- 1Departments of Surgery, Keck School of Medicine, University of Southern California, Los Angeles, CA, United States
- 2Pathology, Keck School of Medicine, University of Southern California, Los Angeles, CA, United States
- 3Stem Cell Biology and Regenerative Medicine, Keck School of Medicine, University of Southern California, Los Angeles, CA, United States
- 4Department of Medicine, Rosalind Franklin University School of Medicine and Science, Chicago, IL, United States
- 5Translational Genomics, Keck School of Medicine, University of Southern California, Los Angeles, CA, United States
- 6Department of Pediatrics, Boston Children’s Hospital, Boston, MA, United States
- 7Department of Genetics, Harvard Medical School, Boston, MA, United States
- 8Pediatrics, Keck School of Medicine, University of Southern California, Los Angeles, CA, United States
- 9Department of Surgery, University of Nebraska Medical Center, Omaha, NE, United States
Objective: Eighty percent of patients with a diagnosis of tetralogy of Fallot (TOF) do not have a known genetic etiology or syndrome. We sought to identify key molecular pathways and biological processes that are enriched in non-syndromic TOF, the most common form of cyanotic congenital heart disease, rather than single driver genes to elucidate the pathogenesis of this disease.
Methods: We undertook exome sequencing of 362 probands with non-syndromic TOF and their parents within the Pediatric Cardiac Genomics Consortium (PCGC). We identified rare (minor allele frequency <1 × 10−4), de novo variants to ascertain pathways and processes affected in this population to better understand TOF pathogenesis. Pathways and biological processes enriched in the PCGC TOF cohort were compared to 317 controls without heart defects (and their parents) from the Simons Foundation Autism Research Initiative (SFARI).
Results: A total of 120 variants in 117 genes were identified as most likely to be deleterious, with CHD7, CLUH, UNC13C, and WASHC5 identified in two probands each. Gene ontology analyses of these variants using multiple bioinformatic tools demonstrated significant enrichment in processes including cell cycle progression, chromatin remodeling, myocyte contraction and calcium transport, and development of the ventricular septum and ventricle. There was also a significant enrichment of target genes of SOX9, which is critical in second heart field development and whose loss results in membranous ventricular septal defects related to disruption of the proximal outlet septum. None of these processes was significantly enriched in the SFARI control cohort.
Conclusion: Innate molecular defects in cardiac progenitor cells and genes related to their viability and contractile function appear central to non-syndromic TOF pathogenesis. Future research utilizing our results is likely to have significant implications in stratification of TOF patients and delivery of personalized clinical care.
1. Introduction
Congenital heart disease (CHD) represents the most common birth defect, impacting roughly 1.8% of all live births globally, and is the leading non-infectious cause of infant mortality and pediatric hospitalization costs (1–4). Despite the devastating consequences and relatively high incidence, the etiology of most structural cardiac disease remains unknown. Tetralogy of Fallot (TOF), originally coined as “blue baby syndrome,” is the most prevalent form of cyanotic congenital heart disease (5%–10% of all CHD diagnoses, ∼3 per 10,000 live births) (5–8). TOF is defined by the combined presence of a sub-aortic ventricular septal defect, an overriding aorta, right ventricular hypertrophy and right ventricular outflow tract obstruction. TOF often requires corrective surgery during the first year of life, with a median total cost in the United States of America of $179,494 for primary repair or $222,799 for staged repair (9). While approximately 20% of cases of TOF are syndromic with an identified pathogenic gene, such as DiGeorge Syndrome (22q11.2 deletion), trisomy 21, Alagille Syndrome (JAG1), Ritscher-Schinzel-like Syndrome (WASHC5), and CHARGE Syndrome (CHD7) (10–14), 80% of cases are of unknown etiology (8). As the overall incidence and the increasing cost of care reduces accessibility to necessary treatments (15), it is imperative that the biological processes underlying aberrant heart development leading to TOF be defined.
Identification of the pathogenic origins of TOF have thus far focused on single driver genes, primarily by pedigree studies (7, 8). Conversely, animal model studies have elucidated important pathogenic pathways that may be driven by polygenic or environmental perturbations. There are several animal models of CHD, including conotruncal defects that present similar to TOF pathology observed in humans (16–20). Some of these models present with cardiac structural defects related to an environmental cause (i.e., prenatal alcohol exposure, maternal diabetes), while others are induced by genetic knockouts and haploinsufficiency, or a combination of both (21, 22, 23, 24, 25, 26). What is shared amongst these varied models is a disruption to either second heart field viability and proliferation, or cardiac neural crest cell viability and migration necessary for the maturation of the developing cardiac outflow tract. As such, a variety of perturbations in seemingly disparate genes and pathways may converge on the same biological processes and result in developmental derangements leading to TOF. For instance, regulation of secondary heart field cardioblast proliferation (GO:0003266; amigo.geneontology.org) has 60 associated genes. A deleterious mutation in any of the upstream genes in this process has the potential to result in TOF. Rare variants (MAF <0.01) with functional effects of transcripts involved in second heart field biology indeed were previously identified in a study of 93 non-syndromic TOF patients (27). We therefore hypothesize that deleterious genetic variants are enriched in specific pathways or biological processes among probands with non-syndromic TOF. Specifically, we hypothesize that TOF may be the result of impaired biological processes that are critical to progenitor cell development, mainly second heart field derived cells and cardiac neural crest cells which are the primary cell populations responsible for cardiac outflow tract development and contribute to development of the right ventricle and ventricular septum (28–30).
We set out to test this hypothesis by conducting de novo variant analysis on exome sequencing data of TOF patients collected and sequenced by the Pediatric Cardiac Genomics Consortium (PCGC). Our focus was on rare (minor allele frequency [MAF] of <1 × 10−4 according to Exome Aggregation Consortium) loss-of-function (LoF) and likely deleterious missense and non-frameshift indel (D-Miss/Indel) variants to maximize the likelihood of identifying variants with true pathogenic potential. We conducted a parallel de novo analysis of exome sequencing data from unaffected siblings who participated in the Simons Foundation Autism Research Initiative (SFARI) (i.e., those without autism spectrum disorder or heart defects/pathology) and their parents as a control group to evaluate the specificity of our TOF cohort enrichment results, as has been done in previous studies (31, 32). A variety of genetic analyses have been conducted utilizing the PCGC database studying de novo variants, single nucleotide variants, copy number variants, and indels in CHD more generally (i.e., after cohort aggregation of multiple heart defect phenotypes), and identified enrichment for cilia-related and chromatin-modifying genes (31, 33, 34). The study we performed here is unique by focusing solely on non-syndromic TOF probands, an approach that was recently successful in identifying novel candidate CHD genes in a study of non-rare, de novo variants in patients with TOF from the European Genome-phenome Archive (35).
2. Methods
2.1. Study population
2.1.1. PCGC
The National Heart, Lung, and Blood Institute funded the PCGC and established the Congenital Heart Disease Genetic Network Study across six main and four ancillary recruitment sites across the United States of America and the United Kingdom (36). All participant members of the consortium provided informed consent. All cardiac diagnoses were made using an echocardiogram, other advanced imaging modality and operative reports. Information about the participant's demographics, family history, and additional data were collected through a combination of interviews and the participant's medical record. A total of 3,937 probands from PCGC were examined to select for cases with TOF (Supplementary Figures 1A, B). A total of 747 non-syndromic participants with TOF without a known chromosomal abnormality were identified. 404 had exome sequencing performed for the proband and both parents.
2.1.2. SFARI
SFARI was started in 2003 to identify targets for research furthering the diagnosis and treatment of autism spectrum disorder (37). Within the many projects run by the foundation is the Simons Foundation Powering Autism Research (SPARK) initiative, which allows for use of its database for research outside of autism spectrum disorder (38). The initial SPARK cohort collected between 2016 and 2017 included 18,089 individuals with autism spectrum disorder and 28,515 family members. Trios were selected for this study to be comprised of an unaffected sibling and their parents, generating a proper control trio wherein the sibling proband has neither autism spectrum disorder diagnosis nor congenital heart disease. 350 randomly selected control trios from SFARI were analyzed.
2.2. Bioinformatic analysis
FASTQ files for all probands and their parents were assessed for quality control using FASTQC and MultiQC (Figure 1A) (39, 40). 90% of the FASTQ files for the selected TOF trios and 91% of SFARI control trios passed quality control (i.e., the proband and both parents passed) and were used for our analyses of de novo variants (PCGC TOF n = 362; SFARI control n = 317). Files were aligned with BWA-MEM to GRCh38 and then processed according to GATK Best Practices workflow (41, 42). Variants were called using GATK HaplotypeCaller. Variants were filtered for only high confidence de novo variants using GATK CalculateGenotypePosteriors, wherein both parents had a confident call for being homozygous for the reference allele. Functional annotation was next performed with snpEff and filtered with snpSift (43, 44). Additional functional annotation was conducted with ANNOVAR, including for MAF annotation derived from the Exome Aggregation Consortium (ExAC) and Combined Annotation-Dependent Depletion (CADD) scoring (45–47). CADD scores can score SNPs and indels based on their likelihood to be causal both on factors such as species conservation and impact on protein functionality. This analysis pipeline was automated utilizing Snakemake (48, 49). The complete bioinformatic pipeline for this analysis can be accessed via github1.
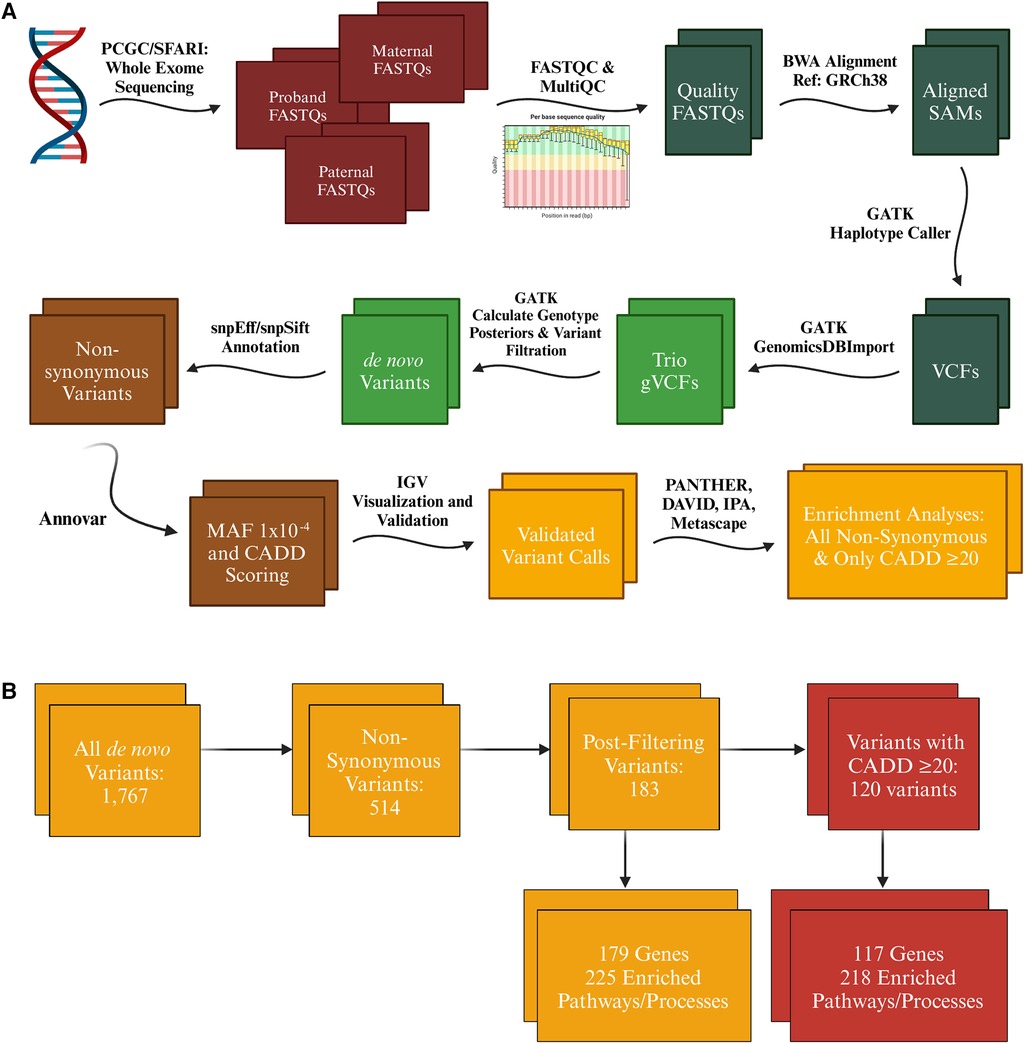
Figure 1. Variant call analysis of tetralogy of Fallot cohort. (A) Exome sequencing raw data was acquired from the Pediatric Cardiac Genomics Consortium (PCGC) for patients with non-syndromic tetralogy of Fallot (TOF) and Simons Foundation Autism Research Initiative (SFARI) for controls in the form of FASTQ and CRAM files respectively (which were converted to FASTQ format using samtools) for the proband and their associated parents. FASTQC and MultiQC were utilized to identify only high quality FASTQ files for analysis. All files were aligned to GRCh38 using BWA-MEM, then processed further according to GATK's best practices workflow until high confidence de novo variant calls were achieved for each trio. These variants were functionally annotated with snpEff, snpSift, and Annovar to identify variants that met our minor allele frequency threshold of 1 × 10−4 and were determined by snpEff (moderate or high impact) and the variant's CADD score (≥20) to likely be deleterious. (B) Initial variant calls included synonymous variants, which were first filtered down just to non-synonymous variants annotated as moderate or high impact by snpEff. Further filtering eliminated variants identified to be likely false positive calls and those with a minor allele frequency greater than 1 × 10−4. The number of variants was further reduced by filtering for only variants that had a defined CADD score of 20 or greater. A similar winnowing occurred with the SFARI cohort, however resulting in a smaller number of enriched pathways and processes in both the analysis of all non-synonymous variants (103) and only those with a CADD score of 20 or greater (59), all of which came from the Disease and Function analysis in IPA. * = Term enriched in both TOF and SFARI cohort. Figure generated with Biorender.com.
2.3. Variant filtering and enrichment analysis
Variants annotated as synonymous, upstream, downstream, 5′ or 3′ UTR, and intronic were filtered out (Figures 1A,B). Variants in genes known to be false positive calls in exome sequencing research were also filtered out (50). To focus on rare alleles exclusively we selected a MAF threshold of 1 × 10−4 according to ExAC via annotation by ANNOVAR. Only variants annotated by snpEff to be of moderate or high impact and a defined CADD phred score of 20 (representing approximately the top 1% of single nucleotide variants that had the highest prediction of functional effect) or greater were included in the enrichment analysis, with a secondary analysis conducted on all variants with a moderate or high impact annotation (snpEff) but no inclusion cutoff based on CADD phred scores.
A combination of Gene Ontology (GO) tools was used to determine enrichment of biological processes and pathways: the Protein ANalysis THrough Evolutionary Relationships (PANTHER) classification system2, the Database for Annotation, Visualization, and Integrated Discovery (DAVID) software3, Metascape4) and Qiagen's Ingenuity Pathway Analysis (IPA) software (51–55). The input to each of these tools was the variant list generated from our pipeline, one list of all de novo, non-synonymous variants and one list of only those with a defined CADD score of 20 or greater (Figure 1B). Genes identified in the variant analysis were evaluated for expression levels in the fetal heart via the Brotman Baty Institute's Atlas of Gene Expression During Development5 and those related to heart development were identified using IPA (56). All candidate variants were validated by visualization using Integrative Genomics Viewer (IGV) and for 65 probands that additionally had genome sequencing data available, variant calls from the exome sequence data were compared against variant calls from genome sequence data previously conducted by PCGC. None of the variants identified in the PCGC cohort was identified in the SFARI cohort, further supporting that effects seen are highly unlikely to be due to random enrichment of nonpathogenic alleles. Statistical analyses were conducted either by the GO analysis tools (PANTHER, DAVID, Metascape, IPA) or using the stats package in R (Version 4.0.2) (57).
2.4. Data availability
The data and study materials are under restricted access, as the data use agreements with PCGC and SFARI prohibit distribution of patient-level data, but upon application may be available to other researchers for the purposes of reproducibility or replicating the procedure. Data can be requested from both PCGC6 and SFARI7 by researchers. Exome sequencing data from PCGC was accessed via dbGaP authorized access of study phs001194.v3.p2 and exome sequencing data from SFARI was collected from the Simons Foundation Powering Autism Research for Knowledge (SPARK) project.
3. Results
3.1. Characteristics of study sample
Of the 362 patients in the final TOF cohort more individuals were assigned male at birth (n = 203) than female (n = 159; 56.08% vs. 43.92%). As these are just the cases of TOF that had exome sequencing data for the proband and both parents and the associated FASTQ files for all three passed initial quality metrics, this does not mean there is such a discrepancy amongst the total non-syndromic TOF population in the PCGC database. When looking at all non-syndromic conotruncal defects from PCGC however, a similar proportion was described with 57.6% described as male and 42.4% female (58). A similar breakdown was achieved in the SFARI cohort of 317 unaffected siblings of children with autism (53% male vs. 47% female). The racial and ethnic composition of the TOF cohort (Supplementary Figure 1A) is skewed more heavily white (83.1% vs. 72%), with the heaviest differences coming from far fewer Black individuals (5.8% vs. 13%) compared to the U.S. population at the time of sample collection and no individuals in our TOF cohort identified as American Indian, Alaska Native, Native Hawaiian or Pacific Islander. Unlike participants in PCGC's database, those in the SPARK project for SFARI did not have race or ethnicity data collected. Instead HapMap haplotypes were utilized to categorize ancestral background. The SFARI control cohort ancestral superclass composition was 3.42% African, 9.63% Admixed American, 1.24% East Asian, 79.81% European, 1.86% South Asian and 4.04% unknown ancestral superclass. While one cannot presume that the 79.81% EUR population is entirely white, the probability is high that the control cohort like the TOF cohort is predominately white.
3.2. Variant rate and characteristics of variants
The de novo mutation rate in the TOF cohort was 0.33 per proband for variants with a CADD score of 20 or greater (120 variants, 117 genes, 105 probands) and 0.56 per proband for all variants annotated as moderate or high impact by snpEff (183 variants, 179 genes, 147 probands; Supplementary Table 1). All variants identified after filtering are listed in Supplementary Table 1, with those with the 15 highest CADD scores listed in Table 1. The de novo mutation rate in our control cohort was 0.31 (97 variants, 97 genes, 82 probands) for those with a CADD score of 20 or greater and 0.51 overall (161 variants, 161 genes, 123 probands), neither of which was statistically different from the TOF cohort (Chi-square p = 0.88 and p = 0.47 respectively).
Examining variants with a CADD score of 20 or greater in the PCGC TOF probands, 11.67% were LoF mutations and 88.33% D-Miss/Indel, compared to all variants where 14.75% were LoF and 85.25% D-Miss/Indel (Supplementary Table 2). By comparison, there were significantly fewer LoF variants with a CADD score of 20 or greater in the SFARI control cohort (Supplementary Figure 1C 4.12%, 4/97, OR 3.07, 95% CI 0.98–9.66, p = 0.05). There was no statistical difference in LoF variants between the two cohorts when examining all variants with moderate/high impact from snpEff alone (13.04%, 21/161, OR 1.15, 95% CI 0.62–2.13, p = 0.65). An evaluation of gene expression of candidate genes using the Brotman Baty Institute's Atlas demonstrated that more than half of all genes affected both in the CADD 20 analysis (50.43%, 59/117) and analysis of all variants (53.63%, 96/179) were found to be expressed in more than 1% of fetal cardiac tissue (56). This is significantly more than the number of genes affected in the control cohort, where only slightly over a third of genes were found to be expressed in the fetal heart in both analyses (CADD 20 analysis: 37.11%, 36/97, OR 1.72, 95% CI 0.99–2.98, p = 0.05; overall analysis: 36.02%, 58/161, OR 2.05, 95% CI 1.33–3.18, p = 0.001).
GO analysis found CHD (7-fold enrichment, p = 5.01 × 10−7, Benjamini-Hochberg (B–H) p = 0.003) to be enriched in our analysis, including TOF (8.5-fold enrichment, p = 1.26 × 10−6, B–H p = 0.004) and CHARGE syndrome (a syndromic form of TOF; 21-fold enrichment, p = 3.98 × 10−5, B–H p = 0.04; Figure 2, Table 2). Only CHD was significant in the analysis of all variants (5-fold enrichment, p = 6.31 × 10−6, B–H p = 0.02). According to IPA Disease and Function analysis there was also a significant overlap with genes involved in morphogenesis of the cardiovascular system (p = 1.94 × 10−6, B–H p = 5.69 × 10−5) and congenital anomaly of the cardiovascular system (p = 1.1 × 10−6, B–H p = 3.03 × 10−5). Overlap with these genes was less significant in the analysis of all variants (morphogenesis p = 8.57 × 10−5, B–H p = 1.67 × 10−3; anomaly p = 3.39 × 10−4, B–H = 5.85 × 10−3). The control cohort did not have overlap with any of these terms related to CHD or cardiac development.
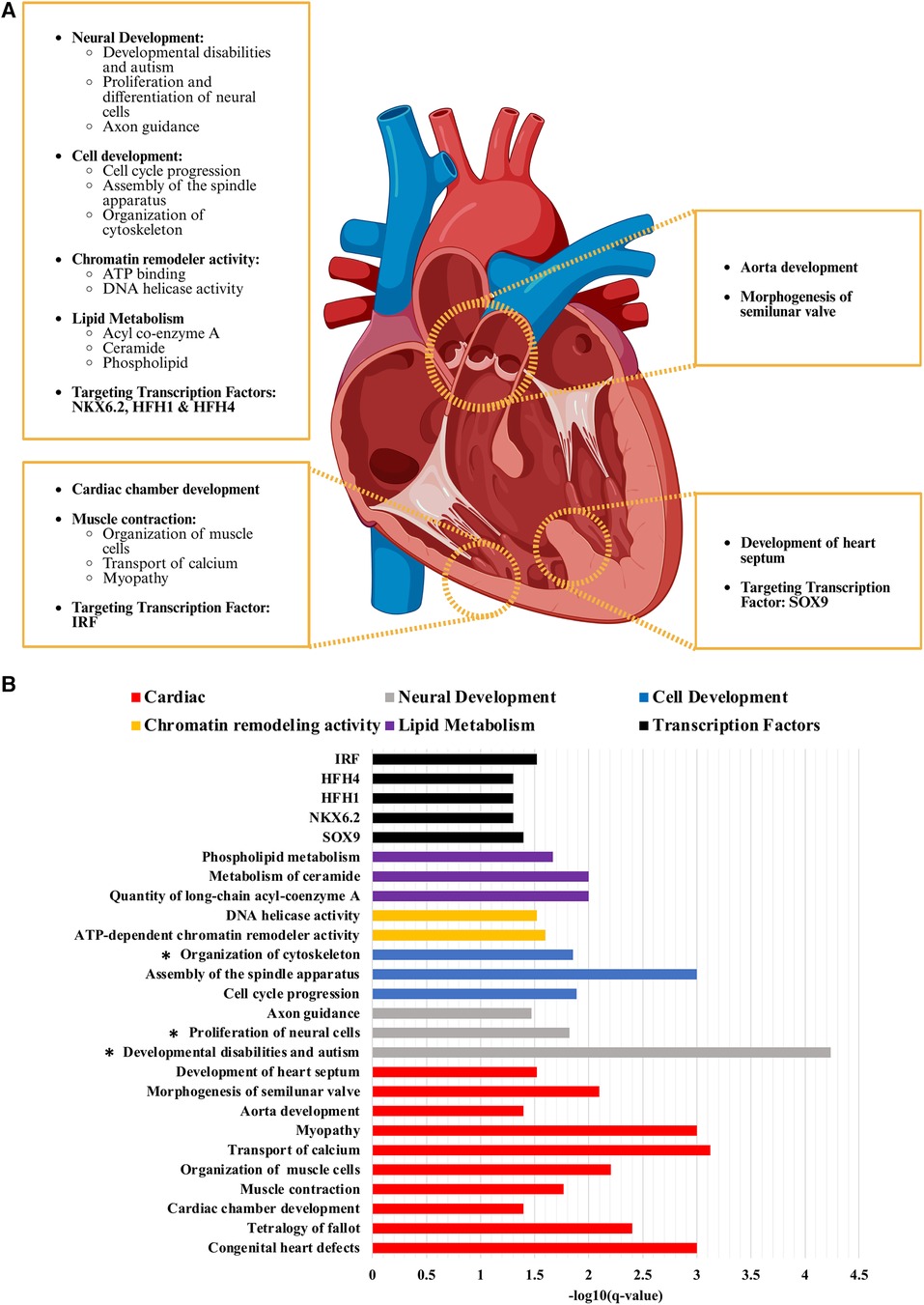
Figure 2. Critical pathways and biological processes enriched in non-syndromic tetralogy of Fallot. (A) While there are a variety of pathways and processes enriched in the analyses conducted on the de novo variants with a CADD score of 20 or greater identified from our cohort of non-syndromic tetralogy of Fallot, the most relevant can be considered in four main categories: those affecting the ventricle, the outflow tract, the ventricular septum, and extracardiac or more general pathways and processes (i.e. neural development, cell cycle progression). (B) The scale of how significantly enriched each term was determined by taking the negative log10 value of the B–H p-value (q-value) which has been corrected for multiple comparison testing. Cardiac related terms had an average -log10 of 2.19 ± 0.21 (S.E.M.), neural development 2.51 ± 0.87, cell development 2.25 ± 0.38, chromatin remodelling activity 1.56 ± 0.04, lipid metabolism 1.89 ± 0.11 and the transcription factor targets 1.36 ± 0.05. Figure generated with Biorender.com.
A total of four genes were mutated in two probands in the TOF cohort: CHD7, CLUH, UNC13C, and WASHC5 (Table 3). CHD7 is a helicase critical in chromatin remodeling, CLUH is a mRNA binding protein involved in mitochondrial regulation, UNC13C is a membrane fusion protein involved in vesicle maturation and synaptic transmission and WASHC5 is a component of the WASH complex that functions in endosomes (59–62). Variants in these genes were annotated as having a high impact by snpEff or an average CADD score greater than 20. No variant was identified in more than two probands. Of the genes mutated in two probands, WASHC5, CLUH and CHD7 were identified to be expressed in the heart, of which WASCH5 and CHD7 have previously been identified to be related to CHD pathogenesis, including syndromic cases of TOF (8, 11, 14, 31, 63–65). Our findings further the case that these genes are also important in non-syndromic TOF. CLUH and UNC13C represent novel candidate genes that have not been previously associated with TOF pathogenesis, but are of high interest due to their observed perturbation in more than one unrelated probands.
3.3. Confirmation of previous findings in tetralogy of Fallot genomic studies
The rate of likely damaging de novo variants (0.56) in our study is comparable to other studies of rare, de novo variants in cohorts of children with CHD (31, 66). Our analysis also identified variants in genes, pathways and biological processes previously identified in other studies of TOF. CHD7, JAG1, and WASHC5 have been implicated in TOF, and ATP2A2, CHD4, DNAH6, DTNA, EDNRA, FAT4, MED13l, RASA1, and SLC5A2 in CHD generally according to the literature and our IPA analysis (Supplementary Table 3) (8, 10, 11, 14, 35, 63, 64, 66, 67). A number of these genes have been established to be critical to the progenitor populations necessary for heart development: first and second heart field derived cells and cardiac neural crest cells. EDNRA is expressed for instance in the first heart field and EDNRA null mice have hypoplastic ventricles (68). MED13l is expressed in migratory cardiac neural crest cells, is heavily associated with transposition of the great arteries and coarctation and in cardiac energy metabolism and apoptosis (69). FAT4 is expressed in cardiac mesoderm that produces both the first and second heart fields and has been demonstrated to regulate cardiac progenitor proliferation and planar cell polarity (70). Four genes had de novo variants that were found in multiple probands in our cohort, of which two (CHD7 and WASHC5) are in genes previously implicated in TOF (Table 3). This finding speaks both to the centrality of these genes in TOF biology and the ability of our analysis to detect variants with high likelihood for pathogenicity.
Many exome sequencing studies of TOF have identified an enrichment for variants in genes related to autism, chromatin remodeling, cilia and ciliopathy, VEGF signaling, Notch signaling, and the Wnt/β-catenin pathway (71–77). Chromatin remodeler activity (19.61-fold enrichment, p = 7.54 × 10−5, B–H p = 0.025) was indeed found to be enriched in our cohort (Table 2), as was a significant relationship found for genes related to autism spectrum disorder and intellectual disability (p = 2.18 × 10−6, B–H p = 5.74 × 10−5). It is worthy to note that autism spectrum disorder and intellectual disability (p = 0.003, B–H p = 0.03) was also found to be enriched in our control cohort though to a lesser degree. This may be in relation to the large number of genes encompassed in this disease (SFARI's Gene database lists 942 genes as of 2022) and a reflection of the disagreement between datasets and research groups on what true risk genes are for autism spectrum disorder and intellectual disability (78–80). Ciliary body morphogenesis (GO:0061073; 42.89-fold enrichment, p = 0.03, B–H p = 1.00), the Notch signaling pathway (P00045; 7.63-fold enrichment, p = 0.03, B–H p = 0.81) and the Wnt signaling pathway (P00057; 3.82-fold enrichment, p = 0.003, B–H p = 0.43) were found to be enriched in the variants called in our TOF cohort; however, these pathways were not significant after correction for multiple comparison testing.
3.4. Pathways and biological processes identified with pathogenic potential
Molecular function GO analysis demonstrated that ATP binding (2.75-fold enrichment, p = 5.61 × 10−6, B–H p = 0.005) and DNA helicase activity (11.59-fold enrichment, p = 9.62 × 10−5, B–H p = 0.03) were found to be enriched in our identified variants with a CADD score of 20 or greater (Figure 2, Table 2). Both terms were also significantly enriched in the analysis of all variants, in addition to meiotic cell cycle (GO:0051321; 6.83-fold enrichment, p = 2.40 × 10−6, B–H p = 0.05). Biological function GO analysis elicited that aorta development (21.9-fold enrichment, p = 3.39 × 10−6, B–H p = 0.04), cardiac chamber development (10.9-fold enrichment, p = 4.00 × 10−6, B–H p = 0.04), and general cell development (2.78-fold enrichment, p = 6.19 × 10−7, B–H p = 0.01) were significantly enriched. By comparison, no functional GO terms were significantly enriched in the analysis of all variants. Diseases identified to be enriched related to our variants are myopathy (5.7-fold enrichment, p = 1.58 × 10−7, B–H p = 0.001), congenital ear anomaly (13-fold enrichment, p = 1.00 × 10−6, B–H p = 0.004) and developmental disabilities (7.3-fold enrichment, p = 1.26 × 10−6, B–H p = 0.004).
IPA analysis detected a significant overlap with genes involved in cell development, neural development, muscle development and function, lipid metabolism, hearing impairment (p = 5.93 × 10−6, B–H p = 1.42 × 10−4), perinatal death (p = 6.53 × 10−5, B–H p = 0.001), development of the body axis (p = 1.16 × 10−4, B–H p = 0.002), morphogenesis of semilunar valve (p = 5.3 × 10−4, B–H p = 0.008), and development of the heart septum (p = 0.003, B–H p = 0.03). Processes involved in cell development include assembly of the spindle apparatus (p = 7.13 × 10−5, B–H p = 0.001), adhesion of cell-associated matrix (p = 1.87 × 10−4, B–H p = 0.003), cell cycle progression (p = 9.81 × 10−4, B–H p = 0.013), and organization of cytoskeleton (p = 0.001, B–H p = 0.014). Included in neurocognitive development are familial central nervous system disease (p = 6.14 × 10−6, B–H p = 1.46 × 10−4), proliferation of neural cells (p = 0.001, B–H p = 0.015), innervation of neurons (p = 0.001, B–H p = 0.016), growth of axons (p = 0.0015, B–H p = 0.018), and guidance of axons (p = 0.003, B–H p = 0.034). The analysis of all variants revealed an additional enrichment for differentiation of neural cells (p = 6.95 × 10−4, B–H p = 0.05). Related to muscle development and function are transport of calcium (p = 3.76 × 10−5, B–H p = 7.53 × 10−4), organization of muscle cells (p = 4.09 × 10−4, B–H p = 0.006), and muscle contraction (p = 0.001, B–H p = 0.017). We have grouped calcium transport into the category of muscle development and function as we believe that it is distinctly tied to dysfunction of the sarcoplasmic reticulum. ATP2A2 and RYR1 are shared between calcium transport, muscle contraction and organization of muscle, with ANXA6 and EDNRA additionally shared between calcium transport and muscle contraction. While not significant after multiple comparison testing, both calcium regulation in cardiac cells (WP536; 8.49-fold enrichment, p = 3.24 × 10–4, B–H p = 0.25) and sarcoplasmic reticulum calcium ion transport (GO:0070296; 22.88-fold enrichment, p = 0.004, B–H p = 0.65) specifically were enriched. Related to lipid metabolism, quantity of long-chain acyl-coenzyme A (p = 8.57 × 10−4, B–H p = 0.01) and metabolism of ceramide (p = 9.52 × 10−4, B–H p = 0.01) were found to be significant, with the additional terms of accumulation of phosphatidylinositol 3,4-diphosphate (p = 0.001, B–H p = 0.02), accumulation of phospholipid (p = 0.002, B–H p = 0.02), and accumulation of phosphoinositide (p = 0.004, B–H p = 0.05) significantly enriched in the analysis of all variants. A majority of the enrichments from the CADD 20 analysis were found in the IPA analysis of all variants (Supplementary Table 3). Of note, terms associated with calcium handling and muscle contraction were only identified in the CADD 20 analysis (concentration of Ca+2, liberation of Ca+2, oscillation of Ca+2, transmembrane transport of Ca+2, formation of muscle, hereditary myopathy, and muscle contraction; p-values and B–H values in Supplementary Table 3), as was development of heart septum, demonstrating the critical nature of the CADD 20 analysis.
Of final note, there was also significant enrichment of target genes of four different transcription factors, all of which have previously been tied to cardiac development or injury response. The most significant is SOX9 (7.6-fold enrichment, p = 3.98 × 10−5, B–H p = 0.04), which coincidentally is the only transcription factor that has been previously reported in relation to TOF and is critical for ventricular septum formation (81, 82). NKX6.2 (7.3-fold enrichment, p = 5.01 × 10−5, B–H p = 0.05) has been previously tied to CHD. While no specific role of NKX6.2 in cardiac development has been identified, the Nkx family (particularly NKX2.5) plays a critical role in specification of the second heart field pharyngeal mesoderm (83, 84). HFH1 (7.2-fold enrichment, p = 6.31 × 10−5, B–H p = 0.05), also known as FOXQ1, is involved in cell proliferation, differentiation, and myocardial fibrosis, though it has mainly been studied in cancer (85, 86). HFH4 (6.9-fold enrichment, p = 7.94 × 10−5, B–H p = 0.05) has been implicated in ciliogenesis. This process by itself is not enriched in our TOF cohort but has been reported previously in other genomic analyses of TOF and left-right body axis formation, which we demonstrated is enriched in our cohort (34, 87). In contrast, none of the four transcription factors enriched in the CADD 20 variant list is significant after multiple comparison correction in the overall list. This again demonstrates the importance of the CADD 20 analysis in identifying the variants and their associated pathways that would not be elucidated from the analysis of all de novo non-synonymous variants in the cohort. IRF is the only transcription factor of significance (6.2-fold enrichment, p = 1.58 × 10−5, B–H p = 0.03). IRF is enriched in the CADD 20 analysis (5.3-fold enrichment, p = 0.003, B–H p = 0.46), though not after correction for multiple comparison testing. IRF is predominately connected to cardiac biology through its role in cardiac fibrosis, ventricular remodeling, and heart failure (88, 89, 90). The fact it is significantly enriched in the larger analysis, that it has a functional similarity to HFH1/4, and the stringency of our various filtering metrics warrants further consideration of its role in TOF pathogenesis and prognosis.
Enrichment analyses of the control cohort resulted in no identified enriched pathways outside of analysis with IPA. The only overlap in the IPA analysis was for enrichment in growth of axons (p = 5.47 × 10−4, B–H p = 0.009), proliferation of neural cells (p = 0.002, B–H p = 0.02), organization of cytoskeleton (p = 0.005, B–H p = 0.04), and autism spectrum disorder or intellectual disability (p = 0.003, B–H p = 0.03). While growth of axons was more significantly enriched than in the TOF cohort, all other terms were enriched to a lesser degree than the TOF cohort. Whether or not these should not be considered as potentially implicated in TOF pathogenesis requires further study.
4. Discussion
This work is the largest exome sequencing rare, de novo variant analysis to date focused exclusively on non-syndromic TOF, a critical step to addressing the yet unknown pathogenesis underlying the most prevalent cyanotic heart defect. Our analysis revealed a significant enrichment of variants in pathways involved in aortic and cardiac chamber development, and CHD, including a specific enrichment for TOF. Further, a high percentage of deleterious variants were observed in genes expressed in the fetal heart. Similar to prior studies, our work also identified the importance of chromatin remodeling genes in cardiac development and overlap with genes involved in autism spectrum disorder and neurodevelopmental disorders (71, 75).
While this study is focused on the crucial nature of pathways and processes found to be significantly enriched in our TOF cohort to elucidate knowledge about its etiology, the potential critical nature of the four genes found to be mutated in multiple probands should not be ignored. CHD7 is involved in cardiac development via neural crest cells for which it has been described as critical for their migration, a critical function related to their role in cardiac outflow tract development (59, 91, 92). CHD7 also plays a critical role in the transition of cardiac specified mesoderm becoming the second heart field, including sharing numerous enhancer sites with the critical second heart field gene ISL1 (93). The second heart field importantly is the primary progenitor population responsible for outflow tract development. WASHC5, previously known as KIAA0196, is responsible for production of strumpellin and has been implicated not only in heart development, but also in motor neuron function (62). CLUH and UNC13C represent novel, putative candidate genes involved in TOF pathogenesis. CLUH is an RNA binding protein critical in regulating mitochondrial fission and both oxidative phosphorylation and fatty acid metabolism in the mitochondria (60, 94). Cardiomyocyte development relies on a switch from dependence on glycolysis to oxidative phosphorylation during embryonic development and eventually fatty acid oxidation during late fetal and postnatal development, a lack of which is incompatible with cardiomyocyte differentiation and maturation (95–97). A cessation of mitochondrial fission is additionally required for cardiomyocyte differentiation (98). The critical nature of these changes (demonstrated both in rodent models and stem cell models of cardiac development) may explain the potential role for CLUH in TOF pathogenesis, as a lack of cardiac differentiation would explain the structural defects that define TOF (99). An alternative hypothesis is that mitophagy has also been demonstrated to begin upon differentiation of stem cells to cardiac progenitors and is necessary for proper mitochondrial network formation, cellular stress response and cell survival (100). Knockdown of CLUH has been demonstrated to cause a block of mitophagy (101). While CLUH knockout mice have been reported to have phenotypically normal hearts, the knockout was associated with neonatal lethality (94). UNC13C is involved in membrane fusion and is a central player in synaptic transmission processes, including calcium coupling (61). UNC13C's centrality in synaptic transmission adds to the evidence of a potential link directly between CHD and nervous system development and autism which has also been associated with synaptic dysfunction (102).
We believe that the critical underlying thread shared throughout the pathways and biological processes found to be significantly enriched in our cohort is a vulnerability related to cell cycle progression, differentiation and typical organization that prohibits formation of the ventricular septum and proper function of the ventricle. Animal models of tetralogy-type double outlet right ventricle (DORV) provide mechanistic insights into the role of progenitor cell proliferation and organization in this defect (19, 20, 103). When there is an inadequate proliferation of second heart field and cardiac neural crest progenitor cells, there is a reduction in the pool of cells available to incorporate into and elongate the developing cardiac outflow tract, resulting in improper alignment (104, 105). A foreshortened outflow tract, unable to appropriately align over the two ventricles, results in TOF-type defects (19, 20). Cellular organization is also crucial for elongation and, more importantly, appropriate rotation of the developing outflow tract. Early results in these animal models indicate a close interaction between neural crest cells and second heart field progenitors that regulates polarized migration of cells into the outflow tract for its appropriate elongation and rotation (103). Our human data in the current study supports this hypothesis as indicated by significant overlap of pathways associated with cell proliferation and with genes necessary for normal ventricle, outflow tract and myocyte development that are expressed in the first and second heart field, such as EDNRA and FAT4, and migratory cardiac neural crest cells, such as MED13l. In this regard, the enrichment for target genes of SOX9 is particularly intriguing, as loss of SOX9 has been demonstrated to result in TOF in murine models via a loss of second heart field contribution to outflow tract mesenchyme resulting in a perturbation of the proximal outflow tract septum and an associated membranous ventricular septal defect (81). This loss of SOX9 was also associated with cleft palate in addition to skeletal malformations and sex reversal. We have shown that the most common heart defect over-represented in children with cleft disease is TOF (106). Furthermore, in our focused genome analysis of children with concomitant cleft and outflow tract congenital heart defect, we identified a de novo mutation in the MED12 gene as likely pathogenic (107), and this gene is known to interact with SOX9. Finally, in relation to the importance of second heart field biology, a potential link may exist with the enrichment found in lipid metabolism. While deficiencies in maternal phospholipid and fatty acid metabolism have been associated with CHD, with potential implications specifically for maternal diabetes related CHD, a direct relationship between fetal lipid metabolism and cardiac development has not yet been established (108). Cell membrane lipid composition and lipid metabolism, however, have been demonstrated to be of critical importance in pathways critical both to second heart field development and TOF pathogenesis, Notch and Wnt signaling (109–113).
Our analysis also identified variants that impacted processes related to sarcoplasmic reticulum dysfunction (muscle cell organization, calcium handling, and contraction) and thereby, may impact ventricular function. A prior study of targeted sequencing of 22 families with non-syndromic TOF probands also identified sarcomere dysfunction as enriched in their cohort (72). Only one shared gene (MYOM2) was affected in our cohort and theirs. Such an overlap has been previously observed in the pathogenesis of nemaline myopathy in skeletal muscle, where there is association between myopathy, sarcomere function, and contractile deficits (114). However, a defect in cardiomyocyte contraction and sarcomere function is particularly interesting in the context of care of patients with TOF. Following complete surgical repair of TOF, a subset of patients develops right ventricular dilation and dysfunction over time. It would therefore be interesting if there is correlation between these clinical outcomes and any of the genetic abnormalities identified in our analysis. Early identification of patients with deleterious variants in pathways related to ventricular function could allow for more personalized clinical care approaches in the future.
As in other genetic studies of CHD, this study demonstrates an overlap between genes involved in autism spectrum disorder and intellectual disabilities and TOF (71, 75). The basis for this overlap is unclear, and additional research is required to understand if there are shared molecular pathways between organization of myocyte and axon cytoarchitecture. There appears to be a significant enrichment for variants involved in neural cell progenitor proliferation and differentiation. In addition to the effects these genes/variants may have in brain development, it is an intriguing possibility that these may also impact cardiac neural crest cells and may be of relevance in heart development. Lastly, what effect these genetic variants in neurons have on neurologic outcomes in children with TOF has important clinical implications. This is particularly relevant for a disease like TOF, where cyanosis is ubiquitous in early infancy and surgical repair requires the use of cardiopulmonary bypass with its attendant adverse effects on the developing nervous system.
There are distinct limitations to our study in relation to its demographic composition and overall size. The demographics of our study cohort, like most studies in biomedical research, were skewed toward a white population, including no reported American Indian, Alaska Native, Native Hawaiian or Pacific Islander individuals. Due to documented methodologic inadequacies and structural barriers rooted in white, settler colonialism, Indigenous patients may be a part of our cohort but are simply not identified as such, as has been observed in documenting the incidence of other diseases such as COVID-19 (115, 116). This is less concerning to this analysis given its focus on de novo variants rather than germline mutations. However, continued work remains necessary to include populations most disproportionately impacted by CHD-associated morbidity and mortality in future studies (117, 118). Additionally, while there appears to be a higher number of individuals assigned male at birth in our TOF cohort than female, we are unable to make any inference from our results as to a potential sex bias in the pathology due to the filtering of individuals in the PCGC database with non-syndromic TOF that did not have exome sequencing or if one or both of their parents lacked exome sequencing. There have been conflicting reports as to whether TOF is more common amongst those assigned male at birth or if there is no significant sex-based difference (58, 119–122).
Although ours is the largest non-syndromic TOF exome sequencing study of de novo variants to date, the sample size is still limited and precludes more extensive enrichment analyses. Given the high statistical threshold required to reach significance when correcting for multiple comparison testing in pathway enrichment analysis, it is important to note that there are some processes that were identified as being enriched but not significant in our analyses after this correction. Some of these processes may still have biological relevance, as evidenced by the fact the Wnt and Notch signaling pathways have previously been identified in animal models as being associated with TOF pathology and were enriched in our study, yet neither was significant after correcting for multiple comparisons. Even with a limited sample size, we were still able to identify both novel candidate genes and pathways and processes as has been done in other limited exome sequencing studies with fewer trios than our TOF cohort (123, 124).
5. Conclusion
In summary, using exome sequencing in a cohort of 362 non-syndromic TOF patients, we demonstrate that innate molecular defects in cardiac progenitor cells related to their viability and contractile function play an important role in TOF pathogenesis. Whether and how these genetic variants impact clinical outcomes in these patients requires more focused analyses that correlate variants in these pathways to clinical outcomes and long-term complications. The critical next step is to utilize this work to experimentally study disruption of the identified pathways and biological processes, with particular attention to the genes mutated in our cohort.
Data availability statement
The data analyzed in this study is subject to the following licenses/restrictions: The data and study materials are under restricted access, as the data use agreements with PCGC and SFARI prohibit distribution of patient-level data, but upon application may be available to other researchers for the purposes of reproducibility or replicating the procedure. Requests to access these datasets should be directed to PCGC, https://www.ncbi.nlm.nih.gov/projects/gap/cgi-bin/study.cgi?study_id=phs000571.v6.p2. SFARI, https://www.sfari.org/resource/sfari-base/. Further enquiries can be directed to the corresponding author.
Ethics statement
Ethical approval was not required for the study involving humans in accordance with the local legislation and institutional requirements. Written informed consent to participate in this study was not required from the participants or the participants’ legal guardians/next of kin in accordance with the national legislation and the institutional requirements.
Author contributions
Experimental design and acquisition of data for this project was conducted by DH, RV, SM, BH, JS, and SK. Analysis of the data was performed by DH, BS, SM, BH, and SK. The initial manuscript was written by DH. All authors contributed to the article and approved the submitted version.
Funding
Study supported by R03HL154301 to SK and 1F30AA029936 to DH.
Conflict of interest
The authors declare that the research was conducted in the absence of any commercial or financial relationships that could be construed as a potential conflict of interest.
Publisher's note
All claims expressed in this article are solely those of the authors and do not necessarily represent those of their affiliated organizations, or those of the publisher, the editors and the reviewers. Any product that may be evaluated in this article, or claim that may be made by its manufacturer, is not guaranteed or endorsed by the publisher.
Supplementary material
The Supplementary Material for this article can be found online at: https://www.frontiersin.org/articles/10.3389/fcvm.2023.1249605/full#supplementary-material
Footnotes
1https://github.com/RiyaS-Verma/VariantCalling-Snakemake
3https://david.ncifcrf.gov/home.jsp
4https://metascape.org/gp/index.html#/main/step1
6https://www.ncbi.nlm.nih.gov/projects/gap/cgi-bin/study.cgi?study_id=phs000571.v6.p2
7https://www.sfari.org/resource/sfari-base/
References
1. Zimmerman MS, Smith AGC, Sable CA, Echko MM, Wilner LB, Olsen HE, et al. Global, regional, and national burden of congenital heart disease, 1990–2017: a systematic analysis for the global burden of disease study 2017. Lancet Child Adolesc Health. (2020) 4(3):185–200. doi: 10.1016/S2352-4642(19)30402-X
2. Egbe A, Uppu S, Stroustrup A, Lee S, Ho D, Srivastava S. Incidences and sociodemographics of specific congenital heart diseases in the United States of America: an evaluation of hospital discharge diagnoses. Pediatr Cardiol. (2014) 35(6):975–82. doi: 10.1007/s00246-014-0884-8
3. Hoffman JIE, Kaplan S. The incidence of congenital heart disease. J Am Coll Cardiol. (2002) 39(12):1890–900. doi: 10.1016/S0735-1097(02)01886-7
4. Simeone RM, Oster ME, Cassell CH, Armour BS, Gray DT, Honein MA. Pediatric inpatient hospital resource use for congenital heart defects. Birth Defects Res A Clin Mol Teratol. (2014) 100(12):934–43. doi: 10.1002/bdra.23262
5. Neill CA, Clark EB. Tetralogy of Fallot. The first 300 years. Tex Heart Inst J. (1994) 21(4):272–9. PMCID: PMC325189
6. Villafañe J, Feinstein JA, Jenkins KJ, Vincent RN, Walsh EP, Dubin AM, et al. Hot topics in tetralogy of Fallot. J Am Coll Cardiol. (2013) 62(23):2155–66. doi: 10.1016/j.jacc.2013.07.100
7. Morgenthau A, Frishman WH. Genetic origins of tetralogy of Fallot. Cardiol Rev. (2018) 26(2):86–92. doi: 10.1097/CRD.0000000000000170
8. Althali NJ, Hentges KE. Genetic insights into non-syndromic tetralogy of Fallot. Front Physiol. (2022) 13. doi: 10.3389/fphys.2022.1012665
9. O'Bryne ML, Glatz AC, Huang Y, Shung V, Kelleman MS, Petit CJ, et al. Comparative costs of management strategies for neonates with symptomatic tetralogy of Fallot. J Am Coll Cardiol. (2022) 79(12):1170–80. doi: 10.1016/j.jacc.2021.12.036
10. McElhinney DB, Krantz ID, Bason L, Piccoli DA, Emerick KM, Spinner NB, et al. Analysis of cardiovascular phenotype and genotype-phenotype correlation in individuals with a JAG1 mutation and/or alagille syndrome. Circulation. (2002) 106(20):2567–74. doi: 10.1161/01.CIR.0000037221.45902.69
11. Lalani SR, Safiullah AM, Fernbach SD, Phillips M, Bacino CA, Molinari LM, et al. SNP genotyping to screen for a common deletion in CHARGE syndrome. BMC Med Genet. (2005) 6(1):8. doi: 10.1186/1471-2350-6-8
12. Johnson MC, Hing A, Wood MK, Watson MS. Chromosome abnormalities in congenital heart disease. Am J Med Genet. (1997) 70(3):292–8. doi: 10.1002/(SICI)1096-8628(19970613)70:3%3C292::AID-AJMG15%3E3.0.CO;2-G
13. Rauch R, Hofbeck M, Zweier C, Koch A, Zink S, Trautmann U, et al. Comprehensive genotype-phenotype analysis in 230 patients with tetralogy of Fallot. J Med Genet. (2010) 47(5):321–31. doi: 10.1136/jmg.2009.070391
14. Gjerulfsen CE, Møller RS, Fenger CD, Hammer TB, Bayat A. Expansion of the CCDC22 associated Ritscher-Schinzel/3C syndrome and review of the literature: should the minimal diagnostic criteria be revised? Eur J Med Genet. (2021) 64(7):104246. doi: 10.1016/j.ejmg.2021.104246
15. Wu W, He J, Shao X. Incidence and mortality trend of congenital heart disease at the global, regional, and national level, 1990–2017. Medicine (Baltimore). (2020) 99(23):e20593. doi: 10.1097/MD.0000000000020593
16. Starr JP. Tetralogy of fallot: yesterday and today. World J Surg. (2010) 34(4):658–68. doi: 10.1007/s00268-009-0296-8
17. Valdeomillos E, Jalal Z, Metras A, Roubertie F, Benoist D, Bernus O, et al. Animal models of repaired tetralogy of fallot: current applications and future perspectives. Can J Cardiol. (2019) 35(12):1762–71. doi: 10.1016/j.cjca.2019.07.622
18. Bove T, Alipour Symakani R, Verbeke J, Bral A, El Haddad M, De Wilde H, et al. Study of the time-relationship of the mechano-electrical interaction in an animal model of tetralogy of Fallot: implications for the risk assessment of ventricular arrhythmias. Interact Cardiovasc Thorac Surg. (2020) 31(1):129–37. doi: 10.1093/icvts/ivaa047
19. De Zoysa P, Toubat O, Harvey D, Choi J, Kumar SR. Murine model of cardiac defects observed in adams-oliver syndrome driven by delta-Like Ligand-4 haploinsufficiency. Stem Cells Dev. (2021) 30(12):611–21. doi: 10.1089/scd.2021.0058
20. De Zoysa P, Liu J, Toubat O, Choi J, Moon A, Gill PS, et al. Delta-like ligand-4 mediated Notch signaling controls proliferation of second heart field progenitor cells by regulating Fgf8 expression. Development. (2020) 147:dev185249. doi: 10.1242/dev.185249
21. Yang J, Qiu H, Qu P, Zhang R, Zeng L, Yan H. Prenatal alcohol exposure and congenital heart defects: a meta-analysis. PLoS One. (2015) 10(6):e0130681. doi: 10.1371/journal.pone.0130681
22. Chen Z, Li S, Guo L, Peng X, Liu Y. Prenatal alcohol exposure induced congenital heart diseases: from bench to bedside. Birth Defects Res. (2021) 113:521–34. doi: 10.1002/bdr2.1743
23. Cavieres MF, Smith SM. Genetic and developmental modulation of cardiac deficits in prenatal alcohol exposure. Alcohol Clin Exp Res. (2000) 24(1):102–9. doi: 10.1111/j.1530-0277.2000.tb04559.x
24. Akhirome E, Walton NA, Nogee JM, Jay PY. The complex genetic basis of congenital heart defects. Circ J. (2017) 81(5):629–34. doi: 10.1253/circj.CJ-16-1343
25. Basu M, Garg V. Maternal hyperglycemia and fetal cardiac development: clinical impact and underlying mechanisms. Birth Defects Res. (2018) 110(20):1504–16. doi: 10.1002/bdr2.1435
26. Harvey DC, De Zoysa P, Toubat O, Kishore J, Choi J, Kumar SR. Concomitant genetic defects potentiate the adverse impact of prenatal alcohol exposure on cardiac outflow tract maturation. Birth Defects Res. (2022) 114(3-4):105–11. doi: 10.1002/bdr2.1968
27. Töpf A, Griffin HR, Glen E, Soemedi R, Brown DL, Hall D, et al. Functionally significant, rare transcription factor variants in tetralogy of fallot. PLoS One. (2014) 9(8):e95453. doi: 10.1371/journal.pone.0095453
28. Kelly RG, Buckingham ME, Moorman AF. Heart fields and cardiac morphogenesis. Cold Spring Harb Perspect Med. (2014) 4(10):a015750. doi: 10.1101/cshperspect.a015750
29. Verzi MP, McCulley DJ, de Val S, Dodou E, Black BL. The right ventricle, outflow tract, and ventricular septum comprise a restricted expression domain within the secondary/anterior heart field. Dev Biol. (2005) 287(1):134–45. doi: 10.1016/j.ydbio.2005.08.041
30. Waldo KL, Hutson MR, Stadt HA, Zdanowicz M, Zdanowicz J, Kirby ML. Cardiac neural crest is necessary for normal addition of the myocardium to the arterial pole from the secondary heart field. Dev Biol. (2005) 281(1):66–77. doi: 10.1016/j.ydbio.2005.02.011
31. Zaidi S, Choi M, Wakimoto H, Ma L, Jiang J, Overton JD, et al. De novo mutations in histone-modifying genes in congenital heart disease. Nature. (2013) 498(7453):220–3. doi: 10.1038/nature12141
32. Richter F, Morton SU, Kim SW, Kitaygorodsky A, Wasson LK, Chen KM, et al. Genomic analyses implicate noncoding de novo variants in congenital heart disease. Nat Genet. (2020) 52(8):769–77. doi: 10.1038/s41588-020-0652-z
33. Glessner JT, Bick AG, Ito K, Homsy JG, Rodriguez-Murillo L, Fromer M, et al. Increased frequency of de novo copy number variants in congenital heart disease by integrative analysis of single nucleotide polymorphism array and exome sequence data. Circ Res. (2014) 115(10):884–96. doi: 10.1161/CIRCRESAHA.115.304458
34. Watkins WS, Hernandez EJ, Wesolowski S, Bisgrove BW, Sunderland RT, Lin E, et al. De novo and recessive forms of congenital heart disease have distinct genetic and phenotypic landscapes. Nat Commun. (2019) 10(1):4722. doi: 10.1038/s41467-019-12582-y
35. Page DJ, Miossec MJ, Williams SG, Monaghan RM, Fotiou E, Cordell HJ, et al. Whole exome sequencing reveals the Major genetic contributors to nonsyndromic tetralogy of Fallot. Circ Res. (2019) 124(4):553–63. doi: 10.1161/CIRCRESAHA.118.313250
36. Gelb B, Brueckner M, Chung W, Goldmuntz E, Kaltman J, Kaski JP, et al. The congenital heart disease genetic network study: rationale, design, and early results. Circ Res. (2013) 112(4):698–706. doi: 10.1161/CIRCRESAHA.111.300297
37. Banerjee-Basu S, Packer A. SFARI Gene: an evolving database for the autism research community. Dis Model Mech. (2010) 3(3-4):133–5. doi: 10.1242/dmm.005439
38. Feliciano P, Daniels AM, Green Snyder L, Beaumont A, Camba A, Esler A, et al. SPARK: a US cohort of 50,000 families to accelerate autism research. Neuron. (2018) 97(3):488–93. doi: 10.1016/j.neuron.2018.01.015
39. Andrews S. FastQC: A Quality Control Tool for High Throughput Sequence Data. http://www.bioinformatics.babraham.ac.uk/projects/fastqc/
40. Ewels P, Magnusson M, Lundin S, Käller M. MultiQC: summarize analysis results for multiple tools and samples in a single report. Bioinformatics. (2016) 32(19):3047–8. doi: 10.1093/bioinformatics/btw354
41. McKenna A, Hanna M, Banks E, Sivachenko A, Cibulskis K, Kernytsky A, et al. The genome analysis toolkit: a MapReduce framework for analyzing next-generation DNA sequencing data. Genome Res. (2010) 20(9):1297–303. doi: 10.1101/gr.107524.110
42. Van der Auwera GA, Carneiro MO, Hartl C, Poplin R, del Angel G, Levy-Moonshine A, et al. From FastQ data to high-confidence variant calls: the genome analysis toolkit best practices pipeline. Curr Protoc Bioinformatics. (2013) 43(1):11.10.1–11.10.33. doi: 10.1002/0471250953.bi1110s43
43. Cingolani P, Platts A, Wang LL, Coon M, Nguyen T, Wang L, et al. A program for annotating and predicting the effects of single nucleotide polymorphisms. SnpEff. Fly (Austin). (2012) 6(2):80–92. doi: 10.4161/fly.19695
44. Cingolani P, Patel VM, Coon M, Nguyen T, Land SJ, Ruden DM, et al. Using Drosophila melanogaster as a model for genotoxic chemical mutational studies with a new program, SnpSift. Front Genet. (2012) 3:1–9. doi: 10.3389/fgene.2012.00035
45. Wang K, Li M, Hakonarson H. ANNOVAR: functional annotation of genetic variants from high-throughput sequencing data. Nucleic Acids Res. (2010) 38(16):e164–e164. doi: 10.1093/nar/gkq603
46. Bahcall OG. ExAC boosts clinical variant interpretation in rare diseases. Nat Rev Genet. (2016) 17(10):584–584. doi: 10.1038/nrg.2016.121
47. Rentzsch P, Witten D, Cooper GM, Shendure J, Kircher M. CADD: predicting the deleteriousness of variants throughout the human genome. Nucleic Acids Res. (2019) 47(D1):D886–94. doi: 10.1093/nar/gky1016
48. Mölder F, Jablonski KP, Letcher B, Hall MB, Tomkins-Tinch CH, Sochat V, et al. Sustainable data analysis with snakemake. F1000Res. (2021) 10:33. doi: 10.12688/f1000research.29032.2
49. Koster J, Rahmann S. Snakemake–a scalable bioinformatics workflow engine. Bioinformatics. (2012) 28(19):2520–2. doi: 10.1093/bioinformatics/bts480
50. Fuentes Fajardo KV, Adams D, Mason CE, Sincan M, Tifft C, Toro C, et al. Detecting false-positive signals in exome sequencing. Hum Mutat. (2012) 33(4):609–13. doi: 10.1002/humu.22033
51. Thomas PD, Ebert D, Muruganujan A, Mushayahama T, Albou L, Mi H. PANTHER: making genome-scale phylogenetics accessible to all. Protein Sci. (2022) 31(1):8–22. doi: 10.1002/pro.4218
52. Mi H, Muruganujan A, Huang X, Ebert D, Mills C, Guo X, et al. Protocol update for large-scale genome and gene function analysis with the PANTHER classification system (v.14.0). Nat Protoc. (2019) 14(3):703–21. doi: 10.1038/s41596-019-0128-8
53. Sherman BT, Hao M, Qiu J, Jiao X, Baseler MW, Lane HC, et al. DAVID: a web server for functional enrichment analysis and functional annotation of gene lists (2021 update). Nucleic Acids Res. (2022) 50(W1):W216–21. doi: 10.1093/nar/gkac194
54. Krämer A, Green J, Pollard J, Tugendreich S. Causal analysis approaches in ingenuity pathway analysis. Bioinformatics. (2014) 30(4):523–30. doi: 10.1093/bioinformatics/btt703
55. Zhou Y, Zhou B, Pache L, Chang M, Khodabakshi AH, Tanaseichuk O, et al. Metascape provides a biologist-oriented resource for the analysis of systems-level datasets. Nat Commun. 2019;10(1):1523. doi: 10.1038/s41467-019-09234-6
56. Cao J, O'Day DR, Pliner HA, Kingsley PD, Deng M, Daza RM, et al. A human cell atlas of fetal gene expression. Science. (2020) 370(6518):eaba7721. doi: 10.1126/science.aba7721
58. Hoang TT, Goldmuntz E, Roberts AE, Chung WK, Kline JK, Deanfield JE, et al. The congenital heart disease genetic network study: cohort description. PLoS One. (2018) 13(1):e0191319. doi: 10.1371/journal.pone.0191319
59. Okuno H, Mihara FR, Ohta S, Fukuda K, Kurosawa K, Akamatsu W, et al. CHARGE Syndrome modeling using patient-iPSCs reveals defective migration of neural crest cells harboring CHD7 mutations. Elife. (2017) 6:e21114. doi: 10.7554/eLife.21114
60. Yang H, Sibilla C, Liu R, Yun J, Hay BA, Blackstone C, et al. Clueless/CLUH regulates mitochondrial fission by promoting recruitment of Drp1 to mitochondria. Nat Commun. (2022) 13(1):1582. doi: 10.1038/s41467-022-29071-4
61. Dittman JS. Unc13: a multifunctional synaptic marvel. Curr Opin Neurobiol. (2019) 57:17–25. doi: 10.1016/j.conb.2018.12.011
62. Clemen CS, Schmidt A, Winter L, Canneva F, Wittig I, Becker L, et al. N471d WASH complex subunit strumpellin knock-in mice display mild motor and cardiac abnormalities and BPTF and KLHL11 dysregulation in brain tissue. Neuropathol Appl Neurobiol. (2022) 48(1):e12750. doi: 10.1111/nan.12750
63. Jin SC, Homsy J, Zaidi S, Lu Q, Morton S, DePalma SR, et al. Contribution of rare inherited and de novo variants in 2,871 congenital heart disease probands. Nat Genet. (2017) 49(11):1593–601. doi: 10.1038/ng.3970
64. Ji W, Ferdman D, Copel J, Scheinost D, Shabanova V, Brueckner M, et al. De novo damaging variants associated with congenital heart diseases contribute to the connectome. Sci Rep. (2020) 10(1):7046. doi: 10.1038/s41598-020-63928-2
65. Bittel DC, Zhou XG, Kibiryeva N, Fiedler S, O'Brien JE Jr, Marshall J, et al. Ultra high-resolution gene centric genomic structural analysis of a non-syndromic congenital heart defect, tetralogy of fallot. PLoS One. (2014) 9(1):e87472. doi: 10.1371/journal.pone.0087472
66. Sevim Bayrak C, Zhang P, Tristani-Firouzi M, Gelb BD, Itan Y. De novo variants in exomes of congenital heart disease patients identify risk genes and pathways. Genome Med. (2020) 12(1):1–18. doi: 10.1186/s13073-019-0709-8
67. Pasipoularides A. The new era of whole-exome sequencing in congenital heart disease: brand-new insights into rare pathogenic variants. J Thorac Dis. (2018) 10:S1923–9. doi: 10.21037/jtd.2018.05.56
68. Asai R, Kurihara Y, Fujisawa K, Sato T, Kawamura Y, Kokubo H, et al. Endothelin receptor type A expression defines a distinct cardiac subdomain within the heart field and is later implicated in chamber myocardium formation. Development. (2010) 137(22):3823–33. doi: 10.1242/dev.054015
69. Zhou W, Cai H, Li J, Xu H, Wang X, Men H, et al. Potential roles of mediator Complex subunit 13 in cardiac diseases. Int J Biol Sci. (2021) 17(1):328–38. doi: 10.7150/ijbs.52290
70. Ragni CV, Diguet N, Le Garrec JF, Novotova M, Resende TP, Pop S, et al. Amotl1 mediates sequestration of the hippo effector Yap1 downstream of Fat4 to restrict heart growth. Nat Commun. (2017) 8(1):14582. doi: 10.1038/ncomms14582
71. Manshaei R, Merico D, Reuter MS, Engchuan W, Mojara BA, Chaturvedi R, et al. Genes and pathways implicated in tetralogy of fallot revealed by ultra-rare variant burden analysis in 231 genome sequences. Front Genet. (2020) 11:957. doi: 10.3389/fgene.2020.00957
72. Grunert M, Dorn C, Schueler M, Dunkel I, Schlesinger J, Mebus S, et al. Rare and private variations in neural crest, apoptosis and sarcomere genes define the polygenic background of isolated tetralogy of Fallot. Hum Mol Genet. (2014) 23(12):3115–28. doi: 10.1093/hmg/ddu021
73. Reuter MS, Jobling R, Chaturvedi RR, Manshaei R, Costain G, Heung T, et al. Haploinsufficiency of vascular endothelial growth factor related signaling genes is associated with tetralogy of Fallot. Genet Med. (2019) 21(4):1001–07. doi: 10.1038/s41436
74. Grunert M, Appelt S, Schönhals S, Mika K, Cui H, Cooper A, et al. Induced pluripotent stem cells of patients with Tetralogy of Fallot reveal transcriptional alterations in cardiomyocyte differentiation. Sci Rep. (2020) 10(1):10921. doi: 10.1038/s41598-020-67872-z
75. Chelu A, Williams SG, Keavney BD, Talavera D. Joint analysis of functionally related genes yields further candidates associated with tetralogy of fallot. J Hum Genet. (2022) 67(10):613–5. doi: 10.1038/s10038-022-01051-y
76. Zhang H, Lu L, Li C, Li H, Tian J, Wang H. Downregulated nuclear lncRNA NRON inhibits SHP2/wnt/β-catenin signaling and cardiomyocyte differentiation during the development of Tetralogy of Fallot. Genes Dis. (2023) 10:750–52. doi: 10.1016/j.gendis.2022.10.010
77. Homsy J, Zaidi S, Shen Y, Ware JS, Samocha K, Karczewski KJ, et al. De Novo Mutations in Congenital Heart Disease with Neurodevelopmental and Other Congenital Anomalies.; 2015. https://www.science.org
78. Arpi MNT, Simpson TI. SFARI Genes and where to find them; modelling autism Spectrum disorder specific gene expression dysregulation with RNA-Seq data. Sci Rep. (2022) 12(1):10158. doi: 10.1038/s41598-022-14077-1
79. Schaaf CP, Vetancur C, Yuen RKC, Parr JR, Skuse DH, Gallagher L, et al. A framework for an evidence-based gene list relevant to autism spectrum disorder. Nat Rev Genet. (2020) 21(6):367–76. doi: 10.1038/s41576-020-0231-2
80. Myers SM, Challman TD, Bernier R, Bourgeron T, Chung WK, Constantino JN, et al. Insufficient evidence for “autism-specific” genes. Am J Human Genet. (2020) 106(5):587–95. doi: 10.1016/j.ajhg.2020.04.004
81. Deepe RN, Drummond JR, Wolters RA, Fitzgerald EA, Tarolli HG, Harvey AB, et al. Sox9 expression in the second heart field; A morphological assessment of the importance to cardiac development with emphasis on atrioventricular septation. J Cardiovasc Dev Dis. (2022) 9(11):376. doi: 10.3390/jcdd9110376
82. Akiyama H, Chaboissier MC, Behringer RR, Rowitch DH, Schedl A, Epstein JA, et al. Essential role of Sox9 in the pathway that controls formation of cardiac valves and septa. Proc Natl Acad Sci USA. (2004) 101(17):6502–7. doi: 10.1073/pnas.0401711101
83. Zhang L, Nomura-Kitabayashi A, Sultana N, Cai W, Cai X, Moon A, et al. Mesodermal Nkx2.5 is necessary and sufficient for early second heart field development. Dev Biol. (2014) 390(1):68–79. doi: 10.1016/j.ydbio.2014.02.023
84. Hussein IR, Bader RS, Chaudhary AG, Bassiouni R, Alquaiti M, Ashgan F, et al. Identification of De Novo and rare inherited copy number variants in children with syndromic congenital heart defects. Pediatr Cardiol. (2018) 39(5):924–40. doi: 10.1007/s00246-018-1842-7
85. Zhu H, Ji H, Chen W, Han L, Yu L. Integrin subunit β-like 1 mediates angiotensin II-induced myocardial fibrosis by regulating the forkhead box Q1/snail axis. Arch Biochem Biophys. (2022) 730:109422. doi: 10.1016/j.abb.2022.109422
86. Wang P, Lv C, Zhang T, Liu J, Yang J, Guan F, et al. FOXQ1 Regulates senescence-associated inflammation via activation of SIRT1 expression. Cell Death Dis. (2017) 8(7):e2946–e2946. doi: 10.1038/cddis.2017.340
87. Brody SL, Yan XH, Wuerffel MK, Song SK, Shapiro SD. Ciliogenesis and left–right axis defects in forkhead factor HFH-4–null mice. Am J Respir Cell Mol Biol. (2000) 23(1):45–51. doi: 10.1165/ajrcmb.23.1.4070
88. Bachmaier K, Neu N, Pummerer C, Duncan GS, Mak TW, Matsuyama T, et al. iNOS expression and nitrotyrosine formation in the myocardium in response to inflammation is controlled by the interferon regulatory transcription factor 1. Circulation. (1997) 96(2):585–91. PMID: 9244230
89. Jiang DS, Li L, Huang L, Gong J, Xia H, Liu X, et al. Interferon regulatory factor 1 is required for cardiac remodeling in response to pressure overload. Hypertension. (2014) 64(1):77–86. doi: 10.1161/HYPERTENSIONAHA.114.03229
90. Yu Q, Vazquez R, Khojeini EV, Patel C, Venkataramani R, Larson DF. IL-18 induction of osteopontin mediates cardiac fibrosis and diastolic dysfunction in mice. Am J Physiol Heartry Physio. (2009) 297(1):H76–85. doi: 10.1152/ajpheart.01285.2008
91. Bajpai R, Chen DA, Rada-Iglesias A, Zhang J, Xiong Y, Helms J, et al. CHD7 Cooperates with PBAF to control multipotent neural crest formation. Nature. (2010) 463(7283):958–62. doi: 10.1038/nature08733
92. Schulz Y, Wehner P, Opitz L, Salinas-Riester G, Bongers EMHF, van Ravenswaaij-Arts CMA, et al. CHD7, The gene mutated in CHARGE syndrome, regulates genes involved in neural crest cell guidance. Hum Genet. (2014) 133(8):997–1009. doi: 10.1007/s00439-014-1444-2
93. Stathopoulou A, Wang P, Thellier C, Kelly RG, Zheng D, Scambler PJ. CHARGE syndrome-associated CHD7 acts at ISL1-regulated enhancers to modulate second heart field gene expression. Cardiovasc Res. (2023) 119(11):2089–2105. doi: 10.1093/cvr/cvad059
94. Schatton D, Pla-Martin D, Marx MC, Hansen H, Mourier A, Nemazanyy I, et al. CLUH Regulates mitochondrial metabolism by controlling translation and decay of target mRNAs. J Cell Biol. (2017) 216(3):675–93. doi: 10.1083/jcb.201607019
95. Cox SJ, Gunberg DL. Energy metabolism in isolated rat embryo hearts: effect of metabolic inhibitors. J Embryol Exp Morphol. (1972) 28(3):591–9. doi: 10.1242/dev.28.3.591
96. Mackler B, Grace R, Duncan HM. Studies of mitochondrial development during embryogenesis in the rat. Arch Biochem Biophys. (1971) 144(2):603–10. doi: 10.1016/0003-9861(71)90367-5
97. Porter GA, Hom JR, Hoffman DL, Quintanilla RA, de Mesy Bentley KL, Sheu SS. Bioenergetics, mitochondria, and cardiac myocyte differentiation. Prog Pediatr Cardiol. (2011) 31(2):75–81. doi: 10.1016/j.ppedcard.2011.02.002
98. Ul Fatima N, Ananthanarayanan V. Mitochondrial movers and shapers: recent insights into regulators of fission, fusion and transport. Curr Opin Cell Biol. (2023) 80:102150. doi: 10.1016/j.ceb.2022.102150
99. Correia M, Santos F, da Silva Ferreira R, Ferreira R, Bernardes de Jesus B. Nóbrega-Pereira S. Metabolic determinants in cardiomyocyte function and heart regenerative strategies. Metabolites. (2022) 12(6):500. doi: 10.3390/metabo12060500
100. Lampert MA, Orogo AM, Najor RH, Hammerling BC, Leon LJ, Wang BJ, et al. BNIP3l/NIX And FUNDC1-mediated mitophagy is required for mitochondrial network remodeling during cardiac progenitor cell differentiation. Autophagy. (2019) 15(7):1182–98. doi: 10.1080/15548627.2019.1580095
101. Pla-Martín D, Schatton D, Wiederstein JL, Marx MC, Khiati S, Krüger M, et al. CLUH Granules coordinate translation of mitochondrial proteins with mTORC1 signaling and mitophagy. EMBO J. (2020) 39(9):e102731. doi: 10.15252/embj.2019102731
102. Schwede M, Nagpal S, Gandal MJ, Parikshak NN, Mirnics K, Geschwind DH, et al. Strong correlation of downregulated genes related to synaptic transmission and mitochondria in post-mortem autism cerebral cortex. J Neurodev Disord. (2018) 10(1):18. doi: 10.1186/s11689-018-9237-x
103. Toubat O, De Zoysa P, Choi J, Harvey D, Sucov H, Subramanyan RK. Abstract 12540: neural crest cell-derived Wnt5a regulates planar cell polarity in cranial second heart field progenitor cells. Circulation. (2020) 142(Suppl_3):A12540. doi: 10.1161/circ.142.suppl_3.12540
104. Yelbuz TM, Waldo KL, Kumiski DH, Stadt HA, Wolfe RR, Leatherbury L, et al. Shortened outflow tract leads to altered cardiac looping after neural crest ablation. Circulation. (2002) 106(4):504–10. doi: 10.1161/01.CIR.0000023044.44974.8A
105. Dyer LA, Kirby ML. The role of secondary heart field in cardiac development. Dev Biol. (2009) 336(2):137–44. doi: 10.1016/j.ydbio.2009.10.009
106. Toubat O, Mallios DN, Munabi NCO, Magee WP, Starnes VA, Kumar SR. Clinical importance of concomitant cleft lip/palate in the surgical management of patients with congenital heart disease. World J Pediatr Congenit Heart Surg. (2021) 12(1):35–42. doi: 10.1177/2150135120954814
107. Munabi NCO, Mikhail S, Toubat O, Webb M, Auslander A, Sanchez-Lara PA, et al. High prevalence of deleterious mutations in concomitant nonsyndromic cleft and outflow tract heart defects. Am J Med Genet A. (2022) 188(7):2082–95. doi: 10.1002/ajmg.a.62748
108. Friedman P, Yilmaz A, Ugur Z, Jafar F, Whitten A, Ustun I, et al. Urine metabolomic biomarkers for prediction of isolated fetal congenital heart defect. J Matern Fetal Neonatal Med. (2022) 35(25):6380–7. doi: 10.1080/14767058.2021.1914572
109. Briot A, Bouloumié A, Iruela-Arispe ML. Notch, lipids, and endothelial cells. Curr Opin Lipidol. (2016) 27(5):513–20. doi: 10.1097/MOL.0000000000000337
110. Suckling RJ, Korona B, Whiteman P, Chillakuri C, Holt L, Handford PA, et al. Structural and functional dissection of the interplay between lipid and notch binding by human notch ligands. EMBO J. (2017) 36(15):2204–15. doi: 10.15252/embj.201796632
111. Azbazdar Y, Karabicici M, Erdal E, Ozhan G. Regulation of wnt signaling pathways at the plasma membrane and their misregulation in cancer. Front Cell Dev Biol. (2021) 9:631623. doi: 10.3389/fcell.2021.631623
112. Karabicici M, Azbazdar Y, Iscan E, Ozhan G. Misregulation of wnt signaling pathways at the plasma membrane in brain and metabolic diseases. Membranes (Basel). (2021) 11(11):844. doi: 10.3390/membranes11110844
113. Ozhan G. Editorial: wnt signaling at the plasma membrane: activation, regulation and disease connection. Front Cell Dev Biol. (2021) 9:780163. doi: 10.3389/fcell.2021.780163
114. Ross JA, Levy Y, Ripolone M, Kolb JS, Turmaine M, Holt M, et al. Impairments in contractility and cytoskeletal organisation cause nuclear defects in nemaline myopathy. Acta Neuropathol. (2019) 138(3):477–95. doi: 10.1007/s00401-019-02034-8
115. Jamieson L, Hedges J, Peres MA, Guarnizo-Herreño CC, Bastos JL. Challenges in identifying indigenous peoples in population oral health surveys: a commentary. BMC Oral Health. (2021) 21(1):216. doi: 10.1186/s12903-021-01455-w
116. Skinner A, Raifman J, Ferrara E, Raderman W, Quandelacy TM. Disparities made invisible: gaps in COVID-19 data for American Indian and Alaska native populations. Health Equity. (2022) 6(1):226–9. doi: 10.1089/heq.2021.0129
117. Oster ME, Strickland MJ, Mahle WT. Racial and ethnic disparities in post-operative mortality following congenital heart surgery. J Pediatr. (2011) 159(2):222–6. doi: 10.1016/j.jpeds.2011.01.060
118. Gilboa SM, Devine OJ, Kucik JE, Oster ME, Riehle-Colarusso T, Nembhard WN, et al. Congenital heart defects in the United States: estimating the magnitude of the affected population in 2010. Circulation. (2016) 134(2):101–9. doi: 10.1161/CIRCULATIONAHA.115.019307
119. Chang YL, Kuan TH, Chen CH, Tsai YJ, Chen GB, Lin KL, et al. Differences in cardiopulmonary fitness between boy and girls with repaired tetralogy of fallot. Front Pediatr. (2022) 10:911825. doi: 10.3389/fped.2022.911825
120. Parvar SY, Ghaderpanah R, Naghshzan A. Prevalence of congenital heart disease according to the echocardiography findings in 8145 neonates, multicenter study in southern Iran. Health Sci Rep. (2023) 6(4):e1178. doi: 10.1002/hsr2.1178
121. Baliento L, Dal Bianco L, Bagato F, Secco E, Sarubbi B, Mazzotti E, et al. Gender differences and role of pregnancy in the history of post-surgical women affected by tetralogy of fallot. PLoS One. (2012) 7(12):e49729. doi: 10.1371/journal.pone.0049729
122. Shi W, Sheng X, Dorr KM, Hutton JE, Emerson JI, Davies HA, et al. Cardiac proteomics reveals sex chromosome-dependent differences between males and females that arise prior to gonad formation. Dev Cell. (2021) 56(21):3019–34.e7. doi: 10.1016/j.devcel.2021.09.022
123. Salvoro C, Bortoluzzi S, Coppe A, Valle G, Feltrin E, Mostacciuolo ML, et al. Rare risk variants identification by identity-by-descent mapping and whole-exome sequencing implicates neuronal development pathways in schizophrenia and bipolar disorder. Mol Neurobiol. (2018) 55(9):7366–76. doi: 10.1007/s12035-018-0922-2
Keywords: tetralogy of fallot, exome sequencing, congenital heart defect, de novo variants, conotruncal defects
Citation: Harvey DC, Verma R, Sedaghat B, Hjelm BE, Morton SU, Seidman JG and Kumar SR (2023) Mutations in genes related to myocyte contraction and ventricular septum development in non-syndromic tetralogy of Fallot. Front. Cardiovasc. Med. 10:1249605. doi: 10.3389/fcvm.2023.1249605
Received: 28 June 2023; Accepted: 8 September 2023;
Published: 28 September 2023.
Edited by:
Joan Sanchez-de-Toledo, Sant Joan de Déu Hospital, SpainReviewed by:
Robert Kelly, UMR7288 Institut de Biologie du Développement de Marseille (IBDM), FranceMarlin Touma, University of California, United States
© 2023 Harvey, Verma, Sedaghat, Hjelm, Morton, Seidman and Kumar. This is an open-access article distributed under the terms of the Creative Commons Attribution License (CC BY). The use, distribution or reproduction in other forums is permitted, provided the original author(s) and the copyright owner(s) are credited and that the original publication in this journal is cited, in accordance with accepted academic practice. No use, distribution or reproduction is permitted which does not comply with these terms.
*Correspondence: S. Ram Kumar cnN1YnJhbWFueWFuQHVubWMuZWR1