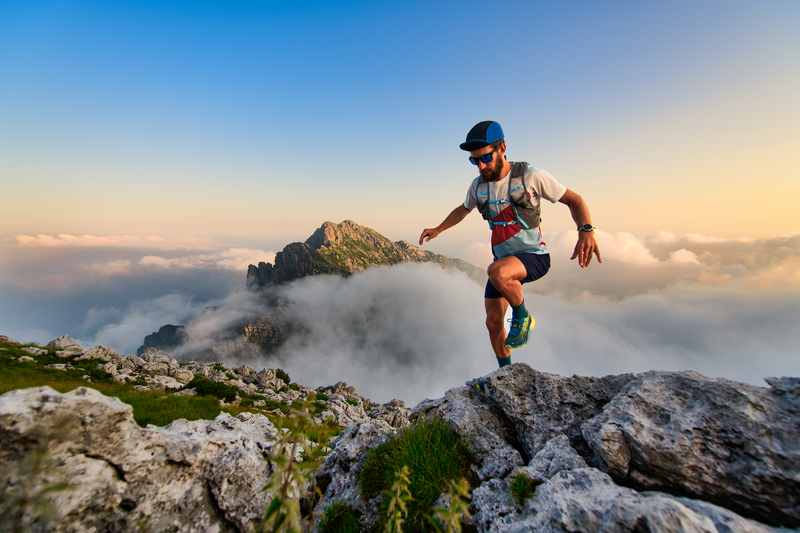
95% of researchers rate our articles as excellent or good
Learn more about the work of our research integrity team to safeguard the quality of each article we publish.
Find out more
REVIEW article
Front. Cardiovasc. Med. , 18 April 2024
Sec. Cardiovascular Pharmacology and Drug Discovery
Volume 11 - 2024 | https://doi.org/10.3389/fcvm.2024.1372055
Inflammation and dyslipidemia are critical inducing factors of atherosclerosis. Peroxisome proliferator-activated receptors (PPARs) are ligand-activated transcription factors and control the expression of multiple genes that are involved in lipid metabolism and inflammatory responses. However, synthesized PPAR agonists exhibit contrary therapeutic effects and various side effects in atherosclerosis therapy. Natural products are structural diversity and have a good safety. Recent studies find that natural herbs and compounds exhibit attractive therapeutic effects on atherosclerosis by alleviating hyperlipidemia and inflammation through modulation of PPARs. Importantly, the preparation of natural products generally causes significantly lower environmental pollution compared to that of synthesized chemical compounds. Therefore, it is interesting to discover novel PPAR modulator and develop alternative strategies for atherosclerosis therapy based on natural herbs and compounds. This article reviews recent findings, mainly from the year of 2020 to present, about the roles of natural herbs and compounds in regulation of PPARs and their therapeutic effects on atherosclerosis. This article provides alternative strategies and theoretical basis for atherosclerosis therapy using natural herbs and compounds by targeting PPARs, and offers valuable information for researchers that are interested in developing novel PPAR modulators.
Cardiovascular disease (CVD) has become the number one cause of human death due to changes in lifestyle, especially high-fat and high-caloric diet, and aging of population. It is estimated that approximately 170,000 people die from CVD each year (1). Of note, atherosclerosis is an important cause of CVD events (2). In the year of 2020, nearly 2 billion people suffered from carotid atherosclerosis in the world including 270 million people in China (3, 4). Atherosclerosis is a chronic inflammatory and degenerative process that primarily occurs in large- and medium-sized arteries. This disease is characterized by accumulation of fatty and fibrous materials and calcium minerals in the intima layer of arteries (5, 6).
Inflammation drives all phases of atherosclerosis including initiation, metaphase, advanced phase, and rupture or regression (6). Thus, inflammatory factors, such as C-reactive protein (CRP), interleukin (IL)-6, and tumor necrosis factor-α (TNF-α), are consistently elevated in atherosclerosis. Furthermore, receptors and other molecules involved in inflammation, such as toll-like receptor (TLR), particularly, TLR2 and TLR4, are augmented in human atherosclerotic plaques (7, 8). Dyslipidemia, characterized by high levels of total cholesterol (TC) and triglyceride (TG) and low levels of high-density lipoprotein (HDL) cholesterol (HDL-c), is equally or even more dangerous for the onset and development of atherosclerosis. It is acknowledged that low-density lipoprotein (LDL) cholesterol (LDL-c) or LDL particles and hypertriglyceridemia or TG-rich lipoproteins are leading inducing factors of atherosclerosis (9, 10).
Peroxisome proliferator-activated receptors (PPARs) are recognized as promoters of peroxisome proliferation more than 40 years ago (11). Due to their various functions, research on PPARs has grown exponentially in recent years. Notably, the distribution and function of PPARs exhibit organ- and cell-specificity. PPARα is chiefly expressed in heart, liver, skeletal muscle, and cardiovascular system; PPARβ/δ is widely distributed in the body; and PPARγ is highly expressed in white adipose tissue (12–14). The roles of PPARs in physiological and pathological conditions have been reviewed recently by distinct groups (14–16). Mechanistically, PPARs heterodimerize with retinoid X receptor (RXR) and bind to specific DNA regions of target genes (AGGTCAXAGGTCA, with X being a random nucleotide) that are termed as peroxisome proliferator hormone response elements. Ligand activation triggers conformational changes of PPAR-RXR and finally activate the transcription of target genes. Notably, PPARs regulate multiple genes associated with cellular lipid metabolism and inflammation in cardiovascular system (14). Downregulation of PPARα is found to decrease hepatic de novo lipogenesis, while PPARα agonists restore lipid homeostasis in the liver (17). Mechanistically, PPARα induces the expression of genes involved in fatty acid uptake, conversion, and catabolism through β-oxidation pathway, leading to reductions in fatty acid and TG synthesis and hepatic very low-density lipoprotein production. Similar to PPARα, PPARβ activates carnitine palmitoyl transferase (CPT), which facilitates fatty acid transport across mitochondrial membrane and the subsequent β-oxidation (18). Furthermore, PPARβ activation enhances energy expenditure through upregulation of heat-producing enzymes including uncoupling protein 1 and 3 in brown adipose tissue, thereby protecting against obesity and fatty liver. On the contrary, PPARγ agonists, such as rosiglitazone, cannot decrease TG and fatty acid levels. Mechanistically, PPARγ increases glucose utilization, thereby decreasing glucose–fatty acid cycle and the subsequent upregulation of the genes involved in fatty acid synthesis and uptake (19).
Moreover, activated PPARs can interact with other transcription factors that are involved in inflammation, such as activator protein 1 (AP-1) and nuclear factor kappa B (NF-κB), resulting in transcriptional repression (14, 20). For instance, PPARα activation suppresses inflammatory responses in different cells by inhibiting TLR4/NF-κB and AP-1 signaling pathways (14, 17–22). PPARβ is demonstrated to decrease inflammation via activation of AMP-activated protein kinase (AMPK) and inactivation of mitogen-activated protein kinase (MAPK) signaling pathways. However, deletion or repression of PPARβ expression in myeloid cells also decreases atherosclerosis and inflammatory molecules by modulating the PPARβ/B cell lymphoma 6 axis (14, 23). Moreover, PPARγ activation has been demonstrated to inhibit release of inflammatory factors via activating AMPK and suppressing multiple signaling pathways including TLR4, MAPK, and WNT/β-catenin (14). Therefore, PPARs are considered as important targets for CVD therapy and other diseases.
In a previous article, we reviewed PPARs' regulation and their roles in atherosclerosis as well as synthesized PPAR agonists and antagonists (14). Although synthetic PPAR modulators exhibit attractive potential in atherosclerosis therapy, these compounds induce various side effects and show contrary therapeutic effects in different participants and animal models. Notably, phytochemical compounds show therapeutic effects in different diseases by modulation of PPARs (24, 25), and they are considered as preventive agents for metabolic syndrome including nonalcoholic fatty liver disease (NAFLD) by targeting PPARs (26, 27). Given multiple diseases, particularly NAFLD, diabetes, obesity, and fibrosis, are closely associated with the onset and development of atherosclerosis (28–32), compounds with the activities of ameliorating the above diseases are useful for atherosclerosis therapy. Importantly, the majority of natural products exhibit good therapeutic efficacy and safety compared to synthetic medications (15, 33). These properties suggest that natural products are potential candidate molecules for atherosclerosis therapy. This article reviews the roles of natural herbs and compounds in treatment of atherosclerosis through activation of PPARs by focusing on lipid metabolism and inflammation. Recent literatures, mainly from 2020 to present, published in PubMed, Web of Science, and Google Scholar were screened out using traditional Chinese medicine (TCM), flavonoid, acid, alkaloid, terpenoid, phenolic compound, and carbohydrate in combination with PPAR as key words.
TCMs have been used for treatment of metabolic disorders and CVDs for hundreds of years. Recent studies have demonstrated that TCMs ameliorate hyperlipidemia and atherosclerosis through modulation of PPARs (Figure 1). Huo-Xue-Qu-Yu formula (HXQY, 活血祛瘀方) ameliorates lipid profiles including apolipoprotein (Apo) B and ApoA1 in rats with NAFLD via upregulating the expression of PPARα and CPT-1 in the liver, thereby improving symptoms of NAFLD (34). Similarly, heart-protecting musk pill (麝香护心丸) is found to attenuate atherosclerosis partially via activating PPARα/CPT-1α signaling pathway in ApoE-deficient mice (35). TCM believes that “phlegm stasis interjunction (痰瘀互结)” is an important inducing factor in the occurrence and development of atherosclerosis. Dan-Lou prescription (丹蒌方) has been demonstrated to reduce phlegm, repair diseased blood vessels, and eliminate hyperlipidemia, thus ameliorating atherosclerosis. Notably, this prescription enhances cholesterol efflux by activating PPARα/ATP-binding cassette transporter (ABC) A1 signaling pathway (36).
Figure 1. Mechanisms of action of TCM prescriptions and natural bioactive molecules in atherosclerosis therapy by targeting peroxisome proliferator-activated receptors (PPARs). TCM prescriptions and natural bioactive molecules including flavonoids, natural acids, alkaloids, terpenoids, and phenolic compounds mainly decreases lipid accumulation by activating AMP-activated protein kinase (AMPK) and the subsequent signaling pathways including PPARα/carnitine palmitoyl transferase (CPT)-1 and acyl-CoA oxidase 1 (ACOX1)-mediated fatty acid β-oxidation in liver and PPARγ/liver X receptor (LXR) α/ATP-binding cassette transporter (ABC) A1/ABCG1-mediated cholesterol efflux from macrophages to apolipoprotein (Apo) A1 and high-density lipoprotein (HDL) particles, thereby decreasing foam cell formation. Moreover, some TCM prescriptions and natural molecules may decrease cluster of differentiation (CD) 36-mediated lipid absorption via suppressing PPARγ, thereby reducing lipid accumulation in macrophages. Notably, TCM prescriptions and natural molecules primarily ameliorate inflammation by suppressing mitogen-activated protein kinase (MAPK)/nuclear factor kappa B (NK-κB) and phosphoinositide-3 kinase (PI3K)/protein kinase B (AKT/PKB)/NK-κB signaling pathways through activation of PPARγ. Furthermore, these natural compounds can inhibit Toll-like receptor (TLR)4/myeloid differentiation factor 88 (MyD88)/NF-κB signaling pathway and promote macrophage shift to an anti-inflammatory M2 type through activation of PPARα. Notably, natural compounds may stimulate PPARγ coactivator (PGC)-1β–estrogen related receptor α to activate PPARβ/PPARγ signaling pathways and enhance protein kinase A (PKA)/AMPK signaling pathway to upregulate PPARα in the liver. Except for NF-κB, nuclear transcription factor activator protein 1 (AP-1) is involved in the modulatory effects of PPARs on anti-inflammation. These beneficial effects of TCMs are supposed to retard the development of atherosclerosis. IKK: inhibitor of nuclear factor κB kinase subunit; IL: interleukin; TNF-α: tumor necrosis factor α.
In addition to activating PPARα signaling, many TCM prescriptions stimulate PPARγ–liver X receptor (LXR) α–ABCA1/ABCG1 signaling pathways, thereby ameliorating lipid profiles and atherosclerosis through upregulation of reverse cholesterol transport (RCT). Qi-Huang-Zhu-Yu Formula (QHZY, 岐黄茱萸方) enhances cholesterol efflux by activating PPARγ–LXRα–ABCA1/ABCG1 signaling pathways (37). Notably, Qing-Xue-Xiao-Zhi formula (QXXZ, 清血消脂方), Si-Ni decoction (四逆汤), Qi-Shen-Yi-Qi Pill (芪参益气丸), and Yin-Xing-Tong-Mai decoction (银杏通脉汤) have been demonstrated to attenuate hyperlipidemia and atherosclerosis by facilitating RCT through upregulation of PPARγ–LXRα–ABCA1/ABCG1 signaling pathways (38–41). Moreover, Dang-Gui-Shao-Yao-San (当归芍药散), a well-known Chinese medicine formula, improves lipid metabolism and inhibits neuroinflammation by activating LXR–PPARγ signaling pathway (42). However, Hua-Tan-Jiang-Zhuo decoction (化痰降浊汤) alleviates TC and TG levels mainly by inhibiting the gene expression of PPARγ, cholesterol 7-α-hydroxylase A1, and sterol response element-binding protein (SREBP)-1c in hyperlipidemic rats (43).
TCMs also suppress inflammation by modulating PPAR signaling pathways (Figure 1). Shen-Tong-Zhu-Yu decoction (参通逐瘀汤) reduces secretion of pro-inflammatory cytokines, such as IL-1β, IL-6, and TNF-α in rheumatoid arthritis fibroblast-like synoviocytes. Mechanistically, this decoction inhibits phosphorylation of p38 MAPK and activates PPARγ, thereby modulating the p38-MAPK/PPARγ signaling pathway (44). Shen-Hong-Tong-Luo Formula (参红通络方) has been used in clinic for more than 30 years in China. This formula inhibits reactive oxygen species (ROS) accumulation and reverses lipopolysaccharide (LPS)- and oxidized LDL-induced inflammation and lipid accumulation in macrophages by activating PPARγ/LXRα/ABCA1 pathway (45). Schisandra sphenanthera improves liver steatosis and inflammation via activating PPARα/γ signaling in C57BL/6J mice with NAFLD (46). Compound Dan-Shen Dripping Pill (CDDP, 复方丹参滴丸) or QHZY alleviates inflammation via modulating PPARγ/NF-κB p65 signaling pathway (37, 47). The major anti-atherosclerotic components of Compound Dan-Shen formula are ginsenoside Rg1, notoginsenoside R1, and protocatechuic aldehyde; these molecules inhibit endothelial cell damage via suppressing focal adhesion kinase (FAK)-phosphatidylinositol 3-kinase (PI3K)/protein kinase B (PKB/AKT) signaling pathway (48). In ApoE-deficient mice, QXXZ inhibits inflammation by suppressing TLR4-myeloid differentiation factor 88 (MyD88)-NF-κB signaling pathway (38). Notably, Bu-Shen-Kang-Shuai formula and Tan-Yu-Tong-Zhi formula ameliorate atherosclerosis potentially via promoting macrophage polarization towards an M2 phenotype through activation of PPARγ and downregulation of NK-κB (49, 50). However, Gynostemma pentaphyllum, a TCM that is generally used to treat hypercholesterolemia and inflammation, has been demonstrated to reduce obesity and obesity-related inflammation by down-regulating PPARγ signaling pathway (51).
Plants-derived flavonoids have been demonstrated to improve lipid metabolism and inflammation by modulating PPAR signaling pathways (Figures 1, 2). These natural flavonoids provide a new therapeutic direction for treatment of atherosclerosis. The anti-inflammatory and anti-allergic potential as well as the basic structure of some dietary flavonoids have been reviewed recently by Rakha et al. (52). Moreover, the CVD-protecting effects of myricetin have been summarized in the literature (53).
Figure 2. Chemical structures of some bioactive flavonoids with potential anti-atherosclerotic effects.
Citrus flavonoids play an important role in treatment of dyslipidemia and atherosclerosis. The roles of Citrus fruits-derived compounds in modulation of metabolic diseases have been reviewed recently by Aslan et al. (54). Nobiletin is an active component of citrus peel. This molecule increases the expression of PPARγ but not PPARα. Furthermore, it activates AMPK, thus promoting the expression of ABC transporters including ABCA1 and ABCG1. Notably, the LXRα–PPARγ loop amplifies its action (55). Rosa rugosa Thunb- and Rosa davurica Pall. fruits-derived flavonoids upregulate the expression of PPARα and its downstream genes that are involved in lipid metabolism (56, 57). Genestein improves lipid metabolism by upregulating PPARα and activating estrogen receptor β-AKT-mammalian target of rapamycin (mTOR) signaling pathway (58). Hesperidin decreases TG levels by enhancing PPARα and suppressing PPARγ and other lipogenic genes including SREBP-1, fatty acid synthesis (FAS), and stearoyl-CoA desaturase; it reduces TC by suppressing cholesterol absorption through downregulation of fatty acid binding protein (FABP) and retinol binding protein (59).
Flavonoids are found to improve RCT by activating PPARγ/PPARα–ABC transporter pathway. For example, sea buckthorn flavonoids improve hyperlipidemia by up-regulating PPARα/CPT-1α and PPARγ/ABCA1 signaling pathways (53). Total flavonoid extract obtained from Psoralea corylifolia L. seeds alleviates oxidized-LDL-induced foam cell formation via enhancing PPARγ–ABCA1/ABCG1 signaling pathways in vitro and in LDLR-deficient mice (60). In line with these findings, other compounds including mangiferin, quercetin, astragalin, and biochanin A have been demonstrated to ameliorate atherosclerosis via enhancing macrophage cholesterol efflux and RCT through activation of the PPARγ–LXRα–ABCA1/ABCG1 signaling pathways (61–66). Similarly, S. baicalensis-derived flavonoids and baicalein regulates glucose and lipid homeostasis through upregulation of AMPK/PPARγ/LXRα signaling pathway (67, 68). Homoeriodictyol and hesperidin-7-O-β-D-glucopyranoside are found to significantly increase the level of PPARγ protein, providing new candidates for treatment of atherosclerosis (69). Interestingly, amentoflavone prevents oxidized-LDL-induced lipid accumulation by suppressing PPARγ/cluster of differentiation (CD) 36-mediated lipid uptake (70). It is worth noting that several flavonoids exhibit powerful lipid-lowering effects in clinical studies as reviewed recently Gouveia et al. (71).
Accumulating evidence have demonstrated that some flavonoids reduce inflammation by regulating PPAR signaling pathway (Figure 1). Formononetin, an Astragalus-derived isoflavone, inhibits inflammation by reducing the release of proinflammatory cytokines (72). Furthermore, it reduces oxidized-LDL-induced endothelial injury by stimulating PPARγ signaling pathway, contributing to its anti-atherosclerotic effects (9). Biochanin A activates PPARγ/LXRα/ABC transporter and PPARγ/heme oxygenase 1 signaling pathways to suppress hyperlipidemia-induced inflammation in ApoE-deficient mice (65). Similarly, astragalin stimulates PPARγ–LXRα–ABCA1/ABCG1 signaling pathways, which in turn suppress TLR4/NF-κB signaling pathway, thereby inhibiting inflammation in foam cells (64). Propolis-derived flavonoids reduce inflammatory cytokines and endoplasmic reticulum (ER) stress by activating PPARγ in a myocardial infarction model (73). Saikosaponin A and anthocyanins decrease the release of pro-inflammatory cytokines by activating PPARγ, thereby suppressing the NF-κB signaling pathway (74, 75). However, genistein reverses Ang II-induced downregulation of PPARγ to inhibit the expression of CRP and matrix metalloproteinase 9 in vascular smooth muscle cells (VSMCs), thereby reducing inflammatory responses in atherosclerosis (76).
The structure of some bioactive natural acids and their mechanisms of action are shown in Figures 1, 3. The widely distributed chlorogenic acid and caffeine acid are demonstrated to benefit health and cardiovascular system (77). The anti-obesity properties of chlorogenic acid have been recently reviewed by Kumar et al. (78). Notably, chlorogenic acid and caffeine acid may act synergistically on reducing lipid deposition in macrophages via inhibiting PPARγ signaling pathway (77). Furthermore, 5-aminolevulinic acid-mediated sonodynamic therapy improves cholesterol efflux via activating PPARγ–LXRα–ABCA1/ABCG1 signaling pathways, enhancing efferocytosis and cholesterol efflux, and eventually ameliorating atherosclerosis (79).
Oleic acid prevents intracellular lipid accumulation in human macrophages through modulation of PPARs and down-regulation of ApoB48 receptor, suggesting the role of monounsaturated fatty acid in regulation of postprandial TG-rich lipoprotein/ApoB48 receptor axis (80). Dodecahexaenoic acid (DHA) ameliorates postprandial hyperlipidemia potentially by upregulating PPARα and the genes involved in fatty acid β-oxidation and down-regulating TG and ApoB secretion (81). Furthermore, ω-3 polyunsaturated fatty acids (PUFAs) attenuate hepatic steatosis through upregulation of PPARα/CPT-1α signaling pathway (82). Supplement of DHA-rich fish oil increases PPARγ activity in peripheral blood mononuclear cells of the participants (83). However, administration of DHA rapidly increases the production of cyclic adenosine monophosphate inside cilia, and finally activates PPARγ to initiate adipogenesis in preadipocytes (84).
Hydroxypentaenoic acid reduces LDL-c levels and increases HDL-c levels in atherosclerotic animal models, leading to reductions in aortic atherosclerotic plaques (85–87). Mechanistically, this molecule acts as a PPAR ligand and elevates LXRs–ABCA1/ABCG1 signaling pathways (85, 88). Similarly, 12-Hydroxyeicosapentaenoic acid reduces foam cell formation and atherosclerosis via activation of PPARγ–ABCA1/ABCG1 signaling pathways (89, 90). 8-hydroxyeicosapentaenoic acid is a pan PPAR activator and has beneficial effects against dyslipidemia and atherosclerosis (86). However, medium-chain structured lipids ameliorate high-fat diet-induced atherosclerosis potentially by reducing the expression of PPARγ (91). It seems that carbon number of fatty acids plays a role in regulation of PPARγ.
Notably, arachidonic acid acts as an activator of PPARα (92) and has a therapeutic effect on atherosclerosis (93). Taurine is one of the most abundant arachidonic acid in animals. It counteracts chronic inflammation in adipose tissues potentially via promoting macrophage polarization toward an anti-inflammatory M2 phenotype (94). Similarly, 12-Hydroxyeicosapentaenoic acid promotes macrophage shift towards an anti-inflammatory M2 phenotype (90, 95), thereby inhibiting atherosclerosis (96). β-aminoisobutyric acid protects against vascular inflammation via upregulating PPARγ coactivator (PGC)-1β–estrogen related receptor α–PPARβ/PPARγ signaling pathways (97). As reviewed recently, amino acid derivatives may alleviate inflammation and improve energy expenditure and obesity by targeting PPARs (98). Furthermore, PUFAs are involved in resolution of inflammation (99, 100). Dietary ω-3 and ω-6 PUFAs upregulate gene expression of PPARs, thereby suppressing inflammation and lipid accumulation (101). For instance, both PPARγ and PPARα can be activated by ω-3 PUFAs (102). Unfortunately, ω-3 PUFAs show limited effects on CVD events in clinical trials (9).
Some natural alkaloids and their derivatives are reported to be PPAR modulators (Figures 1, 4). For instance, (S)-tryptophan-betaxanthin and berberrubine are demonstrated to be leading compounds of pan PPAR activators based on a screening of 30,000 TCM candidates (103). Berberine, an isoquinoline alkaloid, has been used for treatment of CVDs as reviewed recently by Song et al. (104). In diabetic atherosclerosis, berberine stimulates Krüppel-like factor 16/PPARα signaling pathway, thereby improving lipid metabolism (105). In adipose tissue, berberine activates AMPK/Sirtuin 1 axis, an energy metabolic sensing pathway, increasing PPARγ deacetylation, thereby promoting adipose tissue remodeling and thermogenesis through upregulation of uncoupling protein 1 (106). In liver, berberine treatment increases lipid oxidation by upregulating PPARα and its downstream genes including CPT-1α and acyl-CoA oxidase 1 (ACOX1) (107). In vitro, berberine and its major metabolite berberrubine attenuate lipid accumulation in HepG2 cells via upregulating PPARα signaling pathway (108). Similarly, the protective effect of theobromine against NAFLD is partially attributed to its upregulation of PPARα and CPT-1α (109). Furthermore, nuciferine improves hepatic steatosis by activating PPARα/PGC-1α pathway in diabetic mice (110). Betaine attenuates hyperlipidemia by activating PPARα and PPARγ and their downstream gene LXRα (111). Hericerin, an indolinone meroterpenoid alkaloid, has been defined as a strong PPARγ agonist with potential hypoglycemic and hypolipidemic effects (112). Coffee-derived trigonelline, an alkaloid derivative of niacin (vitamin B3), alleviates hyperlipidemia by increasing PPARα and decreasing PPARγ expression (113). Additionally, capsaicin may suppress obesity by suppressing PPARγ signaling pathway (42, 114).
Berberine treatment suppresses systemic inflammation by reducing the production of inflammatory factors including TNF-α and LPS through activation of PPARα and its potential target thyroid hormone responsive (107). The anti-inflammatory mechanisms of betaine are associated with inhibition of TLR4/NF-κB signaling pathway and regulation of PPARs (115). Furthermore, betaine alleviates high-fat diet-induced inflammation by modulating silent information regulator 1/SREBP1/PPARα signaling pathway, thereby suppressing the expression of NF-κB (116). Capsaicin inhibits oxidized-LDL-induced ROS generation and VSMC phenotypic switching by activating PPARα (117), and ameliorates diabetic retinopathy by suppressing PPARγ–poldip2–NADPH oxidase 4 signaling pathway (118). Interestingly, the anti-inflammatory effect of capsaicin is LXRα–PPARγ dependent (119). Moreover, trigonelline exhibits antioxidant and anti-inflammatory effects partially by activation of PPARα (113, 120), piperine inhibits cardiac fibrosis via activating PPARγ and the following inhibition of AKT/glycogen synthase 3β signaling pathway (121), and nuciferine suppresses myocardial injury by upregulating PPARγ in mice (122).
Terpenoids are found to ameliorate hyperlipidemia by targeting PPARs (Figures 1, 5). Eugenol, a phenolic monoterpenoid, increases the expression of PPARα, partially contributing to its hypolipidemic and antioxidant properties in diabetic rats (123). Sweroside protects against obesity mainly by enhancing PPARα (124). Ginsenoside Rg1, baicalin, and Resina Commiphora-derived terpenoids also improve lipid metabolism and atherosclerosis through upregulation of PPARα and its target genes including CPT-1 and ACOX1 (125–127). Saikosaponin D and diosgenin serve as PPARα agonists, promoting PPARα-mediated fatty acid oxidation and inhibiting CD36-mediated fatty acid uptake and SREBP-1c-mediated de novo lipogenesis (128, 129). Oleanolic acid, a pentacyclic triterpenoid, and (E)-β-caryophyllene, a bicyclic sesquiterpene hydrocarbon, act as dual activator of PPARα and PPARγ, decreasing hyperglycemia and lipid accumulation (130, 131). Furthermore, Saikosaponin A and ginsenoside 20(R/S)-Rg3 act as natural PPARγ activators, ameliorating hyperlipidemia and atherosclerosis (72, 132). However, ginsenoside Rg1 inhibits lipid uptake via downregulation of PPARγ (125), Ganoderic acid A suppresses oxidized-LDL-induced lipid accumulation in THP-1-derived macrophages by inhibiting Notch1-PPARγ-CD36 signaling pathway (111), and D-limonene, decreases lipid anabolism by decreasing the expression of PPARγ and SREBP-1c, and activating the AMPK signaling pathway in high-calorie diet-induced obese rats (133).
Sea cucumber saponins reduce lipogenesis and promote fatty acid β-oxidation via inhibiting SREBP-1c and enhancing the expression of PPARα and ACOX1, respectively, thereby improving lipid deposition in rodents (134–137). In combination with eicosapentaenoic acid-enriched phospholipids, sea cucumber saponins further reduce hepatic TG partially by enhancing the expression of PPARα as reviewed by Lin et al. (137). Interestingly, sea cucumber saponin treatment induces changes of lipid metabolism-related genes including PPARα in rhythm, suggesting saponin may modulate lipid metabolism by regulating the clock genes, such as CLOCK and BMAL1 (137, 138). The major bioactive component of saponin, echinoside A, also regulates the expression of some key genes that are involved in lipid metabolism in a diurnal manner (139). The marine-derived PPAR activators have been reviewed recently by D'Aniello et al. (140).
PPARγ plays a vital role in anti-inflammatory mechanisms of action of terpenoids (Figure 1). Saponin notoginsenoside Fc ameliorates inflammatory response in high glucose-induced endothelial cell injury partly by activation of PPARγ (141). Stevioside attenuates inflammation by upregulating PPARγ, thereby activating PI3K/AKT signaling pathway in a middle cerebral artery occlusion/reperfusion rat model (142). Ginsenoside Rg3 represses FAK-mediated expression of vascular cell adhesion molecule (VCAM)-1 and intercellular cell adhesion molecule (ICAM)-1 through activation of PPARγ (143). Saikosaponin A or britannin decreases inflammation potentially by activating PPARγ, thereby downregulating NF-κB signaling pathway (74, 144). Furthermore, lycopene, β-carotene, and oridonin may act as PPARγ modulators (145, 146). Interestingly, geraniol, an acyclic mono-terpenoid alcohol, decreases LPS/interferon γ-induced NLRP3 inflammasome activation and macrophage M1-type polarization through inhibiting PPARγ methylation (147). However, Akebia saponin D is reported to ameliorate high-fat diet-induced gut barrier injury via repressing PPARγ-FABP4 signaling pathway (110). Additionally, β-elemene augments the mRNA expression of PPARβ and CPT-1α and sirtuin 3, thereby blocking lipid-induced inflammatory pathways (148).
Phenolic compounds are widely distributed bioactive compounds, they are found to exert lipid-modulatory and anti-inflammatory functions by regulating PPARs (Figures 1, 6). Resveratrol ameliorates hepatocyte steatosis via activating protein kinase A/AMPK/PPARα signaling pathway (149). It abolishes intestinal fatty acid and monoglyceride accumulation via activation of PPARα/PPARγ and their downstream ABCA1 and ABCG1 transporters in atherosclerotic mice (150). Furthermore, it is found to promote fatty acid β-oxidation by enhancing MAPK/PPAR signaling pathway (151). Polydatin, the glucoside of resveratrol, activates PPARβ signaling pathway to improve lipid metabolism (152). Raspberry ketone increases phosphorylation of AMPK to improve fatty acid oxidation through upregulation of PPARα and CPT-1 (153).
Figure 6. Structure of some bioactive phenolic compounds with potential anti-atherosclerotic effects.
Ellagic acid has anti-atherogenic and cardioprotective properties, suggesting its role in atherosclerosis therapy (154). Mechanistically, ellagic acid regulates the genes that are mainly correlated with PPAR signaling pathway, thereby ameliorating lipid metabolism (155). Hydroxytyrosol, a polyphenol, decreases the expression of FAS, SREBP-1c, and PPARγ, ameliorating TC and TG levels and hepatic steatosis in ethanol-induced HepG2 cells (156). In addition, methyl brevifolincarboxylate, a polyphenolic compound, improves hepatic lipid accumulation through upregulation of AMPKα/PPARα signaling pathway and the target genes of PPARα including CPT-1 and ACOX1 in free fatty acid-treated hepatocytes (157). Sesamol, a phenolic compound derived from sesame oil, activates PPAR signaling pathway, leading to enhanced fatty acid oxidation, cholesterol efflux, and catabolism, thus accelerating lipid consumption and reducing intracellular lipid accumulation in HepG2 cells (158).
Proanthocyanidin A2 and ellagic acid exhibit anti-inflammatory properties potentially by upregulating PPARγ signaling pathway (159, 160). Cannabidiol, a nonpsychoactive cannabinoid, inhibits inflammation through downregulation of TLR4/NLRP3/Caspase-1 signaling pathway in a PPARγ-dependent manner in Caco-2 cells (161). Furthermore, cannabidiol might exert anti-inflammatory effects by either directly or indirectly modulating PPARγ/NF-κB/nuclear factor erythroid 2-related factor 2 signaling in urothelial cells (162). Honokiol dramatically reduces production of proinflammatory cytokines in mice with ulcerative colitis that is induced by dextran sulfate sodium (DSS) partially via upregulating PPARγ and suppressing TLR4/NF-κB signaling pathway (163). The activation of PPARγ by honokiol is also associated with its effects on preventing against hyperglycemia and CVD (20). Furthermore, resveratrol suppresses hepatic inflammation via activation of PPARγ and downregulation of ER stress-mediated apoptosis (164). Forsythiaside A regulates PPARγ/RXR-α complex, inhibiting TLR4/MAPK/NF-κB and NF-κB/MLCK/MLC2 signal pathways, thus suppressing LPS-induced inflammation and epithelial barrier damages. However, forsythiaside A enhances the expression of PPARγ/RXR-α complex in lung and inhibits this complex in colon, suggesting its cellular-specific effects (165). Additionally, proanthocyanidin alleviates liver ischemia/reperfusion injury by suppressing autophagy and apoptosis through regulation of PPARα/PGC-1α signaling pathway (166). Mechanisms of action of phenolic compounds in regulation of inflammation is concluded in Figure 1.
Polysaccharides are a kind of carbohydrate polymers that are generally consisted of more than ten monosaccharides through glycosidic linkages in linear or branched chains. Given polysaccharides generally have low toxicity and various biological activities, such as antioxidant, anti-inflammatory, and anti-atherosclerosis, some polysaccharides have been used in medical and biochemical areas as reviewed by different groups (137, 167–169). Notably, carbohydrates are found to exert their function via activating PPAR signaling pathways (Figure 7).
Figure 7. Mechanisms of action of carbohydrates in atherosclerosis therapy. Carbohydrates prevent against lipid accumulation by enhancing PPARα/PPARγ–liver X receptor (LXR)–ABC transporter and PPARα–mediated fatty acid β-oxidation. Alternatively, some polysaccharides alleviate lipid accumulation by suppressing fatty acid synthesis-related genes including PPARγ, fatty acid synthase, and sterol response element-binding protein (SREBP)-1c potentially through up-regulation of AMP-activated protein kinase (AMPK) in the liver. Furthermore, they inhibit phosphoinositide-3 kinase (PI3K)/protein kinase B (AKT/PKB)/mammalian target of rapamycin (mTOR) and mitogen-activated protein kinase (MAPK)/nuclear factor kappa B (NK-κB) signaling pathways to suppress inflammation. In the small intestine, carbohydrates decrease cholesterol absorption and increase cholesterol excretion by decreasing the level of Niemann-Pick C1-like 1 protein and enhancing the LXR/ABCG5/8 signaling pathway, respectively. Moreover, carbohydrates suppress inflammation by inhibiting Toll-like receptor (TLR)/myeloid differentiation factor 88 (MyD88)/NF-κB and modulating PPARγ/NK-κB signaling pathway.
Different groups have demonstrated that brown seaweed fucoidans attenuate hyperlipidemia and atherosclerosis by modulating PPARs in different animal models (170). For instance, Kjellmaniella crassifolia-derived fucoidan ameliorates hyperlipidemia by improving PPARα-mediated fatty acid β-oxidation in Wistar rats (171). Similarly, Saccharina sculpera-derived fucoidans improve hyperlipidemia potentially by enhancing the gene expression of PPARα and PPARγ in Wistar rats (172). Cladosiphon okamuranus-derived fucoidan improves hyperlipidemia and atherosclerosis partially by elevating the expression of PPARα and inhibiting SREBP-1 (173). Except for PPARα, PPARγ activation also stimulates LXR/ABC transporter signaling pathways, thereby accelerating lipid transport and excretion (14). However, Ascophyllum nodosum-derived fucoidan is found to inhibit the expression of PPARγ and elevate the expression of PPARα, thereby attenuating hyperlipidemia and atherosclerosis in ApoE-deficient mice (174).
Besides brown seaweeds, sea cucumber-derived polysaccharides improve lipid metabolism in different models (137). For instance, Isostihopus badionotus-derived fucosylated chondroitin sulfate (4,300 Da) exhibits a hypolipidemic effect in mice partially by down-regulating the expression of FAS and PPARγ (175). Acaudina molpadioides-derived fucoidan inhibits adipocyte proliferation and differentiation via enhancing Wnt/β-Catenin signaling pathway and suppressing the expression of SREBP-1c and PPARγ (176, 177). Glycosaminoglycans isolated from sea cucumber Holothuria leucospilota are found to ameliorate hyperlipidemia in male BALB/c mice by improving the expression of PPARα and ameliorating gut microbiota (137, 178).
Polysaccharides isolated from plants and fungi also exhibit powerful lipid-lowering effects as reviewed recently by distinct groups (179, 180). Cyclocarya paliurus-, Saussurea involucrata-, Astragalus membranaceus-, and Cordyceps militaris-derived polysaccharides exert therapeutic effects in hyperlipidemic rats partially via upregulating PPARα/CPT signaling pathway (181–184). A water-soluble polysaccharide from Morchella esculenta alleviates obesity and liver injury mainly by restoring Firmicutes/Bacteroidetes ratio and increasing SCFA production. However, it decreases hepatic gene expression including PPARα and PPARγ (185). Similarly, Liriope spicata var. prolifera- and Platycodon grandiflorus-derived polysaccharides exhibit strong lipid-lowering and hepatoprotective effects potentially by downregulating the expressions of PPARγ in vivo (186). Interestingly, P. grandiflorus-derived polysaccharides may control PPAR signaling by increasing the production of SCFAs including acetate, propionate, and butyrate in the gut through upregulation of SCFAs-producing gut bacteria (187). Similarly, Pueraria lobata- and Pueraria thomsonii-derived polysaccharides show therapeutic effects in type 2 diabetes mellitus through regulation of PPAR signaling pathway. Mechanistically, P. lobata-derived polysaccharides increase the abundance of Romboutsia bacteria to reduce serum concentration of taurocholic acid, thereby regulating the PPAR signaling pathway, such as inhibiting PPARγ. P. thomsonii-derived polysaccharides reduce the abundance of Klebsiella bacteria to decrease the serum levels of uric acid, thereby regulating PPAR signaling pathway to exert a therapeutic effect on insulin resistance (188). Lycium barbarum polysaccharide and Astragalus polysaccharide ameliorate lipid disorders by decreasing the gene expression of PPARγ, CD36, and FAS, and ameliorating gut microbiota (189, 190). Moreover, C. militaris-derived polysaccharide CM3-SII is demonstrated to inhibit the level of Niemann-Pick C1-like 1 protein, suggesting this polysaccharide may decrease cholesterol absorption (184).
Except for polysaccharide, monosaccharide and oligosaccharide have been demonstrated to modulate lipid metabolism by targeting PPARs. For instance, D-psicose regulates lipid metabolism via stimulating AMPK2α/PPARα signaling in rats (191). D-mannose promotes fatty acid oxidation via enhancing PPARα (192). Our group demonstrates that N-acetylneuraminic acid reduces TC and particularly TG partially by enhancing PPARα in ApoE-deficient mice (193, 194). Aging enhances the expression of SREBP-1c and decreases the expression of PPARα. Interestingly, oral intake of trehalose reverses these changes in aged liver, suggesting trehalose decreases lipogenesis and boosts fatty acid β-oxidation (195). Fructose is considered as a lipogenic nutrient. It suppresses transcriptional activity of PPARα and its target gene CPT-1α, potentially via modulating PGC-1α acetylation and CPT-1α acetylation (196).
In a comparative study, fucoidans obtained from Undaria pinnatifida, F. vesiculosus, Macrocystis pyrifera, A. nodosum, and Laminaria japonica reduce production of pro-inflammatory cytokines in a dose-dependent manner in LPS-induced cells (197). Mechanistically, fucoidans suppress MAPK/NF-κB, Janus kinase/signal transducer and activator of transcription-1/3, and TLR/MyD88/NF-κB signaling pathways (198). Furthermore, sea cucumber Apostichopus japonicus-derived fucoidan decreases LPS-induced inflammation by suppressing phosphorylation of p38-MAPK and the downstream NF-κB and AKT/mTOR pathway (176). Moreover, Sargassum horneri-derived fucoidan is found to suppress inflammation by inhibiting phosphorylation of p38-MAPK, c-Jun amino-terminal kinases (JNK), and extracellular signal-regulated kinase (ERK) (199). As PPAR activation modulates inflammation-related signaling pathway (14), fucoidans may suppress inflammation partially by regulating the expression of PPARs as mentioned above.
Notably, L. japonica-derived fucoidan decreases intestinal inflammation potentially by upregulating PPARα and improving gut microbiota (200), S. involucrata polysaccharide alleviates ultraviolet radiation-induced inflammatory responses by activating PPARα (183). Furthermore, L. barbarum polysaccharide inhibits LPS-induced inflammation by upregulating PPARγ and suppressing phosphorylation of p38-MAPK, JNK, and ERK, suggesting this polysaccharide alleviating inflammatory reactions through modulation of PPARγ/MAPK/NF-κB signaling pathway (201). Similarly, Moringa oleifera leaf polysaccharide ameliorates DSS-induced colitis by enhancing PPARγ and decreasing TLR/MyD88/NF-κB signaling pathway (202). Interestingly, polysaccharides may suppress hyperlipidemia-induced inflammation by decreasing PPARγ in hyperlipidemic animals. For instance, Tibetan burnip polysaccharide reduces the expression of ICAM-1, VCAM-1, IL-6, IL-1β, and TNF-α partially by downregulating PPARγ in hyperlipidemic rats (203). Moreover, intake of low dose sucrose (7.5 mg/ml) is found to activate PPARγ via restoring microbial dysfunction and upregulating SCFAs levels, thereby suppressing MAPK/NF-κB signaling pathway, while high dose sucrose (30 mg/ml) exacerbates DSS-induced colitis (204). Additionally, Astragalus polysaccharide inhibits protein kinase A/p38 MAPK signaling pathway and the expression of PPARγ and PGC-1α, suppressing inflammation in heart failure rats (190). These data suggest that polysaccharides may control inflammatory response by differently regulating the expression of PPARγ based on the actual situation. Given polysaccharides with big molecular weight and great hydrophilic property are hard to be absorbed, their microbiota-derived metabolites including SCFAs may play a key role in regulation of PPARs and atherosclerotic therapy.
TCMs, especially TCM prescriptions, and natural compounds including flavonoids, acids, alkaloids, terpenoids, phenolic compounds, and carbohydrates are effective in suppression of dyslipidemia and inflammatory responses with good safety by targeting PPARs, thereby retarding the progression of atherosclerosis. Notably, these natural molecules exhibit equivalent effects compared to chemically synthetic compounds but the former exhibit less harmful side effects (15). Furthermore, TCMs have been used for atherosclerosis therapy for hundreds of years in Asia, especially in China. Importantly, several natural compounds, such as anthocyanins, resveratrol, hesperidin, quercetin, epicatechin, and genistein, have been promoted to clinical trials (71). In this study, we also listed some clinical trials as shown in Table 1. Collectively, natural compounds are useful for atherosclerosis therapy by regulation of PPARs.
However, the research in this field has several limitations. First, although prescription/formula is a characteristic of TCM, it is necessary to clarify the key active ingredients and their mechanisms of action to enable TCM to enter the international market. In this aspect, artemisinin is a very good example. Secondly, seldom natural compounds have been applied in clinic. It seems that researchers are impelled to explore modified natural compounds to improve their novelty, bioavailability, and commercial value of interested molecules. These chemical modifications are sure to induce further environmental pollution. Therefore, researchers need to balance the beneficial and harmful aspects during drug discovery. Thirdly, as the distribution and action of PPARs show tissue-specificity, it is interesting to investigate the combined effects of interested compounds based on their pharmacokinetic characteristics and tissue distribution. Fourth, both activation and inactivation of PPARβ and particularly PPARγ may achieve similar therapeutic effects, suggesting some complex regulatory mechanisms are involved in PPARs' therapy of atherosclerosis. For instance, PPARγ activation is demonstrated to suppress inflammation via inhibiting NF-κB signaling pathway and decrease lipid accumulation via enhancing RCT through upregulation of LXRs–ABCA1/G1 signaling pathways; while PPARγ inactivation is indicated to decrease lipogenesis and CD36-mediated lipid uptake, thereby suppressing lipid accumulation and hyperlipidemia-induced inflammation. To elucidate the detailed mechanisms of action of an interested compound, it is necessary to investigated the above-mentioned mechanisms in one study in the future. Last but not least, rodents have distinct lipid profiles and lifestyles compared to our human, it is necessary to explore humanized models for drug screening in future to improve the potential translation of interested compounds.
YZ: Formal Analysis, Writing – original draft. XZ: Formal Analysis, Investigation, Software, Writing – original draft. SS: Formal Analysis, Software, Writing – original draft. CM: Formal Analysis, Investigation, Software, Writing – original draft. YL: Investigation, Writing – original draft. WS: Funding acquisition, Project administration, Supervision, Writing – review & editing. SG: Funding acquisition, Project administration, Writing – review & editing.
The author(s) declare financial support was received for the research, authorship, and/or publication of this article.
This work is supported by the National Natural Science Foundation of China (82070469, 81770463, 82271803).
The authors declare that the research was conducted in the absence of any commercial or financial relationships that could be construed as a potential conflict of interest.
All claims expressed in this article are solely those of the authors and do not necessarily represent those of their affiliated organizations, or those of the publisher, the editors and the reviewers. Any product that may be evaluated in this article, or claim that may be made by its manufacturer, is not guaranteed or endorsed by the publisher.
ABC, ATP-binding cassette; ACOX1, acyl-CoA oxidase 1; AMPK, AMP-activated protein kinase; AP-1, activator protein 1; Apo, apolipoprotein; ATGL, adipose triglyceride lipase; CPT, carnitine palmitoyl transferase; CRP, C-reactive protein; CVD, cardiovascular disease; DSS, dextran sulfate sodium; FABP, fatty acid binding protein; FAS, fatty acid synthase; HDL, high-density lipoprotein; HDL-c, high-density lipoprotein cholesterol; ICAM, intercellular cell adhesion molecule; IKK, inhibitor of κB kinases; IL, interleukin; LDL-c, low-density lipoprotein cholesterol; LXR, liver X receptor; NAFLD, nonalcoholic fatty liver disease; NF-κB, nuclear factor kappa B; PGC, peroxisome proliferator-activated receptor gamma coactivator; PI3K, Phosphoinositide 3-kinase; PPAR, peroxisome proliferator-activated receptor; RXR, retinoid X receptor; SREBP, sterol response element-binding protein; STAT, signal transducer and activator of transcription; TC, total cholesterol; TCM, traditional Chinese medicine; TG, triglyceride; TLR, toll-like receptor; TNF-α, tumor necrosis factor-alpha.
1. Chinwong S, Doungsong K, Channaina P, Phrommintikul A, Chinwong D. Association between medication adherence and cardiovascular outcomes among acute coronary syndrome patients. Res Social Adm Pharm. (2021) 17(9):1631–5. doi: 10.1016/j.sapharm.2021.01.003
2. Huang H, Sun Z, Xu J, Wang L, Zhao J, Li J, et al. Yang-Xin-Shu-Mai granule alleviates atherosclerosis by regulating macrophage polarization via the TLR9/MyD88/NF-κB signaling pathway. J Ethnopharmacol. (2024) 318(Pt A):116868. doi: 10.1016/j.jep.2023.116868
3. Kim JM, Lee WS, Kim J. Therapeutic strategy for atherosclerosis based on bone-vascular axis hypothesis. Pharmacol Ther. (2020) 206:107436. doi: 10.1016/j.pharmthera.2019.107436
4. Song P, Fang Z, Wang H, Cai Y, Rahimi K, Zhu Y, et al. Global and regional prevalence, burden, and risk factors for carotid atherosclerosis: a systematic review, meta-analysis, and modelling study. Lancet Glob Health. (2020) 8(5):e721–9. doi: 10.1016/s2214-109x(20)30117-0
5. Vilaplana-Carnerero C, Giner-Soriano M, Dominguez À, Morros R, Pericas C, Álamo-Junquera D, et al. Atherosclerosis, cardiovascular disease, and COVID-19: a narrative review. Biomedicines. (2023) 11(4):1206. doi: 10.3390/biomedicines11041206
6. Lin P, Ji HH, Li YJ, Guo SD. Macrophage plasticity and atherosclerosis therapy. Front Mol Biosci. (2021) 8:679797. doi: 10.3389/fmolb.2021.679797
7. Yoon W, Kim EJ, Park Y, Kim S, Park YK, Yoo Y. Bacterially delivered miRNA-mediated toll-like receptor 8 gene silencing for combined therapy in a murine model of atopic dermatitis: therapeutic effect of mirtlr8 in AD. Microorganisms. (2021) 9(8):1715. doi: 10.3390/microorganisms9081715
8. Piconi S, Parisotto S, Rizzardini G, Passerini S, Meraviglia P, Schiavini M, et al. Atherosclerosis is associated with multiple pathogenic mechanisms in HIV-infected antiretroviral-naive or treated individuals. Aids. (2013) 27(3):381–9. doi: 10.1097/QAD.0b013e32835abcc9
9. Zhang B, Hao Z, Zhou W, Zhang S, Sun M, Li H, et al. Formononetin protects against ox-LDL-induced endothelial dysfunction by activating PPAR-γ signaling based on network pharmacology and experimental validation. Bioengineered. (2021) 12(1):4887–98. doi: 10.1080/21655979.2021.1959493
10. Qiao YN, Zou YL, Guo SD. Low-density lipoprotein particles in atherosclerosis. Front Physiol. (2022) 13:931931. doi: 10.3389/fphys.2022.931931
11. Sun J, Yu L, Qu X, Huang T. The role of peroxisome proliferator-activated receptors in the tumor microenvironment, tumor cell metabolism, and anticancer therapy. Front Pharmacol. (2023) 14:1184794. doi: 10.3389/fphar.2023.1184794
12. Gross B, Pawlak M, Lefebvre P, Staels B. PPARs in obesity-induced T2DM, dyslipidaemia and NAFLD. Nat Rev Endocrinol. (2017) 13(1):36–49. doi: 10.1038/nrendo.2016.135
13. Corrales P, Vidal-Puig A, Medina-Gómez G. PPARs and metabolic disorders associated with challenged adipose tissue plasticity. Int J Mol Sci. (2018) 19(7):2124. doi: 10.3390/ijms19072124
14. Miao M, Wang X, Liu T, Li YJ, Yu WQ, Yang TM, et al. Targeting PPARs for therapy of atherosclerosis: a review. Int J Biol Macromol. (2023) 242(2):125008. doi: 10.1016/j.ijbiomac.2023.125008
15. Zou H, Gong Y, Ye H, Yuan C, Li T, Zhang J, et al. Dietary regulation of peroxisome proliferator-activated receptors in metabolic syndrome. Phytomedicine. (2023) 116:154904. doi: 10.1016/j.phymed.2023.154904
16. Hong F, Pan S, Guo Y, Xu P, Zhai Y. PPARs as nuclear receptors for nutrient and energy metabolism. Molecules. (2019) 24(14):2545. doi: 10.3390/molecules24142545
17. Yang X, Wang J, Chang C-Y, Zhou F, Liu J, Xu H, et al. Leukemia inhibitory factor suppresses hepatic de novo lipogenesis and induces cachexia in mice. Nat Commun. (2024) 15(1):627. doi: 10.1038/s41467-024-44924-w
18. Koga T, Peters JM. Targeting peroxisome proliferator-activated receptor-β/δ (PPARβ/δ) for the treatment or prevention of alcoholic liver disease. Biol Pharm Bull. (2021) 44(11):1598–606. doi: 10.1248/bpb.b21-00486
19. Mirza AZ, Althagafi II, Shamshad H. Role of PPAR receptor in different diseases and their ligands: physiological importance and clinical implications. Eur J Med Chem. (2019) 166:502–13. doi: 10.1016/j.ejmech.2019.01.067
20. Cheang WS, Tian XY, Wong WT, Huang Y. The peroxisome proliferator-activated receptors in cardiovascular diseases: experimental benefits and clinical challenges. Br J Pharmacol. (2015) 172(23):5512–22. doi: 10.1111/bph.13029
21. Neve BP, Corseaux D, Chinetti G, Zawadzki C, Fruchart JC, Duriez P, et al. PPARalpha agonists inhibit tissue factor expression in human monocytes and macrophages. Circulation. (2001) 103(2):207–12. doi: 10.1161/01.cir.103.2.207
22. Delerive P, Gervois P, Fruchart JC, Staels B. Induction of IkappaBalpha expression as a mechanism contributing to the anti-inflammatory activities of peroxisome proliferator-activated receptor-alpha activators. J Biol Chem. (2000) 275(47):36703–7. doi: 10.1074/jbc.M004045200
23. Chinetti-Gbaguidi G, Staels B. PPARβ in macrophages and atherosclerosis. Biochimie. (2017) 136:59–64. doi: 10.1016/j.biochi.2016.12.008
24. Venkataraman B, Ojha S, Belur PD, Bhongade B, Raj V, Collin PD, et al. Phytochemical drug candidates for the modulation of peroxisome proliferator-activated receptor γ in inflammatory bowel diseases. Phytother Res. (2020) 34(7):1530–49. doi: 10.1002/ptr.6625
25. Enayati A, Ghojoghnejad M, Roufogalis BD, Maollem SA, Sahebkar A. Impact of phytochemicals on PPAR receptors: implications for disease treatments. PPAR Res. (2022) 2022:4714914. doi: 10.1155/2022/4714914
26. Villarroel-Vicente C, Gutiérrez-Palomo S, Ferri J, Cortes D, Cabedo N. Natural products and analogs as preventive agents for metabolic syndrome via peroxisome proliferator-activated receptors: an overview. Eur J Med Chem. (2021) 221:113535. doi: 10.1016/j.ejmech.2021.113535
27. Pan J, Zhou W, Xu R, Xing L, Ji G, Dang Y. Natural PPARs agonists for the treatment of nonalcoholic fatty liver disease. Biomed Pharmacother. (2022) 151:113127. doi: 10.1016/j.biopha.2022.113127
28. Li H, Yu XH, Ou X, Ouyang XP, Tang CK. Hepatic cholesterol transport and its role in non-alcoholic fatty liver disease and atherosclerosis. Prog Lipid Res. (2021) 83:101109. doi: 10.1016/j.plipres.2021.101109
29. Beckman JA, Creager MA, Libby P. Diabetes and atherosclerosis: epidemiology, pathophysiology, and management. JAMA. (2002) 287(19):2570–81. doi: 10.1001/jama.287.19.2570
30. Liu L, Shi Z, Ji X, Zhang W, Luan J, Zahr T, et al. Adipokines, adiposity, and atherosclerosis. Cell. Mol Life Sci. (2022) 79(5):272. doi: 10.1007/s00018-022-04286-2
31. Abdallah LR, de Matos RC, e Souza YPDM, Vieira-Soares D, Muller-Machado G, Pollo-Flores P. Non-alcoholic fatty liver disease and its links with inflammation and atherosclerosis. Curr Atheroscler Rep. (2020) 22(1):7. doi: 10.1007/s11883-020-0820-8
32. Singh S, Torzewski M. Fibroblasts and their pathological functions in the fibrosis of aortic valve sclerosis and atherosclerosis. Biomolecules. (2019) 9(9):472. doi: 10.3390/biom9090472
33. Ren L, Zhang J, Zhang T. Immunomodulatory activities of polysaccharides from Ganoderma on immune effector cells. Food Chem. (2021) 340:127933. doi: 10.1016/j.foodchem.2020.127933
34. Cheng B, Zhou AZ, Ge W, Yao XM, Wang J. Mechanism of Huo-Xue-Qu-Yu formula in treating nonalcoholic hepatic steatosis by regulating lipid metabolism and oxidative stress in rats. eCAM. (2021) 29:6026319. doi: 10.1155/2021/6026319
35. Lu L, Qin Y, Chen C, Zhang X, Xu X, Lv C, et al. The atheroprotective roles of heart-protecting musk pills against atherosclerosis development in apolipoprotein E-deficient mice. Ann Transl Med. (2019) 7(23):714. doi: 10.21037/atm.2019.12.22
36. Deng H, Wu DB, Guo MJ, Sun C, Lu B, Yang L, et al. Ethanol extracts of Danlou tablet attenuate atherosclerosis via inhibiting inflammation and promoting lipid effluent. Pharmacol Res. (2019) 146:104306. doi: 10.1016/j.phrs.2019.104306
37. Wang M, Xiang Q, Sun W, Zhang H, Shi R, Guo J, et al. Qihuang Zhuyu formula attenuates atherosclerosis via targeting PPARγ to regulate cholesterol efflux and endothelial cell inflammation. Oxid Med Cell Longev. (2022) 30:2226168. doi: 10.1155/2022/2226168
38. Li Y, Zhang L, Ren P, Yang Y, Li S, Qin X, et al. Qing-Xue-Xiao-Zhi formula attenuates atherosclerosis by inhibiting macrophage lipid accumulation and inflammatory response via TLR4/MyD88/NF-κB pathway regulation. Phytomedicine. (2021) 93:153812. doi: 10.1016/j.phymed.2021.153812
39. Ju S, Chang X, Wang J, Zou X, Zhao Z, Huang Z, et al. Sini decoction intervention on atherosclerosis via PPARγ-LXRα-ABCA1 pathway in rabbits. Open Life Sci. (2018) 13:446–55. doi: 10.1515/biol-2018-0053
40. Xie J, Peng L, Wang T, Yang C, Chen N, Feng X, et al. Qishenyiqi pill inhibits atherosclerosis by promoting reverse cholesterol transport PPARγ-LXRα/β-ABCA1 pathway. J Ethnopharmacol. (2023) 315:116684. doi: 10.1016/j.jep.2023.116684
41. Zheng S, Huang H, Li Y, Wang Y, Zheng Y, Liang J, et al. Yin-xing-tong-mai decoction attenuates atherosclerosis via activating PPARγ-LXRα-ABCA1/ABCG1 pathway. Pharmacol Res. (2021) 169:105639. doi: 10.1016/j.phrs.2021.105639
42. Liu P, Zhou X, Zhang H, Wang R, Wu X, Jian W, et al. Danggui-Shaoyao-San attenuates cognitive impairment via the microbiota-gut-brain axis with regulation of lipid metabolism in scopolamine-induced amnesia. Front Immunol. (2022) 13:796542. doi: 10.3389/fimmu.2022.796542
43. Ren Q, Liu XQ, Zhou XW, Zhou X, Fang G, Wang B, et al. Effects of Huatan Jiangzhuo decoction on diet-induced hyperlipidemia and gene expressions in rats. Chin J Nat Med. (2021) 19(2):100–11. doi: 10.1016/S1875-5364(21)60011-0
44. Han Y, Wang J, Jin M, Jia L, Yan C, Wang Y. Shentong Zhuyu decoction inhibits inflammatory response, migration, and invasion and promotes apoptosis of rheumatoid arthritis fibroblast-like synoviocytes via the MAPK p38/PPARγ/CTGF pathway. Biomed Res Int. (2021) 13:6187695. doi: 10.1155/2021/6187695
45. Zhang Z, Zhai L, Lu J, Sun S, Wang D, Zhao D, et al. Shen-Hong-Tong-Luo formula attenuates macrophage inflammation and lipid accumulation through the activation of the PPAR-γ/LXR-α/ABCA1 pathway. Oxid Med Cell Longev. (2020) 19:3426925. doi: 10.1155/2020/3426925
46. Chen Z, Liu F, Zheng N, Guo M, Bao L, Zhan Y, et al. Wuzhi capsule (schisandra sphenanthera extract) attenuates liver steatosis and inflammation during non-alcoholic fatty liver disease development. Biomed Pharmacother. (2019) 110:285–93. doi: 10.1016/j.biopha.2018.11.069
47. Lei W, Li X, Li L, Huang M, Cao Y, Sun X, et al. Compound danshen dripping pill ameliorates post ischemic myocardial inflammation through synergistically regulating MAPK, PI3K/AKT and PPAR signaling pathway. J. Ethnopharmacol. (2021) 281:114438. doi: 10.1016/j.jep.2021.114438
48. Zhang L, Li Y, Ma X, Liu J, Wang X, Zhang L, et al. Ginsenoside Rg1-notoginsenoside R1-protocatechuic aldehyde reduces atherosclerosis and attenuates low-shear stress-induced vascular endothelial cell dysfunction. Front Pharmacol. (2021) 11:588259. doi: 10.3389/fphar.2020.588259
49. Xie Y, Tian L, Fang Z, Zhong A, Ao Z, Xu S, et al. Bushen Kangshuai tablet inhibits progression of atherosclerosis by intervening in macrophage autophagy and polarization. J Tradit Chin Med. (2020) 40(1):28–37. doi: 10.19852/j.cnki.jtcm.2020.01.003
50. Ma L, Dai X, Wu C, Li M, Sheng H, Mao W. Tanyu Tongzhi formula delays atherosclerotic plaque progression by promoting alternative macrophage activation via PPARγ and AKT/ERK signal pathway in ApoE knock-out mice. Front Pharmacol. (2021) 12:734589. doi: 10.3389/fphar.2021.734589
51. Akter R, Ling L, Rupa EJ, KyuPark J, Mathiyalagan R, Nahar J, et al. Binary effects of gynostemma gold nanoparticles on obesity and inflammation via downregulation of PPARγ/CEPBα and TNF-α gene expression. Molecules. (2022) 27(9):2795. doi: 10.3390/molecules27092795
52. Rakha A, Umar N, Rabail R, Butt MS, Kieliszek M, Hassoun A, et al. Anti-inflammatory and anti-allergic potential of dietary flavonoids: a review. Biomed Pharmacother. (2022) 156:113945. doi: 10.1016/j.biopha.2022.113945
53. Song X, Tan L, Wang M, Ren C, Guo C, Yang B, et al. Myricetin: a review of the most recent research. Biomed Pharmacother. (2021) 134:111017. doi: 10.1016/j.biopha.2020.111017
54. Aslan MN, Sukan-Karaçağıl B, Acar-Tek N. Roles of citrus fruits on energy expenditure, body weight management, and metabolic biomarkers: a comprehensive review. Nutr Rev. (2023):1–16. doi: 10.1093/nutrit/nuad116
55. Tsuboi T, Lu R, Yonezawa T, Watanabe A, Woo JT, Abe-Dohmae S, et al. Molecular mechanism for nobiletin to enhance ABCA1/G1 expression in mouse macrophages. Atherosclerosis. (2020) 297:32–9. doi: 10.1016/j.atherosclerosis.2020.01.024
56. Baiyisaiti A, Wang Y, Zhang X, Chen W, Qi R. Rosa rugosa flavonoids exhibited PPARα agonist-like effects on genetic severe hypertriglyceridemia of mice. J Ethnopharmacol. (2019) 240:111952. doi: 10.1016/j.jep.2019.111952
57. Shen CY, Hao YF, Hao ZX, Liu Q, Zhang L, Jiang CP, et al. Flavonoids from Rosa davurica pall. Fruits prevent high-fat diet-induced obesity and liver injury via modulation of the gut microbiota in mice. Food Funct. (2021) 12(20):10097–106. doi: 10.1039/d1fo01373d
58. Qin H, Song Z, Shaukat H, Zheng W. Genistein regulates lipid metabolism via estrogen receptor β and its downstream signal Akt/mTOR in HepG2 cells. Nutrients. (2021) 13(11):4015. doi: 10.3390/nu13114015
59. Xiong H, Wang J, Ran Q, Lou G, Peng C, Gan Q, et al. Hesperidin: a therapeutic agent for obesity. Drug Des Devel Ther. (2019) 13:3855–66. doi: 10.2147/DDDT.S227499
60. Liu J, Zhang W, Li Y, Li X, Li Y, Guo F. Flavonoids extract from the seeds of Psoralea corylifolia L. (PFE) alleviates atherosclerosis in high-fat diet-induced LDLR(-/-) mice. Phytomedicine. (2022) 98:153983. doi: 10.1016/j.phymed.2022.153983
61. Ren K, Li H, Zhou HF, Liang Y, Tong M, Chen L, et al. Mangiferin promotes macrophage cholesterol efflux and protects against atherosclerosis by augmenting the expression of ABCA1 and ABCG1. Aging (Albany NY. (2019) 11(23):10992–1009. doi: 10.18632/aging.102498
62. Ren K, Jiang T, Zhao GJ. Quercetin induces the selective uptake of HDL-cholesterol via promoting SR-BI expression and the activation of the PPARγ/LXRα pathway. Food Funct. (2018) 9(1):624–35. doi: 10.1039/c7fo01107e
63. Jia Q, Cao H, Shen D, Li S, Yan L, Chen C, et al. Quercetin protects against atherosclerosis by regulating the expression of PCSK9, CD36, PPARγ, LXRα and ABCA1. Int J Mol Med. (2019) 44(3):893–902. doi: 10.3892/ijmm.2019.4263
64. Zhao ZW, Zhang M, Wang G, Zou J, Gao JH, Zhou L, et al. Astragalin retards atherosclerosis by promoting cholesterol efflux and inhibiting the inflammatory response via upregulating ABCA1 and ABCG1 expression in macrophages. J Cardiovasc Pharmacol. (2021) 77(2):217–27. doi: 10.1097/fjc.0000000000000944
65. Yu XH, Chen JJ, Deng WY, Xu XD, Liu QX, Shi MW, et al. Biochanin a mitigates atherosclerosis by inhibiting lipid accumulation and inflammatory response. Oxid Med Cell Longev. (2020) 2020:8965047. doi: 10.1155/2020/8965047
66. Cui Y, Hou P, Li F, Liu Q, Qin S, Zhou G, et al. Quercetin improves macrophage reverse cholesterol transport in apolipoprotein E-deficient mice fed a high-fat diet. Lipids Health Dis. (2017) 16(1):9. doi: 10.1186/s12944-016-0393-2
67. Baradaran Rahimi V, Askari VR, Hosseinzadeh H. Promising influences of Scutellaria baicalensis and its two active constituents, baicalin, and baicalein, against metabolic syndrome: a review. Phytother Res. (2021) 35(7):3558–74. doi: 10.1002/ptr.7046
68. Zhang ZZ, Yu XH, Tan WH. Baicalein inhibits macrophage lipid accumulation and inflammatory response by activating the PPARγ/LXRα pathway. Clin Exp Immunol. (2022) 209(3):316–25. doi: 10.1093/cei/uxac062
69. Shen CY, Lin JJ, Jiang JG, Wang TX, Zhu W. Potential roles of dietary flavonoids from Citrus aurantium L. Var. amara engl. In atherosclerosis development. Food Funct. (2020) 11(1):561–71. doi: 10.1039/c9fo02336d
70. Zhuang JL, Liu YY, Li ZZ, Zhuang QZ, Tang WZ, Xiong Y, et al. Amentoflavone prevents ox-LDL-induced lipid accumulation by suppressing the PPARγ/CD36 signal pathway. Toxicol Appl Pharmacol. (2021) 431:115733. doi: 10.1016/j.taap.2021.115733
71. Gouveia H, Urquiza-Martínez MV, Manhães-de-Castro R, Costa-de-Santana BJR, Villarreal JP, Mercado-Camargo R, et al. Effects of the treatment with flavonoids on metabolic syndrome components in humans: a systematic review focusing on mechanisms of action. Int J Mol Sci. (2022) 23(15):8344. doi: 10.3390/ijms23158344
72. Yi L, Cui J, Wang W, Tang W, Teng F, Zhu X, et al. Formononetin attenuates airway inflammation and oxidative stress in murine allergic asthma. Front Pharmacol. (2020) 11:533841. doi: 10.3389/fphar.2020.533841
73. Wang Q, Chen R, Tang Q, Chen JY, Wang Y, Sui DJ, et al. Flavonoid extract from propolis provides cardioprotection following myocardial infarction by activating PPAR-γ. Evid Based Complement Alternat Med. (2022) 2022:1333545. doi: 10.1155/2022/1333545
74. Feng P, Xu Y, Tong B, Tong X, Bian Y, Zhao S, et al. Saikosaponin a attenuates hyperlipidemic pancreatitis in rats via the PPAR-γ/NF-κB signaling pathway. Exp Ther Med. (2020) 19(2):1203–12. doi: 10.3892/etm.2019.8324
75. Aboonabi A, Aboonabi A. Anthocyanins reduce inflammation and improve glucose and lipid metabolism associated with inhibiting nuclear factor-kappaB activation and increasing PPAR-γ gene expression in metabolic syndrome subjects. Free Radic Biol Med. (2020) 150:30–9. doi: 10.1016/j.freeradbiomed.2020.02.004
76. Xu L, Liu JT, Li K, Wang SY, Xu S. Genistein inhibits Ang II-induced CRP and MMP-9 generations via the ER-p38/ERK1/2-PPARγ-NF-κB signaling pathway in rat vascular smooth muscle cells. Life Sci. (2019) 216:140–6. doi: 10.1016/j.lfs.2018.11.036
77. Marino M, Del Bo C, Tucci M, Venturi S, Mantegazza G, Taverniti V, et al. A mix of chlorogenic and caffeic acid reduces C/EBPβ and PPAR-γ1 levels and counteracts lipid accumulation in macrophages. Eur J Nutr. (2022) 61(2):1003–14. doi: 10.1007/s00394-021-02714-w
78. Kumar R, Sharma A, Iqbal MS, Srivastava JK. Therapeutic promises of chlorogenic acid with special emphasis on its anti-obesity property. Curr Mol Pharmacol. (2020) 13(1):7–16. doi: 10.2174/1874467212666190716145210
79. Wang H, Yang Y, Sun X, Tian F, Guo S, Wang W, et al. Sonodynamic therapy-induced foam cells apoptosis activates the phagocytic PPARγ-LXRα-ABCA1/ABCG1 pathway and promotes cholesterol efflux in advanced plaque. Theranostics. (2018) 8(18):4969–84. doi: 10.7150/thno.26193
80. Varela LM, Ortega-Gomez A, Lopez S, Abia R, Muriana FJ, Bermudez B. The effects of dietary fatty acids on the postprandial triglyceride-rich lipoprotein/apoB48 receptor axis in human monocyte/macrophage cells. J Nutr Biochem. (2013) 24(12):2031–9. doi: 10.1016/j.jnutbio.2013.07.004
81. Kimura R, Takahashi N, Lin S, Goto T, Murota K, Nakata R, et al. DHA Attenuates postprandial hyperlipidemia via activating PPARα in intestinal epithelial cells. J Lipid Res. (2013) 54(12):3258–68. doi: 10.1194/jlr.M034942
82. Tian F, Wang J, Sun H, Yang J, Wang P, Wan S, et al. N-3 polyunsaturated fatty acids ameliorate hepatic steatosis via the PPAR-α/CPT-1α pathway in a mouse model of parenteral nutrition. Biochem Biophys Res Commun. (2018) 501(4):974–81. doi: 10.1016/j.bbrc.2018.05.095
83. Naeini Z, Toupchian O, Vatannejad A, Sotoudeh G, Teimouri M, Ghorbani M, et al. Effects of DHA-enriched fish oil on gene expression levels of p53 and NF-κB and PPAR-γ activity in PBMCs of patients with T2DM: a randomized, double-blind, clinical trial. Nutr Metab Cardiovasc Dis. (2020) 30(3):441–7. doi: 10.1016/j.numecd.2019.10.012
84. Hilgendorf KI, Johnson CT, Mezger A, Rice SL, Norris AM, Demeter J, et al. Omega-3 fatty acids activate ciliary FFAR4 to control adipogenesis. Cell. (2019) 179(6):1289–1305.e21. doi: 10.1016/j.cell.2019.11.005
85. Yamada H, Uemura A, Miyasaka R. 8(R)-Hydroxyeicosapentaenoic Acid (8R-HEPE) induces transcription of cholesterol efflux receptors via activation of liver X receptor in macrophages. Biosci Biotechnol Biochem. (2023) 87(6):584–91. doi: 10.1093/bbb/zbad025
86. Ishida N, Yamada H, Hirose M. Euphausia pacifica (north pacific krill): review of chemical features and potential benefits of 8-HEPE against metabolic syndrome, dyslipidemia, NAFLD, and atherosclerosis. Nutrients. (2021) 13(11):3765. doi: 10.3390/nu13113765
87. Saito M, Ishida N, Yamada H, Ibi M, Hirose M. 8-HEPE-Concentrated Materials from pacific krill improve plasma cholesterol levels and hepatic steatosis in high cholesterol diet-fed low-density lipoprotein (LDL) receptor-deficient mice. Biol Pharm Bull. (2020) 43(6):919–24. doi: 10.1248/bpb.b20-00162
88. Yamada H, Kikuchi S, Hakozaki M, Motodate K, Nagahora N, Hirose M. 8-Hydroxyeicosapentaenoic Acid decreases plasma and hepatic triglycerides via activation of peroxisome proliferator-activated receptor alpha in high-fat diet-induced obese mice. J Lipids. (2016) 2016:7498508. doi: 10.1155/2016/7498508
89. Nagatake T, Shibata Y, Morimoto S, Node E, Sawane K, Hirata SI, et al. 12-Hydroxyeicosapentaenoic Acid inhibits foam cell formation and ameliorates high-fat diet-induced pathology of atherosclerosis in mice. Sci Rep. (2021) 11(1):10426. doi: 10.1038/s41598-021-89707-1
90. Bouhlel MA, Derudas B, Rigamonti E, Dièvart R, Brozek J, Haulon S, et al. PPARgamma activation primes human monocytes into alternative M2 macrophages with anti-inflammatory properties. Cell Metab. (2007) 6(2):137–43. doi: 10.1016/j.cmet.2007.06.010
91. Yue C, Li M, Li J, Han X, Zhu H, Yu G, et al. Medium-, long- and medium-chain-type structured lipids ameliorate high-fat diet-induced atherosclerosis by regulating inflammation, adipogenesis, and gut microbiota in ApoE(-/-) mice. Food Funct. (2020) 11(6):5142–55. doi: 10.1039/d0fo01006e
92. Rankin L, Fowler CJ. The basal pharmacology of palmitoylethanolamide. Int J Mol Sci. (2020) 21(21):7942. doi: 10.3390/ijms21217942
93. Martinelli N, Girelli D, Malerba G, Guarini P, Illig T, Trabetti E, et al. FADS Genotypes and desaturase activity estimated by the ratio of arachidonic acid to linoleic acid are associated with inflammation and coronary artery disease. Am J Clin Nutr. (2008) 88(4):941–9. doi: 10.1093/ajcn/88.4.941
94. Lin S, Hirai S, Yamaguchi Y, Goto T, Takahashi N, Tani F, et al. Taurine improves obesity-induced inflammatory responses and modulates the unbalanced phenotype of adipose tissue macrophages. Mol Nutr Food Res. (2013) 57(12):2155–65. doi: 10.1002/mnfr.201300150
95. Feng S, Reuss L, Wang Y. Potential of natural products in the inhibition of adipogenesis through regulation of PPARγ expression and/or its transcriptional activity. Molecules. (2016) 21(10):1278. doi: 10.3390/molecules21101278
96. Mantovani A, Garlanda C, Locati M. Macrophage diversity and polarization in atherosclerosis: a question of balance. Arterioscler Thromb Vasc Biol. (2009) 29(10):1419–23. doi: 10.1161/atvbaha.108.180497
97. Sawada M, Yamamoto H, Ogasahara A, Tanaka Y, Kihara S. β-aminoisobutyric acid protects against vascular inflammation through PGC-1β-induced antioxidative properties. Biochem Biophys Res Commun. (2019) 516(3):963–8. doi: 10.1016/j.bbrc.2019.06.141
98. Zheng J, Xiao H, Duan Y, Song B, Zheng C, Guo Q, et al. Roles of amino acid derivatives in the regulation of obesity. Food Funct. (2021) 12(14):6214–25. doi: 10.1039/d1fo00780g
99. Vega OM, Abkenari S, Tong Z, Tedman A, Huerta-Yepez S. Omega-3 polyunsaturated fatty acids and lung cancer: nutrition or pharmacology? Nutr Cancer. (2021) 73(4):541–61. doi: 10.1080/01635581.2020.1761408
100. Ghasemi Darestani N, Bahrami A, Mozafarian MR, Esmalian Afyouni N, Akhavanfar R, Abouali R, et al. Association of polyunsaturated fatty acid intake on inflammatory gene expression and multiple sclerosis: a systematic review and meta-analysis. Nutrients. (2022) 14(21):4627. doi: 10.3390/nu14214627
101. Walker ME, Matthan NR, Goldbaum A, Meng H, Lamon-Fava S, Lakshman S, et al. Dietary patterns influence epicardial adipose tissue fatty acid composition and inflammatory gene expression in the Ossabaw pig. J Nutr Biochem. (2019) 70:138–46. doi: 10.1016/j.jnutbio.2019.04.013
102. Jung T, Hudson R, Rushlow W, Laviolette SR. Functional interactions between cannabinoids, omega-3 fatty acids, and peroxisome proliferator-activated receptors: implications for mental health pharmacotherapies. Eur J Neurosci. (2022) 55(4):1088–100. doi: 10.1111/ejn.15023
103. Chen KC, Chang SS, Huang HJ, Lin TL, Wu YJ, Chen CY. Three-in-one agonists for PPAR-α, PPAR-γ, and PPAR-δ from traditional Chinese medicine. J Biomol Struct Dyn. (2012) 30(6):662–83. doi: 10.1080/07391102.2012.689699
104. Song D, Hao J, Fan D. Biological properties and clinical applications of berberine. Front Med. (2020) 14(5):564–82. doi: 10.1007/s11684-019-0724-6
105. Man B, Hu C, Yang G, Xiang J, Yang S, Ma C. Berberine attenuates diabetic atherosclerosis via enhancing the interplay between KLF16 and PPARα in ApoE(-/-) mice. Biochem Biophys Res Commun. (2022) 624:59–67. doi: 10.1016/j.bbrc.2022.07.072
106. Xu Y, Yu T, Ma G, Zheng L, Jiang X, Yang F, et al. Berberine modulates deacetylation of PPARγ to promote adipose tissue remodeling and thermogenesis via AMPK/SIRT1 pathway. Int J Biol Sci. (2021) 17(12):3173–87. doi: 10.7150/ijbs.62556
107. Ke X, Zhang R, Li P, Zuo L, Wang M, Yang J, et al. Hydrochloride berberine ameliorates alcohol-induced liver injury by regulating inflammation and lipid metabolism. Biochem Biophys Res Commun. (2022) 610:49–55. doi: 10.1016/j.bbrc.2022.04.009
108. Yang S, Cao S, Li C, Zhang J, Liu C, Qiu F, et al. Berberrubine, a main metabolite of berberine, alleviates non-alcoholic fatty liver disease via modulating glucose and lipid metabolism and restoring gut microbiota. Front Pharmacol. (2022) 13:913378. doi: 10.3389/fphar.2022.913378
109. Wei D, Wu S, Liu J, Zhang X, Guan X, Gao L, et al. Theobromine ameliorates nonalcoholic fatty liver disease by regulating hepatic lipid metabolism via mTOR signaling pathway in vivo and in vitro. Can J Physiol Pharmacol. (2021) 99(8):775–85. doi: 10.1139/cjpp-2020-0259
110. Yang S, Hu T, Liu H, Lv YL, Zhang W, Li H, et al. Akebia saponin D ameliorates metabolic syndrome (MetS) via remodeling gut microbiota and attenuating intestinal barrier injury. Biomed Pharmacother. (2021) 138:111441. doi: 10.1016/j.biopha.2021.111441
111. Wang T, Lu H. Ganoderic acid A inhibits ox-LDL-induced THP-1-derived macrophage inflammation and lipid deposition via Notch1/PPARγ/CD36 signaling. Adv Clin Exp Med. (2021) 30(10):1031–41. doi: 10.17219/acem/137914
112. Zheng Z, Chen B, Wang K, Bao L, Wang Z, Xie L, et al. A concise total synthesis and PPAR activation activity of hericerin from Hericium erinaceum. J Antibiot (Tokyo). (2020) 73(9):646–9. doi: 10.1038/s41429-020-0303-8
113. Farias-Pereira R, Park CS, Park Y. Mechanisms of action of coffee bioactive components on lipid metabolism. Food Sci Biotechnol. (2019) 28(5):1287–96. doi: 10.1007/s10068-019-00662-0
114. Ávila DL, Nunes NAM, Almeida P, Gomes JAS, Rosa COB, Alvarez-Leite JI. Signaling targets related to antiobesity effects of capsaicin: a scoping review. Adv Nutr. (2021) 12(6):2232–43. doi: 10.1093/advances/nmab064
115. Wang C, Ma C, Gong L, Dai S, Li Y. Preventive and therapeutic role of betaine in liver disease: a review on molecular mechanisms. Eur J Pharmacol. (2021) 912:174604. doi: 10.1016/j.ejphar.2021.174604
116. Jin M, Shen Y, Pan T, Zhu T, Li X, Xu F, et al. Dietary betaine mitigates hepatic steatosis and inflammation induced by a high-fat-diet by modulating the Sirt1/Srebp-1/Pparα pathway in juvenile black seabream (Acanthopagrus schlegelii). Front Immunol. (2021) 12:694720. doi: 10.3389/fimmu.2021.694720
117. Zhou Y, Wang X, Guo L, Chen L, Zhang M, Chen X, et al. TRPV1 Activation inhibits phenotypic switching and oxidative stress in vascular smooth muscle cells by upregulating PPARα. Biochem Biophys Res Commun. (2021) 545:157–63. doi: 10.1016/j.bbrc.2021.01.072
118. Liu K, Gao X, Hu C, Gui Y, Gui S, Ni Q, et al. Capsaicin ameliorates diabetic retinopathy by inhibiting poldip2-induced oxidative stress. Redox Biol. (2022) 56:102460. doi: 10.1016/j.redox.2022.102460
119. Tang J, Luo K, Li Y, Chen Q, Tang D, Wang D, et al. Capsaicin attenuates LPS-induced inflammatory cytokine production by upregulation of LXRα. Int Immunopharmacol. (2015) 28(1):264–9. doi: 10.1016/j.intimp.2015.06.007
120. Chowdhury AA, Gawali NB, Munshi R, Juvekar AR. Trigonelline insulates against oxidative stress, proinflammatory cytokines and restores BDNF levels in lipopolysaccharide induced cognitive impairment in adult mice. Metab Brain Dis. (2018) 33(3):681–91. doi: 10.1007/s11011-017-0147-5
121. Ma ZG, Yuan YP, Zhang X, Xu SC, Wang SS, Tang QZ. Piperine attenuates pathological cardiac fibrosis via PPAR-γ/AKT pathways. EBioMedicine. (2017) 18:179–87. doi: 10.1016/j.ebiom.2017.03.021
122. Li R, Qin X, Yue L, Liu W, Gao Y, Zhu F, et al. Nuciferine improves cardiac function in mice subjected to myocardial ischemia/reperfusion injury by upregulating PPAR-γ. Heliyon. (2023) 9(2):e13630. doi: 10.1016/j.heliyon.2023.e13630
123. Kokabiyan Z, Yaghmaei P, Jameie SB, Hajebrahimi Z. Effect of eugenol on lipid profile, oxidative stress, sex hormone, liver injury, ovarian failure, and expression of COX-2 and PPAR-α genes in a rat model of diabetes. Mol Biol Rep. (2023) 50(4):3669–79. doi: 10.1007/s11033-022-08108-3
124. Yang Q, Shu F, Gong J, Ding P, Cheng R, Li J, et al. Sweroside ameliorates NAFLD in high-fat diet induced obese mice through the regulation of lipid metabolism and inflammatory response. J Ethnopharmacol. (2020) 255:112556. doi: 10.1016/j.jep.2020.112556
125. Gao Y, Zhang S, Li J, Zhao J, Xiao Q, Zhu Y, et al. Effect and mechanism of ginsenoside Rg1-regulating hepatic steatosis in HepG2 cells induced by free fatty acid. Biosci Biotechnol Biochem. (2020) 84(11):2228–40. doi: 10.1080/09168451.2020.1793293
126. Zhu SS, Liu JW, Yan YM, Liu Y, Mao Z, Cheng YX. Terpenoids from Resina commiphora regulating lipid metabolism via activating PPARα and CPT1 expression. Org Lett. (2020) 22(9):3428–32. doi: 10.1021/acs.orglett.0c00898
127. Wu Y, Wang F, Fan L, Zhang W, Wang T, Du Y, et al. Baicalin alleviates atherosclerosis by relieving oxidative stress and inflammatory responses via inactivating the NF-κB and p38 MAPK signaling pathways. Biomed Pharmacother. (2018) 97:1673–9. doi: 10.1016/j.biopha.2017.12.024
128. Gu Y, Duan S, Ding M, Zheng Q, Fan G, Li X, et al. Saikosaponin D attenuates metabolic associated fatty liver disease by coordinately tuning PPARα and INSIG/SREBP1c pathway. Phytomedicine. (2022) 103:154219. doi: 10.1016/j.phymed.2022.154219
129. Chen S, Sun S, Feng Y, Li X, Yin G, Liang P, et al. Diosgenin attenuates nonalcoholic hepatic steatosis through the hepatic FXR-SHP-SREBP1C/PPARα/CD36 pathway. Eur J Pharmacol. (2023) 952:175808. doi: 10.1016/j.ejphar.2023.175808
130. Loza-Rodríguez H, Estrada-Soto S, Alarcón-Aguilar FJ, Huang F, Aquino-Jarquín G, Fortis-Barrera Á, et al. Oleanolic acid induces a dual agonist action on PPARγ/α and GLUT4 translocation: a pentacyclic triterpene for dyslipidemia and type 2 diabetes. Eur J Pharmacol. (2020) 883:173252. doi: 10.1016/j.ejphar.2020.173252
131. Scandiffio R, Bonzano S, Cottone E, Shrestha S, Bossi S, De Marchis S, et al. Beta-Caryophyllene modifies intracellular lipid composition in a cell model of hepatic steatosis by acting through CB2 and PPAR receptors. Int J Mol Sci. (2023) 24(7):6060. doi: 10.3390/ijms24076060
132. Guo M, Guo G, Xiao J, Sheng X, Zhang X, Tie Y, et al. Ginsenoside Rg3 stereoisomers differentially inhibit vascular smooth muscle cell proliferation and migration in diabetic atherosclerosis. J Cell Mol Med. (2018) 22(6):3202–14. doi: 10.1111/jcmm.13601
133. Liao JT, Huang YW, Hou CY, Wang JJ, Wu CC, Hsieh SL. D-limonene promotes anti-obesity in 3T3-L1 adipocytes and high-calorie diet-induced obese rats by activating the AMPK signaling pathway. Nutrients. (2023) 15(2):267. doi: 10.3390/nu15020267
134. Meng J, Hu X, Zhang T, Dong P, Li Z, Xue C, et al. Saponin from sea cucumber exhibited more significant effects than ginsenoside on ameliorating high fat diet-induced obesity in C57BL/6 mice. Medchemcomm. (2018) 9(4):725–34. doi: 10.1039/c7md00653e
135. Hu XQ, Wang YM, Wang JF, Xue Y, Li ZJ, Nagao K, et al. Dietary saponins of sea cucumber alleviate orotic acid-induced fatty liver in rats via PPARalpha and SREBP-1c signaling. Lipids Health Dis. (2010) 9:25. doi: 10.1186/1476-511x-9-25
136. Guo Y, Han X, Che H, Li Z, Dong P, Xue C, et al. Synergistic effect of eicosapentaenoic acid-enriched phospholipids and sea cucumber saponin on orotic acid-induced non-alcoholic fatty liver disease in rats. R Soc Open Sci. (2018) 5(7):172182. doi: 10.1098/rsos.172182
137. Lin P, Shen N, Yin F, Guo SD. Sea cucumber-derived compounds for treatment of dyslipidemia: a review. Front Pharmacol. (2022) 13:1000315. doi: 10.3389/fphar.2022.1000315
138. Wen M, Cui J, Xu J, Xue Y, Wang J, Xue C, et al. Effects of dietary sea cucumber saponin on the gene expression rhythm involved in circadian clock and lipid metabolism in mice during nighttime-feeding. J Physiol Biochem. (2014) 70(3):801–8. doi: 10.1007/s13105-014-0349-9
139. Wen M, Fu X, Han X, Hu X, Dong P, Xu J, et al. Sea cucumber saponin echinoside a (EA) stimulates hepatic fatty acid β-oxidation and suppresses fatty acid biosynthesis coupling in a diurnal pattern. J Nutr Sci Vitaminol (Tokyo. (2016) 62(3):170–7. doi: 10.3177/jnsv.62.170
140. D'Aniello E, Amodeo P, Vitale RM. Marine natural and nature-inspired compounds targeting peroxisome proliferator activated receptors (PPARs). Mar Drugs. (2023) 21(2):89. doi: 10.3390/md21020089
141. Liu J, Jiang C, Ma X, Wang J. Notoginsenoside Fc attenuates high glucose-induced vascular endothelial cell injury via upregulation of PPAR-γ in diabetic Sprague-Dawley rats. Vascul Pharmacol. (2018) 109:27–35. doi: 10.1016/j.vph.2018.05.009
142. Zhang Y. Mechanism of neuroprotective effect of stevioside on cerebral ischemia-reperfusion injury via PPAR-γ activation. Immunopharmacol Immunotoxicol. (2021) 43(6):704–12. doi: 10.1080/08923973.2021.1966034
143. Geng J, Fu W, Yu X, Lu Z, Liu Y, Sun M, et al. Ginsenoside Rg3 alleviates ox-LDL induced endothelial dysfunction and prevents atherosclerosis in ApoE(-/-) mice by regulating PPARγ/FAK signaling pathway. Front Pharmacol. (2020) 11:500. doi: 10.3389/fphar.2020.00500
144. Abdolmohammadi MH, Roozbehani M, Hamzeloo-Moghadam M, Heidari F, Fallahian F. Targeting PPARγ/NF-κB signaling pathway by britannin, a sesquiterpene lactone from Inula aucheriana dc., in gastric cancer. Anticancer Agents Med Chem. (2023) 23(19):2102–10. doi: 10.2174/1871520623666230918140559
145. Ngoc NB, Lv P, Zhao WE. Suppressive effects of lycopene and β-carotene on the viability of the human esophageal squamous carcinoma cell line EC109. Oncol Lett. (2018) 15(5):6727–32. doi: 10.3892/ol.2018.8175
146. Liu P, Du J. Oridonin is an antidepressant molecule working through the PPAR-γ/AMPA receptor signaling pathway. Biochem Pharmacol. (2020) 180:114136. doi: 10.1016/j.bcp.2020.114136
147. Ma J, Xu Y, Zhang M, Li Y. Geraniol ameliorates acute liver failure induced by lipopolysaccharide/D-galactosamine via regulating macrophage polarization and NLRP3 inflammasome activation by PPAR-γ methylation Geraniol alleviates acute liver failure. Biochem Pharmacol. (2023) 210:115467. doi: 10.1016/j.bcp.2023.115467
148. Shao M, Wang M, Ma L, Wang Q, Gao P, Tian X, et al. β-elemene blocks lipid-induced inflammatory pathways via PPARβ activation in heart failure. Eur J Pharmacol. (2021) 910:174450. doi: 10.1016/j.ejphar.2021.174450
149. Huang Y, Lang H, Chen K, Zhang Y, Gao Y, Ran L, et al. Resveratrol protects against nonalcoholic fatty liver disease by improving lipid metabolism and redox homeostasis via the PPARα pathway. Appl Physiol Nutr Metab. (2020) 45(3):227–39. doi: 10.1139/apnm-2019-0057
150. Ye G, Chen G, Gao H, Lin Y, Liao X, Zhang H, et al. Resveratrol inhibits lipid accumulation in the intestine of atherosclerotic mice and macrophages. J Cell Mol Med. (2019) 23(6):4313–25. doi: 10.1111/jcmm.14323
151. Li Q, Zheng Y, Sun Y, Xu G. Resveratrol attenuated fatty acid synthesis through MAPK-PPAR pathway in red tilapia. Comp Biochem Physiol C Toxicol Pharmacol. (2023) 268:109598. doi: 10.1016/j.cbpc.2023.109598
152. Wu Y, Xue L, Du W, Huang B, Tang C, Liu C, et al. Polydatin restores endothelium-dependent relaxation in rat aorta rings impaired by high glucose: a novel insight into the PPARβ-NO signaling pathway. PLoS One. (2015) 10(5):e0126249. doi: 10.1371/journal.pone.0126249
153. Askar ME, Ali SI, Younis NN, Shaheen MA, Zaher ME. Raspberry ketone ameliorates nonalcoholic fatty liver disease in rats by activating the AMPK pathway. Eur J Pharmacol. (2023) 957:176001. doi: 10.1016/j.ejphar.2023.176001
154. Sharifi-Rad J, Quispe C, Castillo CMS, Caroca R, Lazo-Vélez MA, Antonyak H, et al. Ellagic acid: a review on its natural sources, chemical stability, and therapeutic potential. Oxid Med Cell Longev. (2022) 2022:3848084. doi: 10.1155/2022/3848084
155. Duan J, Pan J, Sun M, Fang Y. Comparative multiomics study of the effects of ellagic acid on the gut environment in young and adult mice. Food Res Int. (2022) 161:111819. doi: 10.1016/j.foodres.2022.111819
156. Fang X, Cao J, Tao Z, Yang Z, Dai Y, Zhao L. Hydroxytyrosol attenuates ethanol-induced liver injury by ameliorating steatosis, oxidative stress and hepatic inflammation by interfering STAT3/iNOS pathway. Redox Rep. (2023) 28(1):2187564. doi: 10.1080/13510002.2023.2187564
157. Geethangili M, Lin CW, Mersmann HJ, Ding ST. Methyl Brevifolincarboxylate attenuates free fatty acid-induced lipid metabolism and inflammation in hepatocytes through AMPK/NF-κB signaling pathway. Int J Mol Sci. (2021) 22(18):10062. doi: 10.3390/ijms221810062
158. Shi L, Karrar E, Wang X. Sesamol ameliorates hepatic lipid accumulation and oxidative stress in steatosis HepG2 cells via the PPAR signaling pathway. J Food Biochem. (2021) 45(11):e13976. doi: 10.1111/jfbc.13976
159. Cisneros-Zevallos L, Bang WY, Delgadillo-puga C. Ellagic acid and urolithins a and b differentially regulate fat accumulation and inflammation in 3t3-l1 adipocytes while not affecting adipogenesis and insulin sensitivity. Int J Mol Sci. (2020) 21(6):2086. doi: 10.3390/ijms21062086
160. Xiao Y, Li X, Wang L, Hu M, Liu Y. Proanthocyanidin A2 attenuates the activation of hepatic stellate cells by activating the PPAR-γ signalling pathway. Autoimmunity. (2023) 56(1):2250101. doi: 10.1080/08916934.2023.2250101
161. Corpetti C, Del Re A, Seguella L, Palenca I, Rurgo S, De Conno B, et al. Cannabidiol inhibits SARS-Cov-2 spike (S) protein-induced cytotoxicity and inflammation through a PPARγ-dependent TLR4/NLRP3/Caspase-1 signaling suppression in Caco-2 cell line. Phytother Res. (2021) 35(12):6893–903. doi: 10.1002/ptr.7302
162. Kuret T, Kreft ME, Romih R, Veranič P. Cannabidiol as a promising therapeutic option in IC/BPS: in vitro evaluation of its protective effects against inflammation and oxidative stress. Int J Mol Sci. (2023) 24(5):5055. doi: 10.3390/ijms24055055
163. Wang N, Kong R, Han W, Bao W, Shi Y, Ye L, et al. Honokiol alleviates ulcerative colitis by targeting PPAR-γ-TLR4-NF-κB signaling and suppressing gasdermin-D-mediated pyroptosis in vivo and in vitro. Int Immunopharmacol. (2022) 111:109058. doi: 10.1016/j.intimp.2022.109058
164. Ma Z, Sheng L, Li J, Qian J, Wu G, Wang Z, et al. Resveratrol alleviates hepatic fibrosis in associated with decreased endoplasmic reticulum stress-mediated apoptosis and inflammation. Inflammation. (2022) 45(2):812–23. doi: 10.1007/s10753-021-01586-w
165. Wang J, Xue X, Zhao X, Luo L, Liu J, Dai S, et al. Forsythiaside a alleviates acute lung injury by inhibiting inflammation and epithelial barrier damages in lung and colon through PPAR-γ/RXR-α complex. J Adv Res. (2023) S2090-1232(23):00222-9 doi: 10.1016/j.jare.2023.08.006
166. Yao Z, Liu N, Lin H, Zhou Y. Proanthocyanidin alleviates liver ischemia/reperfusion injury by suppressing autophagy and apoptosis via the PPARα/PGC1α signaling pathway. J Clin Transl Hepatol. (2023) 11(6):1329–40. doi: 10.14218/jcth.2023.00071
167. Wu Q, Wang Q, Fu J, Ren R. Polysaccharides derived from natural sources regulate triglyceride and cholesterol metabolism: a review of the mechanisms. Food Funct. (2019) 10(5):2330–9. doi: 10.1039/c8fo02375a
168. Ye D, Zhao Q, Ding D, Ma BL. Preclinical pharmacokinetics-related pharmacological effects of orally administered polysaccharides from traditional Chinese medicines: a review. Int J Biol Macromol. (2023) 252:126484. doi: 10.1016/j.ijbiomac.2023.126484
169. Li J, Cai C, Yang C, Li J, Sun T, Yu G. Recent advances in pharmaceutical potential of brown algal polysaccharides and their derivatives. Curr Pharm Des. (2019) 25(11):1290–311. doi: 10.2174/1381612825666190618143952
170. Yang Z, Liu G, Wang Y, Yin J, Wang J, Xia B, et al. Fucoidan A2 from the brown seaweed Ascophyllum nodosum lowers lipid by improving reverse cholesterol transport in C57BL/6J mice fed a high-fat diet. J Agric Food Chem. (2019) 67(20):5782–91. doi: 10.1021/acs.jafc.9b01321
171. Peng Y, Wang Y, Wang Q, Luo X, He Y, Song Y. Hypolipidemic effects of sulfated fucoidan from Kjellmaniella crassifolia through modulating the cholesterol and aliphatic metabolic pathways. J Funct Foods. (2018) 51:8–15. doi: 10.1016/j.jff.2018.10.013
172. Ren D, Wang Q, Yang Y, Hu Y, Song Y, He Y, et al. Hypolipidemic effects of fucoidan fractions from Saccharina sculpera (laminariales, phaeophyceae). Int J Biol Macromol. (2019) 140:188–95. doi: 10.1016/j.ijbiomac.2019.08.002
173. Yokota T, Nomura K, Nagashima M, Kamimura N. Fucoidan alleviates high-fat diet-induced dyslipidemia and atherosclerosis in ApoE(shl) mice deficient in apolipoprotein E expression. J Nutr Biochem. (2016) 32:46–54. doi: 10.1016/j.jnutbio.2016.01.011
174. Yin J, Wang J, Li F, Yang Z, Yang X, Sun W, et al. The fucoidan from the brown seaweed Ascophyllum nodosum ameliorates atherosclerosis in apolipoprotein E-deficient mice. Food Funct. (2019) 10(8):5124–39. doi: 10.1039/c9fo00619b
175. Li S, Li J, Mao G, Hu Y, Ye X, Tian D, et al. Fucosylated chondroitin sulfate oligosaccharides from Isostichopus badionotus regulates lipid disorder in C57BL/6 mice fed a high-fat diet. Carbohydr Polym. (2018) 201:634–42. doi: 10.1016/j.carbpol.2018.08.020
176. Yu L, Ge L, Xue C, Chang Y, Zhang C, Xu X, et al. Structural study of fucoidan from sea cucumber Acaudina molpadioides: a fucoidan containing novel tetrafucose repeating unit. Food Chem. (2014) 142:197–200. doi: 10.1016/j.foodchem.2013.06.079
177. Xu H, Wang J, Chang Y, Xu J, Wang Y, Long T, et al. Fucoidan from the sea cucumber Acaudina molpadioides exhibits anti-adipogenic activity by modulating the Wnt/β-catenin pathway and down-regulating the SREBP-1c expression. Food Funct. (2014) 5(7):1547–55. doi: 10.1039/c3fo60716j
178. Zhao F, Ma T, Zhang X, Zhao Q, Zhu K, Cao J, et al. Holothuria leucospilota polysaccharides improve immunity and the gut microbiota in cyclophosphamide-treated immunosuppressed mice. Mol Nutr Food Res. (2023) 67(8):e2200317. doi: 10.1002/mnfr.202200317
179. Kalita P, Ahmed AB, Sen S, Chakraborty R. A comprehensive review on polysaccharides with hypolipidemic activity: occurrence, chemistry and molecular mechanism. Int J Biol Macromol. (2022) 206:681–98. doi: 10.1016/j.ijbiomac.2022.02.189
180. Miao M, Yu WQ, Li Y, Sun YL, Guo SD. Structural elucidation and activities of Cordyceps militaris-derived polysaccharides: a review. Front Nutr. (2022) 9:898674. doi: 10.3389/fnut.2022.898674
181. Yang ZW, Ouyang KH, Zhao J, Chen H, Xiong L, Wang WJ. Structural characterization and hypolipidemic effect of Cyclocarya paliurus polysaccharide in rat. Int J Biol Macromol. (2016) 91:1073–80. doi: 10.1016/j.ijbiomac.2016.06.063
182. Huang Z, Ye Y, Xu A, Li Z. Effects of Astragalus membranaceus polysaccharides on growth performance, physiological and biochemical parameters, and expression of genes related to lipid metabolism of spotted sea bass, Lateolabrax maculatus. Aquac Nutr. (2023) 2023:6191330. doi: 10.1155/2023/6191330
183. Wang L, Yang K, Jing R, Zhao W, Guo K, Hu Z, et al. Protective effect of Saussurea involucrata polysaccharide against skin dryness induced by ultraviolet radiation. Front Pharmacol. (2023) 14:1089537. doi: 10.3389/fphar.2023.1089537
184. Yu WQ, Wang XL, Ji HH, Miao M, Zhang BH, Li H, et al. CM3-SII Polysaccharide obtained from Cordyceps militaris ameliorates hyperlipidemia in heterozygous LDLR-deficient hamsters by modulating gut microbiota and NPC1L1 and PPARα levels. Int J Biol Macromol. (2023) 239:124293. doi: 10.1016/j.ijbiomac.2023.124293
185. Liu B, Yu L, Zhai Q, Li M, Li L, Tian F, et al. Effect of water-soluble polysaccharides from Morchella esculenta on high-fat diet-induced obese mice: changes in gut microbiota and metabolic functions. Food Funct. (2023) 14(11):5217–31. doi: 10.1039/d3fo00574g
186. Liu YH, Xiang ZN, Chen C, Wan LS, Chen JC. Hypolipidemic and hepatoprotective effects of polysaccharides extracted from Liriope spicata var. prolifera in C57BL/6J mice with high-fat diet-induced hyperlipidemia. Evid Based Complement Alternat Med. (2020) 2020:8013189. doi: 10.1155/2020/8013189
187. Ke W, Flay KJ, Huang X, Hu X, Chen F, Li C, et al. Polysaccharides from Platycodon grandiflorus attenuates high-fat diet induced obesity in mice through targeting gut microbiota. Biomed Pharmacother. (2023) 166:115318. doi: 10.1016/j.biopha.2023.115318
188. Wang Z, Du H, Peng W, Yang S, Feng Y, Ouyang H, et al. Efficacy and mechanism of Pueraria lobata and Pueraria thomsonii polysaccharides in the treatment of type 2 diabetes. Nutrients. (2022) 14(19):3926. doi: 10.3390/nu14193926
189. Xia H, Zhou B, Sui J, Ma W, Wang S, Yang L, et al. Lycium barbarum polysaccharide regulates the lipid metabolism and alters gut microbiota in high-fat diet induced obese mice. Int J Environ Res Public Health. (2022) 19(19):12093. doi: 10.3390/ijerph191912093
190. Ma D, Wu T, Qu Y, Yang J, Cai L, Li X, et al. Astragalus polysaccharide prevents heart failure-induced cachexia by alleviating excessive adipose expenditure in white and brown adipose tissue. Lipids Health Dis. (2023) 22(1):9. doi: 10.1186/s12944-022-01770-3
191. Chen J, Huang W, Zhang T, Lu M, Jiang B. Anti-obesity potential of rare sugar D-psicose by regulating lipid metabolism in rats. Food Funct. (2019) 10(5):2417–25. doi: 10.1039/c8fo01089g
192. Hu M, Chen Y, Deng F, Chang B, Luo J, Dong L, et al. D-mannose regulates hepatocyte lipid metabolism via PI3K/akt/mTOR signaling pathway and ameliorates hepatic steatosis in alcoholic liver disease. Front Immunol. (2022) 13:877650. doi: 10.3389/fimmu.2022.877650
193. Guo S, Tian H, Dong R, Yang N, Zhang Y, Yao S, et al. Exogenous supplement of N-acetylneuraminic acid ameliorates atherosclerosis in apolipoprotein E-deficient mice. Atherosclerosis. (2016) 251:183–91. doi: 10.1016/j.atherosclerosis.2016.05.032
194. Hou P, Hu S, Wang J, Yang Z, Yin J, Zhou G, et al. Exogenous supplement of N-acetylneuraminic acid improves macrophage reverse cholesterol transport in apolipoprotein E-deficient mice. Lipids Health Dis. (2019) 18(1):24. doi: 10.1186/s12944-019-0971-1
195. Naiini MR, Shahouzehi B, Azizi S, Shafiei B, Nazari-Robati M. Trehalose-induced SIRT1/AMPK activation regulates SREBP-1c/PPAR-α to alleviate lipid accumulation in aged liver. Naunyn Schmiedebergs Arch Pharmacol. (2023) 397(2):1061–70. doi: 10.1007/s00210-023-02644-w
196. Inci MK, Park SH, Helsley RN, Attia SL, Softic S. Fructose impairs fat oxidation: implications for the mechanism of western diet-induced NAFLD. J Nutr Biochem. (2023) 114:109224. doi: 10.1016/j.jnutbio.2022.109224
197. Ahmad T, Eapen MS, Ishaq M, Park AY, Karpiniec SS, Stringer DN, et al. Anti-inflammatory activity of fucoidan extracts in vitro. Mar Drugs. (2021) 19(12):702. doi: 10.3390/md19120702
198. Jayawardena TU, Nagahawatta DP, Fernando IPS, Kim YT, Kim JS, Kim WS, et al. A review on fucoidan structure, extraction techniques, and its role as an immunomodulatory agent. Mar Drugs. (2022) 20(12):755. doi: 10.3390/md20120755
199. Liu X, Chen S, Liu H, Xie J, Hasan KMF, Zeng Q, et al. Structural properties and anti-inflammatory activity of purified polysaccharides from Hen-of-the-woods mushrooms (Grifola frondosa). Front Nutr. (2023) 10:1078868. doi: 10.3389/fnut.2023.1078868
200. Gao Y, Guo M, Zheng P, Liu R, Wang D, Zhao D, et al. Effects of sulfated polysaccharides from Laminaria japonica on regulating the gut microbiota and alleviating intestinal inflammation in obese mice. Food Chem Toxicol. (2022) 168:113401. doi: 10.1016/j.fct.2022.113401
201. Xu T, Liu R, Lu X, Wu X, Heneberg P, Mao Y, et al. Lycium barbarum polysaccharides alleviate LPS-induced inflammatory responses through PPARγ/MAPK/NF-κB pathway in bovine mammary epithelial cells. J Anim Sci. (2022) 100(1):skab345. doi: 10.1093/jas/skab345
202. Mohamed Husien H, Peng W, Su H, Zhou R, Tao Y, Huang J, et al. Moringa oleifera leaf polysaccharide alleviates experimental colitis by inhibiting inflammation and maintaining intestinal barrier. Front Nutr. (2022) 9:1055791. doi: 10.3389/fnut.2022.1055791
203. Hua H, Liu L, Zhu T, Cheng F, Qian H, Shen F, et al. Healthy regulation of Tibetan Brassica rapa L. Polysaccharides on alleviating hyperlipidemia: a rodent study. Food Chem (Oxf). (2023) 6:100171. doi: 10.1016/j.fochms.2023.100171
204. Lee S, Lee EJ, Lee GM, Yun JH, Yoo W. Inhibitory effect of fucoidan on TNF-α-induced inflammation in human retinal pigment epithelium cells. Front Nutr. (2023) 10:1162934. doi: 10.3389/fnut.2023.1162934
205. Yang L, Ling W, Qiu Y, Liu Y, Wang L, Yang J, et al. Anthocyanins increase serum adiponectin in newly diagnosed diabetes but not in prediabetes: a randomized controlled trial. Nutr Metab. (2020) 17(1):78. doi: 10.1186/s12986-020-00498-0
206. Xu Z, Xie J, Zhang H, Pang J, Li Q, Wang X, et al. Anthocyanin supplementation at different doses improves cholesterol efflux capacity in subjects with dyslipidemia-a randomized controlled trial. Eur J Clin Nutr. (2020) 75(2):345–54. doi: 10.1038/s41430-020-0609-4
207. Zhao Y, Xu H, Tian Z, Wang X, Xu L, Li K, et al. Dose-dependent reductions in plasma ceramides after anthocyanin supplementation are associated with improvements in plasma lipids and cholesterol efflux capacity in dyslipidemia: a randomized controlled trial. Clin Nutr. (2021) 40(4):1871–8. doi: 10.1016/j.clnu.2020.10.014
208. Yari Z, Movahedian M, Imani H, Alavian SM, Hedayati M, Hekmatdoost A. The effect of hesperidin supplementation on metabolic profiles in patients with metabolic syndrome: a randomized, double-blind, placebo-controlled clinical trial. Eur J Nutr. (2019) 59(6):2569–77. doi: 10.1007/s00394-019-02105-2
209. Miwa Y, Mitsuzumi H, Sunayama T, Yamada M, Okada K, Kubota M, et al. Glucosyl hesperidin lowers serum triglyceride level in hypertriglyceridemic subjects through the improvement of very low-density lipoprotein metabolic abnormality. J Nutr Sci Vitaminol. (2005) 51(6):460–70. doi: 10.3177/jnsv.51.460
210. Yari Z, Cheraghpour M, Hekmatdoost A. Flaxseed and/or hesperidin supplementation in metabolic syndrome: an open-labeled randomized controlled trial. Eur J Nutr. (2020) 60(1):287–98. doi: 10.1007/s00394-020-02246-9
211. Guevara-Cruz M, Godinez-Salas ET, Sanchez-Tapia M, Torres-Villalobos G, Pichardo-Ontiveros E, Guizar-Heredia R, et al. Genistein stimulates insulin sensitivity through gut microbiota reshaping and skeletal muscle AMPK activation in obese subjects. BMJ Open Diabetes Res Care. (2020) 8(1):e000948. doi: 10.1136/bmjdrc-2019-000948
212. Pokushalov E, Ponomarenko A, Bayramova S, Garcia C, Pak I, Shrainer E, et al. Evaluating the impact of Omega-3 fatty acid (SolowaysTM) supplementation on lipid profiles in adults with PPARG polymorphisms: a randomized, double-blind, placebo-controlled trial. Nutrients. (2023) 16(1):97. doi: 10.3390/nu16010097
213. Murru E, Carta G, Cordeddu L, Melis M, Desogus E, Ansar H, et al. Dietary conjugated linoleic acid-enriched cheeses influence the levels of circulating n-3 highly unsaturated fatty acids in humans. Int J Mol Sci. (2018) 19(6):1730. doi: 10.3390/ijms19061730
214. Chatree S, Sitticharoon C, Maikaew P, Pongwattanapakin K, Keadkraichaiwat I, Churintaraphan M, et al. Epigallocatechin gallate decreases plasma triglyceride, blood pressure, and serum kisspeptin in obese human subjects. Exp Bio Med (Maywood). (2020) 246(2):163–76. doi: 10.1177/1535370220962708
215. Tardif JC, Kouz S, Waters DD, Bertrand OF, Diaz R, Maggioni AP, et al. Efficacy and safety of low-dose colchicine after myocardial infarction. N Engl J Med. (2019) 381(26):2497–505. doi: 10.1056/NEJMoa1912388
216. Haghighatdoost F, Hariri M. Effect of resveratrol on lipid profile: an updated systematic review and meta-analysis on randomized clinical trials. Pharmacol Res. (2018) 129:141–50. doi: 10.1016/j.phrs.2017.12.033
217. Ni H-x, Yu N-j, Yang X-h. The study of ginsenoside on PPARγ expression of mononuclear macrophage in type 2 diabetes. Mol Bio Rep. (2009) 37(6):2975–9. doi: 10.1007/s11033-009-9864-0
218. Kim JY, Paik JK, Kim OY, Park HW, Lee JH, Jang Y, et al. Effects of lycopene supplementation on oxidative stress and markers of endothelial function in healthy men. Atherosclerosis. (2011) 215(1):189–95. doi: 10.1016/j.atherosclerosis.2010.11.036
219. Jenko Pražnikar Z, Mohorko N, Gmajner D, Kenig S, Petelin A. Effects of four different dietary fibre supplements on weight loss and lipid and glucose serum profiles during energy restriction in patients with traits of metabolic syndrome: a comparative, randomized, placebo-controlled study. Foods. (2023) 12(11):2122. doi: 10.3390/foods12112122
220. Thondre PS, Shafat A, Clegg ME. Molecular weight of barley β-glucan influences energy expenditure, gastric emptying and glycaemic response in human subjects. Br J Nutr. (2013) 110(12):2173–9. doi: 10.1017/s0007114513001682
221. Iacoviello L, Zito F, Rago L, Di Castelnuovo A, De Curtis A, Zappacosta B, et al. Prolonged administration of Ascophyllum nodosum to healthy human volunteers and cardiovascular risk. Nutrafoods. (2014) 12(4):137–44. doi: 10.1007/s13749-013-0059-x
222. Gao L-L, Li Y-X, Ma J-M, Guo Y-Q, Li L, Gao Q-H, et al. Effect of Lycium barbarum polysaccharide supplementation in non-alcoholic fatty liver disease patients: study protocol for a randomized controlled trial. Trials. (2021) 22(1):566. doi: 10.1186/s13063-021-05529-6
223. Sargowo D, Ovianti N, Susilowati E, Ubaidillah N, Widya Nugraha A, Siwi Proboretno K, et al. The role of polysaccharide peptide of Ganoderma lucidum as a potent antioxidant against atherosclerosis in high risk and stable angina patients. Indian Heart J. (2018) 70(5):608–14. doi: 10.1016/j.ihj.2017.12.007
Keywords: PPARα, PPARβ, PPARγ, lipid metabolism, inflammation, cardiovascular disease
Citation: Zhang Y, Zhang X-Y, Shi S-R, Ma C-N, Lin Y-P, Song W-G and Guo S-D (2024) Natural products in atherosclerosis therapy by targeting PPARs: a review focusing on lipid metabolism and inflammation. Front. Cardiovasc. Med. 11:1372055. doi: 10.3389/fcvm.2024.1372055
Received: 17 January 2024; Accepted: 9 April 2024;
Published: 18 April 2024.
Edited by:
Jun Yu, Temple University, United StatesReviewed by:
Chao Zhong, Jiangxi University of Chinese Medicine, China© 2024 Zhang, Zhang, Shi, Ma, Lin, Song and Guo. This is an open-access article distributed under the terms of the Creative Commons Attribution License (CC BY). The use, distribution or reproduction in other forums is permitted, provided the original author(s) and the copyright owner(s) are credited and that the original publication in this journal is cited, in accordance with accepted academic practice. No use, distribution or reproduction is permitted which does not comply with these terms.
*Correspondence: Wen-Gang Song cy5jb21AMTYzLmNvbQ== Shou-Dong Guo U0QtR1VPQGhvdG1haWwuY29t
†These authors have contributed equally to this work
Disclaimer: All claims expressed in this article are solely those of the authors and do not necessarily represent those of their affiliated organizations, or those of the publisher, the editors and the reviewers. Any product that may be evaluated in this article or claim that may be made by its manufacturer is not guaranteed or endorsed by the publisher.
Research integrity at Frontiers
Learn more about the work of our research integrity team to safeguard the quality of each article we publish.