- 1Department of Cardio-Thoracic-Vascular Diseases, Foundation IRCCS Ca’ Granda Ospedale Maggiore Policlinico, Milan, Italy
- 2Department of Clinical Sciences and Community Health, University of Milan, Milan, Italy
- 3Department of Anatomical Pathology and Clinical Morphology, Yerevan State Medical University after M. Heratsi, Yerevan, Armenia
- 4Department of Pharmacological and Biomolecular Sciences “Rodolfo Paoletti”, University of Milan, Milan, Italy
- 5Cardioncology Unit, Cardioncology and Second Opinion Division, European Institute of Oncology, I.R.C.C.S., Milan, Italy
Background: Oxidative stress induced by the excessive production of reactive oxygen species is one of the primary mechanisms implicated in anthracycline (ANT)-induced cardiotoxicity. There is a strong clinical need for a molecule capable of effectively preventing and reducing the oxidative damage caused by ANT. In vitro and in vivo studies conducted in mice have shown that melatonin stimulates the expression of antioxidative agents and reduces lipid peroxidation induced by ANT.
Methods: We investigated this issue through a meta-analysis of murine model studies. The outcome of the meta-analysis was to compare oxidative damage, estimated by products of lipid peroxidation (MDA = Malondialdehyde) and markers of oxidative stress (SOD = Superoxide Dismutase, GSH = Glutathione), along with a marker of cardiac damage (CK-MB = creatine kinase–myocardial band), assessed by measurements in heart and/or blood samples in mice undergoing ANT chemotherapy and assuming melatonin vs. controls. The PubMed, OVID-MEDLINE and Cochrane library databases were analysed to search English-language review papers published from the inception up to August 1st, 2023. Studies were identified by using Me-SH terms and crossing the following terms: “melatonin”, “oxidative stress”, “lipid peroxidation”, “anthracycline”, “cardiotoxicity”.
Results: The metanalysis included 153 mice administered melatonin before, during or immediately after ANT and 153 controls from 13 studies. Compared with controls, the levels of all oxidative stress markers were significantly better in the pooled melatonin group, with standardized mean differences (SMD) for MDA, GSH and SOD being −8.03 ± 1.2 (CI: −10.43/−5.64, p < 0.001), 7.95 ± 1.8 (CI: 4.41/11.5, p < 0.001) and 3.94 ± 1.6 (CI: 0.77/7.12, p = 0.015) respectively. Similarly, compared with controls, CK-MB levels reflecting myocardial damage were significantly lower in the pooled melatonin group, with an SMD of −4.90 ± 0.5 (CI: −5.82/−3.98, p < 0.001).
Conclusion: Melatonin mitigates the oxidative damage induced by ANT in mouse model. High-quality human clinical studies are needed to further evaluate the use of melatonin as a preventative/treatment strategy for ANT-induced cardiotoxicity.
1. Introduction
Anthracyclines (ANT) are very effective drugs for the treatment of different types of malignant cancers. However, ANT clinical application is dose-limited due to the potential side effect of cardiotoxicity, in particular for doxorubicin (DOX) (1).
Multiple mechanisms involved in ANT-induced cardiotoxicity have been explored and the increased oxidative stress by the overproduction of reactive oxygen species (ROS) is one of the main hypothesis suggested in literature (2). Cardiomyocytes are enriched in mitochondria, a major subcellular target of ANT, due to its affinity to cardiolipin, a component of the inner mitochondrial membrane (3). The heart is vulnerable to ANT-induced oxidative damage because of the abundancy of mitochondria and the relatively low levels of antioxidant enzymes compared to other tissues,. As a result, a reduction of oxidative stress can be considered a clinical target for ANT-induce cardiotoxicity prevention and/or treatment (3).
Melatonin (N-acetyl-5-methoxytryptamine), a secretory product of the pineal gland, is a ROS scavenger of high potency, and with its amphiphilic property, melatonin is capable of crossing mitochondrial membrane (4, 5). In vitro and in vivo studies (e.g., in mice) reported that melatonin stimulates of the expression of antioxidative agents including Superoxide Dismutase (SOD) and Glutathione (GSH) and reduces lipid peroxidation induced by ANT, estimated by the dosage of Malondialdehyde (MDA), product of this process (6–32).
Evidence from clinical studies in humans evaluating the specific effect of melatonin in the prevention and/or treatment of ANT-induced cardiotoxicity are lacking. Only a study is currently available, which is focused on patients with advanced-stage solid tumors and poor functional status and found that melatonin reduced the occurrence of cardiotoxicity (33). Our hypothesis is that melatonin may prove effective in preventing and counteracting the oxidative damage induced by ANT, and thus play a role in preventing cardiotoxicity.
Therefore, having supportive basic science data on the role of melatonin in this setting from single studies on mouse model, we investigated this issue through a meta-analysis of studies that reported data on molecular oxidative damage in mice undergoing ANT chemotherapy and supplemented with melatonin vs. controls.
2. Methods
The present research was performed following the Preferred Reporting Items for Systematic reviews and Meta-Analyses (PRISMA) guidelines (34). The PubMed, OVID-MEDLINE and Cochrane library databases were analysed to search English-language review papers published from the inception up to August 1st, 2023. Studies were identified by using Me-SH terms and crossing the following terms: “melatonin”, “oxidative stress”, “lipid peroxidation”, “anthracycline”, “cardiotoxicity”. Main inclusion criteria were: (1) English papers published in peer-reviewed journals; (2) studies providing parameters of lipid peroxidation, oxidative stress, and/or cardiac damage; (3) minimum set of mouse model population characteristics. Specific exclusion criteria were: (1) studies with less than 4 mice; no predetermined maximum sample size was set; (2) studies reporting data on oxidative damage with incompatible and/or non-convertible units of measurement and therefore not amenable to metanalysis; (3) reviews, editorials, and case reports were excluded from analyses (but examined for potential additional references).
Literature search and data extraction were performed by two reviewers (A.F. and L.D) and independently checked by another reviewer (E.G) that resolved disagreements on study judgments. Where complete data were not available, they were requested from the specific corresponding author and, if it was not possible to obtain them, they were extrapolated from the figures of the studies. Although it has not been specifically designed for basic science research studies, the Newcastle– Ottawa Scale (NOS) was used to measure study quality (http://www.ohri.ca/programs/clinical_epidemiology/oxford.htm).
The outcome of the meta-analysis was to compare oxidative damage estimated by products of lipid peroxidation and markers of oxidative stress (ie, MDA, GSH, SOD), along with marker of cardiac damage (creatine kinase–myocardial band CK-MB), assessed by dosage in heart and/or blood samples, in mice undergoing ANT chemotherapy and assuming melatonin (before, during, or after chemotherapy) vs. controls not assuming melatonin. To this purpose, a pooled analysis of these parameters was performed using fixed or random effects models by Comprehensive Meta-Analysis Version 2, Biostat, Englewood, NJ, USA. Heterogeneity was estimated by using I-square, Q and tau-square values; random or fixed effect models were applied when heterogeneity across studies (I2) was higher or lower than 75%, respectively. Standard means difference (SMD) with 95% confidence interval (CI) was calculated to evaluate the statistical difference of variables in melatonin treated mice and controls. The limit of statistical significance was set at P < .05. Publication bias was assessed by using the funnel plot method according to the trim and fill test. Observed and adjusted values, their lower and upper limits have been calculated. To assess the effect of individual studies on the pooled result, we conducted a sensitivity analysis by excluding each study one by one and recalculating the combined estimates on remaining studies.
3. Limitations
Our meta-analysis has several limitations that we would like to address. Some of the variables evaluated in the included studies are heterogeneous and expressed in different units of measurement, requiring conversion. Certain studies were excluded precisely due to the inability to render the data amenable to meta-analysis. Additionally, despite all the various regimens of ANT chemotherapy administered in the studies being sufficient to induce cardiotoxicity, they vary greatly from one another, even in terms of time frames (e.g., models of acute, subacute, chronic toxicity). Similarly, the administration of melatonin is not consistent across the different studies in terms of dosage, route and timing of administration. Furthermore, as none of the studies evaluated the potential gender-specific efficacy of melatonin, no conclusions can be drawn regarding its gender-related impact in this setting. Finally, our meta-analysis focused solely on those outcomes related to oxidative damage that were amenable for meta-analysis, while others exist in the literature (e.g., nitric oxide, glutathione peroxidase enzyme activity, 4-hydroxyalkenals, thiobarbituric acid reactive substances, conjugated dienes, and protein carbonyl) but lack sufficient data for a meta-analytic study.
4. Results
4.1. Characteristics of the studies
After removing duplicates, the initial literature search identified 55 papers. The PRISMA flowchart showing the search strategy and manuscripts selection process is illustrated in Figure 1.
After the initial screening of titles and abstracts, 21 studies were excluded as they were not related to the topic. Therefore, 34 studies were reviewed; of these, 3 did not report data on oxidative damage, 11 reported data on oxidative damage with incompatible and/or non-convertible units of measurement and therefore not amenable to metanalysis, 4 were reviews, commentaries, and editorials, and 2 were excluded for miscellaneous reasons. According to the NOS, the quality of the studies ranged from 6 to 9 (i.e., a score that identifies studies of fair or good quality). Therefore, no study was excluded based on its limited quality.
On the whole 306 mice undergoing ANT chemotherapy were included in 13 studies (sample size ranging from 12 to 70) performed in 3 continental areas (Europe = 1, Asia = 10, Africa = 2): 153 mice assuming melatonin before, during or immediately after chemotherapy and 153 controls not assuming melatonin (6–16, 18, 19). Since the study of Oz et al. (10) included 3 different analysable groups (melatonin before ANT group called “OZ pre”, melatonin after ANT group called “Oz post”, and control group) it was considered twice. For this reason, despite the actual number of included studies being 13, the meta-analysis was conducted on 14 different melatonin treatment groups vs. control groups. Table 1 summarizes the main findings of selected studies such as Authors, year of publication, sample size, chemotherapy regimen, timing and route of melatonin administration, mice species, gender and biomarkers of oxidative damage (MDA, GSH, SOD and CK-MB) assessed at the end of the chemotherapy and melatonin cycles. The cumulative dose (mg/kg) of ANT which was sufficient to develop cardiotoxicity was administered to each mouse, generally intraperitoneally (11 intraperitoneal and 3 intravenous). The comparison of biomarkers of oxidative stress between mice assuming melatonin and controls was conducted at the end of the chemotherapeutic and melatonin cycles (chemotherapy duration ranging from 1 to 30 days, melatonin duration ranging from 3 to 30 days). Notably, only one study included female mice, the adopted ANT was DOX in every study, the preferred melatonin route of administration was intraperitoneal (10 studies intraperitoneal, 2 studies subcutaneous, 2 studies oral) and melatonin administration mainly started before the chemotherapy regimen (5 studies before + during + immediately after, 4 studies during, 3 studies only before, 2 studies during + immediately after, 1 study only immediately after chemotherapy).
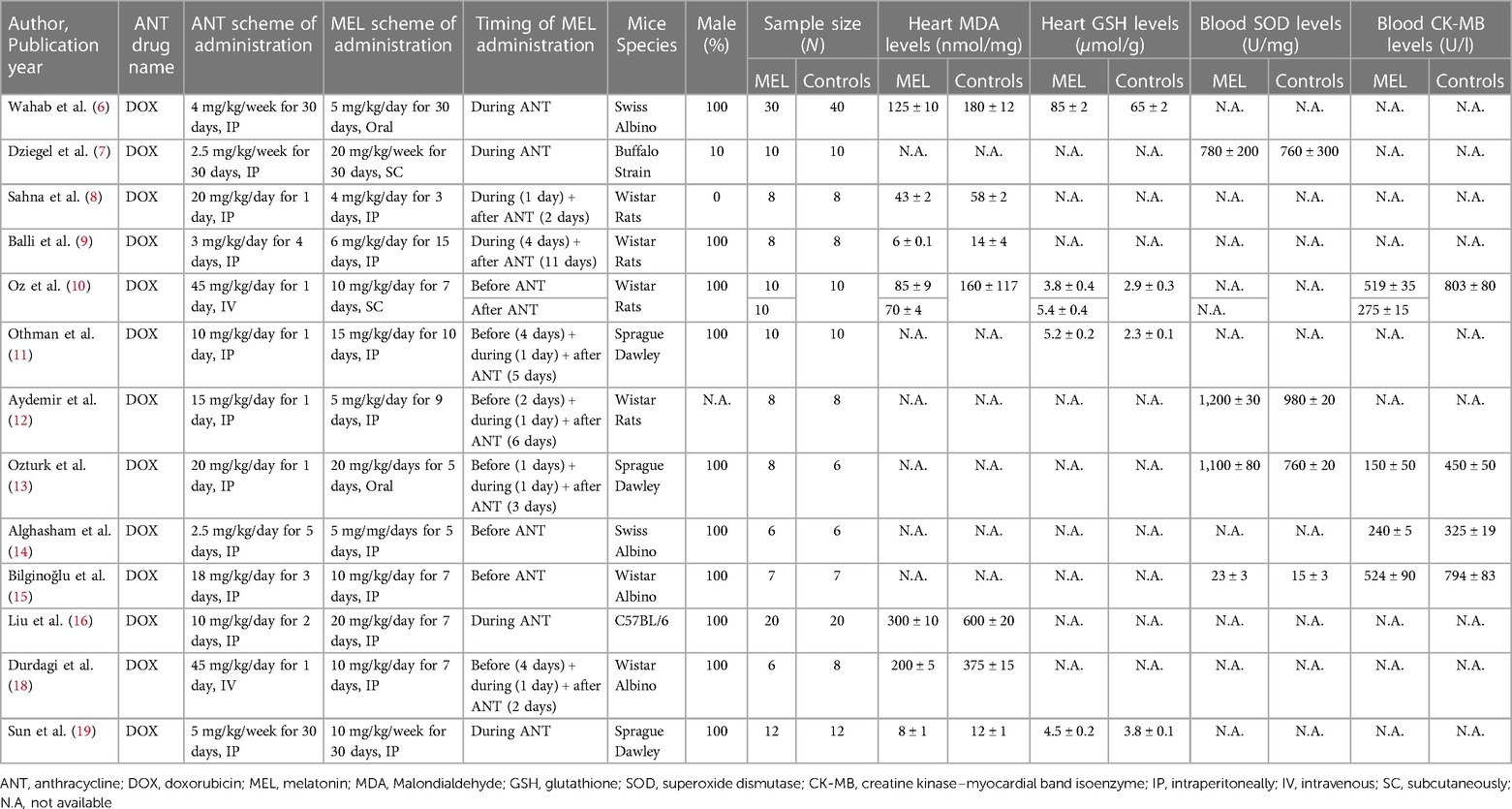
Table 1. Summary of study characteristics of oxidative damage biomarkers in mice included in the systematic review and meta-analysis.
4.2. Effect of melatonin on heart MDA levels
Compared with controls (N = 106), MDA levels measured on heart tissue samples after ANT chemotherapy with DOX was significantly better in the pooled melatonin group (N = 104). As shown in Figure 2, MDA levels (104.2 ± 6.8 vs. 194.5 ± 26.7 nmol/mg, data from 7 studies and from 8 vs. 8 analysable groups) were significantly lower in the pooled melatonin group than in the control group; SMD being −8.03 ± 1.2 (CI: −10.43/−5.64, p < 0.001). The presence of a single study effect was excluded at sensitivity analysis; a relevant publication bias was not present for studies reporting MDA in melatonin mice and controls. The difference in MDA levels between the melatonin group and controls was still present after correction for publication bias (SMD: −9.19, CI: −12.56/−5.82).
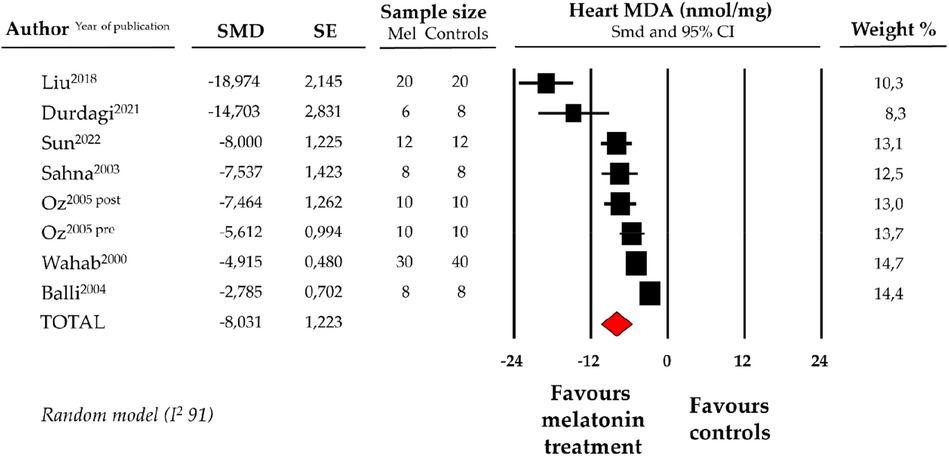
Figure 2. Forest plot for standard means difference (SMD) of heart malondialdehyde (MDA) levels in melatonin treated mice and controls. Relative weight of each study is reported on the right side. CI, confidence interval.
4.3. Effect of melatonin on heart GSH levels
Compared with controls (N = 72), GSH levels measured on heart tissue samples after ANT chemotherapy with DOX were significantly better in the pooled melatonin group (N = 72). As shown in Figure 3, GSH levels (20.8 ± 4.5 vs. 15.3 ± 2.5 micromol/g, data from 4 studies and from 5 vs. 5 analysable groups) were significantly higher in the pooled melatonin group vs. control group; SMD being 7.95 ± 1.8 (CI: 4.41/11.5, p < 0.001). Publication bias was not present for studies reporting GSH in melatonin mice and controls.
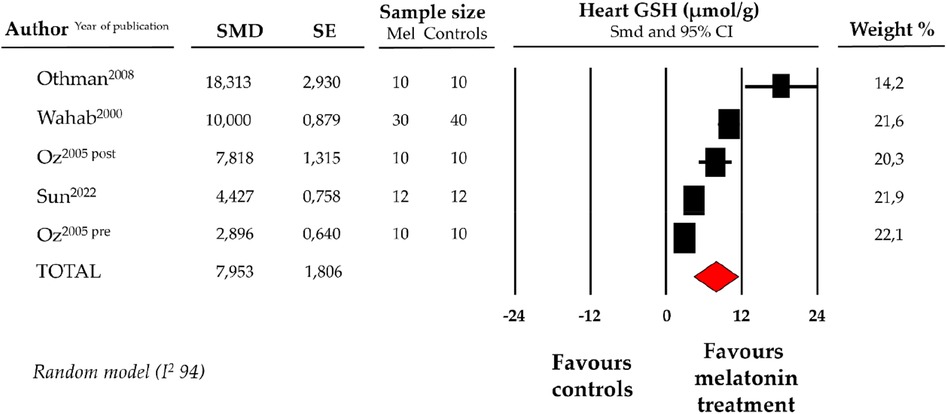
Figure 3. Forest plot for standard means difference (SMD) of heart glutathione (GSH) levels in melatonin treated mice and controls. Relative weight of each study is reported on the right side. CI, confidence interval.
4.4. Effect of melatonin on blood levels of SOD
Compared with controls (N = 31), SOD levels, measured on peripheric blood sample after ANT chemotherapy with DOX, were significantly better in the pooled melatonin group (N = 33). As shown in Figure 4, SOD levels (775.6 ± 409.1 vs. 627.97 ± 309.4 U/mg, data from 4 studies) were relevantly higher in the pooled melatonin group than in the control group; SMD being 3.94 ± 1.6 (CI: 0.77/7.12, p = 0.015). A difference in SOD levels between the melatonin group and controls was still present after correction for publication bias, even if not reaching statistical significance (SMD: 1.32, CI: −1.63/4.28) (Supplementary Figure S1).
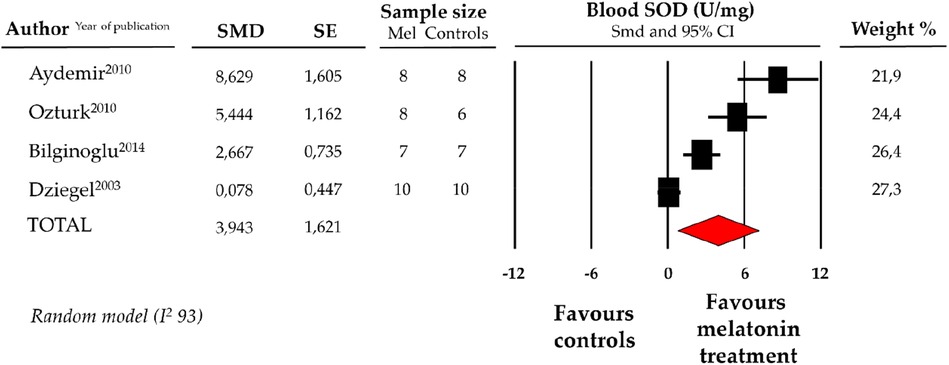
Figure 4. Forest plot for standard means difference (SMD) of blood superoxide dismutase (SOD) levels in melatonin treated mice and controls. Relative weight of each study is reported on the right side. CI, confidence interval.
4.5. Effect of melatonin on blood levels of CK-MB
Compared with controls (N = 29), CK-MB levels measured on peripheric blood sample after ANT chemotherapy with DOX was significantly better in the pooled melatonin group (N = 41). As shown in Figure 5, CK-MB levels (337.6 ± 40.2 vs. 634.5 ± 118.5 U/L, data from 4 studies and from 5 vs. 5 analysable groups) were relevantly lower in the pooled melatonin group than in the control group; SMD being −4.90 ± 0.5 (CI: −5.82/−3.98, p < 0.001). The presence of a single study effect was excluded at sensitivity analysis; a relevant publication bias was not present for studies reporting CK-MB in melatonin mice and controls. The difference in CK-MB levels between the melatonin group and controls was still present after correction for publication bias (SMD: −4.21, CI: −5.04/−3.37).
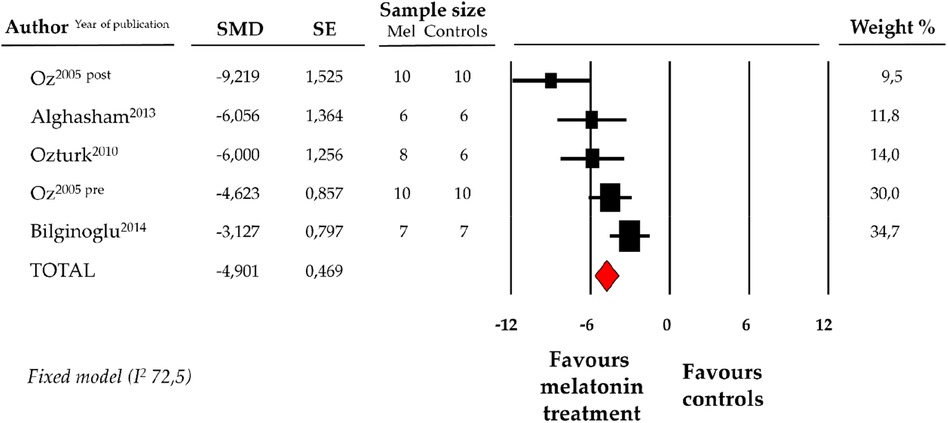
Figure 5. Forest plot for standard means difference (SMD) of blood creatine kinase–myocardial band (CK-MB) levels in melatonin treated mice and controls. Relative weight of each study is reported on the right side. CI, confidence interval.
5. Discussion
Based on the existing literature, melatonin emerges as a promising cardioprotective agent in the context of ANT-induced cardiotoxicity in mouse models. Its cardioprotective effects appear to be associated not only with its ability to modulate redox system biomarkers (evaluated by MDA, GSH, and SOD levels) but also with its beneficial influence on cardiomyocyte injury, as evidenced by CK-MB levels (Table 2).
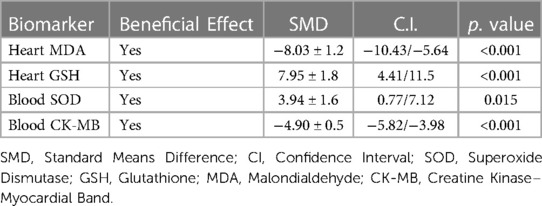
Table 2. Summary table of the overall effect of melatonin on oxidative damage and myocardial injury biomarkers.
The myocardium is an energy-dependent tissue with a well-developed mitochondrial apparatus (35). However, the antioxidant reserve in cardiomyocytes is limited, making them highly susceptible to oxidative damage. Oxidative stress is considered one of the main mechanisms in the development and progression of many cardiovascular diseases (36). In particular, in the pathogenesis of ANT-induced cardiotoxicity, oxidative stress is recognized as a main contributor to cardiomyocytes damage and death (37). DOX, a cationic molecule with hydrophilic and hydrophobic sights, penetrates easily organelle membranes (38). Its cationic nature allows DOX to readily enter the inner mitochondrial membrane, where it forms a stable complex with cardiolipin, disrupting its function and stimulating the production of superoxide radicals responsible for oxidative damage and lipid peroxidation (39).
Currently, neurohormonal therapies and statins are the only cardiovascular drugs suggested by the European guidelines to reduce the risk of significant ANT-induced cardiotoxicity (1). Although the efficacy of statins in this setting may be in question, recent data and a 2023 meta-analysis of randomized clinical trials conducted by Agarwal et al. have shown that statins are associated with a 54% reduction in the relative risk of ANT-induced cardiac dysfunction (40, 41). In addition, sodium-glucose cotransporter 2 inhibitors have shown promising results in preventing ANT-related cardiac dysfunction on mouse models (42). Moreover, our group has recently published the first human case-series demonstrating clinical benefits of sodium-glucose cotransporter 2 inhibitors in the specific treatment of ANT-related cardiac dysfunction (43). Finally, a recent Cochrane Systematic Review and Meta-analysis demonstrated the actual efficacy of dexrazoxane in preventing and reducing cardiotoxicity in adults undergoing ANT treatment, with no evidence of a negative impact on tumor response rates (44). However, currently available medical strategies to prevent and to treat ANT-induced cardiotoxicity need further refinement. In particular, a molecule capable of preventing and/or reducing ANT-mediated oxidative damage would be highly desirable.
Within this scenario, the critical assessment of the molecular cascades of melatonin's potential cardioprotective effects and their extrapolation to the model of ANT-induced cardiotoxicity appears relevant. Molecular evidence suggests that melatonin could exert a significant cardioprotective effect, primarily through its anti-oxidant properties (45–47). Out of the numerous available antioxidants, melatonin has undergone extensive investigation for its potential to prevent ANT-induced cardiotoxicity through both in vitro and in vivo studies conducted in mice (48). Melatonin improves survival rates in mice with tumours receiving high cumulative doses of ANT and particularly benefits the structural and functional parameters of the myocardium (49). It is worth emphasizing that melatonin does not seem impairing the effectiveness of chemotherapy, positioning this hormone as a potentially useful biological compound to be used in under-treated and post-treatment cancer patients (50).
The anti-oxidant effects of melatonin [due to its amphiphilic nature it can easily diffuse through biological membrane and penetrate into cardiomyocytes (51) responsible for cardio-protection in experimental models of ANT-induced cardiotoxicity is hypothesized to be related to different and complex molecular processes (48), practically summarizable into two main mechanisms:
5.1. Melatonin enhances antioxidant agents
ANT increases oxidative stress by generating ROS via a redox cycling mechanism. The complex I of electron transport chain reduces DOX, a quinone compound, to a semiquinone which in turn donates an unstable electron to an oxygen molecule and formed a dangerous ROS, namely superoxide anion (O2 −) (52). Normally, antioxidative enzymes defend against oxidative damaged via the detoxification of the generated ROS. In particular, the circulant antioxidant agent SOD is able to convert the superoxide anion (O2 −) to hydrogen peroxide (H2O2), which is then antioxidized by catalase and glutathione peroxidase enzyme into an harmless water molecule (53). ANT decrease the levels and activity of SOD (13). The present meta-analysis highlights that in mouse models exposed to ANT, the use of melatonin significantly increases blood levels of the antioxidant agent SOD compared to controls without melatonin (Figure 4) (51, 54).
GSH, the most plentiful thiol-containing substance of low molecular weight in cells, is a crucial antioxidant and antidote in all mammalian tissues, particularly in the cardiomyocytes. Indeed, heart GSH quenches oxidizing substances (such as reactive hydroxyl free radicals, peroxynitrite, and H2O2) directly or reduces H2O2 to water (55). In this instance as well, melatonin treatment appears capable of restoring and maintaining significantly higher levels of heart GSH in mouse models exposed to ANT (Figure 3) (10, 22). Finally, melatonin also appears to exert antioxidative properties via direct scavenging of ROS, mainly by single electron transfer, hydrogen transfer, and radical adduction formation (46).
5.2. Melatonin directly mitigates lipid peroxidation, a product of oxidative damage
One of the consequences of uncontrolled oxidative stress (imbalance between the prooxidant and antioxidant levels in favor of prooxidants) is cells, tissues, and organs injury caused by oxidative damage. In particular, it has long been recognized that high levels of ROS can inflict direct damage to lipids (56). Indeed, ROS attack lipids containing carbon-carbon double bond(s), especially polyunsaturated fatty acids (PUFAs), with oxygen insertion resulting in lipid peroxidation process whose main secondary product is MDA (57). Under high lipid peroxidation rates the extent of oxidative damage overwhelms repair capacity and the cells auto-induce apoptosis or necrosis programmed cell death (58). In this setting, MDA has been widely used for many years and it is currently still adopted as a convenient biomarker for evaluation of lipid peroxidation and, in general, of oxidative stress (59, 60). In mouse models ANT significantly increase the level of lipid peroxidation products, especially MDA, but also 4-hydroxyalkenals, thiobarbituric acid reactive substances, conjugated dienes and protein carbonyl (32, 19). Extensive basic science literature has suggested that the use of melatonin can directly mitigate lipid peroxidation induced by ANT, mainly assessed by detecting MDA levels in the heart of mouse models (30, 28). Supporting this hypothesis, our meta-analysis results indicate that mice exposed to ANT and administered melatonin exhibit significantly lower MDA (i.e., lipid peroxidation) levels compared to controls (Figure 2).
Although not the primary focus of this systematic review, there are additional mechanisms beyond the discussed protection against oxidative damage that contribute to the cardioprotective effect of melatonin against ANT-induced cardiotoxicity (48). Notably, studies conducted both in vitro and in vivo (using mouse models) suggest that melatonin exerts its cardioprotective effects by safeguarding, regenerating, and enhancing mitochondrial functions (61). Indeed, as it is known, ANT rapidly disrupts the structural and functional integrity of mitochondria, resulting in advanced mitochondrial dysfunction (62). Melatonin-induced normalization of Sirt1/Nrf2 pathways increases the levels of PGC-1α and ultimately suppresses the expression of Drp-1, leading to a reduction in mitochondrial fission. Moreover, melatonin supports mitochondrial biogenesis by preventing the decrease of PGC-1α and normalizing signal transmission in models of ANT-induced cardiotoxicity (16). These protective effects counteract mitochondrial dysfunction and improve mitochondrial clearance, also providing essential antiapoptotic actions (19). Melatonin also regulates ANT-induced myocardial cell death through a direct impact on the essential autophagy and mitophagy pathways (63). Due to these melatonin's antiapoptotic effects, there might be concerns about its potential interference with the effectiveness of ANT cancer treatment. Fortunately, evidence suggests that melatonin has dual effects on apoptosis, selectively enhancing the antiapoptotic effect on normal cells while triggering apoptotic pathways in cancer cells. Therefore, it does not appear to compromise the efficacy of chemotherapy (64).
Moreover, melatonin stabilizes iron metabolism (65), preventing excessive formation of the Fenton reaction and ferroptosis, which play a significant role in the development of ANT-induced cardiotoxicity (66).
In addition, ANT induces biochemical stress in the myocardium, leading to a switch in metabolic substrate utilization from fatty acids to glucose. This metabolic switch leads to fatty acids accumulation, which can result in ROS formation, with subsequent mitochondrial dysfunction and endoplasmic reticulum stress-related protein misfolding. Melatonin and its active metabolites appear to limit and decelerate all the above-mentioned changes (67).
Finally, melatonin exhibits significant anti-fibrotic potential achieved by inhibiting the lncR-MALAT1/miR-141-mediated activation of NLRP3 inflammasome and TGF-β1/Smad signalling pathways stabilization (68). This effect is particularly relevant concerning the pro-fibrotic effects of ANT, as the myocardium is a highly differentiated morphological unit, and in response to injury and cell death, fibroblast differentiation with subsequent production of connective tissue and fibrosis occurs (69).
Finally, the ability of melatonin to protect the heart from the ANT-induced oxidative damage, together with the additional cardioprotective mechanisms discussed above, would seem to directly impact cardiomyocyte viability. As supported by the results of our meta-analysis, melatonin is associated with significantly lower levels of myocardionecrosis indexes (i.e., CK-MB) than controls in mice exposed to toxic doses of ANT (Figure 5). The protective effect on the myocardium could have significant clinical implications, suggesting that melatonin could mitigate ANT-induced myocardial damage and consequently prevent the development of left ventricular dysfunction and other manifestations of cardiotoxicity. In support of this hypothesis, some studies, conducted on murine models, have shown that melatonin ameliorates the reduction of left ventricular ejection fraction, of stroke volume, and of fractional shortening that are induced by ANT (70, 71). Indeed, encouraging echocardiographic findings from Liu et al. propose that melatonin may alleviate ANT-induced systolic dysfunction, as assessed by left ventricular ejection fraction and left ventricular fractional shortening (16). To date, there is only a single human study that has evaluated the impact of melatonin in patients with cancer. This study was a randomized trial involving 250 individuals with advanced-stage solid tumors and compromised functional status, some of them treated with ANT. The findings of this study were encouraging, indicating that melatonin potentially enhanced the 1-year survival rate and decreased the occurrence of cardiotoxicity (33).
6. Conclusions
The findings of the present comprehensive systematic revision of the basic science literature, along with the conducted meta-analysis, support the hypothesis that melatonin mitigates the oxidative damage caused by ANT and the subsequent myocardial damage. Consequently, it holds promise as a potential effective clinical approach for preventing and/or treating ANT-induced cardiotoxicity in humans. However, high quality clinical studies are thus warranted, and indeed to be recommended to further evaluate the use of melatonin as a preventative/treatment strategy for ANT-induced cardiotoxicity.
Data availability statement
The data analyzed in this study is subject to the following licenses/restrictions: Created dataset is available upon request. Requests to access these datasets should be directed to andreafaggiano95@gmail.com.
Author contributions
AF: Conceptualization, Data curation, Formal Analysis, Methodology, Project administration, Supervision, Writing – original draft, Writing – review & editing. EG: Conceptualization, Data curation, Formal Analysis, Methodology, Project administration, Software, Supervision, Writing – original draft, Writing – review & editing. AA: Writing – original draft, Writing – review & editing. MR: Supervision, Writing – review & editing. LD: Data curation, Writing – review & editing. MF: Supervision, Writing – review & editing. CC: Supervision, Writing – review & editing. MV: Supervision, Writing – review & editing. SC: Supervision, Writing – review & editing. DC: Conceptualization, Supervision, Validation, Writing – review & editing.
Funding
The author(s) declare financial support was received for the research, authorship, and/or publication of this article.
This work was partially funded by Italian Ministry of University and Research (grant number 2022ZPS49L to MR and SC), by Italian Ministry of Health, Ricerca Corrente 2023 Fondazione IRCCS Ca' Granda Ospedale Maggiore Policlinico (to SC) and by the European Union - Next Generation EU, Italian National Recovery and Resilience Plan, (Mission 4, Component 2, Investment 1.5 “Innovation Ecosystems”), project MUSA (to SC and MR).
Conflict of interest
The authors declare that the research was conducted in the absence of any commercial or financial relationships that could be construed as a potential conflict of interest.
The author(s) declared that they were an editorial board member of Frontiers, at the time of submission. This had no impact on the peer review process and the final decision.
Publisher's note
All claims expressed in this article are solely those of the authors and do not necessarily represent those of their affiliated organizations, or those of the publisher, the editors and the reviewers. Any product that may be evaluated in this article, or claim that may be made by its manufacturer, is not guaranteed or endorsed by the publisher.
Supplementary material
The Supplementary Material for this article can be found online at: https://www.frontiersin.org/articles/10.3389/fcvm.2023.1289384/full#supplementary-material
Supplementary Figure S1
Funnel plot assessing publication bias for standard means difference (SMD) of Superoxide Dismutase (SOD) in melatonin treated mice and controls. Observed (white symbols) and imputed (black symbols) values.
References
1. Lyon AR, López-Fernández T, Couch LS, Asteggiano R, Aznar MC, Bergler-Klein J, et al. 2022 ESC guidelines on cardio-oncology developed in collaboration with the European hematology association (EHA), the European society for therapeutic radiology and oncology (ESTRO) and the international cardio-oncology society (IC-OS): developed by the the task force on cardio-oncology of the European Society of Cardiology (ESC). Eur Heart J. (2022) 23(10):E333–465. doi: 10.1093/eurheartj/ehac244
2. Songbo M, Lang H, Xinyong C, Bin X, Ping Z, Liang S. Oxidative stress injury in doxorubicin-induced cardiotoxicity. Toxicol Lett. (2019) 307:41–48. doi: 10.1016/j.toxlet.2019.02.013
3. Goormaghtigh E, Huart P, Praet M, Brasseur R, Ruysschaert JM. Structure of the adriamycin-cardiolipin complex. Role in mitochondrial toxicity. Biophys Chem. (1990) 35(2–3):247–57. doi: 10.1016/0301-4622(90)80012-V
4. García JJ, Lõpez-Pingarrõn L, Almeida-Souza P, Tres A, Escudero P, García-Gil FA, et al. Protective effects of melatonin in reducing oxidative stress and in preserving the fluidity of biological membranes: a review. J Pineal Res. (2014) 56:225–37. doi: 10.1111/jpi.12128
5. Rosales-Corral S, Tan DX, Reiter RJ, Valdivia-Velázquez M, Martínez-Barboza G, Acosta- Martínez JP, et al. Orally administered melatonin reduces oxidative stress and proinflammatory cytokines induced by amyloid-β peptide in rat brain: a comparative, in vivo study versus vitamin C and E. J Pineal Res. (2003) 35(2):80–84. doi: 10.1034/j.1600-079X.2003.00057.x
6. Wahab MHA, Akoul ESEMS, Abdel-Aziz AAH. Modulatory effects of melatonin and vitamin E on doxorubicin-induced cardiotoxicity in Ehrlich ascites carcinoma-bearing mice. Tumori. (2000) 86(2):157–62. doi: 10.1177/030089160008600210
7. Dziȩgiel P, Murawska-Ciałowicz E, Jethon Z, Januszewska L, Podhorska-Okołów M, Surowiak P, et al. Melatonin stimulates the activity of protective antioxidative enzymes in myocardial cells of rats in the course of doxorubicin intoxication. J Pineal Res. (2003) 35(3):183–7. doi: 10.1034/j.1600-079X.2003.00079.x
8. Sahna E, Parlakpinar H, Ozer MK, Ozturk F, Ozugurlu F, Acet A. Melatonin protects against myocardial doxorubicin toxicity in rats: role of physiological concentrations. J Pineal Res. (2003) 35(4):257–61. doi: 10.1034/j.1600-079X.2003.00084.x
9. Balli E, Mete UÖ, Tuli A, Tap Ö, Kaya M. Effect of melatonin on the cardiotoxicity of doxorubicin. Histol Histopathol. (2004) 19(4). Available at: http://hdl.handle.net/10201/2162515375752
10. Öz E, Erbaş D, Sürücü HS, Düzgün E. Prevention of doxorubicin-induced cardiotoxicity by melatonin. Mol Cell Biochem. (2006) 282(1–2):31–37. doi: 10.1007/s11010-006-1153-9
11. Othman AI, El-Missiry MA, Amer MA, Arafa M. Melatonin controls oxidative stress and modulates iron, ferritin, and transferrin levels in adriamycin treated rats. Life Sci. (2008) 83(15–16):563–8. doi: 10.1016/j.lfs.2008.08.004
12. Aydemir S, Özdemir I, Kart A. Role of exogenous melatonin on adriamycin-induced changes in the rat heart. Eur Rev Med Pharmacol Sci. (2010) 14(5):435–41. Available at: https://web.p.ebscohost.com/abstract?direct=true&profile=ehost&scope=site&authtype=crawler&jrnl=11283602&AN=51344180&h=H4Q647mHJ3CS5D%2fJrPb6lKF290rwii6lB1E67eB0tpPmMla9%2bvcvjfeEdC60ZaQ9y1sFq7PzpCXJQlzCMY9tMg%3d%3d&crl=c&resultNs=AdminWebAuth&resultLocal=ErrCrlNotAuth&crlhashurl=login.aspx%3fdirect%3dtrue%26profile%3dehost%26scope%3dsite%26authtype%3dcrawler%26jrnl%3d11283602%26AN%3d5134418020556922
13. Ozturk M, Ozler M, Kurt YG, Ozturk B, Uysal B, Ersoz N, et al. Efficacy of melatonin, mercaptoethylguanidine and 1400 W in doxorubicin- and trastuzumab-induced cardiotoxicity. J Pineal Res. (2011) 50(1):89–96. doi: 10.1111/j.1600-079X.2010.00818.x
14. Alghasham AA. Comparative assessment of melatonin-afforded protection in liver, kidney and heart of male mice against doxorubicin induced toxicity. Pharmacol Pharm. (2013) 04(08):590–98. doi: 10.4236/pp.2013.48085
15. Bilginoğlu A, Aydin D, Özsoy Ş, Aygün H. Protective effect of melatonin on adriamycin- induced cardiotoxicity in rats. Turk Kardiyol Dern Ars. (2014) 42(3):265–73. doi: 10.5543/tkda.2014.36089
16. Liu D, Ma Z, Di S, Yang Y, Yang J, Xu L, et al. AMPK/PGC1α activation by melatonin attenuates acute doxorubicin cardiotoxicity via alleviating mitochondrial oxidative damage and apoptosis. Free Radic Biol Med. (2018) 129:59–72. doi: 10.1016/j.freeradbiomed.2018.08.032
17. Pehlivan DY, Durdagi G, Oz Oyar E, Akyol S, Ozbek M. The effects of melatonin and thymoquinone on doxorubicin-induced cardiotoxicity in rats. Bratislava Med J. (2020) 121(10):753–9. doi: 10.4149/BLL_2020_123
18. Durdagi G, Pehlivan DY, Oyar EO, Bahceci SA, Ozbek M. Effects of melatonin and adrenomedullin in reducing the cardiotoxic effects of doxorubicin in rats. Cardiovasc Toxicol. (2021) 21(5):354–364. doi: 10.1007/s12012-020-09625-y
19. Sun X, Sun P, Zhen D, Xu X, Yang L, Fu D, et al. Melatonin alleviates doxorubicin-induced mitochondrial oxidative damage and ferroptosis in cardiomyocytes by regulating YAP expression. Toxicol Appl Pharmacol. (2022) 437:115902. doi: 10.1016/j.taap.2022.115902
20. Morishima I, Toki Y, Okumura K, Ito T. Melatonin, a pineal hormone with antioxidant property, protects against adriamycin cardiomyopathy in rats. J Am Coll Cardiol. (1998) 31(7):511–21. doi: 10.1016/S0735-1097(98)81113-3
21. Morishima I, Okumura K, Matsui H, Kaneko S, Numaguchi Y, Kawakami K, et al. Zinc accumulation in adriamycin-induced cardiomyopathy in rats: effects of melatonin, a cardioprotective antioxidant. J Pineal Res. (1999) 26(4):204–10. doi: 10.1111/j.1600-079X.1999.tb00585.x
22. Agapito MT, Antolín Y, Del Brio MT, López-Burillo S, Pablos MI, Recio JM. Protective effect of melatonin against adriamycin toxicity in the rat. J Pineal Res. (2001) 31(1):23–30. doi: 10.1034/j.1600-079X.2001.310104.x
23. Xu MF, Ho S, Qian ZM, Tang PL. Melatonin protects against cardiac toxicity of doxorubicin in rat. J Pineal Res. (2001) 31(4):301–7. doi: 10.1034/j.1600-079X.2001.310403.x
24. Xu MF, Tang PL, Qian ZM, Ashraf M. Effects by doxorubicin on the myocardium are mediated byoxygen free radicals. Life Sci. (2001) 68(8):889–901. doi: 10.1016/S0024-3205(00)00990-5
25. Dziȩgiel P, Jethon Z, Suder E, Sopel M, Rabczyński J, Surowiak P, et al. Role of exogenous melatonin in reducing the cardiotoxic effect of daunorubicin and doxorubicin in the rat. Exp Toxicol Pathol. (2002) 53(6):433–9. doi: 10.1078/0940-2993-00217
26. Zhang W, Wang X, Tang Y, Huang C. Melatonin alleviates doxorubicin-induced cardiotoxicity via inhibiting oxidative stress, pyroptosis and apoptosis by activating Sirt1/Nrf2 pathway. Biomed Pharmacother. (2023) 162:114591. doi: 10.1016/j.biopha.2023.114591
27. Koçak G, Erbil KM, Özdemir I, Aydemir S, Sunar B, Tuncel M, et al. The protective effect of melatonin on adriamycin-induced acute cardiac injury. Can J Cardiol. (2003) 19(5):535–41. PMID: 12717489.
28. Han J, Kim C, Kim N, Park J, Yang Y, Kim E. The protective effect of melatonin administration against adriamycin-induced cardiotoxicity in rats. Korean J Physiol Pharmacol. (2001) 5(4):333–42. Available at: https://koreascience.kr/article/JAKO200103039573294.page
29. Ahmed HH, Mannaa F, Elmegeed GA, Doss SH. Cardioprotective activity of melatonin and its novel synthesized derivatives on doxorubicin-induced cardiotoxicity. Bioorganic Med Chem. (2005) 13(5):1847–57. doi: 10.1016/j.bmc.2004.10.066
30. Kim C, Kim N, Joo H, Youm JB, Park WS, Van Cuong D, et al. Modulation by melatonin of the cardiotoxic and antitumor activities of adriamycin. J Cardiovasc Pharmacol. (2005) 46(2):200–10. doi: 10.1097/01.fjc.0000171750.97822.a2
31. Guven A, Yavuz O, Cam M, Ercan F, Bukan N, Comunoglu C. Melatonin protects against epirubicin-induced cardiotoxicity. Acta Histochem. (2007) 109(1):52–60. doi: 10.1016/j.acthis.2006.09.007
32. Arinno A, Maneechote C, Khuanjing T, Ongnok B, Prathumsap N, Chunchai T, et al. Cardioprotective effects of melatonin and metformin against doxorubicin-induced cardiotoxicity in rats are through preserving mitochondrial function and dynamics. Biochem Pharmacol. (2021) 192:114743. doi: 10.1016/j.bcp.2021.114743
33. Lissoni P, Barni S, Mandalà M, Ardizzoia A, Paolorossi F, Vaghi M, et al. Decreased toxicity and increased efficacy of cancer chemotherapy using the pineal hormone melatonin in metastatic solid tumour patients with poor clinical status. Eur J Cancer. (1999) 35(12):1688–92. doi: 10.1016/S0959-8049(99)00159-8
34. Tricco AC, Lillie E, Zarin W, O’Brien KK, Colquhoun H, Levac D, et al. PRISMA extension for scoping reviews (PRISMA-ScR): checklist and explanation. Ann Intern Med. (2018) 169(7):467–73. doi: 10.7326/M18-0850
35. Ng SM, Neubauer S, Rider OJ. Myocardial metabolism in heart failure. Curr Heart Fail Rep. (2023) 20:63–75. doi: 10.1007/s11897-023-00589-y
36. Hou J, Yuan Y, Chen P, Lu K, Tang Z, Liu Q, et al. Pathological roles of oxidative stress in cardiac microvascular injury. Curr Probl Cardiol. (2023) 48:101399. doi: 10.1016/j.cpcardiol.2022.101399
37. Avagimyan A, Kakturskiy L, Heshmat-Ghahdarijani K, Pogosova N, Sarrafzadegan N. Anthracycline associated disturbances of cardiovascular homeostasis. Curr Probl Cardiol. (2022) 47.
38. Bin WB, Leung KT, Poon ENY. Mitochondrial-targeted therapy for doxorubicin-induced cardiotoxicity. Int J Mol Sci. (2022) 23:1912. doi: 10.3390/ijms23031912
39. Avagimyan AA, Mkrtchyan LH, Gevorkyan AA, Kononchuk NB, Kakturskiy L V, Djndoyan ZT. Relationship between chemotherapy and atrial fibrillation: clinical case. Ration Pharmacother Cardiol. (2021) 17(5):785–91. doi: 10.20996/1819-6446-2021-10-17
40. Ruscica M, Ferri N, Banach M, Sirtori CR, Corsini A. Side effects of statins: from pathophysiology and epidemiology to diagnostic and therapeutic implications. Cardiovasc Res. (2023) 118:3288–304. doi: 10.1093/cvr/cvac020
41. Agarwal S, Guha A, Krishan S, Naqash AR, Addison D, Yang EH, et al. Statins for primary prevention of anthracycline chemotherapy-related cardiac dysfunction: a systematic review and meta-analysis of randomized controlled trials. Am J Cardiol. (2023) 206:63–6. doi: 10.1016/j.amjcard.2023.08.123
42. Faggiano A, Gherbesi E, Cardinale D, Vicenzi M, Carugo S. SGLT2-i Prevent left ventricular dysfunction induced by anthracycline in mouse model: a systematic-review and meta- analysis. Vasc Pharmacol. (2023) 150:107171. doi: 10.1016/j.vph.2023.107171
43. Giangiacomi F, Faggiano A, Cardinale D, Rossi FG, Pollina A, Gherbesi E, et al. Case report: sodium-glucose cotransporter 2 inhibitors induce left ventricular reverse remodeling in anthracycline-related cardiac dysfunction—a case series. Front Cardiovasc Med. (2023) 10:1250185. doi: 10.3389/fcvm.2023.1250185
44. de Baat EC, Mulder RL, Armenian S, Feijen EA, Grotenhuis H, Hudson MM, et al. Dexrazoxane for preventing or reducing cardiotoxicity in adults and children with cancer receiving anthracyclines. Cochrane Database Syst Rev. (2022) 9(9):CD014638. doi: 10.1002/14651858.CD014638.pub2
45. Claustrat B, Leston J. Melatonin: physiological effects in humans. Neurochirurgie. (2015) 61:77–84. doi: 10.1016/j.neuchi.2015.03.002
46. Galano A, Tan DX, Reiter RJ. On the free radical scavenging activities of melatonin’s metabolites, AFMK and AMK. J Pineal Res. (2013) 54:245–57. doi: 10.1111/jpi.12010
47. An P, Wan S, Luo Y, Luo J, Zhang X, Zhou S, et al. Micronutrient supplementation to reduce cardiovascular risk. J Am Coll Cardiol. (2022) 80(24):2269–85. doi: 10.1016/j.jacc.2022.09.048
48. Attachaipanich T, Chattipakorn SC, Chattipakorn N. Potential roles of melatonin in doxorubicin-induced cardiotoxicity: from cellular mechanisms to clinical application. Pharmaceutics. (2023) 15:785. doi: 10.3390/pharmaceutics15030785
49. Davoodvandi A, Nikfar B, Reiter RJ, Asemi Z. Melatonin and cancer suppression: insights into its effects on DNA methylation. Cell Mol Biol Lett. (2022) 27(1):1–11. doi: 10.1186/s11658-022-00375-z
50. Ma Z, Xu L, Liu D, Zhang X, Di S, Li W, et al. Utilizing melatonin to alleviate Side effects of chemotherapy: a potentially good partner for treating cancer with ageing. Oxid Med Cell Longevity. (2020) 2020:6841581. doi: 10.1155/2020/6841581
51. Reiter RJ, Mayo JC, Tan DX, Sainz RM, Alatorre-Jimenez M, Qin L. Melatonin as an antioxidant: under promises but over delivers. J Pineal Res. (2016) 61(3):253–78. doi: 10.1111/jpi.12360
52. Davies KJA, Doroshow JH. Redox cycling of anthracyclines by cardiac mitochondria. I. Anthracycline radical formation by NADH dehydrogenase. J Biol Chem. (1986) 261(7):3060–7. doi: 10.1016/S0021-9258(17)35746-0
53. Doroshow JH, Davies KJA. Redox cycling of anthracyclines by cardiac mitochondria. II. Formation of superoxide anion, hydrogen peroxide, and hydroxyl radical. J Biol Chem. (1986) 261(7):3068–74. doi: 10.1016/S0021-9258(17)35747-2
54. Zhang HM, Zhang Y. Melatonin: a well-documented antioxidant with conditional pro-oxidant actions. J Pineal Res. (2014) 57:131–46. doi: 10.1111/jpi.12162
55. Tan M, Yin Y, Ma X, Zhang J, Pan W, Tan M, et al. Glutathione system enhancement for cardiac protection: pharmacological options against oxidative stress and ferroptosis. Cell Death Dis. (2023) 14:131. doi: 10.1038/s41419-023-05645-y
56. Ayala A, Muñoz MF, Argüelles S. Lipid peroxidation: production, metabolism, and signaling mechanisms of malondialdehyde and 4-hydroxy-2-nonenal. Oxid Med Cell Longevity. (2014) 2014:360438. doi: 10.1155/2014/360438
57. Yin H, Xu L, Porter NA. Free radical lipid peroxidation: mechanisms and analysis. Chem Rev. (2011) 111:5944–72. doi: 10.1021/cr200084z
58. Volinsky R, Kinnunen PKJ. Oxidized phosphatidylcholines in membrane-level cellular signaling: from biophysics to physiology and molecular pathology. FEBS J. (2013) 280:2806–16. doi: 10.1111/febs.12247
59. Esterbauer H, Cheeseman KH, Dianzani MU, Poli G, Slater TF. Separation and characterization of the aldehydic products of lipid peroxidation stimulated by ADP-Fe2+ in rat liver microsomes. Biochem J. (1982) 208(1):129–40. doi: 10.1042/bj2080129
60. Esterbauer H, Eckl P, Ortner A. Possible mutagens derived from lipids and lipid precursors. Mutat Res Genet Toxicol. (1990) 238(3):223–33. doi: 10.1016/0165-1110(90)90014-3
61. Govender J, Loos B, Marais E, Engelbrecht AM. Melatonin improves cardiac and mitochondrial function during doxorubicin-induced cardiotoxicity: a possible role for peroxisome proliferator-activated receptor gamma coactivator 1-alpha and sirtuin activity? Toxicol Appl Pharmacol. (2018) 358:86–101. doi: 10.1016/j.taap.2018.06.031
62. Kong CY, Guo Z, Song P, Zhang X, Yuan YP, Teng T, et al. Underlying the mechanisms of doxorubicin-induced acute cardiotoxicity: oxidative stress and cell death. Int J Biol Sci. (2022) 18:760. doi: 10.7150/ijbs.65258
63. Luo F, Sandhu AF, Rungratanawanich W, Williams GE, Akbar M, Zhou S, et al. Melatonin and autophagy in aging-related neurodegenerative diseases. Int J Mol Sci. (2020) 21:7174. doi: 10.3390/ijms21197174
64. Bizzarri M, Proietti S, Cucina A, Reiter RJ. Molecular mechanisms of the pro-apoptotic actions of melatonin in cancer: a review. Expert Opin Ther Targets. (2013) 17:1483–96. doi: 10.1517/14728222.2013.834890
65. Ichikawa Y, Ghanefar M, Bayeva M, Wu R, Khechaduri A, Prasad SVN, et al. Cardiotoxicity of doxorubicin is mediated through mitochondrial iron accumulation. J Clin Invest. (2014) 124(2):617–30. doi: 10.1172/JCI72931
66. Park WR, Choi B, Kim YJ, Kim YH, Park MJ, Kim DI, et al. Melatonin regulates iron homeostasis by inducing hepcidin expression in hepatocytes. Int J Mol Sci. (2022) 23(7):3593. doi: 10.3390/ijms23073593
67. Govender J, Loos B, Engelbrecht AM. Melatonin: a protective role against doxorubicin-induced cardiotoxicity. Future Oncol. (2015) 11:2003–6. doi: 10.2217/fon.15.48
68. Che H, Wang Y, Li H, Li Y, Sahil A, Lv J, et al. Melatonin alleviates cardiac fibrosis via inhibiting lncRNA MALAT1/miR-141-mediated NLRP3 inflammasome and TGF-β1/smads signaling in diabetic cardiomyopathy. FASEB J. (2020) 34(4):5282–98. doi: 10.1096/fj.201902692R
69. Levick SP, Soto-Pantoja DR, Bi J, Hundley WG, Widiapradja A, Manteufel EJ, et al. Doxorubicin-induced myocardial fibrosis involves the neurokinin-1 receptor and direct effects on cardiac fibroblasts. Hear Lung Circ. (2019) 28(10):1598–605. doi: 10.1016/j.hlc.2018.08.003
70. Liu X, Chen Z, Chua CC, Ma YS, Youngberg GA, Hamdy R, et al. Melatonin as an effective protector against doxorubicin-induced cardiotoxicity. Am J Physiol Hear Circ Physiol. (2002) 283(1):H254–63. doi: 10.1152/ajpheart.01023.2001
Keywords: melatonin, anthracycline, cardiotoxicity, oxidative stress, oxidative damage
Citation: Faggiano A, Gherbesi E, Avagimyan A, Ruscica M, Donisi L, Fedele MA, Cipolla CM, Vicenzi M, Carugo S and Cardinale D (2023) Melatonin mitigates oxidative damage induced by anthracycline: a systematic-review and meta-analysis of murine models. Front. Cardiovasc. Med. 10:1289384. doi: 10.3389/fcvm.2023.1289384
Received: 5 September 2023; Accepted: 30 October 2023;
Published: 23 November 2023.
Edited by:
Canan G. Nebigil, INSERM U1260 Nanomedicine régénératrice (RNM), FranceReviewed by:
Gaurav Sharma, University of Texas Southwestern Medical Center, United StatesSakiko Miyazaki, Juntendo University, Japan
Anna Narezkina, University of California, United States
© 2023 Faggiano, Gherbesi, Avagimyan, Ruscica, Donisi, Fedele, Cipolla, Vicenzi, Carugo and Cardinale. This is an open-access article distributed under the terms of the Creative Commons Attribution License (CC BY). The use, distribution or reproduction in other forums is permitted, provided the original author(s) and the copyright owner(s) are credited and that the original publication in this journal is cited, in accordance with accepted academic practice. No use, distribution or reproduction is permitted which does not comply with these terms.
*Correspondence: Andrea Faggiano YW5kcmVhZmFnZ2lhbm85NUBnbWFpbC5jb20= Daniela Cardinale ZGFuaWVsYS5jYXJkaW5hbGVAaWVvLml0
†These authors have contributed equally to this work
Abbreviations ANT, anthracyclines; DOX, doxorubicin; SMD, standard means difference; PRISMA, preferred reporting items for systematic reviews and meta-analyses; CI, confidence interval; ROS, reactive oxygen species; SOD, superoxide dismutase; GSH, glutathione; MDA, malondialdehyde; CK-MB, creatine kinase–myocardial band.
‡ORCID Andrea Faggiano orcid.org/0000-0002-3247-3203