- 1Department of Cardiology, Radboud University Medical Center, Nijmegen, Netherlands
- 2Department of Cardiology, Northwest Clinics, Alkmaar, Netherlands
- 3Department of Cardiology, MedStar Washington Hospital Center, Washington, DC, United States
- 4Dutch Network for Cardiovascular Research (WCN), Utrecht, Netherlands
Coronary atherosclerosis remains a leading cause of morbidity and mortality worldwide. The underlying pathophysiology includes a complex interplay of endothelial dysfunction, lipid accumulation and inflammatory pathways. Multiple structural and inflammatory features of the atherosclerotic lesions have become targets to identify high-risk lesions. Various intracoronary imaging devices have been developed to assess the morphological, biocompositional and molecular profile of the intracoronary atheromata. These techniques guide interventional and therapeutical management and allow the identification and stratification of atherosclerotic lesions. We sought to provide an overview of the inflammatory pathobiology of atherosclerosis, distinct high-risk plaque features and the ability to visualize this process with contemporary intracoronary imaging techniques.
1 Introduction
Atherosclerotic cardiovascular disease continues to be a major health burden worldwide (1). Ischemic heart disease is responsible for more than 15% of all global deaths (2, 3). The coronary vessel wall experiences accumulation of inflammatory cells, lipids, fibrous tissue and calcium leading to progressive narrowing of its lumen (4–6). Rupture of the fibrous cap or plaque erosion can trigger local thrombosis, which extends into the coronary lumen and subsequently impedes blood flow (7, 8). Plaque rupture is the most common mechanism of fatal acute myocardial infarction and sudden cardiac death (9). To reduce cardiovascular events, lipid-lowering- and plaque stabilization therapies, including statins and ezetimibe, have become cornerstones in treatment strategy. More recently, Proprotein Convertase Subtilisin Kexin type 9 (PCSK9) monoclonal antibodies have been added to the treatment possibilities (10, 11). Despite these intensive lipid-lowering treatments, residual risks persists. This most likely reflects mechanisms in the biology of atherosclerosis that are incompletely managed by controlling dyslipidemia, which includes the inflammatory response (12, 13). As exemplification, patients with target concentrations of low-density-lipoprotein (LDL) below 1.8 mmol/L and a high-sensitivity C-reactive protein (hsCRP) < 2 mg/L have the best clinical outcomes (14, 15). Furthermore, the anti-inflammatory drugs canakinumab and colchicine have demonstrated to reduce recurrent cardiovascular events (16, 17). Detection of coronary artery wall inflammation might identify which patients benefit from anti-inflammatory therapy (18).
Intracoronary imaging has greatly improved our understanding of the pathophysiology in atherosclerotic cardiovascular disease (19). Most articles discussing intracoronary imaging, focus on the structural characteristics of high-risk plaque. In addition to structural features, the present review aims to address the underlying inflammatory process and whether it is feasible to visualize markers of this process with current and future invasive in vivo imaging.
2 Inflammation in atherosclerosis
Atherosclerosis is initiated at the inner layer of the intima (Figure 1). LDL particles accumulate in the subendothelial space at sites with endothelial dysfunction and turbulent flow (20). Subsequently, LDL particles cluster and become oxidized by reactive oxygen species. Oxidized LDL increases local endothelial permeability (21). As a result, patrolling monocytes enter the subintimal space and differentiate into macrophages. These macrophages amass lipids through cholesterol uptake by scavenger receptors, leading to “foam cell” formation. Foam cells induce chemokine and cytokine production, which attracts new leukocytes (22). This positive feedback loop perpetuates and increases plaque formation. The accumulated inflammatory cells secrete a wide range of proteases. Cathepsins and matrix metalloproteinases are common, macrophage-derived, proteases within the atherosclerotic plaque. These proteases are involved in proteolysis of the extracellular matrix, lesion progression and plaque instability (23, 24).
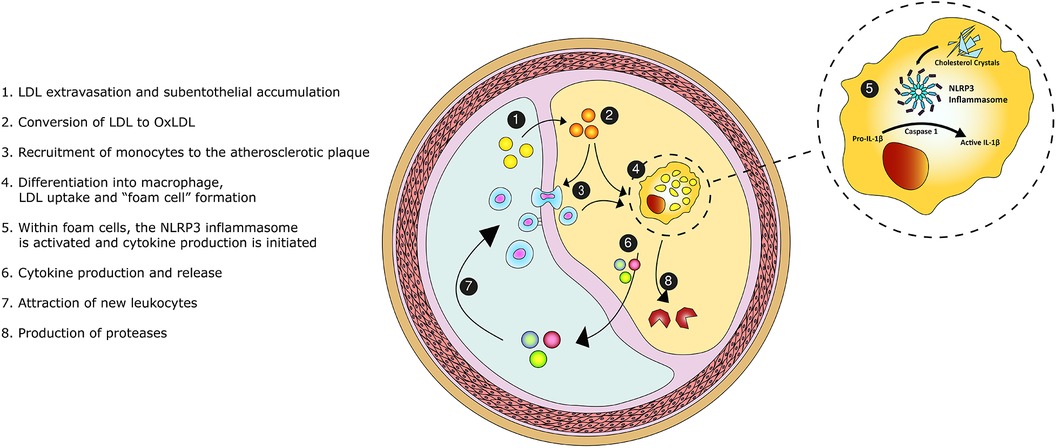
Figure 1. Graphical overview of the inflammatory pathobiology of atherosclerosis. Legend: Low-density-lipoprotein (LDL) enters the intimal space. The accumulated LDL particles become oxidized and promote the recruitment of monocytes. Monocytes transform into macrophages. Upon the scavenging of lipids, macrophage differentiate into foam cells. Within foam cells, the NOD-, LRR- and pyrin domain-containing protein 3 (NLRP3) inflammasome becomes activated and initiates cytokine en chemokine production, perpetuating the inflammatory process. Foam cells secrete proteases causing proteolysis of the extracellular matrix and destabilizing the atherosclerotic plaque.
In addition to inflammatory cells, vascular smooth muscle cells have shown to migrate into the intima (20). Upon exposure to lipids and cytokines, vascular smooth muscle cells transform into a proliferating cell type, expressing markers of macrophages (25). These macrophage-like cells can also take up lipids and may promote inflammation (20, 26).
Within the monocytes and macrophages, the NOD-, LRR- and pyrin domain-containing protein 3 (NLRP3) inflammasome pathway initiates cytokine production (27, 28). This protein complex is activated by cholesterol crystals (29, 30). Upon activation, Caspase 1 cleaves the inactive interleukin-1β (IL-1β) precursor. Thereafter, activated IL-1β is released into the circulation. IL-1β induces the inflammatory function of human endothelial cells and stimulates adhesion molecules that recruit leukocytes. IL-1β triggers the release of multiple cytokines, chemokines and other inflammatory mediators (31). For example, interleukin-6 is a downstream cytokine which induces the production of C-reactive protein (CRP) and fibrinogen, promoting thrombosis (28). Therefore, IL-1β is perceived to be the pivotal cytokine in the inflammatory cascade and a driver of atherosclerosis (32). Our understanding of atherosclerosis has thereby evolved to a complex, cholesterol crystal-induced, inflammation of the arterial wall.
3 Morphological features of high-risk plaque
Identification of high-risk lesions is of great importance, given that most atherosclerotic plaques responsible for acute coronary syndromes (ACS) are angiographically mild (33, 34). High-risk plaque refers to a lesion at high short-term risk of causing an acute clinical event (5). Lipid pools, cholesterol crystals, presence of macrophage, a large necrotic core, intraplaque hemorrhage and microcalcifications have been identified as hallmarks for high-risk lesions and represent markers of the underlying inflammatory driven process of atherosclerosis (35, 36). These hallmarks have become targets of intracoronary imaging techniques (Figure 2).
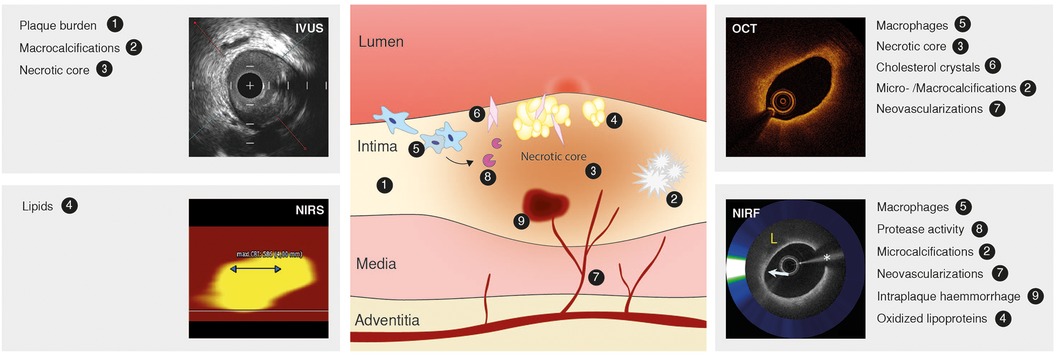
Figure 2. Atherosclerotic lesion, markers of inflammation and high-risk plaque and intravascular imaging techniques. Atherosclerotic lesion (middle) depicting the inflammatory pathobiology of atherosclerosis. Contemporary intracoronary imaging tools (intravascular ultrasound, IVUS; near-infrared spectroscopy, NIRS; optical coherence tomography, OCT; near-infrared fluorescence, NIRF) and their ability to display the process. Image of NIRF signal reused with permission from Ughi et al. (122).
3.1 Lipid pools and cholesterol crystals
Plasma-derived lipids accumulate in the subintimal space in the initial phase of plaque formation (20). Lipid pools do not only initiate an inflammatory reaction, but also increase biomechanical stress (37). Liquid cholesterol in these pools crystalizes, leading to volume expansion (38). This volume increase destabilizes the atherosclerotic plaque. Moreover, cholesterol crystals can injure the arterial wall and disrupt the plaque (39).
Cholesterol crystals are found in ∼39% of de novo culprit lesions of patients with either ACS or chronic coronary syndrome (CCS), and correlate with high-risk morphological features of culprit lesions (40). Cholesterol crystals are more often observed in culprit lesions in ST-elevation ACS patients compared to non-ST-elevation ACS patients, as is an increase in macrophage accumulation, spotty calcifications, mean lipid arc, thin-cap fibroatheromas (TCFAs) and thrombus (41). This supports the hypothesis that cholesterol crystals increase plaque vulnerability and trigger plaque rupture.
3.2 Macrophage and necrotic core
The necrotic core results from cell death and the inability to clear this debris. Macrophages play a major role in this process (42). Hypoxia, lipids and oxidative stress have shown to induce apoptosis in different cell types, including leukocytes and vascular smooth muscle cells (43). These signals trigger DNA fragmentation and expression of cell-surface markers that attract phagocytes (44). As macrophages are also the main phagocytes within the atherosclerotic plaque, effective clearance depends on neighboring cells. This process is called efferocytosis (45). There is no inflammatory reaction associated with apoptosis or efferocytosis, as cellular constituents are phagocytosed instead of released in the surroundings (44, 46). Within lipid-laden foam cells, intracellular cholesterol crystallizes and induces apoptosis (39). Efferocytosis becomes insufficient, which leads to a pool of dead macrophage forming a necrotic core.
A TCFA containing a large necrotic core, infiltrated by a high amount of macrophages is often displayed as “a classical example” of a lesion prone for rupture (47–49). The fibrous cap is defined as a distinctive layer of connective tissue overlying the necrotic core. It consists of smooth muscle cells in an extracellular matrix of collagen, proteoglycans and elastin. The media and adjacent adventitia may be infiltrated by varying degrees of lymphocytes, macrophages and foam cells (50, 51). Historically, fibrous caps with a minimum thickness of <65 µm are considered to be thin, as histopathological analysis showed a cap thickness of <64 µm in 95% of arteries with ruptured plaque (49).
3.3 Plaque neovascularization and intraplaque hemorrhage
Neovascularization and intraplaque hemorrhage are common phenomenons within atherosclerotic plaques (52, 53). Neovessels are already established in the early phase of the atherosclerotic process and mainly originate from angiogenesis out of the vasa vasorum (53). Such neovessels are thin-walled, more fragile and may function as entrance for erythrocytes, lipids and inflammatory cells (35). As example, neovessel density is more prominent at sites infiltrated by macrophages and lymphocytes (53). Neovessels exhibit inadequate endothelial integrity, making them susceptible for microvascular leakage, which is thought to induce intraplaque hemorrhage (54). Accumulation of erythrocytes is associated with lesion instability and necrotic core expansion (52). Therefore, intraplaque hemorrhage may promote inflammation.
3.4 Microcalcifications
Atherosclerotic calcification is initiated within areas of inflammation (55). Serial in vivo imaging in apoE−/− mice showed that inflammation precedes osteogenic activity and that the initially formed crystals colocalize with macrophages (56). Proposed mechanisms of calcification include the nucleation of necrotic debris into calcium phosphate crystals, reduced activity of inhibitors of vascular calcification and transdifferentiation of intraplaque vascular smooth muscle cells and circulating hematopoietic stem cells into an osteo-, and chondrogenesis phenotype (55, 57). In turn, calcium phosphate crystals have shown to induce a pro-inflammatory response by macrophages (58). This suggests a positive feedback loop between inflammation and calcification. The calcium phosphate crystals congregate into microcalcifications. These microcalcifications, if present in the fibrous cap, may cause microfractures that could destabilize the atherosclerotic plaque (59). Therefore, presence of microcalcifications may refer to a more vulnerable phase in the progression of atherosclerotic plaque within regions of inflammation. Whereas increasing density is thought to reflect a stabilizing process (55).
4 Invasive imaging
4.1 Coronary angiography
Invasive coronary angiography has established itself as a reference standard for the assessment of coronary artery disease (60). It provides a two-dimensional representation of the coronary lumina, by injecting contrast media and performing different radiographic projections, with minimal information on the vessel wall. Coronary angiography is able to identify, albeit suboptimally, the presence of calcification and thrombus. Calcified lesions can be recognized as apparent radiopacities before contrast injection (61). Thrombus can be determined by contrast filling defects and intraluminal lucencies on the “luminogram” (62). The application of other intracoronary diagnostic tools offers the opportunity to look beyond luminal dimensions to identify previously indiscernible lesions.
Thermography was introduced in the early 2000s as an alternative tool to detect coronary artery wall inflammation (63). It was suggested that temperature heterogeneity could identify high-risk lesions. “Hot plaque”, was supposed to reflect the higher metabolic rate of inflammatory cells. However, intracoronary thermography could not meet its expectations (63). In vivo experiments showed that intracoronary thermistors could not detect subtle changes in temperature during substantial influence of pressure, cardiac motion and coronary blood flow (64). Thereafter, it fell in oblivion. Notwithstanding, contemporary imaging techniques do have the ability to target inflammation.
4.2 Intravascular ultrasound (IVUS)
Novel high-definition IVUS may reach an axial resolution of approximately 40–60 µm using high-frequency ultrasound signals (60 MHz) (65). IVUS can differentiate between various plaque components, since calcified plaques are brighter with acoustic shadowing, while lipid-rich plaques appear less echo dense. Furthermore, IVUS can evaluate serial changes in coronary atheroma, to measure for example the effect of statins or PCSK9 inhibitors on atheroma volume (66–69). Using spectral analysis of the “backscattered” ultrasound signals, IVUS offers the opportunity to estimate plaque composition, so-called virtual histology IVUS (VH-IVUS). VH-IVUS has been used to differentiate fibrous plaque, fibrofatty plaque, necrotic core and calcium (Figure 3), which correlates with histologic samples (70). Moreover, serial changes in plaque morphology and pharmacological effects can be identified with VH-IVUS. For example, the “Integrated Biomarker and Imaging Study 2” (IBIS-2) trial showed that darapladib, a “lipoprotein-associated phospholipase A2 inhibitor”, prevented necrotic core expansion after 12 months (71). However, not all studies could confirm the accuracy of VH-IVUS. Necrotic core determined by VH-IVUS did not correlate with histology within a porcine model (72). Therefore, concerns about the validity remain.
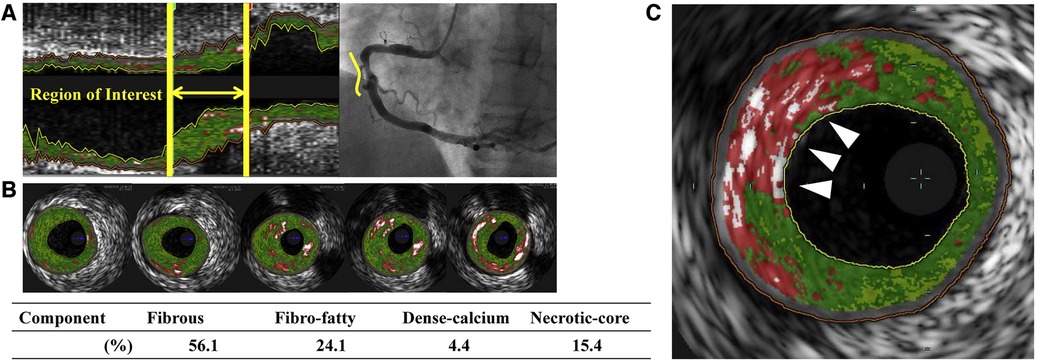
Figure 3. Virtual histology intravascular ultrasound (VH-IVUS) image of atherosclerotic plaque. Based on the reflected ultrasound signals, VH-IVUS automatically provides a colorized tissue map of plaque composition: fibrous (dark green), fibrofatty (light green), calcified (white) and necrotic core (red). (A) Longitudinal VH-IVUS image of an atherosclerotic lesion in the right coronary artery. (B) Cross-sectional images within the region of interest showing different tissue compositions. (C) A thin-cap fibroatheroma (TCFA), characterized as a necrotic-core rich lesion without a clear overlying fibrous cap (arrows). Reused with permission from Kuroda et al. (126).
IVUS is less suited to detect microcytic or molecular factors of inflammation, such as macrophage accumulation or cholesterol crystals, owing to its relatively low resolution (73). However, high-risk plaque features detected by IVUS seem to be positively correlated with circulating inflammatory biomarkers, reflecting higher inflammatory activity (74–77). Within the “European Collaborative Project on Inflammation and Vascular Wall Remodeling in Atherosclerosis—Intravascular Ultrasound” (ATHEROREMO-IVUS) study, higher plaque burden and TCFA lesions were associated with higher levels of circulating tumor necrosis factor alpha and lower levels of circulating interleukin-10 (74). Furthermore, lesions with a plaque burden of ≥70% or TCFA were independently associated with higher rate of major adverse cardiac events within the same study (78).
In addition to tissue characterization, mechanical stress can affect coronary arteries (79). It is feasible that plaque rupture occurs at a location subject to higher mechanical stress. Mechanical strain refers to the tensile stress caused by the pulsatile intravascular blood pressure, whereas wall shear stress results from the tangential component of shearing deformation from blood flow (79). Mechanical strain can be assessed by using the displacement of radiofrequent IVUS signals at two different intracoronary pressures. This technique is called palpography (80). Patients presenting with ACS have more high strain spots than patients with CCS (80). Furthermore, the number of high strain spots seemed positively correlated with levels of hsCRP (80). Another in vivo study using Yucatan minipigs showed that regions with high strain levels were associated with presence of macrophage (81). However, within “The Providing Regional Observations to Study Predictors of Events in the Coronary Tree” (PROSPECT) trial, no difference was found in strain values between thin- and thick-cap fibroatheroma. They could not confirm the correlation between high strain spots and hsCRP in humans (82). Therefore, the diagnostic value of palpography remains uncertain.
Although smaller in magnitude than mechanical strain, wall shear stress is receiving increasing attention because of its biomechanical relevance. Low wall shear stress acts as a pro-inflammatory and pro-atherogenic stimulus on endothelial cells (79). A three-dimensional reconstruction of the coronary artery lumen is required, which also can be obtained using coronary angiography in combination with IVUS or optical coherence tomography (OCT). Thereafter, wall shear stress maps can be constructed using coronary geometries and computational fluid dynamics calculations (79). Unfortunately, results from in vivo studies on the correlation of wall shear stress and plaque progression remain scarce and conflicting. Both low and high wall shear stress have been associated with atherosclerosis and inflammation (79). Therefore, more clinical studies are needed to explore its use.
4.3 Near-infrared spectroscopy (NIRS)
IVUS can be combined with near-infrared spectroscopy (NIRS), which projects near-infrared light on the coronary wall, after which the reflected light is analyzed. Since cholesterol has unique features in the wavelength region, NIRS can be applied to characterize lipid-rich plaque, expressed by the lipid core burden index (LCBI) (83, 84). This index is calculated as the number of pixels with a probability of lipid core plaque > 0.6 divided by the total number of pixels and multiplied by 1,000. The MaxLCBI4mm is often used to detect the presence of a large lipid pool, which is the maximum LCBI value for any 4-mm segment (85). The PROSPECT II study showed that highly lipidic lesions with a MaxLCBI4mm ≥ 324.7 were independently associated with future cardiac events (86). Moreover, risk of non-culprit major adverse cardiovascular events (MACE) increased significantly for each 100-unit increase in MaxLCBI4mm in the “Lipid Rich Plaque” (LRP) study (87). NIRS confirms the crucial role lipids fulfil in the development of cardiovascular events and NIRS can be used to assess response to medical therapy, primarily lipid-lowering therapies (88). However, NIRS is unable to identify crystallization of cholesterol and lacks the ability to differentiate between inflamed or non-inflamed lesions. Furthermore, no association between LCBI and inflammatory biomarkers have been found so far (89).
A novel OCT-NIRS catheter is being developed to provide simultaneous microstructural and compositional imaging (Figure 4) (90). The superior resolution and characteristics of OCT could overcome some limitations inherent to IVUS imaging, as discussed in the next paragraph. A first-in-human study using OCT-NIRS is ongoing (NCT05241665).
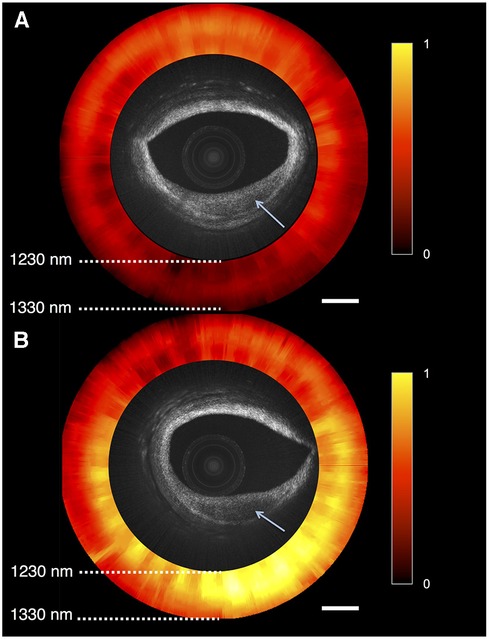
Figure 4. Optical coherence tomography near-infrared spectroscopy (OCT-NIRS) of cadaver coronary artery. Combinded OCT and NIRS (red and yellow circle) imaging of 2 lesions of cadaver coronary artery, showing reduced backscattering (arrows). (A) Lesion with low amounts of lipids, compatible with fibrotic tissue, as it is is shown in red. (B) Lipid-rich plaque displayed as yellow area. Reprinted with permission from Hoang et al. (90) © The Optical Society.
4.4 Optical coherence tomography (OCT)
OCT can provide microstructural images up to a maximal axial resolution of 10 µm at the cost of penetration depth, compared with IVUS (1–2 mm vs. 10 mm). The high resolution not only enables more detailed visualization of calcified nodules, thrombi, TCFAs, plaque erosions and ruptures, but also cholesterol crystal accumulation, macrophages and microvessels (Figure 5) (91, 92).
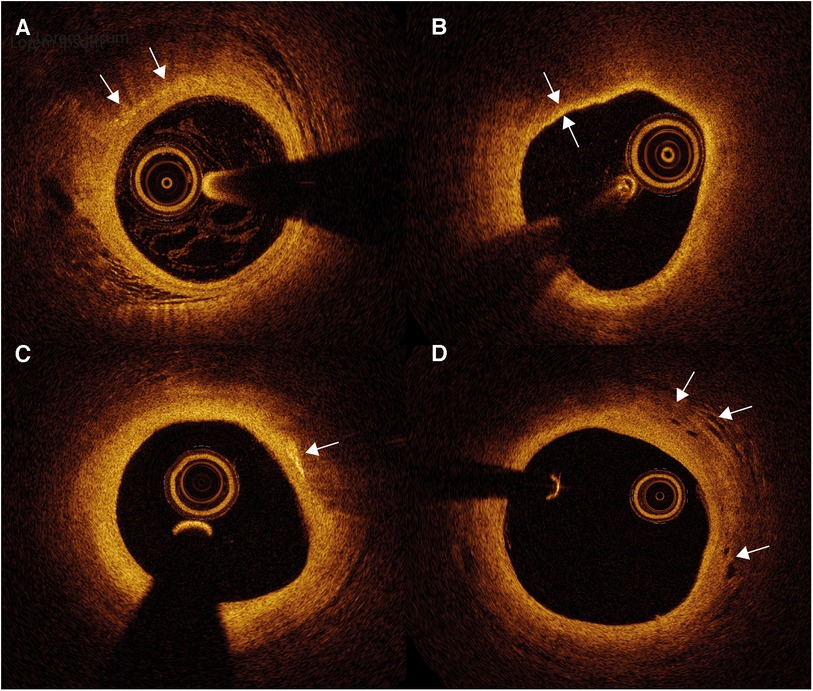
Figure 5. Optical coherence tomography (OCT) images of atherosclerotic plaque and inflammation markers. (A) Macrophages appear as signal-rich bright spots with a signal-poor region below (arrows). (B) Thin-cap fibroatheroma are identified as an atherosclerotic plaque covered by a fibrous cap of <65 µm (between arrows). (C) Cholesterol crystals appear as thin and linear structures with high signal intensity (arrow), often localized nearby lipid-rich plaque. (D) Intraplaque neovessels can be identified as well delineated signal-poor voids (arrows).
Macrophages scatter light efficiently, which creates signal-rich regions called bright spots with a cast shadow behind (93). (Figure 5A) While OCT has not been proven to distinguish between active and inactive macrophages (94), bright spots do have a strong correlation with inflammation measured by hsCRP (95). Bright spot density is significantly higher in lipid plaques compared to fibrous plaques and plaques with TCFA show a trend toward higher bright spot density (95). Moreover, bright spot density is also significantly higher in plaques with rupture than those without (95). These findings imply the ability of OCT to provide an overall estimate of macrophage accumulation. Furthermore, presence of OCT-defined macrophage accumulation is associated with adverse clinical outcome. In the “Relationship Between OCT Coronary Plaque Morphology and Clinical Outcome” (CLIMA) study, presence of macrophage accumulation in native left anterior descending coronary artery was associated with more clinical events, especially in the copresence of other high-risk plaque features including a thin fibrous cap and a large lipid arc (96).
Cholesterol crystals appear as thin, linear structures with high signal intensity, often localized nearby lipid-rich plaque (Figure 5C). It is suggested that needle-shaped cholesterol crystals could perforate the fibrous cap, causing plaque instability (93).
Intraplaque neovessels can be identified as well delineated signal-poor voids, which can be followed in consecutive frames (Figure 5D) (93). An ex vivo OCT study showed that coronary atherosclerotic plaques with neovessels were accompanied with greater luminal narrowing (97). Subsequent neovessel rupture could induce intraplaque hemorrhage. However, studies about neovessels and neovessel rupture on OCT imaging remain scarce.
OCT is the only imaging modality with sufficient spatial resolution to adequately measure fibrous cap thickness (Figure 5B). Numerous prospective and retrospective studies have demonstrated an association between OCT-identified TCFA and clinical outcome, whether or not in combination with other features of plaque instability (96, 98). The fibrous cap thickness cutoff to define TCFA differs between studies, as it has been suggested that the 65 um cutoff obtained in histopathological studies should be enlarged to adjust for potential tissue shrinkage during histopathological tissue processing (93). Nevertheless, Jiang et al. found a similar optimal cutoff of 66.7 um to distinguish lesions at higher risk of causing events (98). In this study, 883 patients were included, all 3 main epicardial vessels were scanned and follow-up lasted up to 4 years. OCT can differentiate whether ACS arises from rupture of the fibrous cap or endothelial injury with an intact fibrous cap, i.e. plaque erosion. These distinct patterns might have different underlying pathobiologies. The presence of OCT-identified culprit plaque rupture is associated with lower levels of T-cells but higher levels of effector molecules involved in the innate immune response compared to ACS with intact fibrous caps (99). This may indicate that the adaptive immune system plays an important role in inducing endothelial erosion. In proteomics analysis, patients with ruptured plaques also had a higher inflammatory response and more MACE during 2 years of follow-up (100).
OCT allows to evaluate change in high-risk plaque characteristics (101). The “High-Resolution Assessment of Coronary Plaques in a Global Evolocumab Randomized Study” (HUYGENS) showed that intensive lipid-lowering therapy with high-dose statins and evolocumab increased minimum fibrous cap thickness and decreased the macrophage index on serial OCT. The combination of statin and evolocumab resulted in more favorable changes than statin therapy alone (102).
4.4.1 Micro optical coherence tomography (µOCT)
In 2011, micro-OCT (μOCT) was introduced to improve the resolution of OCT imaging systems to achieve an axial resolution of 1–2 μm, which is another ten-fold improvement (103). Therefore, μOCT is capable of visualizing independent cells and subcellular features. Moreover, μOCT is able to differentiate between multiple inflammatory cells, including leukocytes, monocytes and macrophages.
An intravascular μOCT catheter suitable for in vivo imaging was recently introduced. This device is able to display a wide range of cells and subcellular structures, including leukocytes, macrophages, smooth muscle cells, cholesterol crystals and platelets within rabbit aortae in vivo and human cadaver coronary arteries (104). To acquire high-resolution images, current μOCT imaging systems emit light with 800 nm wavelength. The use of a shorter wavelength compared to standard OCT might be at the expense of penetration depth, which is already a disadvantage compared to IVUS (105). Nevertheless, μOCT has the potential to visualize local inflammatory processes in vivo such as leukocyte adhesion, foam cell formation or inflammatory cells surrounding cholesterol crystals on microscopic level. More clinical studies are needed to explore further utilities.
4.5 Near-infrared fluorescence (NIRF)
Near-infrared fluorescence (NIRF) imaging is an emerging technique, allowing the visualization of molecular processes within the atherosclerotic plaque. It uses imaging agents which bind to specific targets, including protease activity, LDL, fibrin deposition and microcalcifications (106–108). These imaging agents consist of an fluorochrome, conjugated to an antibody, molecule or peptide. After injection, a NIRF-catheter is advanced within the coronary artery. A continuous wave laser diode emits excitational light within a 650 to 950 nm window (NIR spectral region) to stimulate the fluorophores. The subsequent fluorescence emission is collected and filtered within the NIRF-catheter (109).
Protease-activatable fluorophores have been developed to visualize enzymatic activity. At baseline, fluorophores emittance is quenched, but increases significantly after cleavage (110). Enzymatically active cathepsins, detected by NIRF, seem to colocalize with cathepsins and macrophages on immunohistochemistry in animal and human atheromata (107, 111, 112). Matrix metalloproteinase-specific fluorophores have shown similar results on NIRF imaging (113, 114). Indocyanine green (ICG) is an imaging agent, which can directly visualize macrophages. After injection, ICG is internalized by macrophages and foam cells by binding to albumin or LDL (108). In a recent study, the ICG NIRF signal, measured in freshly isolated carotid plaques, was highest in the most stenotic area. Subsequent histopathological analyses established that ICG targeted endothelial abnormalities, such as disrupted fibrous caps and areas of neovascularization. ICG concentrated on zones of plaque lipids, macrophages and intraplaque hemorrhage (115). Furthermore, fluorophores have been developed to target fibrin deposition, activated factor XIIIa or thrombin activity to assess thrombosis. Validation of these fluorophores mostly rely on non-invasive NIRF imaging techniques (116–118). However, intravascular NIRF has been able to detect fibrin deposition overlying stent struts in rabbits (119).
Hybridization of intravascular molecular and structural imaging could potentially allow further study of the pathophysiological mechanisms of arterial plaques (120, 121). Both NIRF-OCT and NIRF-IVUS are being developed. The dual-of modality OCT and NIRF, can detect fluorescence from naturally occurring molecules, called near-infrared autofluorescence (NIRAF). NIRAF is elevated in advanced necrotic core-containing lesions and is associated with a high-risk morphological plaque phenotype (Figure 6) (122, 123). Interestingly, NIRAF elevation is specific to plaques with macrophage accumulations, as shown by OCT (122). However, the converse is not true, since many areas with elevated macrophage accumulation on OCT were NIRAF negative. This could be explained by low sensitivity/high specificity of NIRAF to macrophage accumulation, or by the concept of different macrophage phenotypes. The underlying molecular and chemical mechanisms that produce NIRAF are not yet fully understood.
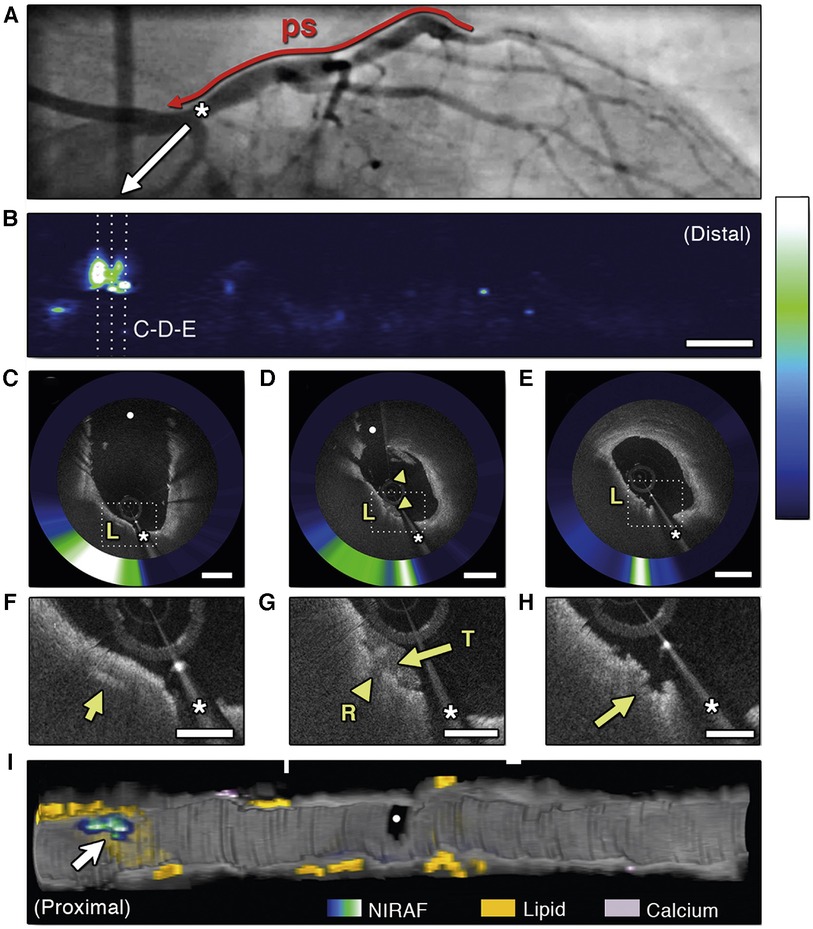
Figure 6. Optical coherence tomography near-infrared autofluorescence (OCT-NIRAF) imaging of a ruptured thin-cap fibroatheroma. (A) Coronary angiography of the left circumflex coronary artery. (B) 2-dimensional map of NIRAF signal. (C–E) Cross sectional OCT—NIRAF images showing a rupture of a thin fibrous cap covered with a small thrombus. The rupture site shows high NIRAF. (F,G) Magnified views revealing a cholesterol crystal (F, arrow), thrombus (G, arrows) and the rupture site (H, arrow). All colocalized with elevated NIRAF. (I) 3-dimensional rendered map demonstrating that the high NIRAF signal appears within regions containing high amount of lipids (arrow). The asterisk (*) corresponds with catheter artefact. L, lipid; R, rupture site; T, thrombus. Reused with permission from Ughi et al. (122).
5 Discussion
Atherosclerotic cardiovascular disease is a complex chronic inflammatory and fibroproliferative process fueled by atherogenic lipoproteins. This implies the requirement of precise diagnostic tools and targeted treatment strategies (124). Systemic inflammation has emerged as a therapeutic target to reduce cardiovascular events (16, 17). Intracoronary imaging allows the judgement of disease state of atheromata and identification of high-risk lesions. Given the inherent characteristics of different imaging modalities, they all facilitate distinctive insights in the inflammatory pathobiology of atherosclerosis (Table 1). IVUS gives a good “overview” of plaque burden and plaque composition. A higher plaque burden is associated with elevated systemic inflammation, reflected by increased pro-inflammatory biomarkers. However, it is unable to directly visualize the inflammatory process. NIRS provides a chemical analysis of the arterial wall but lacks the ability to detect inflammatory markers or cholesterol crystals. OCT is able to detect and measure TCFA, macrophages, neovessels and cholesterol crystals. Moreover, additional increase of resolution with µOCT allows further detection of individual cells and subcellular substances. NIRF imaging displays molecular- and inflammatory activity by targeting specific molecules, thereby allowing detection of early- and advanced stages of atherosclerosis.
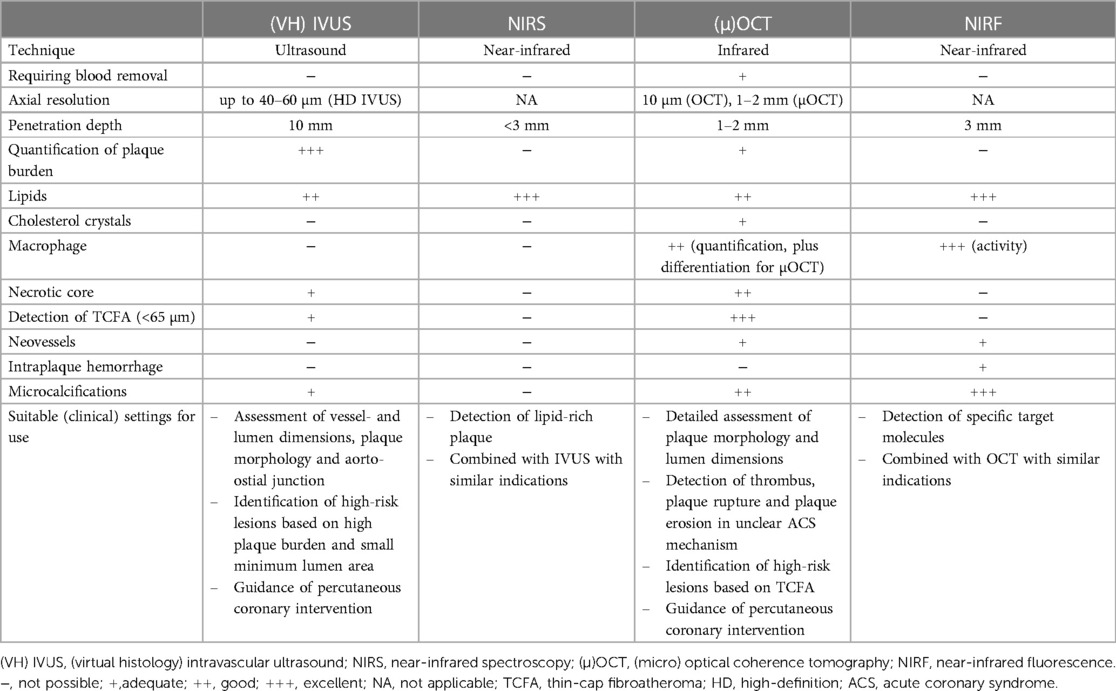
Table 1. Characteristics of the imaging modalities and their ability to display high-risk features and inflammation markers.
Lesions with high-risk features on intracoronary imaging have shown to be predictive of MACE (125). However, current positive predictive value is still moderate. Novel hybrid modalities, in particular NIRF-OCT, could provide complementary morphological and functional imaging, further improving the diagnostic performance and prognostic stratification. In the near future, they may identify high-risk lesions of clinical value to revascularize or optimize medical therapy. For the moment, invasive imaging mainly has clinical indications (Table 1), but they can simultaneously identify more specific inflammatory characteristics, which strengthens the case for inflammation-targeted therapies. Moreover, hallmarks of inflammation and high-risk plaque are useful surrogate endpoints to assess the potency of medical therapy.
6 Conclusion
Contemporary and future intracoronary imaging techniques allow the identification of inflammatory markers within atherosclerotic plaque. They assess the biochemical composition and the underlying pathophysiology. Furthermore, they serve as a mechanism to evaluate drug efficacy. Conscientious implementation may allow the development of patient tailored treatment strategies and improve patient outcome.
Author contributions
JL: Writing – original draft, Writing – review & editing. FM: Writing – review & editing. NM: Writing – review & editing. TO: Writing – review & editing. PD: Writing – review & editing. RV: Writing – review & editing. DP: Writing – review & editing. NvR: Writing – review & editing. HG-G: Writing – review & editing. JC: Writing – review & editing. SEM: Writing – review & editing. R-JvG: Writing – review & editing.
Funding
The author(s) declare that no financial support was received for the research, authorship, and/or publication of this article.
Conflict of interest
PD: received research grants and speaker fees from Abbott Vascular; research grants, speaker fees and consultancy fees from Philips; and research grants from AstraZeneca. NvR: research grants from Abbott, Philpis, Biotronik and Medtronic. Speaker fee: Abbott, Bayer, Rainmed, Microport. HG-G: receives consulting fees from Boston Scientific, Abbott and institutional grants from Phillips, Biotronik, Abbott, MedAlliance, Corflow, Chiesi and Medis. JC: Advisory board Amgen en Novo Nordisk. Received research grants from ZonMw. R-JvG: reported grants and personal fees from Boston Scientific, grants and personal fees from Abbott Vascular, grants and personal fees from Astra Zeneca, grants and personal fees from Amgen, grants from InfraRedx.
The other authors declare that the research was conducted in the absence of any commercial or financial relationships that could be construed as a potential conflict of interest.
Publisher's note
All claims expressed in this article are solely those of the authors and do not necessarily represent those of their affiliated organizations, or those of the publisher, the editors and the reviewers. Any product that may be evaluated in this article, or claim that may be made by its manufacturer, is not guaranteed or endorsed by the publisher.
References
1. Vasan RS, Enserro DM, Xanthakis V, Beiser AS, Seshadri S. Temporal trends in the remaining lifetime risk of cardiovascular disease among middle-aged adults across 6 decades: the framingham study. Circulation. (2022) 145(17):1324–38. doi: 10.1161/CIRCULATIONAHA.121.057889
2. Roth GA, Huffman MD, Moran AE, Feigin V, Mensah GA, Naghavi M, et al. Global and regional patterns in cardiovascular mortality from 1990 to 2013. Circulation. (2015) 132(17):1667–78. doi: 10.1161/CIRCULATIONAHA.114.008720
3. Global, regional, and national age-sex specific all-cause and cause-specific mortality for 240 causes of death, 1990–2013: a systematic analysis for the global burden of disease study 2013. Lancet. (2015) 385(9963):117–71. doi: 10.1016/S0140-6736(14)61682-2
4. Weber C, Noels H. Atherosclerosis: current pathogenesis and therapeutic options. Nat Med. (2011) 17(11):1410–22. doi: 10.1038/nm.2538
5. Naghavi M, Libby P, Falk E, Casscells SW, Litovsky S, Rumberger J, et al. From vulnerable plaque to vulnerable patient: a call for new definitions and risk assessment strategies: part I. Circulation. (2003) 108(14):1664–72. doi: 10.1161/01.CIR.0000087480.94275.97
6. Falk E. Pathogenesis of atherosclerosis. J Am Coll Cardiol. (2006) 47(8 Suppl):C7–12. doi: 10.1016/j.jacc.2005.09.068
7. Libby P, Ridker PM, Hansson GK. Progress and challenges in translating the biology of atherosclerosis. Nature. (2011) 473(7347):317–25. doi: 10.1038/nature10146
8. Prati F, Uemura S, Souteyrand G, Virmani R, Motreff P, Di Vito L, et al. OCT-based diagnosis and management of STEMI associated with intact fibrous cap. JACC Cardiovasc Imaging. (2013) 6(3):283–7. doi: 10.1016/j.jcmg.2012.12.007
9. Fuster V, Moreno PR, Fayad ZA, Corti R, Badimon JJ. Atherothrombosis and high-risk plaque: part I: evolving concepts. J Am Coll Cardiol. (2005) 46(6):937–54. doi: 10.1016/j.jacc.2005.03.074
10. Navarese EP, Robinson JG, Kowalewski M, Kolodziejczak M, Andreotti F, Bliden K, et al. Association between baseline LDL-C level and total and cardiovascular mortality after LDL-C lowering: a systematic review and meta-analysis. JAMA. (2018) 319(15):1566–79. doi: 10.1001/jama.2018.2525
11. Ference BA, Cannon CP, Landmesser U, Lüscher TF, Catapano AL, Ray KK. Reduction of low density lipoprotein-cholesterol and cardiovascular events with proprotein convertase subtilisin-kexin type 9 (PCSK9) inhibitors and statins: an analysis of FOURIER, SPIRE, and the cholesterol treatment trialists collaboration. Eur Heart J. (2018) 39(27):2540–5. doi: 10.1093/eurheartj/ehx450
12. Ridker PM. Residual inflammatory risk: addressing the obverse side of the atherosclerosis prevention coin. Eur Heart J. (2016) 37(22):1720–2. doi: 10.1093/eurheartj/ehw024
13. Mohammadnia N, Opstal TSJ, El Messaoudi S, Bax WA, Cornel JH. An update on inflammation in atherosclerosis: how to effectively treat residual risk. Clin Ther. (2023) 45(11):1055–9. doi: 10.1016/j.clinthera.2023.08.016
14. Ridker PM, Danielson E, Fonseca FA, Genest J, Gotto AM Jr., Kastelein JJ, et al. Reduction in C-reactive protein and LDL cholesterol and cardiovascular event rates after initiation of rosuvastatin: a prospective study of the JUPITER trial. Lancet. (2009) 373(9670):1175–82. doi: 10.1016/S0140-6736(09)60447-5
15. Ridker PM, Cannon CP, Morrow D, Rifai N, Rose LM, McCabe CH, et al. C-reactive protein levels and outcomes after statin therapy. N Engl J Med. (2005) 352(1):20–8. doi: 10.1056/NEJMoa042378
16. Ridker PM, Everett BM, Thuren T, MacFadyen JG, Chang WH, Ballantyne C, et al. Antiinflammatory therapy with canakinumab for atherosclerotic disease. N Engl J Med. (2017) 377(12):1119–31. doi: 10.1056/NEJMoa1707914
17. Nidorf SM, Fiolet ATL, Mosterd A, Eikelboom JW, Schut A, Opstal TSJ, et al. Colchicine in patients with chronic coronary disease. N Engl J Med. (2020) 383(19):1838–47. doi: 10.1056/NEJMoa2021372
18. Biasucci LM, Pedicino D, Liuzzo G. Promises and challenges of targeting inflammation to treat cardiovascular disease: the post-CANTOS era. Eur Heart J. (2020) 41(23):2164–7. doi: 10.1093/eurheartj/ehz586
19. Mintz GS. Clinical utility of intravascular imaging and physiology in coronary artery disease. J Am Coll Cardiol. (2014) 64(2):207–22. doi: 10.1016/j.jacc.2014.01.015
20. Raggi P, Genest J, Giles JT, Rayner KJ, Dwivedi G, Beanlands RS, et al. Role of inflammation in the pathogenesis of atherosclerosis and therapeutic interventions. Atherosclerosis. (2018) 276:98–108. doi: 10.1016/j.atherosclerosis.2018.07.014
21. Mundi S, Massaro M, Scoditti E, Carluccio MA, van Hinsbergh VWM, Iruela-Arispe ML, et al. Endothelial permeability, LDL deposition, and cardiovascular risk factors-a review. Cardiovasc Res. (2018) 114(1):35–52. doi: 10.1093/cvr/cvx226
22. Kim KW, Ivanov S, Williams JW. Monocyte recruitment, specification, and function in atherosclerosis. Cells. (2020) 10(1). doi: 10.3390/cells10010015
23. Johnson JL. Metalloproteinases in atherosclerosis. Eur J Pharmacol. (2017) 816:93–106. doi: 10.1016/j.ejphar.2017.09.007
24. Newby AC. Dual role of matrix metalloproteinases (matrixins) in intimal thickening and atherosclerotic plaque rupture. Physiol Rev. (2005) 85(1):1–31. doi: 10.1152/physrev.00048.2003
25. Shankman LS, Gomez D, Cherepanova OA, Salmon M, Alencar GF, Haskins RM, et al. KLF4-dependent Phenotypic modulation of smooth muscle cells has a key role in atherosclerotic plaque pathogenesis. Nat Med. (2015) 21(6):628–37. doi: 10.1038/nm.3866
26. Bennett MR, Sinha S, Owens GK. Vascular smooth muscle cells in atherosclerosis. Circ Res. (2016) 118(4):692–702. doi: 10.1161/CIRCRESAHA.115.306361
27. Dinarello CA, Simon A, van der Meer JW. Treating inflammation by blocking interleukin-1 in a broad spectrum of diseases. Nat Rev Drug Discov. (2012) 11(8):633–52. doi: 10.1038/nrd3800
28. Ridker PM. From C-reactive protein to interleukin-6 to interleukin-1: moving upstream to identify novel targets for atheroprotection. Circ Res. (2016) 118(1):145–56. doi: 10.1161/CIRCRESAHA.115.306656
29. Duewell P, Kono H, Rayner KJ, Sirois CM, Vladimer G, Bauernfeind FG, et al. NLRP3 Inflammasomes are required for atherogenesis and activated by cholesterol crystals. Nature. (2010) 464(7293):1357–61. doi: 10.1038/nature08938
30. Rajamäki K, Lappalainen J, Oörni K, Välimäki E, Matikainen S, Kovanen PT, et al. Cholesterol crystals activate the NLRP3 inflammasome in human macrophages: a novel link between cholesterol metabolism and inflammation. PLoS One. (2010) 5(7):e11765. doi: 10.1371/journal.pone.0011765
31. Bevilacqua MP, Pober JS, Wheeler ME, Cotran RS, Gimbrone MA Jr. Interleukin 1 acts on cultured human vascular endothelium to increase the adhesion of polymorphonuclear leukocytes, monocytes, and related leukocyte cell lines. J Clin Invest. (1985) 76(5):2003–11. doi: 10.1172/JCI112200
32. Dinarello CA. Interleukin-1 in the pathogenesis and treatment of inflammatory diseases. Blood. (2011) 117(14):3720–32. doi: 10.1182/blood-2010-07-273417
33. Glaser R, Selzer F, Faxon DP, Laskey WK, Cohen HA, Slater J, et al. Clinical progression of incidental, asymptomatic lesions discovered during culprit vessel coronary intervention. Circulation. (2005) 111(2):143–9. doi: 10.1161/01.CIR.0000150335.01285.12
34. Little WC, Constantinescu M, Applegate RJ, Kutcher MA, Burrows MT, Kahl FR, et al. Can coronary angiography predict the site of a subsequent myocardial infarction in patients with mild-to-moderate coronary artery disease? Circulation. (1988) 78(5 Pt 1):1157–66. doi: 10.1161/01.CIR.78.5.1157
35. Bentzon JF, Otsuka F, Virmani R, Falk E. Mechanisms of plaque formation and rupture. Circ Res. (2014) 114(12):1852–66. doi: 10.1161/CIRCRESAHA.114.302721
36. Stone GW, Maehara A, Lansky AJ, de Bruyne B, Cristea E, Mintz GS, et al. A prospective natural-history study of coronary atherosclerosis. N Engl J Med. (2011) 364(3):226–35. doi: 10.1056/NEJMoa1002358
37. Huang H, Virmani R, Younis H, Burke AP, Kamm RD, Lee RT. The impact of calcification on the biomechanical stability of atherosclerotic plaques. Circulation. (2001) 103(8):1051–6. doi: 10.1161/01.CIR.103.8.1051
38. Abela GS, Aziz K. Cholesterol crystals cause mechanical damage to biological membranes: a proposed mechanism of plaque rupture and erosion leading to arterial thrombosis. Clin Cardiol. (2005) 28(9):413–20. doi: 10.1002/clc.4960280906
39. Abela GS. Cholesterol crystals piercing the arterial plaque and intima trigger local and systemic inflammation. J Clin Lipidol. (2010) 4(3):156–64. doi: 10.1016/j.jacl.2010.03.003
40. Nishimura S, Ehara S, Hasegawa T, Matsumoto K, Yoshikawa J, Shimada K. Cholesterol crystal as a new feature of coronary vulnerable plaques: an optical coherence tomography study. J Cardiol. (2017) 69(1):253–9. doi: 10.1016/j.jjcc.2016.04.003
41. Dai J, Tian J, Hou J, Xing L, Liu S, Ma L, et al. Association between cholesterol crystals and culprit lesion vulnerability in patients with acute coronary syndrome: an optical coherence tomography study. Atherosclerosis. (2016) 247:111–7. doi: 10.1016/j.atherosclerosis.2016.02.010
42. Gonzalez L, Trigatti BL. Macrophage apoptosis and necrotic core development in atherosclerosis: a rapidly advancing field with clinical relevance to imaging and therapy. Can J Cardiol. (2017) 33(3):303–12. doi: 10.1016/j.cjca.2016.12.010
43. Van Vré EA, Ait-Oufella H, Tedgui A, Mallat Z. Apoptotic cell death and efferocytosis in atherosclerosis. Arterioscler Thromb Vasc Biol. (2012) 32(4):887–93. doi: 10.1161/ATVBAHA.111.224873
44. Elmore S. Apoptosis: a review of programmed cell death. Toxicol Pathol. (2007) 35(4):495–516. doi: 10.1080/01926230701320337
45. Tabas I, Bornfeldt KE. Macrophage phenotype and function in different stages of atherosclerosis. Circ Res. (2016) 118(4):653–67. doi: 10.1161/CIRCRESAHA.115.306256
46. Savill J, Fadok V. Corpse clearance defines the meaning of cell death. Nature. (2000) 407(6805):784–8. doi: 10.1038/35037722
47. Kolodgie FD, Burke AP, Farb A, Gold HK, Yuan J, Narula J, et al. The thin-cap fibroatheroma: a type of vulnerable plaque: the major precursor lesion to acute coronary syndromes. Curr Opin Cardiol. (2001) 16(5):285–92. doi: 10.1097/00001573-200109000-00006
48. Libby P. Molecular bases of the acute coronary syndromes. Circulation. (1995) 91(11):2844–50. doi: 10.1161/01.CIR.91.11.2844
49. Burke AP, Farb A, Malcom GT, Liang YH, Smialek J, Virmani R. Coronary risk factors and plaque morphology in men with coronary disease who died suddenly. N Engl J Med. (1997) 336(18):1276–82. doi: 10.1056/NEJM199705013361802
50. Virmani R, Kolodgie FD, Burke AP, Farb A, Schwartz SM. Lessons from sudden coronary death: a comprehensive morphological classification scheme for atherosclerotic lesions. Arterioscler Thromb Vasc Biol. (2000) 20(5):1262–75. doi: 10.1161/01.ATV.20.5.1262
51. Stary HC, Chandler AB, Dinsmore RE, Fuster V, Glagov S, Insull W Jr., et al. A definition of advanced types of atherosclerotic lesions and a histological classification of atherosclerosis. A report from the committee on vascular lesions of the council on arteriosclerosis, American heart association. Circulation. (1995) 92(5):1355–74. doi: 10.1161/01.CIR.92.5.1355
52. Kolodgie FD, Gold HK, Burke AP, Fowler DR, Kruth HS, Weber DK, et al. Intraplaque hemorrhage and progression of coronary atheroma. N Engl J Med. (2003) 349(24):2316–25. doi: 10.1056/NEJMoa035655
53. Kumamoto M, Nakashima Y, Sueishi K. Intimal neovascularization in human coronary atherosclerosis: its origin and pathophysiological significance. Hum Pathol. (1995) 26(4):450–6. doi: 10.1016/0046-8177(95)90148-5
54. Sluimer JC, Kolodgie FD, Bijnens AP, Maxfield K, Pacheco E, Kutys B, et al. Thin-walled microvessels in human coronary atherosclerotic plaques show incomplete endothelial junctions relevance of compromised structural integrity for intraplaque microvascular leakage. J Am Coll Cardiol. (2009) 53(17):1517–27. doi: 10.1016/j.jacc.2008.12.056
55. Nakahara T, Dweck MR, Narula N, Pisapia D, Narula J, Strauss HW. Coronary artery calcification: from mechanism to molecular imaging. JACC Cardiovasc Imaging. (2017) 10(5):582–93. doi: 10.1016/j.jcmg.2017.03.005
56. Aikawa E, Nahrendorf M, Figueiredo JL, Swirski FK, Shtatland T, Kohler RH, et al. Osteogenesis associates with inflammation in early-stage atherosclerosis evaluated by molecular imaging in vivo. Circulation. (2007) 116(24):2841–50. doi: 10.1161/CIRCULATIONAHA.107.732867
57. Leszczynska A, O'Doherty A, Farrell E, Pindjakova J, O'Brien FJ, O'Brien T, et al. Differentiation of vascular stem cells contributes to ectopic calcification of atherosclerotic plaque. Stem Cells. (2016) 34(4):913–23. doi: 10.1002/stem.2315
58. Nadra I, Mason JC, Philippidis P, Florey O, Smythe CD, McCarthy GM, et al. Proinflammatory activation of macrophages by basic calcium phosphate crystals via protein kinase C and MAP kinase pathways: a vicious cycle of inflammation and arterial calcification? Circ Res. (2005) 96(12):1248–56. doi: 10.1161/01.RES.0000171451.88616.c2
59. Vengrenyuk Y, Carlier S, Xanthos S, Cardoso L, Ganatos P, Virmani R, et al. A hypothesis for vulnerable plaque rupture due to stress-induced debonding around cellular microcalcifications in thin fibrous caps. Proc Natl Acad Sci U S A. (2006) 103(40):14678–83. doi: 10.1073/pnas.0606310103
60. Windecker S, Kolh P, Alfonso F, Collet JP, Cremer J, Falk V, et al. 2014 ESC/EACTS guidelines on myocardial revascularization: the task force on myocardial revascularization of the European society of cardiology (ESC) and the European association for cardio-thoracic surgery (EACTS)Developed with the special contribution of the European association of percutaneous cardiovascular interventions (EAPCI). Eur Heart J. (2014) 35(37):2541–619. doi: 10.1093/eurheartj/ehu278
61. Mintz GS, Popma JJ, Pichard AD, Kent KM, Satler LF, Chuang YC, et al. Patterns of calcification in coronary artery disease. A statistical analysis of intravascular ultrasound and coronary angiography in 1155 lesions. Circulation. (1995) 91(7):1959–65. doi: 10.1161/01.CIR.91.7.1959
62. Chan KH, Ng MK. Is there a role for coronary angiography in the early detection of the vulnerable plaque? Int J Cardiol. (2013) 164(3):262–6. doi: 10.1016/j.ijcard.2012.01.027
63. Nissen SE. Vulnerable plaque and Einstein’s definition of insanity. J Am Coll Cardiol. (2020) 75(12):1383–5. doi: 10.1016/j.jacc.2020.01.043
64. Cuisset T, Beauloye C, Melikian N, Hamilos M, Sarma J, Sarno G, et al. In vitro and in vivo studies on thermistor-based intracoronary temperature measurements: effect of pressure and flow. Catheter Cardiovasc Interv. (2009) 73(2):224–30. doi: 10.1002/ccd.21780
65. Garcia-Guimaraes M, Antuña P, De la Cuerda F, Maruri-Sanchez R, Cuesta J, Bastante T, et al. High-definition IVUS versus OCT to assess coronary artery disease and results of stent implantation. JACC Cardiovasc Imaging. (2020) 13(2 Pt 1):519–21. doi: 10.1016/j.jcmg.2019.08.019
66. Nicholls SJ, Puri R, Anderson T, Ballantyne CM, Cho L, Kastelein JJP, et al. Effect of evolocumab on coronary plaque composition. J Am Coll Cardiol. (2018) 72(17):2012–21. doi: 10.1016/j.jacc.2018.06.078
67. Nissen SE, Tuzcu EM, Schoenhagen P, Brown BG, Ganz P, Vogel RA, et al. Effect of intensive compared with moderate lipid-lowering therapy on progression of coronary atherosclerosis: a randomized controlled trial. JAMA. (2004) 291(9):1071–80. doi: 10.1001/jama.291.9.1071
68. Nissen SE, Nicholls SJ, Sipahi I, Libby P, Raichlen JS, Ballantyne CM, et al. Effect of very high-intensity statin therapy on regression of coronary atherosclerosis: the ASTEROID trial. JAMA. (2006) 295(13):1556–65. doi: 10.1001/jama.295.13.jpc60002
69. Nicholls SJ, Ballantyne CM, Barter PJ, Chapman MJ, Erbel RM, Libby P, et al. Effect of two intensive statin regimens on progression of coronary disease. N Engl J Med. (2011) 365(22):2078–87. doi: 10.1056/NEJMoa1110874
70. Groves EM, Seto AH, Kern MJ. Invasive testing for coronary artery disease: FFR, IVUS, OCT, NIRS. Heart Fail Clin. (2016) 12(1):83–95. doi: 10.1016/j.hfc.2015.08.007
71. Serruys PW, García-García HM, Buszman P, Erne P, Verheye S, Aschermann M, et al. Effects of the direct lipoprotein-associated phospholipase A(2) inhibitor darapladib on human coronary atherosclerotic plaque. Circulation. (2008) 118(11):1172–82. doi: 10.1161/CIRCULATIONAHA.108.771899
72. Thim T, Hagensen MK, Wallace-Bradley D, Granada JF, Kaluza GL, Drouet L, et al. Unreliable assessment of necrotic core by virtual histology intravascular ultrasound in porcine coronary artery disease. Circ Cardiovasc Imaging. (2010) 3(4):384–91. doi: 10.1161/CIRCIMAGING.109.919357
73. Brown AJ, Obaid DR, Costopoulos C, Parker RA, Calvert PA, Teng Z, et al. Direct comparison of virtual-histology intravascular ultrasound and optical coherence tomography imaging for identification of thin-cap fibroatheroma. Circ Cardiovasc Imaging. (2015) 8(10):e003487. doi: 10.1161/CIRCIMAGING.115.003487
74. Battes LC, Cheng JM, Oemrawsingh RM, Boersma E, Garcia-Garcia HM, de Boer SP, et al. Circulating cytokines in relation to the extent and composition of coronary atherosclerosis: results from the ATHEROREMO-IVUS study. Atherosclerosis. (2014) 236(1):18–24. doi: 10.1016/j.atherosclerosis.2014.06.010
75. Sawada T, Shite J, Shinke T, Watanabe S, Otake H, Matsumoto D, et al. Relationship between high sensitive C-reactive protein and coronary plaque component in patients with acute coronary syndrome: virtual histology study. J Cardiol. (2006) 48(3):141–50. PMID: 17007239.17007239
76. Koskinas KC, Zaugg S, Yamaji K, García-García HM, Taniwaki M, Klingenberg R, et al. Changes of coronary plaque composition correlate with C-reactive protein levels in patients with ST-elevation myocardial infarction following high-intensity statin therapy. Atherosclerosis. (2016) 247:154–60. doi: 10.1016/j.atherosclerosis.2016.02.015
77. Hong YJ, Jeong MH, Choi YH, Cho SH, Hwang SH, Ko JS, et al. Relation between high-sensitivity C-reactive protein and coronary plaque components in patients with acute coronary syndrome: virtual histology-intravascular ultrasound analysis. Korean Circ J. (2011) 41(8):440–6. doi: 10.4070/kcj.2011.41.8.440
78. Cheng JM, Garcia-Garcia HM, de Boer SP, Kardys I, Heo JH, Akkerhuis KM, et al. In vivo detection of high-risk coronary plaques by radiofrequency intravascular ultrasound and cardiovascular outcome: results of the ATHEROREMO-IVUS study. Eur Heart J. (2014) 35(10):639–47. doi: 10.1093/eurheartj/eht484
79. Gijsen F, Katagiri Y, Barlis P, Bourantas C, Collet C, Coskun U, et al. Expert recommendations on the assessment of wall shear stress in human coronary arteries: existing methodologies, technical considerations, and clinical applications. Eur Heart J. (2019) 40(41):3421–33. doi: 10.1093/eurheartj/ehz551
80. Schaar JA, Regar E, Mastik F, McFadden EP, Saia F, Disco C, et al. Incidence of high-strain patterns in human coronary arteries: assessment with three-dimensional intravascular palpography and correlation with clinical presentation. Circulation. (2004) 109(22):2716–9. doi: 10.1161/01.CIR.0000131887.65955.3B
81. de Korte CL, Sierevogel MJ, Mastik F, Strijder C, Schaar JA, Velema E, et al. Identification of atherosclerotic plaque components with intravascular ultrasound elastography in vivo: a Yucatan pig study. Circulation. (2002) 105(14):1627–30. doi: 10.1161/01.CIR.0000014988.66572.2E
82. Brugaletta S, Garcia-Garcia HM, Serruys PW, Maehara A, Farooq V, Mintz GS, et al. Relationship between palpography and virtual histology in patients with acute coronary syndromes. JACC Cardiovasc Imaging. (2012) 5(3 Suppl):S19–27. doi: 10.1016/j.jcmg.2011.02.026
83. Gardner CM, Tan H, Hull EL, Lisauskas JB, Sum ST, Meese TM, et al. Detection of lipid core coronary plaques in autopsy specimens with a novel catheter-based near-infrared spectroscopy system. JACC Cardiovasc Imaging. (2008) 1(5):638–48. doi: 10.1016/j.jcmg.2008.06.001
84. Caplan JD, Waxman S, Nesto RW, Muller JE. Near-infrared spectroscopy for the detection of vulnerable coronary artery plaques. J Am Coll Cardiol. (2006) 47(8 Suppl):C92–6. doi: 10.1016/j.jacc.2005.12.045
85. Goldstein JA, Maini B, Dixon SR, Brilakis ES, Grines CL, Rizik DG, et al. Detection of lipid-core plaques by intracoronary near-infrared spectroscopy identifies high risk of periprocedural myocardial infarction. Circ Cardiovasc Interv. (2011) 4(5):429–37. doi: 10.1161/CIRCINTERVENTIONS.111.963264
86. Erlinge D, Maehara A, Ben-Yehuda O, Bøtker HE, Maeng M, Kjøller-Hansen L, et al. Identification of vulnerable plaques and patients by intracoronary near-infrared spectroscopy and ultrasound (PROSPECT II): a prospective natural history study. Lancet. (2021) 397(10278):985–95. doi: 10.1016/S0140-6736(21)00249-X
87. Waksman R, Di Mario C, Torguson R, Ali ZA, Singh V, Skinner WH, et al. Identification of patients and plaques vulnerable to future coronary events with near-infrared spectroscopy intravascular ultrasound imaging: a prospective, cohort study. Lancet. (2019) 394(10209):1629–37. doi: 10.1016/S0140-6736(19)31794-5
88. Kini AS, Baber U, Kovacic JC, Limaye A, Ali ZA, Sweeny J, et al. Changes in plaque lipid content after short-term intensive versus standard statin therapy: the YELLOW trial (reduction in yellow plaque by aggressive lipid-lowering therapy). J Am Coll Cardiol. (2013) 62(1):21–9. doi: 10.1016/j.jacc.2013.03.058
89. Anroedh SS, Akkerhuis KM, Oemrawsingh RM, Garcia-Garcia HM, Brankovic M, Regar E, et al. Associations of 26 circulating inflammatory and renal biomarkers with near-infrared spectroscopy and long-term cardiovascular outcome in patients undergoing coronary angiography (ATHEROREMO-NIRS substudy). Curr Atheroscler Rep. (2018) 20(10):52. doi: 10.1007/s11883-018-0752-8
90. Fard AM, Vacas-Jacques P, Hamidi E, Wang H, Carruth RW, Gardecki JA, et al. Optical coherence tomography–near infrared spectroscopy system and catheter for intravascular imaging. Opt Express. (2013) 21(25):30849–58. doi: 10.1364/OE.21.030849
91. Hoang V, Grounds J, Pham D, Virani S, Hamzeh I, Qureshi AM, et al. The role of intracoronary plaque imaging with intravascular ultrasound, optical coherence tomography, and near-infrared spectroscopy in patients with coronary artery disease. Curr Atheroscler Rep. (2016) 18(9):57. doi: 10.1007/s11883-016-0607-0
92. Tearney GJ, Regar E, Akasaka T, Adriaenssens T, Barlis P, Bezerra HG, et al. Consensus standards for acquisition, measurement, and reporting of intravascular optical coherence tomography studies: a report from the international working group for intravascular optical coherence tomography standardization and validation. J Am Coll Cardiol. (2012) 59(12):1058–72. doi: 10.1016/j.jacc.2011.09.079
93. Araki M, Park SJ, Dauerman HL, Uemura S, Kim JS, Di Mario C, et al. Optical coherence tomography in coronary atherosclerosis assessment and intervention. Nat Rev Cardiol. (2022) 19(10):684–703. doi: 10.1038/s41569-022-00687-9
94. Phipps JE, Vela D, Hoyt T, Halaney DL, Mancuso JJ, Buja LM, et al. Macrophages and intravascular OCT bright spots: a quantitative study. JACC Cardiovasc Imaging. (2015) 8(1):63–72. doi: 10.1016/j.jcmg.2014.07.027
95. Minami Y, Phipps JE, Hoyt T, Milner TE, Ong DS, Soeda T, et al. Clinical utility of quantitative bright spots analysis in patients with acute coronary syndrome: an optical coherence tomography study. Int J Cardiovasc Imaging. (2015) 31(8):1479–87. doi: 10.1007/s10554-015-0714-y
96. Prati F, Romagnoli E, Gatto L, La Manna A, Burzotta F, Ozaki Y, et al. Relationship between coronary plaque morphology of the left anterior descending artery and 12 months clinical outcome: the CLIMA study. Eur Heart J. (2020) 41(3):383–91. doi: 10.1093/eurheartj/ehz520
97. Kume T, Okura H, Yamada R, Koyama T, Fukuhara K, Kawamura A, et al. Detection of plaque neovascularization by optical coherence tomography: ex vivo feasibility study and in vivo observation in patients with angina pectoris. J Invasive Cardiol. (2016) 28(1):17–22. PMID: 26716590.26716590
98. Jiang S, Fang C, Xu X, Xing L, Sun S, Peng C, et al. Identification of high-risk coronary lesions by 3-vessel optical coherence tomography. J Am Coll Cardiol. (2023) 81(13):1217–30. doi: 10.1016/j.jacc.2023.01.030
99. Leistner DM, Kränkel N, Meteva D, Abdelwahed YS, Seppelt C, Stähli BE, et al. Differential immunological signature at the culprit site distinguishes acute coronary syndrome with intact from acute coronary syndrome with ruptured fibrous cap: results from the prospective translational OPTICO-ACS study. Eur Heart J. (2020) 41(37):3549–60. doi: 10.1093/eurheartj/ehaa703
100. Gerhardt T, Seppelt C, Abdelwahed YS, Meteva D, Wolfram C, Stapmanns P, et al. Culprit plaque morphology determines inflammatory risk and clinical outcomes in acute coronary syndrome. Eur Heart J. (2023) 44(38):3911–25. doi: 10.1093/eurheartj/ehad334
101. Komukai K, Kubo T, Kitabata H, Matsuo Y, Ozaki Y, Takarada S, et al. Effect of atorvastatin therapy on fibrous cap thickness in coronary atherosclerotic plaque as assessed by optical coherence tomography: the EASY-FIT study. J Am Coll Cardiol. (2014) 64(21):2207–17. doi: 10.1016/j.jacc.2014.08.045
102. Nicholls SJ, Kataoka Y, Nissen SE, Prati F, Windecker S, Puri R, et al. Effect of evolocumab on coronary plaque phenotype and burden in statin-treated patients following myocardial infarction. JACC Cardiovasc Imaging. (2022) 15(7):1308–21. doi: 10.1016/j.jcmg.2022.03.002
103. Liu L, Gardecki JA, Nadkarni SK, Toussaint JD, Yagi Y, Bouma BE, et al. Imaging the subcellular structure of human coronary atherosclerosis using micro-optical coherence tomography. Nat Med. (2011) 17(8):1010–4. doi: 10.1038/nm.2409
104. Yin B, Piao Z, Nishimiya K, Hyun C, Gardecki JA, Mauskapf A, et al. 3D cellular-resolution imaging in arteries using few-mode interferometry. Light Sci Appl. (2019) 8:104. doi: 10.1038/s41377-019-0211-5
105. Nishimiya K, Tearney G. Micro optical coherence tomography for coronary imaging. Front Cardiovasc Med. (2021) 8:613400. doi: 10.3389/fcvm.2021.613400
106. Khraishah H, Jaffer FA. Intravascular molecular imaging: near-infrared fluorescence as a new frontier. Front Cardiovasc Med. (2020) 7:587100. doi: 10.3389/fcvm.2020.587100
107. Jaffer FA, Kim DE, Quinti L, Tung CH, Aikawa E, Pande AN, et al. Optical visualization of cathepsin K activity in atherosclerosis with a novel, protease-activatable fluorescence sensor. Circulation. (2007) 115(17):2292–8. doi: 10.1161/CIRCULATIONAHA.106.660340
108. Vinegoni C, Botnaru I, Aikawa E, Calfon MA, Iwamoto Y, Folco EJ, et al. Indocyanine green enables near-infrared fluorescence imaging of lipid-rich, inflamed atherosclerotic plaques. Sci Transl Med. (2011) 3(84):84ra45. doi: 10.1126/scitranslmed.3001577
109. Calfon MA, Vinegoni C, Ntziachristos V, Jaffer FA. Intravascular near-infrared fluorescence molecular imaging of atherosclerosis: toward coronary arterial visualization of biologically high-risk plaques. J Biomed Opt. (2010) 15(1):011107. doi: 10.1117/1.3280282
110. Jaffer FA, Weissleder R. Seeing within: molecular imaging of the cardiovascular system. Circ Res. (2004) 94(4):433–45. doi: 10.1161/01.RES.0000119321.18573.5A
111. Chen J, Tung CH, Mahmood U, Ntziachristos V, Gyurko R, Fishman MC, et al. In vivo imaging of proteolytic activity in atherosclerosis. Circulation. (2002) 105(23):2766–71. doi: 10.1161/01.CIR.0000017860.20619.23
112. Jaffer FA, Vinegoni C, John MC, Aikawa E, Gold HK, Finn AV, et al. Real-time catheter molecular sensing of inflammation in proteolytically active atherosclerosis. Circulation. (2008) 118(18):1802–9. doi: 10.1161/CIRCULATIONAHA.108.785881
113. Deguchi JO, Aikawa M, Tung CH, Aikawa E, Kim DE, Ntziachristos V, et al. Inflammation in atherosclerosis: visualizing matrix metalloproteinase action in macrophages in vivo. Circulation. (2006) 114(1):55–62. doi: 10.1161/CIRCULATIONAHA.106.619056
114. Wallis de Vries BM, Hillebrands JL, van Dam GM, Tio RA, de Jong JS, Slart RH, et al. Images in cardiovascular medicine. Multispectral near-infrared fluorescence molecular imaging of matrix metalloproteinases in a human carotid plaque using a matrix-degrading metalloproteinase-sensitive activatable fluorescent probe. Circulation. (2009) 119(20):e534–6. doi: 10.1161/CIRCULATIONAHA.108.821389
115. Verjans JW, Osborn EA, Ughi GJ, Calfon Press MA, Hamidi E, Antoniadis AP, et al. Targeted near-infrared fluorescence imaging of atherosclerosis: clinical and intracoronary evaluation of indocyanine green. JACC Cardiovasc Imaging. (2016) 9(9):1087–95. doi: 10.1016/j.jcmg.2016.01.034
116. McCarthy JR, Patel P, Botnaru I, Haghayeghi P, Weissleder R, Jaffer FA. Multimodal nanoagents for the detection of intravascular thrombi. Bioconjug Chem. (2009) 20(6):1251–5. doi: 10.1021/bc9001163
117. Hara T, Bhayana B, Thompson B, Kessinger CW, Khatri A, McCarthy JR, et al. Molecular imaging of fibrin deposition in deep vein thrombosis using fibrin-targeted near-infrared fluorescence. JACC Cardiovasc Imaging. (2012) 5(6):607–15. doi: 10.1016/j.jcmg.2012.01.017
118. Tung CH, Gerszten RE, Jaffer FA, Weissleder R. A novel near-infrared fluorescence sensor for detection of thrombin activation in blood. Chembiochem. (2002) 3(2–3):207–11. doi: 10.1002/1439-7633(20020301)3:2/3%3C207::AID-CBIC207%3E3.0.CO;2-B
119. Hara T, Ughi GJ, McCarthy JR, Erdem SS, Mauskapf A, Lyon SC, et al. Intravascular fibrin molecular imaging improves the detection of unhealed stents assessed by optical coherence tomography in vivo. Eur Heart J. (2017) 38(6):447–55. doi: 10.1038/nm.2555
120. Yoo H, Kim JW, Shishkov M, Namati E, Morse T, Shubochkin R, et al. Intra-arterial catheter for simultaneous microstructural and molecular imaging in vivo. Nat Med. (2011) 17(12):1680–4. doi: 10.1038/nm.2555
121. Bourantas CV, Jaffer FA, Gijsen FJ, van Soest G, Madden SP, Courtney BK, et al. Hybrid intravascular imaging: recent advances, technical considerations, and current applications in the study of plaque pathophysiology. Eur Heart J. (2017) 38(6):400–12. doi: 10.1093/eurheartj/ehw097
122. Ughi GJ, Wang H, Gerbaud E, Gardecki JA, Fard AM, Hamidi E, et al. Clinical characterization of coronary atherosclerosis with dual-modality OCT and near-infrared autofluorescence imaging. JACC Cardiovasc Imaging. (2016) 9(11):1304–14. doi: 10.1016/j.jcmg.2015.11.020
123. Wang H, Gardecki JA, Ughi GJ, Jacques PV, Hamidi E, Tearney GJ. Ex vivo catheter-based imaging of coronary atherosclerosis using multimodality OCT and NIRAF excited at 633 nm. Biomed Opt Express. (2015) 6(4):1363–75. doi: 10.1364/BOE.6.001363
124. Knuuti J, Wijns W, Saraste A, Capodanno D, Barbato E, Funck-Brentano C, et al. 2019 ESC guidelines for the diagnosis and management of chronic coronary syndromes. Eur Heart J. (2020) 41(3):407–77. doi: 10.1093/eurheartj/ehz425
125. Gallone G, Bellettini M, Gatti M, Tore D, Bruno F, Scudeler L, et al. Coronary plaque characteristics associated with major adverse cardiovascular events in atherosclerotic patients and lesions: a systematic review and meta-analysis. JACC Cardiovasc Imaging. (2023) 16(12):1584–1604. doi: 10.1016/j.jcmg.2023.08.006
126. Kuroda M, Shinke T, Sakaguchi K, Otake H, Takaya T, Hirota Y, et al. Effect of daily glucose fluctuation on coronary plaque vulnerability in patients pre-treated with lipid-lowering therapy: a prospective observational study. JACC Cardiovasc Interv. (2015) 8(6):800–11. doi: 10.1016/j.jcin.2014.11.025
Keywords: atherosclerosis, inflammation, intravascular ultrasound, near-infrared spectroscopy, optical coherence tomography, near-infrared fluorescence imaging
Citation: Los J, Mensink FB, Mohammadnia N, Opstal TSJ, Damman P, Volleberg RHJA, Peeters DAM, van Royen N, Garcia-Garcia HM, Cornel JH, El Messaoudi S and van Geuns R-JM (2024) Invasive coronary imaging of inflammation to further characterize high-risk lesions: what options do we have? Front. Cardiovasc. Med. 11:1352025. doi: 10.3389/fcvm.2024.1352025
Received: 7 December 2023; Accepted: 15 January 2024;
Published: 1 February 2024.
Edited by:
Marco Zimarino, Asl Lanciano Vasto Chieti, ItalyReviewed by:
Francesco Cardaioli, University of Padua, ItalyGiulio Russo, Policlinico Tor Vergata, Italy
© 2024 Los, Mensink, Mohammadnia, Opstal, Damman, Volleberg, Peeters, van Royen, Garcia-Garcia, Cornel, El Messaoudi and van Geuns. This is an open-access article distributed under the terms of the Creative Commons Attribution License (CC BY). The use, distribution or reproduction in other forums is permitted, provided the original author(s) and the copyright owner(s) are credited and that the original publication in this journal is cited, in accordance with accepted academic practice. No use, distribution or reproduction is permitted which does not comply with these terms.
*Correspondence: Robert-Jan M. van Geuns cm9iZXJ0amFuLnZhbmdldW5zQHJhZGJvdWR1bWMubmw=
†These authors have contributed equally to this work and share last authorship
Abbreviations PCSK9, proprotein convertase subtilisin kexin type 9; LDL, low-density-lipoprotein; NLRP3, NOD-, LRR- and pyrin domain-containing protein 3; IL, interleukin; TCFA, thin-cap fibroatheroma; ACS, acute coronary syndrome; CCS, chronic coronary syndrome; IVUS, intravascular ultrasound; VH-IVUS, virtual histology intravascular ultrasound; NIRS, near-infrared spectroscopy; LCBI, lipid core burden index; MACE, major adverse cardiovascular events; OCT, optical coherence tomography; µOCT, micro optical coherence tomography; NIRF, near-infrared fluorescence; ICG, indocyanine green; NIRAF, near-infrared autofluorescence.