- 1Department of Myocardial Function, Imperial College London, National Heart and Lung Institute, London, United Kingdom
- 2Division of Cardiovascular Surgery, University of Pennsylvania School of Medicine, Philadelphia, PA, United States
In recent years, development of mechanical circulatory support devices has proved to be a new treatment modality, in addition to standard pharmacological therapy, for patients with heart failure or acutely depressed cardiac function. These include left ventricular assist devices, which mechanically unload the heart when implanted. As a result, they profoundly affect the acute cardiac mechanics, which in turn, carry long-term consequences on myocardial function and structural function. Multiple studies have shown that, when implanted, mechanical circulatory assist devices lead to reverse remodelling, a process whereby the diseased myocardium reverts to a healthier-like state. Here, we start by first providing the reader with an overview of cardiac mechanics and important hemodynamic parameters. We then introduce left ventricular assist devices and describe their mode of operation as well as their impact on the hemodynamics. Changes in cardiac mechanics caused by device implantation are then extrapolated in time, and the long-term consequences on myocardial phenotype, as well as the physiological basis for these, is investigated.
Introduction
Chronic pathological hemodynamic stress following an index event (e.g., pressure-, or-volume-overload) alters myocardial molecular, cellular, and interstitial factors, manifesting clinically as changes in size, shape, and function of the heart. Each stressor can affect the operation and mechanics of the heart distinctly across time and with often different underlying mechanisms (1, 2). However, when left uncorrected prolonged hemodynamic overload ubiquitously leads to heart failure (HF), characterised by dilation of heart size and deterioration of contractile function. The development of mechanical support devices has provided a new way to both halt the vicious cycle of haemodynamic overload before progression to HF, but also to support the failing heart, allowing reversal of overload-induced structural and functional pathology, in a process termed reverse remodelling.
Mechanical support devices can provide univentricular or biventricular support, can be implanted percutaneously or surgically, provide varying amounts of cardiac output (CO) support and for different durations (3). An important distinction is that although all mechanical support devices provide some degree of circulatory support, not all circulatory support devices mechanically unload the myocardium, and some may even overload it. For example, veno-arterial extracorporeal membrane oxygenation (VA-ECMO) is becoming an established modality to provide circulatory support in the setting of cardiogenic shock, yet it retrogradely perfuses the aorta severely increasing left ventricular (LV) afterload and overloading the myocardium (4, 5).
In this review we focus exclusively on LV assist devices (LVADs)—that is, those that mechanically unload the LV. These include both durable LVADs (d-LVADs), such as the HeartMate 3, which are surgically implanted and can be used for prolonged periods of time, as well as transvalvular micro-axial flow pump devices (tma-LVAD), such as the Impella, which can be percutaneously implanted and are typically used for acute mechanical support and for shorter durations (6). We begin by examining the mechanics of healthy myocardium, assessed in the pressure-volume (PV) plane, and the expected changes to these with progression to HF. Subsequently, we investigate how LVADs alter cardiac mechanics, immediately upon implantation but also in the long-term and how changes in mechanical load may lead to reverse remodelling, as well as the structural and functional changes and implicated underlying mechanisms of this.
Pressure-volume relationships
To understand the effects of mechanical support devices on cardiac mechanics an understanding of pressure-volume (PV) loops is required. A brief description of the main determinants of cardiac mechanics is shown here—for a more detailed review see (7).
Cardiac mechanical load is characterized by afterload and preload, both of which can alter stroke volume (SV) via the Frank-Starling relationship. Preload refers to the mechanical load the heart is exposed to prior to the beginning of systole—it is appreciated by the end-diastolic pressure or volume point in the PV loop (Figure 1). Afterload refers to the mechanical load against which the ventricle contracts and can generally be appreciated by the arterial elastance (Ea) shown as a line that connects the end-diastolic (EDV) and end-systolic (ESV) volume points (Figure 1). The Frank-Starling mechanism relates preload to SV, whereby increased preload results in increased SV.
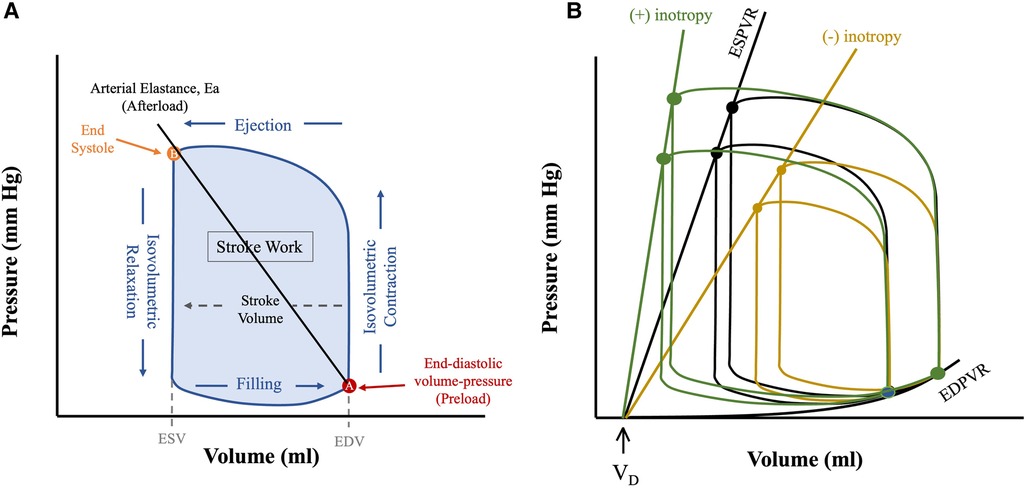
Figure 1. Anatomy of a PV loop. (A) Normal PV loop representing the 4 phases of the cardiac cycle, as the heart transitions from diastolic (A) to systolic (B) state and back. (B) Pressure-volume loops at different preloads and contractile states (Ees). All PV loops are bounded by their corresponding linear ESPVR (drawn from the instantaneous end-systolic pressure-volume points) and the EDPVR (drawn from the corresponding end-diastolic pressure-volume points). The slope of ESPVR represents end-systolic elastance (Ees), a measure of intrinsic contractility, and the x-axis intercept is the dead volume (Vd), below which ventricular pressure generation does not occur. Figures are adapted from: (8). ESV, end systolic volume; EDV, end diastolic volume.
The PV loop is demarcated by the 4 phases of the cardiac cycle (Figure 1): (1) isovolumetric contraction, (2) ejection, (3) isovolumetric relaxation and (4) filling. The width denotes SV, and the area inside the PV loop represents stroke work, which correlates to myocardial oxygen consumption (MVO2) (Figure 1) (9) (see below). Cardiac function is dependent upon preload and afterload and so within the in vivo state where these are constantly varying it is challenging to assess unless they are well controlled for (10) developed a mathematical function, termed time-varying elastance, E(t), that has enabled determination of cardiac function irrespective of mechanical load. E(t) is a load-independent measure of contractile state and is defined using Equation (1) as shown below:
Where E(t) is the instantaneous elastance, P(t) and V(t) instantaneous pressure and volume respectively and VD is dead volume—that is, the volume below which no pressure generation occurs in the heart (11). The maximum elastance, or end-systolic elastance (Ees), can be approximated by the instantaneous end-systolic pressure-volume relationship (ESPVR), which is a load-independent measure of intrinsic contractility (Figure 1B). Increased contractility, known as positive inotropy, shifts the ESPVR leftward and up, with a steeper slope (Ees), while decreased contractility, known as decreased inotropy, has opposite effect (12). A shallow ESPVR is seen in patients with a well-compensated HF with reduced ejection fraction (HFrEF) due to contractile dysfunction (Figure 2). Importantly, the shallower the ESPVR the more afterload-sensitive the heart, such that afterload-reducing interventions can have significant impact on cardiac hemodynamics and symptoms (14). The diastolic properties of the ventricle are defined by the non-linear end-diastolic pressure-volume relationship (EDPVR), which represents passive myocardial stiffness (or ventricular compliance) (15). Pathological ventricular dilatation results in a rightward shift of the EDPVR towards higher volumes, and reverse remodelling following mechanical unloading has been shown to shift it back towards near normal levels (16). Both ESPVR and EDPVR can be used to evaluate myocardial tissue properties in progression from healthy to failing myocardium and vice versa, irrespective of loading conditions (11, 14).
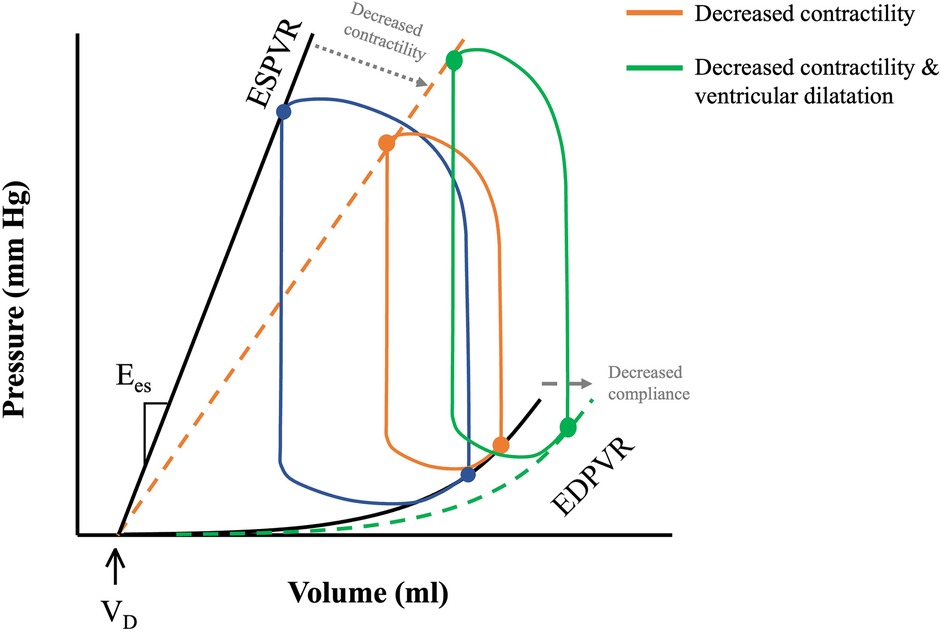
Figure 2. PV loops in health and disease. ESPVR and EDVPR shifts with decreasing contractility and decreased compliance (depicted by the grey dotted arrows) during pathological remodelling of the heart. Decreased cardiac contractility in HFrEF shifts ESPVR rightwards with a shallower slope (orange dotted line). Progressive ventricular remodelling leads to a rightward shift of the EDPVR (green dotted line), and further downward shifting of the ESPVR, causing LV dilatation and decreased LV contractility. Adapted from: (13).
Mechanical circulatory support devices
Mechanical support devices are often used clinically in the management of patients with end-stage HF and acute hemodynamic compromise. Although there are many different types of devices and indications for use, in this paper we discuss d-LVADs and trans-axial tma-LVADs. The mode of operation of these is generally the same, namely, to unload the heart by extracting blood from the LV and ejecting it into the aorta, however, they differ in terms of implantation method, indications for use, and duration of treatment.
Durable left ventricular assist devices
d-LVADs refer to fully implantable devices that provide circulatory support by enhancing systemic blood pressure and CO, while mechanically unloading the LV (Figure 3). The basic principle of LVADs consists of an inflow cannula in the LV apex and an outflow graft in the aorta. This design has remained unchanged despite multiple newer iterations in the operation of the device and implantation method (20).
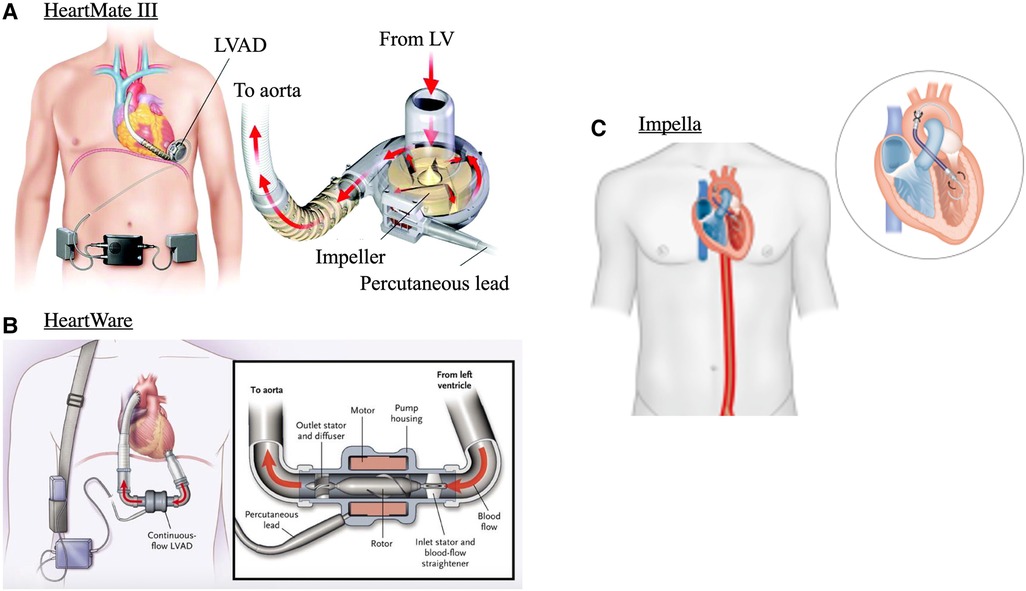
Figure 3. Mechanical circulatory support devices. (A) centrifugal continuous flow durable LVAD (HeartMate 3), (B) continuous axial-flow durable LVAD (HeartWare) and (C) Transvalvular micro-axial flow device (Impella). Images adapted from: (17–19).
First generation pulsatile-flow devices have been replaced by smaller, quieter, and more durable continuous-flow LVADs. These can be either centrifugal-flow (HeartMate 3, Figure 3A) or axial-flow pumps (HeartMate 2, and HeartWare, Figure 3B) (20). Continuous-flow LVADs have generally demonstrated improved end-organ function, and quality of life over pulsatile-flow devices (18, 21). This is interesting given the unphysiological nature of continuous flow and is likely related to decreased risk for thrombosis and embolic episodes due to absence of mechanical bearings in continuous flow LVADs (22). Between continuous-flow devices, centrifugal pumps carry superior outcomes to axial-flow pumps as evidenced by the 5-year outcomes of the MOMENTUM 3 randomised clinical trial. This study compared HeartMate 3 with HeartWare and demonstrated greater composite outcomes of survival to transplant, recovery, and LVAD support free from reoperation or stroke (23). d-LVADs are currently used under three main categories: bridge-to-transplantation, bridge-to-recovery, or as destination therapy (20, 24).
Bridge-to-recovery refers to sufficient myocardial recovery such that device removal is possible; this has been particularly useful in view of the limited number of donor hearts. Evidence that mechanical unloading can lead to sufficient recovery such as to enable LVAD explantation were demonstrated early on by (25). In patients with idiopathic dilated cardiomyopathy, associated with high levels of anti-β1-adrenoceptor autoantibodies (a-β1-AABs), mechanical support with LVAD led to substantial improvement in cardiac structure, function, as well as decreased a-β1-AABs titre levels such that device could be explanted (25). These early studies pioneered the protocol to evaluate for cardiac recovery, as well as developed methods to stratify patients likely to benefit from mechanical support weaning (25). For example, patients with LV internal diastolic diameter and myocardial fibrosis towards the high end of the distribution were less likely to show functional improvement (25). Likewise, to determine suitability of patients for weaning off mechanical support, repeated “off-pump” trials were used. During these, in most patients, LV ejection fraction greater than 45% and LV internal diastolic diameter less than 55 mm were considered cut-offs that signified sufficient cardiac recovery for weaning had occurred (26, 27).
Registry studies have shown that 1%–2% of patients undergo LV recovery to a degree allowing explantation (28–30). However, this low rate of recovery has been questioned as patients who are placed on mechanical support are typically those not-responding to medical therapy; their medication regimen following d-LVAD implantation is thus often poorly optimised, leading to inferior recovery rates. In fact, aggressive medical optimisation as shown in the RESTAGE-HF trial coupled with uniform LVAD protocol resulted in 40% recovery rates (31). Importantly, the findings of this trial were centre-independent suggesting an intrinsic advantage to optimised medical therapy when combined with LVAD for recovery. Recovery rates are important, as survival post-recovery is comparable to that of heart transplants (32).
Aside from paucity of donor hearts, eligibility for cardiac transplantation remains a prominent barrier to treatment of advanced HF. LVADs as destination therapy refers to permanent device support as the last resort for patients ineligible for transplantation, to prolong life while providing better quality of life. Since its FDA approval in 2010, better event-free survival, symptoms and quality of life have been demonstrated with destination therapy over optimal medical management for patients that fit specific hemodynamic profiles (33–35). Destination therapy also allows healthcare centres without transplant resources to provide treatment for this patient cohort. In fact, Brinkley et al. concluded similar outcomes following destination therapy LVAD implantation between transplant and non-transplant centres, at 1 and 12-months (36). However, it is important to note that such program success requires sufficient infrastructure for multidisciplinary team support to assist in the long-term care of these patients.
Transvalvular micro-axial flow pump LVADs
Transvalvular micro-axial flow pumps are mechanical and circulatory support devices that when implanted sit between the LV and aortic root (Figure 3C). There are different types of tma-LVADs, most notably the Impella devices by Abiomed. These are implanted percutaneously or with surgical cut-down, and like d-LVADs continuously push blood from the LV, by means of a rotating helical screw, into the aorta (37). There are many different types of tma-LVADs, the indications and capabilities of each were recently described in detail in (37). Generally, the effects on hemodynamics are the same but depending on device, CO can be supplemented from 2.5 L/min up to 6 L/min (38, 39). As some tma-LVADs can be implanted percutaneously, deployment is faster than d-LVADs, making these devices utilisable for mechanical support in the acute setting. This allows CO and tissue perfusion to be maintained in states of hemodynamic compromise, such as cardiogenic shock. Whether tma-LVADs provide mortality benefit to these patients compared to other percutaneously implanted devices, such as the intra-aortic balloon, is still an area of active research. Though the Impella is more powerful, and thus affords greater degrees of circulatory support, data shows that it carries higher risk of complications, likely related to bigger catheter size, or need for surgical cut-down (40). As such, only patient populations that require heavy circulatory support, that cannot be managed with pharmacological support alone or other circulatory support devices (e.g., intra-aortic balloon) may be warranted to receive prognostic benefit (41).
In the following sections we look at the effects of d-LVADs and tma-LVADs on acute hemodynamic profiles, as well as the long-term consequences of these on cardiac function and structure.
Hemodynamics under LVAD mechanical support
During LVAD mechanical support, blood is continually (and non-physiologically) pumped from the LV to the aorta irrespective of cardiac cycle phase. The continuous antegrade flow leads to a loss of the isovolumic contraction phase of the cardiac cycle, resulting in a “triangular” shaped PV loop that shifts down and to the left (Figure 4A) (42).
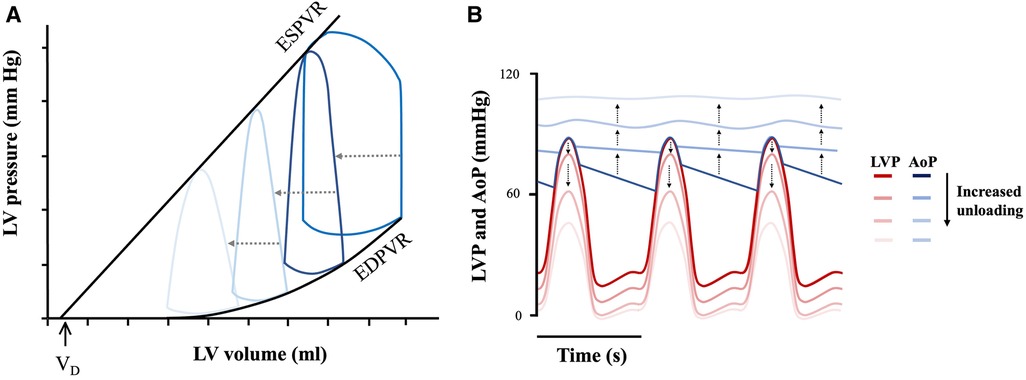
Figure 4. Mechanical circulatory support unloading and effect on cardiac mechanics. (A) Triangular PV loop with progressive leftwards and downwards shift under mechanical support and (B) LV-aortic pressure uncoupling. LV pressure (LVP—represented by the red theme), and aortic pressure (AoP—represented by the blue theme) progressively uncouple with increased device flow rates. Adapted from: (12, 43).
The degree to which the loop will shift to the left, and thus the LV will be unloaded, depends on the extent of support provided by the device (i.e., its flow-rate), which is adjustable (12). Increasing flow-rate increases the amount of blood flowing through the device into the arterial circulation, consequently supplementing CO to a greater degree. This causes a dissociation between LV pressure and aortic pressure as shown in Figure 4B (12). This flow-dependent dissociation is termed LV-aortic pressure uncoupling and it correlates quantitatively to mechanical unloading (44). Such quantifications are in turn important, as degree of mechanical support linearly correlate with myocardial remodelling (45). For example, immediately upon device implantation, flow-dependent decreases in LV end-diastolic pressure (LVEDP) are seen (44). When applied over prolonged durations, this results in a progressive leftward shift in the EDPVR, though not reaching levels seen in normal healthy hearts, as well as with decreased compliance (seen by the increased EDPVR slope) (Figure 5) (43). Thus, although not fully normalized, a lower EDPVR correlates to improved myocardial stiffness, and higher sensitivity to mechanical load (2, 46). Compared to unsupported hearts, LVADs can therefore, to a degree, reverse the negative hemodynamic effects of diastolic dysfunction (43, 46).
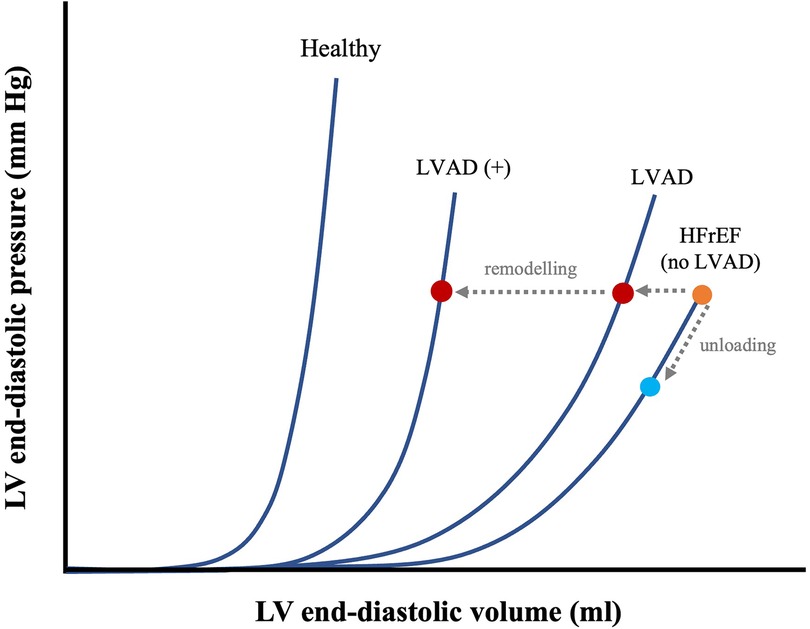
Figure 5. Effects of prolonged mechanical unloading on diastolic function. EDPVR of a healthy vs. HF with reduced ejection fraction (HFrEF) heart and time-dependent LVAD–associated leftward shifts in EDPVR, with shorter (LVAD) and longer duration (LVAD+) of unloading. The effects of immediate mechanical unloading on the point of operation of the heart on the EDPVR are also shown (HFrEF diagonal arrow from orange dot to blue dot). Adapted from: (43).
Myocardial oxygen demand, MVO2, is determined by the area under the PV loop curve and corresponds to stroke work plus potential energy (Figure 6). MVO2 can also be appreciated in Wiggers diagrams and has been termed systolic left ventricular pressure curve index (SPTI) (47, 48) (Figure 7) (9). demonstrated a close relationship between the area under the curve of a PV loop and MVO2: that is—the greater the area under the curve in a PV loop or the higher the SPTI, the higher the MVO2. Accordingly, interventions that decrease these also decrease MVO2.
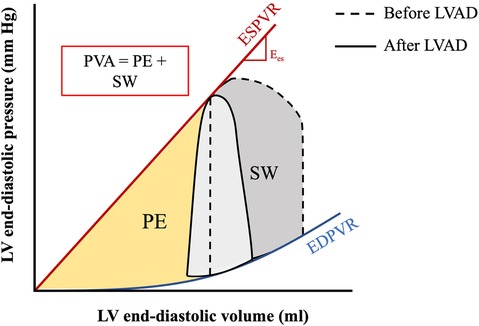
Figure 6. PV area corresponding to myocardial oxygen consumption. The pressure-volume area (PVA) is the sum of the stroke work (SW) and potential energy (PE) and represents the total mechanical work of the ventricle per beat. The PVA is directly correlated to the myocardial oxygen consumption (MVO2). Figure shows PVA before and after implantation of LVAD. Adapted from: (44).
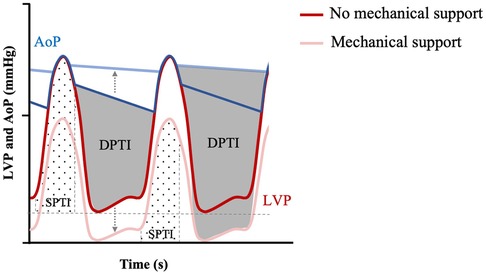
Figure 7. Myocardial O2 demand (SPTI) and supply (DPTI) with or without mechanical unloading. Modified Wigger's diagram showing progressive mechanical unloading (represented by grey dotted arrow) and uncoupling of LV pressure (LVP—red themed loops) and aortic pressure (AoP—blue themed loops), correlated with increased DPTI (grey overlay) and decreased SPTI (hashed overlay. Adapted from: (47).
Under mechanical support, part of the CO is provided by the assist device. As a result, preload, and consequently (a) diastolic stress on the heart, and (b) stroke volume (given Frank-Starling) decrease. In hemodynamically unstable patients this is critical, as decreased myocardial stress correlates to decreased MVO2 in accordance with Laplace's law of the heart, protecting the heart from ischemic injury (49). Decreased MVO2 due to mechanical unloading also has profound long-term consequences. For example, implantation of Impella within 24 h in patients with anterior ST-segment myocardial infarction is associated with an increased left ventricular ejection fraction after ∼4 months from index event compared to control (50). The Protect II randomised controlled trial, showed that Impella provided greater CO power, a metric correlated to mortality (51), compared to other mechanical support devices in cardiogenic shock patients (52). Of note is that the prognostic significance of CO power may be limited to acutely decompensated patients following myocardial infarction only and not apply to patients with acute HF cardiogenic shock (53, 54). Other trials with lower-sample sizes (IMPRESS) did not corroborate these findings (55). Part of these beneficial effects may be explained by decreased infarct size in patients who received acute mechanical unloading, as has been shown in separate studies conducted in dogs and sheep that were subjected to myocardial infarcts by left anterior descending coronary artery ligation followed by mechanical unloading (45, 56).
In addition to lowering oxygen demand, LVAD devices can also increase myocardial oxygen supply by increasing coronary perfusion pressures. Coronary blood flow occurs in diastole and is a function of the pressure gradient between aortic pressure, PAo, and left ventricular diastolic pressure, PLV, according to the Equation (2):
This is known as the diastolic pressure-time index (DPTI) and is shown in Figure 7 together with SPTI (47). The DPTI:SPTI ratio determines the myocardial oxygen supply-demand equation (47) and so an increase in the ratio is associated with improved energy supply. For example, as LVADs displace blood from the LV to the aorta, it increases aortic blood pressure and decreases LV diastolic pressure, resulting in increased diastolic flow according to DPTI and Figure 6 (38, 57). Given that it also lowers the SPTI, due to lower LV pressure (decreased preload), the DPTI:SPTI ratio increases and myocardial oxygen supply- demand mismatch decreases. It is important to note that while the theoretical framework for an improved supply-demand with LVAD may hold true, coronary blood flow also depends on an appropriately opening aortic valve, which can be impaired depending on the orientation of tma-LVADs, while it could also lead to complications such as coronary artery thrombosis (58). Furthermore, studies have shown that as a result of non-pulsatile blood flow, coronary arteries remodel under LVAD support, with increased collagen deposition and breakdown of the internal elastic lamina (59), which can further decrease perfusion to the heart.
Hemodynamic complications
Despite known improvements in CO and left diastolic pressures after LVAD implantation (60), reported a significant increase in right atrial pressure (RAP) and a significant reduction in pulmonary artery pulsatility index (PAPi) [(systolic pulmonary artery pressure—diastolic pulmonary artery pressure)/central venous pressure] overtime with LVAD implantation. Increased RAP and decreased PAPi indicate progressive worsening of right ventricular (RV) function; a dominant cause of vulnerability in LVAD patients that is linked to poor clinical outcomes (60). PAPi can independently predict patients who will require right ventricular assist device (RVAD) support after LVAD implantation (61, 62). As aforementioned, LVADs shift the LVEDP relationship to the left, causing the pulmonary capillary wedge pressure (PCWP) to decrease, thereby reducing RV afterload (12). However, at higher pump speeds, the increase in RV preload (caused by increased CO) predominates over the reduced RV afterload, and likely contributes to RV dysfunction. Mechanistically, an interventricular septal shift towards the LV occurs, likely causing decreased RV contractility due to abnormal geometry (63). In addition to impaired RV force generation, pre-existing pre-existing disease or transient RV tissue trauma during insertion LVAD may also contribute to development of RV dysfunction (61, 62).
Aortic insufficiency (AI) is another common complication of LVAD, affecting up to 20% of patients within 1 year of implantation (64), and can negatively affect survival (65). To maintain a high net forward blood flow in the presence of AI, LVAD pump speed is often increased in clinical practice (66). However, increasing LVAD speeds also increases regurgitant blood flow, which in turn can contribute to aberrant pulmonary vasculature remodelling and increased RV afterload (66). If the RV intrinsic contractility (Ees) is unable to match the pulmonary vasculature afterload (Ea), RV dysfunction occurs. As aforementioned, the increased preload with increased pump speeds can further contribute to this. Alternative treatment strategies, such as blood pressure control with or without increased pump speed and stratified by patient specific hemodynamic parameters, such as decreased coupling ratio (RV Ees/Ea) (66) or increased pre-operative right-to-left end-diastolic diameter, have been proposed (67).
Reverse remodelling following LVAD insertion
Mechanical unloading is associated with recovery of cardiac function, in a process known as reverse remodelling (68, 69). We discuss the structural, functional, cellular, and molecular changes that may be associated with this.
Structural changes
Long-term LVAD use leads to reduced LV and left atrial (LA) volumes (70, 71). Ventricular geometry is designed for minimal energy expenditure and maximal economy of circulation. Tubular ventricles allow maximal conversion of systolic muscle contraction into required pressure, whereas a remodelled, failing heart adopts a spherical configuration (72). Interestingly, LVAD-mediated changes of the LV's 3D geometry occur immediately upon unloading and are flow-type dependent. For example, intra-abdominal axial-flow LVADs, such with the Heartmate 2, lead to a significantly decreased LV volume and more conical (less spherical) cavity (Figure 8A) (63). Conversely, centrifugal-flow LVADs, such as seen with HeartWare, reduce LV volumes, albeit less, and do not have significant changes in sphericity or conicity (Figure 8B) (63). Device location may explain this distortion, as the inflow LV apex cannula is located sub-diaphragmatically in the intra-abdominal device and thus pulls the apex inferiorly as shown in Figure 3C (63). Interestingly, regardless of the apparent differences in shape, the hemodynamic modifications are similar with both devices, suggesting equivalent degrees of unloading (73). These immediate effects on heart structure may be of clinical relevance when selecting optimal device for each patient (73).
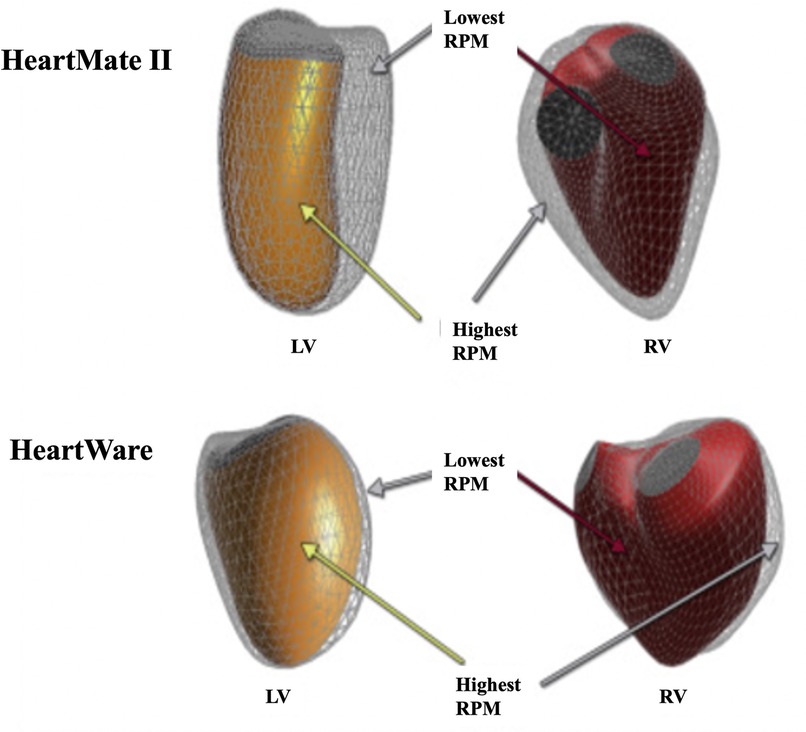
Figure 8. Flow and device specific structural remodelling. 3D illustration of RV (red) and LV (orange) endocardial surfaces geometry obtained following implantation of HeartMate 2 or HeartWare LVADs. Figure adapted from (63).
Recently, such changes in ventricular structure have been shown to be maintained with more prolonged device implantation (74). In patients implanted with either HeartMate 2, 3, or HeartWare, and followed before, at 1-month, and 2-months post- implantation the LV shows evidence of reverse structural remodelling as measured by decreased LV end-diastolic volume, decreased LV sphericity and increased conicity (74). However, these findings are not mirrored in the RV, which instead shows increased longitudinal strain suggesting worsening RV function, in line with this being a common complication of LVAD implantation (74).
Cellular and molecular changes
Reverse remodelling from mechanical unloading can be studied at the cellular and molecular level (Figure 9). Cardiomyocyte hypertrophy occurs as a compensatory mechanism to persistent volume- or pressure-overload and is associated with decompensation of cardiac function and progression into heart failure (75). Reduced cardiomyocyte hypertrophy occurs in response to mechanical unloading (43, 76–78), with some studies on pulsatile devices reporting reduction to the point of cardiomyocyte atrophy and degeneration (76, 79, 80), though others have challenged this (81). Mechanical unloading is also associated with decreased myocyte damage (71, 82), and normalisation of the expression of cytoskeletal proteins including vinculin, desmin, ß-tubulin as well as sarcomeric proteins (Figure 9) (83–86). At the intracellular level, prolonged mechanical support normalises expression of genes involved in calcium handling, such as SERCA2a (87), resulting in increased sarcoplasmic reticulum Ca2+ content (Figure 9). Increased calcium cycling is associated with recovery of contractile function (13), yet these features are only apparent in the LV.
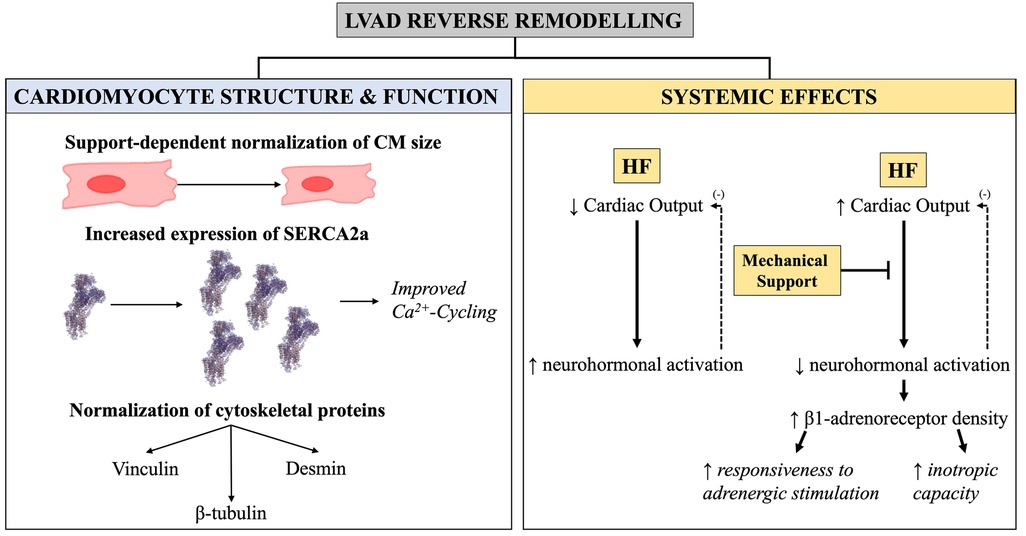
Figure 9. Graphical illustration of the cellular and molecular changes seen with LVAD-induced reverse remodelling. Cellular and molecular changes in LVAD-induced reverse remodelling. (Left) Changes in cardiomyocyte size, expression of Ca2+-handling proteins, and cytoskeletal components as a function of mechanical unloading. (Right) Supplementation of cardiac output from mechanical device halts the negative feedback loop [demarcated with (−) here] leading to decreased neurohormonal activation and improved biochemical milieu—these are systemic effects and impact both the RV and LV.
Of importance is that LVAD-induced reverse remodelling is thought to consist of two arms: those due to the mechanical unloading itself, and those caused by the circulating factors that normalise as a result of supplementation of CO (78, 88). Although the mechanics of the RV are affected by LVAD, with changes in both preload and afterload (89), mechanical unloading impact the LV more than the RV and so are likely to be reflected in the LV more. Conversely, when reversal of phenotype is seen in both RV and LV, then this is likely to be driven by normalisation of the adrenergic axis neurohormonal milieu underlined by supplementation of CO from the device (78). For example, both ventricles exhibit recovery of β-adrenergic responsiveness and receptor density with LVAD support (87, 88, 90, 91). Functionally, this restores myocardial response to ionotropic stimulation by the sympathetic nervous system during exercise, which is the most important regulatory mechanism of cardiovascular performance (Figure 9). Similarly, reversal of ryanodine receptor 2 (RyR2) hyperphosphorylation has been found in both ventricles (88) following LVAD support.
The importance of a correction of the adrenergic system cannot be understated, as the ß-adrenergic system has been repeatedly linked to HF pathogenesis. Down regulation of β1-receptor subtype leads to reduced exercise tolerance and LV inotropic reserve (92), and high levels of sympathetic activity are inversely correlated with prognosis in patients with HF (93).
Extracellular matrix (ECM) composition, structure and its cellular interactions are vital in adaptation to hemodynamic and neurohormonal stressors (94). LVAD-induced changes to collagen, the main structural support molecule of the ECM, is an area of debate. Several studies report reduced myocardial collagen (76, 95, 96), however the majority report no changes (76, 97, 98), or a significant increase (71, 88, 99–101). Increased cross-linked collagen following LVAD support is associated with increased myocardial stiffness (88), so mechanical unloading may lead to decreased compliance. However, such data would not be aligned with the leftward shift in EDPVR seen with LVAD support.
Combination therapy
Numerous clinical studies demonstrate that neurohormonal blockers reverse pathological cardiac remodelling by restoring normal heart geometry, reducing LV volume and mass, and improving LVEF (102). During LVAD support, concomitant administration of pharmacotherapy (ACE-inhibitors, β-blockers, mineralocorticoids antagonists) enhances the degree of reverse remodelling (103). As aforementioned (31), demonstrated that aggressive pharmacological therapy coupled with mechanical unloading can reverse severe HF in a large proportion of patients with non-ischemic cardiomyopathy. Beside the effects caused by direct mechanical unloading, pump support allows the very high doses required for medication-induced reverse remodelling, whilst maintaining CO and renal perfusion that would otherwise be severely impaired. Schnettler et al. (104) have shown that conventional pharmacological agents (e.g., β-blockers, ACE-inhibitors, mineralocorticoid receptor antagonists) are safe to use in patients with HF on LVAD, yet survival benefit is not different after 1-year compared to those without pharmacological support. Despite clear added effects on reverse remodelling when taken together, optimal combination therapy is yet to be elucidated.
Conclusion
An understanding of the effects on mechanical support devices on hemodynamics is essential to appreciate their mechanism of action and therapeutic potential. In this review, we have discussed the use of LVADs as mechanical support devices. We have covered their immediate hemodynamic consequences, the long-term effects of these on the mechanical and neurohormonal axis, as well as the key mechanisms of reverse remodelling as well as the phenotypic alterations.
Author contributions
BP-L: wrote, edited, and reviewed the manuscript. FP: wrote, edited, and reviewed the manuscript. MEI edited and reviewed the manuscript. All authors contributed to the article and approved the submitted version.
Conflict of interest
The authors declare that the research was conducted in the absence of any commercial or financial relationships that could be construed as a potential conflict of interest.
Publisher's note
All claims expressed in this article are solely those of the authors and do not necessarily represent those of their affiliated organizations, or those of the publisher, the editors and the reviewers. Any product that may be evaluated in this article, or claim that may be made by its manufacturer, is not guaranteed or endorsed by the publisher.
References
1. Calderone A, Takahashi N, Izzo NJ, Thaik CM, Colucci WS. Pressure- and volume-induced left ventricular hypertrophies are associated with distinct myocyte phenotypes and differential induction of peptide growth factor mRNAs. Circulation. (1995) 92(9):2385–90. doi: 10.1161/01.CIR.92.9.2385
2. Pitoulis FG, Terracciano CM. Heart plasticity in response to pressure- and volume-overload: a review of findings in compensated and decompensated phenotypes. Front Physiol. (2020) 11:92–108. doi: 10.3389/fphys.2020.00092
3. Salter BS, Gross CR, Weiner MM, Dukkipati SR, Serrao GW, Moss N, et al. Temporary mechanical circulatory support devices: practical considerations for all stakeholders. Nat Rev Cardiol. (2022) 20:263–77. doi: 10.1038/s41569-022-00796-5
4. Donker DW, Brodie D, Henriques JPS, Broomé M. Left ventricular unloading during veno-arterial ECMO: a simulation study. ASAIO J. (2019) 65:11–20. doi: 10.1097/MAT.0000000000000755
5. Schrage B, Burkhoff D, Rübsamen N, Becher PM, Schwarzl M, Bernhardt A, et al. Unloading of the left ventricle during venoarterial extracorporeal membrane oxygenation therapy in cardiogenic shock. JACC Heart Fail. (2018) 6:1035–43. doi: 10.1016/j.jchf.2018.09.009
6. Stulak JM, Lim JY, Maltais S. Ventricular assist device selection: which one and when? Croat Med J. (2014) 55:596–9. doi: 10.3325/cmj.2014.55.596
7. Pitoulis FG, de Tombe PP. Cardiac Contractility BT. In: Terracciano C, Guymer S, editors. Heart of the Matter: key concepts in cardiovascular science. Cham: Springer International Publishing (2019). p. 121–33. doi: 10.1007/978-3-030-24219-0_10
8. Burkhoff D, Mirsky I, Suga H. Assessment of systolic and diastolic ventricular properties via pressure-volume analysis: a guide for clinical, translational, and basic researchers. Am J Physiol Heart Circ Physiol. (2005) 289(2):501–12. doi: 10.1152/AJPHEART.00138.2005/ASSET/IMAGES/LARGE/ZH40080540720009.JPEG
9. Suga H, Hayashi T, Shirahata M. Ventricular systolic pressure-volume area as predictor of cardiac oxygen consumption. Am J Physiol. (1981) 240(1):H39–44. doi: 10.1152/ajpheart.1981.240.1.H39
10. Suga H, Sagawa K, Shoukas AA. Load independence of the instantaneous pressure-volume ratio of the canine left ventricle and effects of epinephrine and heart rate on the ratio. Circ Res. (1973) 32:314–22. doi: 10.1161/01.RES.32.3.314
11. Suga H, Sagawa K. Instantaneous pressure volume relationships and their ratio in the excised, supported canine left ventricle. Circ Res. (1974) 35(1):117–26. doi: 10.1161/01.RES.35.1.117
12. Burkhoff D, Sayer G, Doshi D, Uriel N. Hemodynamics of mechanical circulatory support. J Am Coll Cardiol. (2015) 66(23):2663–74. doi: 10.1016/J.JACC.2015.10.017
13. Uriel N, Sayer G, Annamalai S, Kapur NK, Burkhoff D. Mechanical unloading in heart failure. J Am Coll Cardiol. (2018) 72(5):569–80. doi: 10.1016/J.JACC.2018.05.038
14. Hsu S, Fang JC, Borlaug BA. Hemodynamics for the heart failure clinician: a state-of-the-art review. J Card Fail. (2022) 28(1):133–48. doi: 10.1016/J.CARDFAIL.2021.07.012
15. Pfeffer JM, Pfeffer MA, Fletcher PJ, Braunwald E. Progressive ventricular remodeling in rat with myocardial infarction. Am J Physiol. (1991) 260(5 Pt 2). doi: 10.1152/AJPHEART.1991.260.5.H1406
16. Burkhoff D, Klotz S, Mancini DM. LVAD-induced reverse remodeling: basic and clinical implications for myocardial recovery. J Card Fail. (2006) 12(3):227–39. doi: 10.1016/J.CARDFAIL.2005.10.012
17. Aaronson KD, Slaughter MS, Miller LW, McGee EC, Cotts WG, Acker MA =, et al. Use of an intrapericardial, continuous-flow, centrifugal pump in patients awaiting heart transplantation. Circulation. (2012) 125(25):3191–200. doi: 10.1161/CIRCULATIONAHA.111.058412/-/DC1
18. Slaughter MS, Rogers JG, Milano CA, Russell SD, Conte JV, Feldman D, et al. Advanced heart failure treated with continuous-flow left ventricular assist device. N Engl J Med. (2009) 361(23):2241–51. doi: 10.1056/NEJMOA0909938/SUPPL_FILE/NEJMOA0909938_DISCLOSURES.PDF
19. Wong ASK, Sin SWC. Short-term mechanical circulatory support (intra-aortic balloon pump, impella, extracorporeal membrane oxygenation, TandemHeart): a review. Ann Transl Med. (2020) 8(13):829–829. doi: 10.21037/atm-20-2171
20. Vaidya Y, Riaz S, Dhamoon AS. Left ventricular assist devices. Syracuse, NY: StatPearls (2022). https://www.ncbi.nlm.nih.gov/books/NBK499841/
21. Pagani FD, Miller LW, Russell SD, Aaronson KD, John R, Boyle AJ, et al. Extended mechanical circulatory support with a continuous-flow rotary left ventricular assist device. J Am Coll Cardiol. (2009) 54(4):312–21. doi: 10.1016/J.JACC.2009.03.055
22. Yazdchi F, Rajab TK, Rinewalt D, Loberman D, Shekar P, Percy E, et al. Comparison of heart transplant outcomes between recipients with pulsatile- vs continuous-flow LVAD. J Card Surg. (2019) 34:1062–8. doi: 10.1111/jocs.14210
23. Mehra MR, Naka Y, Uriel N, Goldstein DJ, Cleveland JC, Colombo PC, et al. A fully magnetically levitated circulatory pump for advanced heart failure. N Engl J Med. (2017) 376(5):440–50. doi: 10.1056/NEJMOA1610426
24. Yuzefpolskaya M, Schroeder SE, Houston BA, Robinson MR, Gosev I, Reyentovich A, et al. The society of thoracic surgeons intermacs 2022 annual report: focus on the 2018 heart transplant allocation system. Ann Thorac Surg. (2023) 115(2):311–27. doi: 10.1016/J.ATHORACSUR.2022.11.023
25. Müller J, Wallukat G, Weng YG, Dandel M, Spiegelsberger S, Semrau S, et al. Weaning from mechanical cardiac support in patients with idiopathic dilated cardiomyopathy. Circulation. (1997) 96:542–9. doi: 10.1161/01.CIR.96.2.542
26. Dandel M, Hetzer R. Myocardial recovery during mechanical circulatory support: weaning and explantation criteria. Heart Lung Vessel. (2015) 7:280–8. http://www.ncbi.nlm.nih.gov/pubmed/26811833
27. Dandel M, Weng Y, Siniawski H, Potapov E, Lehmkuhl HB, Hetzer R. Long-term results in patients with idiopathic dilated cardiomyopathy after weaning from left ventricular assist devices. Circulation. (2005) 112:I37–45. doi: 10.1161/CIRCULATIONAHA.104.525352
28. Frazier OH, Baldwin ACW, Demirozu ZT, Segura AM, Hernandez R, Taegtmeyer H, et al. Ventricular reconditioning and pump explantation in patients supported by continuous-flow left ventricular assist devices. J Heart Lung Transplant. (2015) 34(6):766–72. doi: 10.1016/J.HEALUN.2014.09.015
29. Topkara VK, Garan AR, Fine B, Godier-Furnémont AF, Breskin A, Cagliostro B, et al. Myocardial recovery in patients receiving contemporary left ventricular assist devices. Circ Heart Fail. (2016) 9:7. doi: 10.1161/CIRCHEARTFAILURE.116.003157/-/DC1
30. Pan S, Aksut B, Wever-Pinzon OE, Rao SD, Levin AP, Garan AR, et al. Incidence and predictors of myocardial recovery on long-term left ventricular assist device support: results from the united network for organ sharing database. J Heart Lung Transplant. (2015) 34(12):1624–9. doi: 10.1016/j.healun.2015.08.004
31. Birks EJ, Drakos SG, Patel SR, Lowes BD, Selzman CH, Starling RC, et al. Prospective multicenter study of myocardial recovery using left ventricular assist devices (RESTAGE-HF [remission from stage D heart failure]) medium-term and primary end point results. Circulation. (2020) 142:2016–28. doi: 10.1161/CIRCULATIONAHA.120.046415
32. Teuteberg JJ, Cleveland JC, Cowger J, Higgins RS, Goldstein DJ, Keebler M, et al. The society of thoracic surgeons intermacs 2019 annual report: the changing landscape of devices and indications. Ann Thorac Surg. (2020) 109(3):649–60. doi: 10.1016/J.ATHORACSUR.2019.12.005
33. Estep JD, Starling RC, Horstmanshof DA, Milano CA, Selzman CH, Shah KB, et al. Risk assessment and comparative effectiveness of left ventricular assist device and medical management in ambulatory heart failure patients: results from the ROADMAP study. J Am Coll Cardiol. (2015) 66(16):1747–61. doi: 10.1016/J.JACC.2015.07.075
34. Starling RC, Estep JD, Horstmanshof DA, Milano CA, Stehlik J, Shah KB, et al. Risk assessment and comparative effectiveness of left ventricular assist device and medical management in ambulatory heart failure patients: the ROADMAP study 2-year results. JACC Heart Fail. (2017) 5(7):518–27. doi: 10.1016/J.JCHF.2017.02.016
35. Ambardekar AV, Kittleson MM, Palardy M, Mountis MM, Forde-McLean RC, DeVore AD, et al. Outcomes with ambulatory advanced heart failure from the medical arm of mechanically assisted circulatory support (MedaMACS) registry. J Heart Lung Transpl. (2019) 38(4):408. doi: 10.1016/J.HEALUN.2018.09.021
36. Brinkley DM, DeNofrio D, Ruthazer R, Vest AR, Kapur NK, Couper GS, et al. Outcomes after continuous-flow left ventricular assist device implantation as destination therapy at transplant versus nontransplant centers. Circ Heart Fail. (2018) 11(3):e004384. doi: 10.1161/CIRCHEARTFAILURE.117.004384
37. Zein R, Patel C, Mercado-Alamo A, Schreiber T, Kaki A. A review of the impella devices. Interv Cardiol Rev Res Resour. (2022) 17:e05. doi: 10.15420/icr.2021.11
38. Gottula AL, Shaw CR, Milligan J, Chuko J, Lauria M, Swiencki A, et al. Impella in transport: physiology, mechanics, complications, and transport considerations. Air Med J. (2022) 41:114–27. doi: 10.1016/j.amj.2021.10.003
39. Glazier JJ, Kaki A. The impella device: historical background. Clinical applications and future directions. Int J Angiol. (2019) 28:118–23. doi: 10.1055/s-0038-1676369
40. Wernly B, Seelmaier C, Leistner D, Stähli BE, Pretsch I, Lichtenauer M, et al. Mechanical circulatory support with impella versus intra-aortic balloon pump or medical treatment in cardiogenic shock—a critical appraisal of current data. Clin Res Cardiol. (2019) 108:1249–57. doi: 10.1007/s00392-019-01458-2
41. Panuccio G, Neri G, Macrì LM, Salerno N, De Rosa S, Torella D. Use of impella device in cardiogenic shock and its clinical outcomes: a systematic review and meta-analysis. IJC Heart Vasc. (2022) 40:101007. doi: 10.1016/j.ijcha.2022.101007
42. Jain P, Shehab S, Muthiah K, Robson D, Granegger M, Drakos SG, et al. Insights into myocardial oxygen consumption, energetics, and efficiency under left ventricular assist device support using noninvasive pressure-volume loops. Circ Heart Fail. (2019) 12(10):e006191. doi: 10.1161/CIRCHEARTFAILURE.119.006191
43. Madigan JD, Barbone A, Choudhri AF, Morales DLS, Cai B, Oz MC, et al. Time course of reverse remodeling of the left ventricle during support with a left ventricular assist device. J Thorac Cardiovasc Surg. (2001) 121(5):902–8. doi: 10.1067/mtc.2001.112632
44. Burkhoff D, Topkara VK, Sayer G, Uriel N. Reverse remodeling with left ventricular assist devices. Circ Res. (2021) 128(10):1594–612. doi: 10.1161/CIRCRESAHA.121.318160
45. Meyns B, Stolinski J, Leunens V, Verbeken E, Flameng W. Left ventricular support by catheter-mounted axial flow pump reduces infarct size. J Am Coll Cardiol. (2003) 41:1087–95. doi: 10.1016/S0735-1097(03)00084-6
46. Tobushi T, Nakano M, Hosokawa K, Koga H, Yamada A. Improved diastolic function is associated with higher cardiac output in patients with heart failure irrespective of left ventricular ejection fraction. J Am Heart Assoc. (2017) 6:3. doi: 10.1161/JAHA.116.003389
47. Hoffman JIE, Buckberg GD. The myocardial oxygen supply:demand index revisited. J Am Heart Assoc. (2014) 3:285–305. doi: 10.1161/JAHA.113.000285
48. Buckberg GD, Fixler DE, Archie JP, Hoffman JI. Experimental subendocardial ischemia in dogs with normal coronary arteries. Circ Res. (1972) 30:67–81. doi: 10.1161/01.RES.30.1.67
49. Attinger-Toller A, Bossard M, Cioffi GM, Tersalvi G, Madanchi M, Bloch A, et al. Ventricular unloading using the ImpellaTM device in cardiogenic shock. Front Cardiovasc Med. (2022) 9:856870. doi: 10.3389/fcvm.2022.856870
50. Tersalvi G, Attinger-Toller A, Kalathil D, Winterton D, Cioffi GM, Madanchi M, et al. Trajectories of cardiac function following treatment with an impella device in patients with acute anterior ST-elevation myocardial infarction. CJC Open. (2023) 5(1):77–85. doi: 10.1016/J.CJCO.2022.11.002
51. Fincke R, Hochman JS, Lowe AM, Menon V, Slater JN, Webb JG, et al. Cardiac power is the strongest hemodynamic correlate of mortality in cardiogenic shock: a report from the SHOCK trial registry. J Am Coll Cardiol. (2004) 44:340–8. doi: 10.1016/j.jacc.2004.03.060
52. O'Neill WW, Kleiman NS, Moses J, Henriques JPS, Dixon S, Massaro J, et al. A prospective, randomized clinical trial of hemodynamic support with impella 2.5 versus intra-aortic balloon pump in patients undergoing high-risk percutaneous coronary intervention: the PROTECT II study. Circulation. (2012) 126:1717–27. doi: 10.1161/CIRCULATIONAHA.112.098194
53. Belkin MN, Kalantari S, Kanelidis AJ, Miller T, Smith BA, Besser SA, et al. Aortic pulsatility index: a novel hemodynamic variable for evaluation of decompensated heart failure. J Card Fail. (2021) 27:1045–52. doi: 10.1016/j.cardfail.2021.05.010
54. Belkin MN, Shah J, Neyestanak ME, Burkhoff D, Grinstein J. Should we be using aortic pulsatility index over cardiac power output in heart failure cardiogenic shock? Circ Heart Fail. (2022) 15:e009601. doi: 10.1161/CIRCHEARTFAILURE.122.009601
55. Ouweneel DM, Eriksen E, Sjauw KD, van Dongen IM, Hirsch A, Packer EJS, et al. Percutaneous mechanical circulatory support versus intra-aortic balloon pump in cardiogenic shock after acute myocardial infarction. J Am Coll Cardiol. (2017) 69:278–87. doi: 10.1016/j.jacc.2016.10.022
56. Saku K, Kakino T, Arimura T, Sakamoto T, Nishikawa T, Sakamoto K, et al. Total mechanical unloading minimizes metabolic demand of left ventricle and dramatically reduces infarct size in myocardial infarction. PLoS ONE. (2016) 11:e0152911. doi: 10.1371/journal.pone.0152911
57. Remmelink M, Sjauw KD, Henriques JPS, De Winter RJ, Koch KT, Van Der Schaaf RJ, et al. Effects of left ventricular unloading by impella recover LP2.5 on coronary hemodynamics. Catheter Cardiovasc Interv. (2007) 70:532–7. doi: 10.1002/ccd.21160
58. Saito T, Yamamoto H, Oishi S, Uchino G, Murakami H, Kawai H, et al. Left main trunk occlusion due to impella-related thrombus in a patient with extracorporeal cardiopulmonary resuscitation. JACC Cardiovasc Interv. (2021) 14:e313–e6. doi: 10.1016/j.jcin.2021.08.043
59. Ambardekar AV, Weiser-Evans MCM, Li M, Purohit SN, Aftab M, Reece TB, et al. Coronary artery remodeling and fibrosis with continuous-flow left ventricular assist device support. Circ Heart Fail. (2018) 11:e004491. doi: 10.1161/CIRCHEARTFAILURE.117.004491
60. Fujino T, Sayer A, Nitta D, Imamura T, Narang N, Nguyen A, et al. Longitudinal trajectories of hemodynamics following left ventricular assist device implantation. J Card Fail. (2020) 26(5):383–90. doi: 10.1016/J.CARDFAIL.2020.01.020
61. Kang G, Ha R, Banerjee D. Pulmonary artery pulsatility index predicts right ventricular failure after left ventricular assist device implantation. J Heart Lung Transplant. (2016) 35(1):67–73. doi: 10.1016/j.healun.2015.06.009
62. Morine KJ, Kiernan MS, Pham DT, Paruchuri V, Denofrio D, Kapur NK. Pulmonary artery pulsatility index is associated with right ventricular failure after left ventricular assist device surgery. J Card Fail. (2016) 22(2):110–6. doi: 10.1016/j.cardfail.2015.10.019
63. Addetia K, Uriel N, Maffessanti F, Sayer G, Adatya S, Kim GH, et al. 3D morphological changes in LV and RV during LVAD ramp studies. JACC Cardiovasc Imaging. (2018) 11(2):159–69. doi: 10.1016/J.JCMG.2016.12.019
64. Jorde UP, Uriel N, Nahumi N, Bejar D, Gonzalez-Costello J, Thomas SS, et al. Prevalence, significance, and management of aortic insufficiency in continuous flow left ventricular assist device recipients. Circ Heart Fail. (2014) 7:310–9. doi: 10.1161/CIRCHEARTFAILURE.113.000878
65. Cowger JA, Aaronson KD, Romano MA, Haft J, Pagani FD. Consequences of aortic insufficiency during long-term axial continuous-flow left ventricular assist device support. J Heart Lung Transplant. (2014) 33(12):1233–40. doi: 10.1016/j.healun.2014.06.008
66. Grinstein J, Blanco PJ, Bulant CA, Torii R, Bourantas CV, Lemos PA, et al. A computational study of aortic insufficiency in patients supported with continuous flow left ventricular assist devices: is it time for a paradigm shift in management? Front Cardiovasc Med. (2022) 9:1–13. doi: 10.3389/fcvm.2022.933321
67. Kukucka M, Stepanenko A, Potapov E, Krabatsch T, Redlin M, Mladenow A, et al. Right-to-left ventricular end-diastolic diameter ratio and prediction of right ventricular failure with continuous-flow left ventricular assist devices. J Heart Lung Transplant. (2011) 30:64–9. doi: 10.1016/j.healun.2010.09.006
68. Drakos SG, Terrovitis JV, Anastasiou-Nana MI, Nanas JN. Reverse remodeling during long-term mechanical unloading of the left ventricle. J Mol Cell Cardiol. (2007) 43(3):231–42. doi: 10.1016/j.yjmcc.2007.05.020
69. Ibrahim M, Al Masri A, Navaratnarajah M, Siedlecka U, Soppa GK, Moshkov A, et al. Prolonged mechanical unloading affects cardiomyocyte excitation-contraction coupling, transverse-tubule structure, and the cell surface. FASEB J. (2010) 24(9):3321–9. doi: 10.1096/fj.10-156638
70. Shah P, Psotka M, Taleb I, Alharethi R, Shams MA, Wever-Pinzon O, et al. Framework to classify reverse cardiac remodeling with mechanical circulatory support: the Utah-inova stages. Circ Heart Fail. (2021) 14(5):E007991. doi: 10.1161/CIRCHEARTFAILURE.120.007991
71. Mccarthy PM, Nakatani S, Vargo R, Kottke-Marchant K, Harasaki H, James KB, et al. Structural and left ventricular histologic changes after implantable LVAD insertion. Ann Thorac Surg. (1995) 59:609–22. doi: 10.1016/0003-4975(94)00953-8
72. Hutchins GM, Bulkley BH, Moore GW, Piasio MA, Lohr FT. Shape of the human cardiac ventricles. Am J Cardiol. (1978) 41(4):646–54. doi: 10.1016/0002-9149(78)90812-3
73. Uriel N, Sayer G, Addetia K, Fedson S, Kim GH, Rodgers D, et al. Hemodynamic ramp tests in patients with left ventricular assist devices. JACC Heart Fail. (2016) 4(3):208–17. doi: 10.1016/J.JCHF.2015.10.001
74. Sayer G, Medvedofsky D, Imamura T, Kim G, Maffessanti F, Fried J, et al. Short-term ventricular structural changes following left ventricular assist device implantation. ASAIO J. (2021) 67:169–76. doi: 10.1097/MAT.0000000000001214
75. Levy D, Garrison RJ, Savage DD, Kannel WB, Castelli WP. Prognostic implications of echocardiographically determined left ventricular mass in the framingham heart study. N Engl J Med. (2010) 322(22):1561–6. doi: 10.1056/NEJM199005313222203
76. Bruckner BA, Stetson SJ, Perez-Verdia A, Youker KA, Radovancevic B, Connelly JH, et al. Regression of fibrosis and hypertrophy in failing myocardium following mechanical circulatory support. J Heart Lung Transplant. (2001) 20(4):457–64. doi: 10.1016/S1053-2498(00)00321-1
77. Zafeiridis A, Jeevanandam V, Houser SR, Margulies KB. Regression of cellular hypertrophy after left ventricular assist device support. Circulation. (1998) 98(7):656–62. doi: 10.1161/01.CIR.98.7.656
78. Barbone A, Holmes JW, Heerdt PM, The’ AHS, Naka Y, Joshi N, et al. Comparison of right and left ventricular responses to left ventricular assist device support in patients with severe heart failure. Circulation. (2001) 104(6):670–5. doi: 10.1161/HC3101.093903
79. Kinoshita M, Takano H, Takaichi S, Taenaka Y, Nakatani T. Influence of prolonged ventricular assistance on myocardial histopathology in intact heart. Ann Thorac Surg. (1996) 61:640–5. doi: 10.1016/0003-4975(95)01087-4
80. Razeghi P, Sharma S, Ying J, Li YP, Stepkowski S, Reid MB, et al. Atrophic remodeling of the heart in vivo simultaneously activates pathways of protein synthesis and degradation. Circulation. (2003) 108(20):2536–41. doi: 10.1161/01.CIR.0000096481.45105.13
81. Diakos NA, Selzman CH, Sachse FB, Stehlik J, Kfoury AG, Wever-Pinzon O, et al. Myocardial atrophy and chronic mechanical unloading of the failing human heart: implications for cardiac assist device–induced myocardial recovery. J Am Coll Cardiol. (2014) 64(15):1602–12. doi: 10.1016/J.JACC.2014.05.073
82. Rose AG, Park SJ. Pathology in patients with ventricular assist devices: a study of 21 autopsies, 24 ventricular apical core biopsies and 24 explanted hearts. Cardiovasc Pathol. (2005) 14(1):19–23. doi: 10.1016/J.CARPATH.2004.10.002
83. Aquila LA, McCarthy PM, Smedira NG, Young JB, Moravec CS. Cytoskeletal structure and recovery in single human cardiac myocytes. J Heart Lung Transplant. (2004) 23(8):954–63. doi: 10.1016/J.HEALUN.2004.05.018
84. De Jonge N, Lahpor JR, Van Wichen DF, Kirkels H, Gmelig-Meyling FHJ, Van Den Tweel JG, et al. Similar left and right ventricular sarcomere structure after support with a left ventricular assist device suggests the utility of right ventricular biopsies to monitor left ventricular reverse remodeling. Int J Cardiol. (2005) 98(3):465–70. doi: 10.1016/J.IJCARD.2003.12.020
85. Latif N, Yacoub MH, George R, Barton PJR, Birks EJ. Changes in sarcomeric and non-sarcomeric cytoskeletal proteins and focal adhesion molecules during clinical myocardial recovery after left ventricular assist device support. J Heart Lung Transplant. (2007) 26(3):230–5. doi: 10.1016/J.HEALUN.2006.08.011
86. Vatta M, Stetson SJ, Jimenez S, Entman ML, Noon GP, Bowles NE, et al. Molecular normalization of dystrophin in the failing left and right ventricle of patients treated with either pulsatile or continuous flow-type ventricular assist devices. J Am Coll Cardiol. (2004) 43(5):811–7. doi: 10.1016/J.JACC.2003.09.052
87. Heerdt PM, Holmes JW, Cai B, Barbone A, Madigan JD, Reiken S, et al. Chronic unloading by left ventricular assist device reverses contractile dysfunction and alters gene expression in end-stage heart failure. Circulation. (2000) 102(22):2713–9. doi: 10.1161/01.CIR.102.22.2713
88. Klotz S, Barbone A, Reiken S, Holmes JW, Naka Y, Oz MC, et al. Left ventricular assist device support normalizes left and right ventricular beta-adrenergic pathway properties. J Am Coll Cardiol. (2005) 45(5):668–76. doi: 10.1016/J.JACC.2004.11.042
89. Bravo CA, Navarro AG, Dhaliwal KK, Khorsandi M, Keenan JE, Mudigonda P, et al. Right heart failure after left ventricular assist device: from mechanisms to treatments. Front Cardiovasc Med. (2022) 9:1023549. doi: 10.3389/fcvm.2022.1023549
90. Dipla K, Mattiello JA, Jeevanandam V, Houser SR, Margulies KB. Myocyte recovery after mechanical circulatory support in humans with end-stage heart failure. Circulation. (1998) 97(23):2316–22. doi: 10.1161/01.CIR.97.23.2316
91. Ogletree-Hughes ML, Stull LB, Sweet WE, Smedira NG, McCarthy PM, Moravec CS. Mechanical unloading restores beta-adrenergic responsiveness and reverses receptor downregulation in the failing human heart. Circulation. (2001) 104(8):881–6. doi: 10.1161/HC3301.094911
92. Ferrara N, Komici K, Corbi G, Pagano G, Furgi G, Rengo C, et al. β-adrenergic receptor responsiveness in aging heart and clinical implications. Front Physiol. (2013) 4:396. doi: 10.3389/FPHYS.2013.00396
93. Merlet P, Valette H, Dubois-Rande JL, Moyse D, Duboc D, Dove P, et al. Prognostic value of cardiac metaiodobenzylguanidine imaging in patients with heart failure. J Nucl Med. (1992) 33:471–7. doi: 10.1016/j.ijcard.2019.07.068
94. Takawale A, Sakamuri SSVP, Kassiri Z. Extracellular matrix communication and turnover in cardiac physiology and pathology. Compr Physiol. (2015) 5(2):687–719. doi: 10.1002/CPHY.C140045
95. Maybaum S, Mancini D, Xydas S, Starling RC, Aaronson K, Pagani FD, et al. Cardiac improvement during mechanical circulatory support. Circulation. (2007) 115(19):2497–505. doi: 10.1161/CIRCULATIONAHA.106.633180
96. Kato TS, Chokshi A, Singh P, Khawaja T, Cheema F, Akashi H, et al. Effects of continuous-flow versus pulsatile-flow left ventricular assist devices on myocardial unloading and remodeling. Circ Heart Fail. (2011) 4(5):546–53. doi: 10.1161/CIRCHEARTFAILURE.111.962142/-/DC1
97. Catino AB, Ferrin P, Wever-Pinzon J, Horne BD, Wever-Pinzon O, Kfoury AG, et al. Clinical and histopathological effects of heart failure drug therapy in advanced heart failure patients on chronic mechanical circulatory support. Eur J Heart Fail. (2018) 20(1):164–74. doi: 10.1002/EJHF.1018
98. Castillero E, Ali ZA, Akashi H, Giangreco N, Wang C, Stöhr EJ, et al. Structural and functional cardiac profile after prolonged duration of mechanical unloading: potential implications for myocardial recovery. Am J Physiol Heart Circ Physiol. (2018) 315(5):H1463–76. doi: 10.1152/AJPHEART.00187.2018/SUPPL_FILE/SUPPLEMENTAL
99. Drakos SG, Kfoury AG, Hammond EH, Reid BB, Revelo MP, Rasmusson BY, et al. Impact of mechanical unloading on microvasculature and associated central remodeling features of the failing human heart. J Am Coll Cardiol. (2010) 56(5):382–91. doi: 10.1016/J.JACC.2010.04.019
100. Li YY, Feng Y, McTiernan CF, Pei W, Moravec CS, Wang P, et al. Downregulation of matrix metalloproteinases and reduction in collagen damage in the failing human heart after support with left ventricular assist devices. Circulation. (2001) 104(10):1147–52. doi: 10.1161/HC3501.095215
101. Bruggink AH, van Oosterhout MFM, de Jonge N, Ivangh B, van Kuik J, Voorbij RHAM, et al. Reverse remodeling of the myocardial extracellular matrix after prolonged left ventricular assist device support follows a biphasic pattern. J Heart Lung Transplant. (2006) 25(9):1091–8. doi: 10.1016/j.healun.2006.05.011
102. Saraon T, Katz SD. Reverse remodeling in systolic heart failure. Cardiol Rev. (2015) 23(4):173–81. doi: 10.1097/crd.0000000000000068
103. Birks EJ, George RS, Hedger M, Bahrami T, Wilton P, Bowles CT, et al. Reversal of severe heart failure with a continuous-flow left ventricular assist device and pharmacological therapy. Circulation. (2011) 123(4):381–90. doi: 10.1161/CIRCULATIONAHA.109.933960
Keywords: cardiac remodeling, left ventricular assist device (LVAD), impella, pressure volume (PV) curve, reverse remodeling, hemodynamics
Citation: Pamias-Lopez B, Ibrahim ME and Pitoulis FG (2023) Cardiac mechanics and reverse remodelling under mechanical support from left ventricular assist devices. Front. Cardiovasc. Med. 10:1212875. doi: 10.3389/fcvm.2023.1212875
Received: 27 April 2023; Accepted: 5 July 2023;
Published: 2 August 2023.
Edited by:
Sebastian Kelle, German Heart Center Berlin, GermanyReviewed by:
Jonathan Grinstein, The University of Chicago, United StatesKnosalla Christoph, German Heart Center Berlin, Germany
Matthew Caporizzo, University of Vermont, United States
© 2023 Pamias-Lopez, Ibrahim and Pitoulis. This is an open-access article distributed under the terms of the Creative Commons Attribution License (CC BY). The use, distribution or reproduction in other forums is permitted, provided the original author(s) and the copyright owner(s) are credited and that the original publication in this journal is cited, in accordance with accepted academic practice. No use, distribution or reproduction is permitted which does not comply with these terms.
*Correspondence: Fotios G. Pitoulis ZnAxMzE0QGljLmFjLnVr