016/s0361-9230(80)80006-2
39. Nosaka N. Solitary nucleus neurons transmitting vagal visceral input to the forebrain via a direct pathway in rats. Exp Neurol. (1984) 85:493–505. doi: 10.1016/0014-4886(84)90026-8
40. Blessing WW, Li YW, Wesselingh SL. Transneuronal transport of herpes simplex virus from the cervical vagus to brain neurons with axonal inputs to central vagal sensory nuclei in the rat. Neuroscience. (1991) 42:261–74. doi: 10.1016/0306-4522(91)90163-i
41. Raichle ME, Hartman BK, Eichling JO, Sharpe LG. Central noradrenergic regulation of cerebral blood flow and vascular permeability. Proc Natl Acad Sci U S A. (1975) 72:3726–30. doi: 10.1073/pnas.72.9.3726
42. Sarmento A, Borges N, Lima D. Influence of electrical stimulation of locus coeruleus on the rat blood-brain barrier permeability to sodium fluorescein. Acta Neurochir (Wien. (1994) 127:215–9. doi: 10.1007/BF01808769
43. Sarmento A, Borges N, Azevedo I. Adrenergic influences on the control of blood-brain barrier permeability. Naunyn Schmiedebergs Arch Pharmacol. (1991) 343:633–7. doi: 10.1007/BF00184295
44. Korte N, James G, You H, Hirunpattarasilp C, Christie I, Sethi H, et al. Noradrenaline released from locus coeruleus axons contracts cerebral capillary pericytes via α2 adrenergic receptors. J Cereb Blood Flow Metab. (2023) 43:1142–52. doi: 10.1177/0271678X231152549
45. Elfont RM, Sundaresan PR, Sladek CD. Adrenergic receptors on cerebral microvessels: pericyte contribution. Am J Physiol. (1989) 256:R224–30. doi: 10.1152/ajpregu.1989.256.1.R224
46. Joffre J, Lloyd E, Wong E, Chung-Yeh C, Nguyen N, Xu F, et al. Catecholaminergic vasopressors reduce toll-like receptor agonist-induced microvascular endothelial cell permeability but not cytokine production. Crit Care Med. (2021) 49:e315–26. doi: 10.1097/CCM.0000000000004854
47. Estrada MH, Krause DN. Muscarinic cholinergic receptor sites in cerebral blood vessels. J Pharmacol Exp Ther. (1982) 221:85–90.7062294
48. Edvinsson L, MacKenzie ET. Amine mechanisms in the cerebral circulation. Pharmacol Rev. (1977) 28:275–348.
49. Palmer GC. Neurochemical coupled actions of transmitters in the microvasculature of the brain. Neurosci Biobehav Reviews. (1986) 10:79–101. doi: 10.1016/0149-7634(86)90020-5
50. Kirkpatrick CJ, Bittinger F, Nozadze K, Wessler I. Expression and function of the non-neuronal cholinergic system in endothelial cells. Life Sci. (2003) 72:2111–6. doi: 10.1016/s0024-3205(03)00069-9
51. Milner P, Kirkpatrick KA, Ralevic V, Toothill V, Pearson J, Burnstock G. Endothelial cells cultured from human umbilical vein release ATP, substance P and acetylcholine in response to increased flow. Proc Biol Sci. (1990) 241:245–8. doi: 10.1098/rspb.1990.0092
52. Domer FR, Boertje SB, Bing EG, Reddix I. Histamine- and acetylcholine-induced changes in the permeability of the blood-brain barrier of normotensive and spontaneously hypertensive rats. Neuropharmacology. (1983) 22:615–9. doi: 10.1016/0028-3908(83)90153-3
53. Hu X, Dong J, Geng P, Sun Y, Du W, Zhao X, et al. Nicotine treatment ameliorates blood-brain barrier damage after acute ischemic stroke by regulating endothelial scaffolding protein Pdlim5. Transl Stroke Res. (2023). doi: 10.1007/s12975-023-01158-0
54. Aguado L, Joya A, Garbizu M, Plaza-García S, Iglesias L, Hernández MI, et al. Therapeutic effect of α7 nicotinic receptor activation after ischemic stroke in rats. J Cereb Blood Flow Metab. (2023) 43:1301–16. doi: 10.1177/0271678X231161207
55. Chen S, Sun Y, Li F, Zhang X, Hu X, Zhao X, et al. Modulation of α7nAchR by melatonin alleviates ischemia and reperfusion-compromised integrity of blood-brain barrier through inhibiting HMGB1-mediated microglia activation and CRTC1-mediated neuronal loss. Cell Mol Neurobiol. (2022) 42:2407–22. doi: 10.1007/s10571-021-01122-2
Keywords: non-neuronal cholinergic system, non-neuronal cardiac cholinergic system, acetylcholine, blood brain barrier, heart, brain
Citation: Kakinuma Y (2024) Non-neuronal cholinergic system in the heart influences its homeostasis and an extra-cardiac site, the blood-brain barrier. Front. Cardiovasc. Med. 11:1384637. doi: 10.3389/fcvm.2024.1384637
Received: 10 February 2024; Accepted: 18 March 2024;
Published: 27 March 2024.
Edited by:
Sorin Hostiuc, Carol Davila University of Medicine and Pharmacy, RomaniaReviewed by:
Sae Uchida, Tokyo Metropolitan Institute for Geriatrics and Gerontology, Japan© 2024 Kakinuma. This is an open-access article distributed under the terms of the Creative Commons Attribution License (CC BY). The use, distribution or reproduction in other forums is permitted, provided the original author(s) and the copyright owner(s) are credited and that the original publication in this journal is cited, in accordance with accepted academic practice. No use, distribution or reproduction is permitted which does not comply with these terms.
*Correspondence: Yoshihiko Kakinuma azEyNDE3ODUzQG5tcy5hYy5qcA==
Disclaimer: All claims expressed in this article are solely those of the authors and do not necessarily represent those of their affiliated organizations, or those of the publisher, the editors and the reviewers. Any product that may be evaluated in this article or claim that may be made by its manufacturer is not guaranteed or endorsed by the publisher.
Research integrity at Frontiers
Learn more about the work of our research integrity team to safeguard the quality of each article we publish.