- 1Toronto General Hospital Research Institute, University Health Network, Toronto, ON, Canada
- 2Institute of Medical Science, University of Toronto, Toronto, ON, Canada
- 3Division of Vascular Surgery, Department of Surgery, University of Toronto, Toronto, ON, Canada
- 4Peter Munk Cardiac Centre, University Health Network, Toronto, ON, Canada
Extracellular vesicles (EVs) are small, lipid bilayer-enclosed structures released by various cell types that play a critical role in intercellular communication. In atherosclerosis, EVs have been implicated in multiple pathophysiological processes, including endothelial dysfunction, inflammation, and thrombosis. This review provides an up-to-date overview of our current understanding of the roles of EVs in atherosclerosis, emphasizing their potential as diagnostic biomarkers and their roles in disease pathogenesis. We discuss the different types of EVs involved in atherosclerosis, the diverse cargoes they carry, their mechanisms of action, and the various methods employed for their isolation and analysis. Moreover, we underscore the importance of using relevant animal models and human samples to elucidate the role of EVs in disease pathogenesis. Overall, this review consolidates our current knowledge of EVs in atherosclerosis and highlights their potential as promising targets for disease diagnosis and therapy.
1. Introduction
Atherosclerosis is a significant cause of cardiovascular disease (CVD) that can lead to heart attack, stroke, kidney failure, and major amputation (1–4). Approximately 17.9 million people die from CVD annually (5). Atherosclerosis is a chronic inflammatory process characterized by endothelial activation, accumulation of lipoproteins, and recruitment of inflammatory cells that leads to plaques that gradually enlarge and either restrict blood flow or embolize, damaging the heart or peripheral tissues (6). The current diagnostic methods for atherosclerosis are associated with rare but significant procedure-related consequences and considerable cost (7, 8). The classical biomarkers, such as total cholesterol, low-density lipoprotein (LDL), or serum triglyceride levels, are the gold standard diagnostic tests for atherosclerosis (9). C-reactive protein, a non-specific inflammatory marker, has emerged as a clinical marker for residual risk in atherosclerosis patients with good cholesterol control (10, 11). Many of these biomarkers can diagnose CVD but cannot definitively predict stroke or myocardial infarction (MI) risk. There is a need for new CVD biomarkers that are cost-effective, improve detection, and identify novel treatment targets. As we enter the era of precision medicine, we need a more granular understanding of biomarkers that can be used as reliable screening tools with metrics to guide personalized intervention to prevent devastating clinical events.
The American Heart Association proposed seven metrics in 2010 to define and monitor cardiovascular health (12). Managing the disease involves non-pharmacological methods (healthy diet, regular physical activity, and tobacco abstinence) (1) and pharmacological interventions such as statins to control lipoprotein levels (13–15), with newer options such as cholesterol-binding agents (e.g., ezetimibe) (16) and proprotein convertase subtilisin/kexin type 9 (PCSK9, lowers LDL) inhibitors (e.g., evolocumab) (17–19) also available. Notably, several studies have highlighted challenges in achieving therapeutic goals for serum lipids despite high-intensity statin therapy (20–22). In some cases, surgery or stent-based therapies are required to manage more severe atherosclerosis. While current strategies can slow the progression of atherosclerosis and/or prevent clinical events (23), further research is needed to understand the specific cellular and molecular mechanisms underpinning plaque progression to identify targets for stabilization and/or plaque regression. One area of promise includes delineating cellular communication during atherosclerotic plaque development and progression. In this regard, extracellular vesicles (EVs) have been identified as essential cell-cell communicators that may hold promise in improving our understanding of atherosclerotic disease—from biomarkers to disease pathogenesis (24–26) (Figure 1).
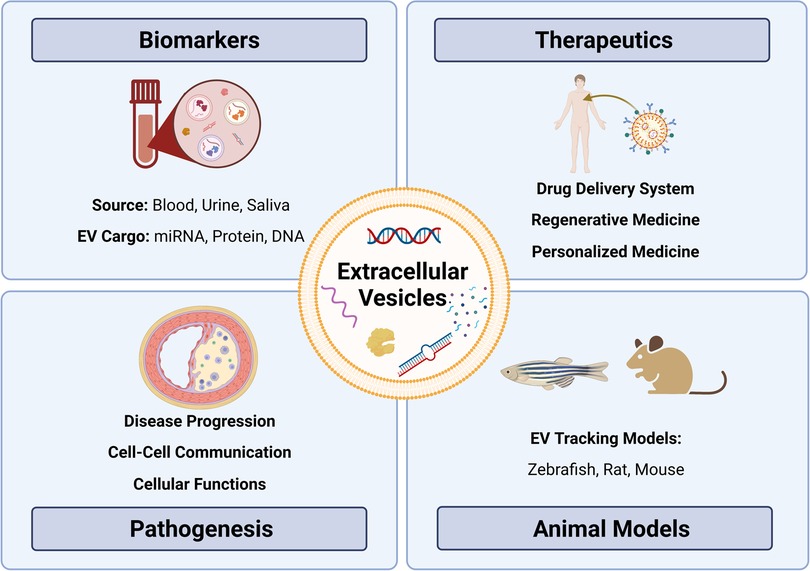
Figure 1. Exploring the role of extracellular vesicles in atherosclerosis: insights from biomarkers, therapeutics, pathobiology, and translational models. Extracellular vesicles (EVs) as versatile entities for various applications. EVs, small membrane-bound particles, have emerged as promising biomarkers for diagnostic and prognostic purposes in various diseases. They have also shown great potential as therapeutic agents for their ability to carry and deliver bioactive molecules. Moreover, EVs have been implicated in the pathogenesis of many diseases, including cancer, neurodegenerative diseases, and atherosclerosis. Animal studies have contributed significantly to our understanding of the biology and functions of EVs, paving the way for their clinical translation.
2. Extracellular vesicles
2.1. Biogenesis, cargo, and functions
EVs are lipid bilayer-bound particles that all cell types release into the extracellular space. They can be classified into three major types based on their biogenesis, morphological, and biochemical properties: exosomes (form as intraluminal vesicles within multivesicular bodies that fuse with the plasma membrane, 30–100 nm), microvesicles (directly bud off from healthy plasma membrane/also referred to as ectosomes, 100–1,000 nm) and apoptotic bodies (form during apoptosis, 1–5 µm) (27, 28). Furthermore, EVs carry cargo that contains biologically active materials, such as DNA, microRNA, messenger RNA, proteins, lipids, and carbohydrates. Once released into the extracellular space, EVs may directly interact with nearby cells (28). EVs can enter biological fluids via transcytosis or by breaching biological barriers, where they can travel throughout the body via the circulation—either blood or lymphatics (28, 29). EVs can then be taken up by recipient cells via endocytosis, fusion with the recipient cell plasma membrane, or binding to target cell membrane proteins (30, 31). The transferred cargo to recipient cells can affect molecular and cellular signalling pathways and functions.
Current EV isolation permits classification based on size, density and surface markers but does not discriminate based on biogenesis (28). That said, proteomic analysis has revealed distinct protein composition for EV subtypes (32), with some markers helping to distinguish EVs by biogenesis pathways. For example, exosome markers include endosomal sorting complexes required for transport (ESCRT) proteins, Alix and tetraspanins, while ectosome markers include Annexin A2/A5, ARF6 and Enolase 1 (32). Although advanced technology will undoubtedly yield more discrimination between EV populations, some promise exists in using inhibition of EV biogenesis by pharmacological therapies. For example, inhibitors of cancer exosome secretion may impact cancer progression and metastasis (33). Ultimately, the ideal strategy will be to find specific inhibitors that can impact EVs associated with pathology but not those that play critical physiological roles (34). To do this, we will need a more nuanced understanding of the kinetics of EV release from the host cell, travel within the circulation, recipient cell uptake, and EV clearance.
EV cargo is biologically active. In cancer, EV cargo can promote neoplastic transformation and cell proliferation, contributing to cancer initiation and progression (35–38). During atherogenesis, EVs released from endothelial cells (ECs) and immune cells promote leukocyte infiltration and plaque maturation (39–41). This suggests that EVs circulating in the plasma could serve as non-invasive disease biomarkers. Both in vitro and in vivo studies have shown that circulating EVs carry microRNA, which can be biomarkers for neurodegenerative diseases (42–45) and CVD (46). EVs possess several unique advantages compared to traditional biomarkers (47). EVs are stably circulated in almost all bodily fluids, they can represent the current disease state by carrying specific cargo from parental cells, and they can be collected sequentially. As a result, EVs have significant potential as clinically valuable biomarkers capable of providing multiple, minimally intrusive assessments of the disease state.
EVs can also be used as a stable drug delivery system that protects cargo from degradation (48–54). EVs have numerous advantages over cell-based therapies in regenerative medicine, such as long shelf life, ease of transportation, long-term storage, and lack of replication (55–57). As drug delivery vehicles, they outperform synthetic drug carriers by crossing tissue and cellular barriers (48). In preclinical studies, EVs have been used as a drug delivery system. For instance, exosome-mediated siRNA delivery has been used in Alzheimer's disease (45), while mesenchymal stem cell-derived exosomes have been used to treat ischemic lung injury (58) and eye disorders (59). However, further understanding of EV circulation dynamics, targeting, internalization, and intracellular trafficking pathways is needed to fully capitalize on the therapeutic potential.
2.2. Characterization
EV isolation is divided into three main approaches based on size, density, and surface markers (31). Size-exclusion chromatography (SEC) is one commonly used technique for EV isolation based on size, while differential ultracentrifugation exploits the distinct density gradients of EVs (60). Finally, magnetic beads/affinity chromatography or flow cytometry uses surface markers to extract EVs with high specificity but low yield (31). There is no single gold standard. Although ultracentrifugation has been widely used in the past, there has been a shift towards SEC attributed in part to the higher EV yield and functionality obtained through SEC (61, 62).
The Minimal Information for Studies of Extracellular Vesicles (MISEV) 2018 provides a key tool for standardizing EV research (31) and has helped to establish rigour in a rapidly emerging field of research by outlining criteria for EV quantification and characterization. Suggestions include EV quantification by nanoparticle tracking analysis (NTA) (63), characterization by surface marker protein expression using western blot (31), and purity control to detect the presence of non-vesicular contamination, such as apolipoprotein A1 and albumin in EVs enriched from plasma (64). Imaging EVs via electron microscopy is recommended (63), while flow cytometry detecting surface markers can be used to characterize the cellular origin of EVs (65, 66). In this way, rigorous determination of cell-specific EVs holds promise as highly specific biomarkers for a disease state. EVs can be further characterized by analyzing their cargo. Mass spectrometry has been used to study EV proteomics in biofluids and tissues (67, 68). Similarly, transcriptomics has been employed to investigate the nucleic acid cargo of EVs, specifically microRNA cargo, primarily through microarrays and RT-qPCR, which are limited to a particular RNA panel (69).
Despite the considerable advancement in technology for EV isolation and characterization, limitations and challenges remain. However, as developing technology continues to refine EV research, it is becoming clear that EVs play crucial roles in biological processes, govern disease, and have emerged as a new avenue in atherosclerosis research.
3. EVs in atherosclerosis
3.1. EVs in plasma
EVs might serve as diagnostic and therapeutic tools for many CVD conditions. EV levels in the blood, urine and saliva have been linked to clinical risk in patients with stable CVD (70) (Table 1). Elevated EVs are associated with risk factors such as smoking, diabetes, and hypercholesteremia (84). The abundance of EVs carried in plasma reflects the potential for utilizing these EVs as biomarkers for CVD, and notably that EVs derived from specific cell types, such as ECs, leukocytes and platelets, correlate with CVD (85). A previous study exploited the surface markers expressed on EVs to purify and isolate cell-specific EVs, followed by enrichment and analysis of EV cargo. In EVs isolated from plasma, CD14 upregulation was linked to a higher risk of ischemic stroke occurrence (80), while increased cystatin C and polygenic immunoglobin receptors were linked to acute coronary syndrome (77).
Circulating EVs from different cellular origins and their distinct cargo (e.g., microRNA, protein) have been linked to pathological conditions such as dyslipidemia, diabetes (86–88), CVD (60, 79), and inflammatory disorders (89–91). This suggests that EVs play a role in the immune response, vascular remodelling, endothelial dysfunction, and apoptosis, all of which underlie atherosclerosis (92, 93). Studies have shown that leukocyte-derived, neutrophil-derived, and activated platelet-derived EVs were significantly higher in patients with atherosclerosis (94, 95). EVs carried in plasma may be helpful as biomarkers for atherosclerosis, but accuracy must be improved to detect changes in EV count from specific cell types. As EVs are heterogeneous in size, composition, and cellular origin, it makes identifying specific populations and correlating them to disease challenging (46). In addition to EV heterogeneity, clinical variables (e.g., age, sex), comorbidities (e.g., obesity), and clinical history (e.g., cancer, medications) affect circulating EVs in plasma (31).
Furthermore, laboratory standardization will be critical before employing EVs as a biomarker: lack of standard protocols for EV isolation, quantification, and characterization leads to variability in results and negates their utility as a biomarker or clinical assessment tool. EV biomarkers lack a standard reference range, making it difficult to compare among populations and studies. No EV-based biomarker has been adopted for CVD, and more research is needed to develop standard protocols to study plasma EVs. Work is ongoing, as the International Society for Extracellular Vesicles has a specific blood task force focused on standardizing plasma and serum-derived EVs (96).
To address the challenges in EV detection, standardization, and clinical translation, technological improvements, standardized protocols, and prospective large clinical trials are needed (97, 98). Precision medicine EV research has recently become more prominent. It is a potential path that enables physicians and researchers to use patient data to develop personalized treatments. For instance, a multi-biomarker approach may incorporate EV evaluation for screening/diagnosis, prognosis, and monitoring of people at risk of atherosclerotic CVD (97). Several subsets of EV biomarkers can be exploited for patient risk evaluations, reclassification, and disease stage diagnosis (97). Applying transcriptomic and proteomic analysis plus artificial intelligence algorithms to clinical data can help identify high-risk individuals and administer preventive strategies quickly (98).
3.2. EVs in plaque
As EVs protect their molecular cargo from degradation and carry surface markers identifying their parent cell, plasma EVs a unique opportunity to study disease states (diagnostic potential). On the other hand, EVs in tissue can contribute to the pathophysiology or progression of plaques (therapeutic targets) (99). At this time, however, screening and tracing EVs released from cells or tissues in vivo remains challenging. EVs are found in early and advanced plaques, suggesting they are involved in both the initiation and advanced phases of plaque development in humans (100–102). Patients with atherosclerosis demonstrated enrichment of proatherogenic EV cargo, such as vascular cell adhesion molecule-1, von Willebrand Factor, endothelial nitric oxide synthase, and angiopoietin-1, compared to healthy control participants (103, 104). Athough EV production, function, and quantity in atherosclerotic lesions still needs more delineatation, some granularity is emerging, with human atherosclerotic plaques containing EVs derived from leukocytes, macrophages, erythrocytes, lymphocytes, and smooth muscle cells (SMCs) (101, 102, 105).
Most of our current understanding of the role of EVs in atherosclerosis has been obtained from studies using EVs derived from cell cultures, which may not accurately represent EVs found in vivo. Nonetheless, EVs have been found to exert significant influence over a range of pro-atherogenic processes, such as inflammation, thrombosis, and angiogenesis (84). In particular, EC-derived EVs have been implicated in endothelial dysfunction (106, 107) and vascular inflammation (108), which may contribute to the development of early atherosclerotic plaques. Moreover, EC-derived EVs can communicate with macrophages (109, 110) and SMCs (111–113) to regulate vascular disease, while monocyte-derived EVs have been found to modulate vascular inflammation and cell death (114–116). Additionally, foam cell-derived EVs have been shown to regulate SMC migration, thereby potentially accelerating the progression of atherosclerotic lesions (117). EVs produced from a range of cell types, including T-cells, platelets, dendritic cells, and monocytic cells, have also been shown to cause macrophage apoptosis (118–122), that may contribute to the development and progression of atherosclerosis. Moreover, EVs have been found to play various multifaceted and environment-dependent roles in other cellular processes, such as endothelial permeability (123), pro- and anti-inflammatory signaling (124–126), leukocyte transmigration and lipid accumulation (127–129), SMC proliferation (130), intravascular calcification (131), extracellular matrix remodeling (132), and plaque rupture. Collectively, the evidence suggests a substantial role for EVs in atherosclerosis pathogenesis, emphasizing the need for additional research into the mechanisms underlying EV-mediated intercellular communication in this disease. New technologies such as flow cytometry and single EV analysis will increase detection precision and provide more detail on cell-specific EV phenotypes, their cargo, and their role in disease regulation. Until then, an emerging resource for EV studies is the development of multicellular models for tracking.
4. EV tracking animal models
Despite recent discoveries, it is still challenging to understand the spatiotemporal distribution and physiological activities of EVs in vivo. Little is known about the biological activities of EVs in vivo, including tissue distribution, blood levels, and clearance dynamics. EVs have been investigated in several disease-simulating animal models, including mouse, rats, and zebrafish.
The transparent nature of zebrafish makes them an ideal model (133, 134). The transgenic zebrafish model was recently established, enabling in vivo identification, tracking, and isolation of endogenous EVs produced by different cell types (135). A cell membrane-tethered fluorophore reporter system in the zebrafish allows cell-specific EV tracking and the potential to track EVs in cell-cell communication within the cardiovascular system (135). In vivo, a live EV tracking model of zebrafish demonstrated inter-organ communication by endogenous exosomes (136). Despite these successes, zebrafish EV tracking models have technological limitations (136) and consequently, more complex models are needed.
EVs have also been studied using more advanced organisms for models of diseases such as cancer (137, 138) and neurological disorders (139–141). For example, a murine model was used to determine the therapeutic effects of immunity and matrix regulatory cell-derived EVs on idiopathic pulmonary fibrosis (142). Several studies have used the rat model to study the role of EVs in spinal cord injuries (143, 144) and repetitive stress (145). Rat models have also been used to investigate the therapeutic potential of EVs to treat diseases such as small cerebral vessel disease (146), colo-cutaneous post-surgical fistula (147), and congenital diaphragmatic hernia (148).
EVs and their relevance to various disease processes have been investigated over the past decade, but EV tracking in vivo remains challenging. Understanding EV biodistribution throughout an organism will be essential before use in clinical practice. Using an EV tracking mouse model, a few studies have demonstrated EV-mediated cell-cell communication and the effects of EVs produced from tumour cells on distant organs (149–151). Investigators used Cre-LoxP mouse models to study cell or tissue-specific EVs by crossing CD9/CD63-GFPf/f exosome reporter mice with Cre-mice (αMHC-MerCreMer for cardiomyocytes, Pax8-Cre for renal tubular epithelial cells, Cdh5-CreERT2 for ECs, villin-Cre for intestine, and alb-Cre for liver) (152–154). In addition, a transgenic rat model (GFP-tagged human CD63) was employed to determine intercellular and mother-to-child EV transfer in vivo (155). However, a complete understanding of the role of cell-specific EVs in atherosclerotic diseases remains elusive. To investigate the potential of EVs as biomarkers or disease modulators, novel animal models that allow for the tracking, characterization, and evaluation of cell-specific EVs are required.
5. Perspectives for future studies
EVs, known to carry biologically active cargoes, appear to play an important role in the pathogenesis of atherosclerosis. However, studying plaque-derived EVs is challenging due to limited accessibility and the complex composition of plaques. Thus, researchers have turned to studying EVs in circulation, particularly in those carried in plasma, to gain insights into atherosclerosis biology. Although plasma is easily accessible, identifying reliable biomarkers is challenging, and pairing biomarkers with clinical events may not reflect the disease state entirely. Therefore, it is necessary to determine whether disease regions release EVs into circulation, which could serve as a potential biomarker. The paired assessment of EVs from circulation and plaques of the same patient is one possible approach, representing a promising and meaningful strategy for an atherosclerosis study.
Further research must elucidate the precise mechanisms by which plaque-derived EVs contribute to atherosclerosis pathogenesis to identify potential therapeutic targets. The primary challenge in studying EVs within the plaque is determining their source (cell-specific EVs) and their functions on neighbouring cells. Transgenic zebrafish models have demonstrated the feasibility of tracking EVs within the cardiovascular system (135). More sophisticated animal models are essential to enable EV-tracking into plaques, better comprehend EV biogenesis and metabolism, and investigate cell-specific EV roles and functions during disease progression. Plasma-derived EVs (e.g., leukocyte origin) have demonstrated potential as biomarkers for assessing plaque vulnerability in patients (156, 157). The potential for EVs carried in plasma to shed light on the biology of atherosclerotic plaque vulnerability to rupture (leading to clinical events such as MI and stroke) is promising and requires further investigation. Using EVs as therapeutic targets for atherosclerosis is a growing area of interest, given their stability as drug-delivery vehicles (158, 159). Overall, EVs represent a promising area for future research in the field of atherosclerosis.
6. Conclusions
EVs have been recognized as important components in the pathogenesis of atherosclerosis. EVs derived from immune and ECs are implicated in developing and destabilizing atherosclerotic plaques. Plasma EVs carry the potential for conveying information related to the vulnerability of atherosclerotic plaques and can serve as potential biomarkers for atherosclerosis and its associated complications, such as MI and stroke. Moreover, the prospect of utilizing EVs as therapeutic targets for atherosclerosis has recently gained substantial interest. While work remains to improve the tools and standardization of EV research, EVs nonetheless represent an encouraging area for future research in the field of atherosclerosis and hold the potential to provide novel insights into the diagnosis, treatment, and prevention of this chronic inflammatory disease.
Author contributions
SP and KH designed the topic of this review article. SP wrote the manuscript with MKG and MS. SP, MKG, and MS performed the literature review and designed the table. SP created the figure. KH participated in editing and provided conceptual input to the manuscript. All authors contributed to the article and approved the submitted version.
Funding
SP is supported by the Toronto General Hospital Research Institute Postdoctoral Fellowship Award. KH is supported by a Canadian Institutes of Health Research (CIHR) Project Grant PJT178006, the Heart and Stroke Foundation of Canada (New Investigator Award), Vascular Cures (Wylie Scholar Award), Blair Early Career Professorship in Vascular Surgery, Peter Munk Cardiac Centre, and University Health Network.
Acknowledgments
BioRender.com was used in the creation of the figure for this review.
Conflict of interest
The authors declare that the research was conducted in the absence of any commercial or financial relationships that could be construed as a potential conflict of interest.
Publisher's note
All claims expressed in this article are solely those of the authors and do not necessarily represent those of their affiliated organizations, or those of the publisher, the editors and the reviewers. Any product that may be evaluated in this article, or claim that may be made by its manufacturer, is not guaranteed or endorsed by the publisher.
References
1. Libby P, Buring JE, Badimon L, Hansson GK, Deanfield J, Bittencourt MS, et al. Atherosclerosis. Nat Rev Dis Primer. (2019) 5(1):56. doi: 10.1038/s41572-019-0106-z
2. Dichgans M, Pulit SL, Rosand J. Stroke genetics: discovery, biology, and clinical applications. Lancet Neurol. (2019) 18(6):587–99. doi: 10.1016/S1474-4422(19)30043-2
3. Valdivielso JM, Rodríguez-Puyol D, Pascual J, Barrios C, Bermúdez-López M, Sánchez-Niño MD, et al. Atherosclerosis in chronic kidney disease. Arterioscler Thromb Vasc Biol. (2019) 39(10):1938–66. doi: 10.1161/ATVBAHA.119.312705
4. Behroozian A, Beckman JA. Microvascular disease increases amputation in patients with peripheral artery disease. Arterioscler Thromb Vasc Biol. (2020) 40(3):534–40. doi: 10.1161/ATVBAHA.119.312859
5. Cardiovascular diseases (CVDs). (cited 2022 Nov 3). Available at: https://www.who.int/news-room/fact-sheets/detail/cardiovascular-diseases-(cvds)
6. Berliner JA, Navab M, Fogelman AM, Frank JS, Demer LL, Edwards PA, et al. Atherosclerosis: basic mechanisms. Circulation. (1995) 91(9):2488–96. doi: 10.1161/01.CIR.91.9.2488
7. Nelson AJ, Ardissino M, Psaltis PJ. Current approach to the diagnosis of atherosclerotic coronary artery disease: more questions than answers. Ther Adv Chronic Dis. (2019) 10:2040622319884819. doi: 10.1177/2040622319884819
8. Knaapen P. Computed tomography to replace invasive coronary angiography? Circ Cardiovasc Imaging. (2019) 12(2):e008710. doi: 10.1161/CIRCIMAGING.119.008710
9. Upadhyay RK. Emerging risk biomarkers in cardiovascular diseases and disorders. J Lipids. (2015) 2015:971453. doi: 10.1155/2015/971453
10. Badimon L, Peña E, Arderiu G, Padró T, Slevin M, Vilahur G, et al. C-Reactive protein in atherothrombosis and angiogenesis. Front Immunol. (2018) 9:430. doi: 10.3389/fimmu.2018.00430
11. Ridker PM, Everett BM, Thuren T, MacFadyen JG, Chang WH, Ballantyne C, et al. Antiinflammatory therapy with canakinumab for atherosclerotic disease. N Engl J Med. (2017) 377(12):1119–31. doi: 10.1056/NEJMoa1707914
12. Sacco RL. The new American heart association 2020 goal: achieving ideal cardiovascular health. J Cardiovasc Med. (2011) 12(4):255. doi: 10.2459/JCM.0b013e328343e986
13. Cholesterol Treatment Trialists’ (CTT) Collaboration, Baigent C, Blackwell L, Emberson J, Holland LE, Reith C, Bhala N, et al. Efficacy and safety of more intensive lowering of LDL cholesterol: a meta-analysis of data from 170,000 participants in 26 randomised trials. Lancet Lond Engl. (201) 376(9753):1670–81. doi: 10.1016/S0140-6736(10)61350-5
14. Baigent C, Keech A, Kearney PM, Blackwell L, Buck G, Pollicino C, et al. Efficacy and safety of cholesterol-lowering treatment: prospective meta-analysis of data from 90,056 participants in 14 randomised trials of statins. Lancet Lond Engl. (2005) 366(9493):1267–78. doi: 10.1016/S0140-6736(05)67394-1
15. Cholesterol Treatment Trialists’ (CTT) Collaborators. The effects of lowering LDL cholesterol with statin therapy in people at low risk of vascular disease: meta-analysis of individual data from 27 randomised trials. Lancet. (2012) 380(9841):581–90. doi: 10.1016/S0140-6736(12)60367-5
16. Hammersley D, Signy M. Ezetimibe: an update on its clinical usefulness in specific patient groups. Ther Adv Chronic Dis. (2017 Jan) 8(1):4–11. doi: 10.1177/2040622316672544
17. Sabatine MS, Giugliano RP, Keech AC, Honarpour N, Wiviott SD, Murphy SA, et al. Evolocumab and clinical outcomes in patients with cardiovascular disease. N Engl J Med. (2017) 376(18):1713–22. doi: 10.1056/NEJMoa1615664
18. Farmaki P, Damaskos C, Garmpis N, Garmpi A, Savvanis S, Diamantis E. PCSK9 Inhibitors and cardiovascular disease: impact on cardiovascular outcomes. Curr Drug Discov Technol. (2020) 17(2):138–46. doi: 10.2174/1570163816666181211112358
19. Sobati S, Shakouri A, Edalati M, Mohammadnejad D, Parvan R, Masoumi J, et al. PCSK9: a key target for the treatment of cardiovascular disease (CVD). Adv Pharm Bull. (2020) 10(4):502–11. doi: 10.34172/apb.2020.062
20. Unni SK, Quek RGW, Biskupiak J, Lee VC, Ye X, Gandra SR. Assessment of statin therapy, LDL-C levels, and cardiovascular events among high-risk patients in the United States. J Clin Lipidol. (2016) 10(1):63–71. e3. doi: 10.1016/j.jacl.2015.09.008
21. Danchin N, Almahmeed W, Al-Rasadi K, Azuri J, Berrah A, Cuneo CA, et al. Achievement of low-density lipoprotein cholesterol goals in 18 countries outside Western Europe: the international ChoLesterol management practice study (ICLPS). Eur J Prev Cardiol. (2018) 25(10):1087–94. doi: 10.1177/2047487318777079
22. García-Gil M, Blanch J, Comas-Cufí M, Daunis-i-Estadella J, Bolíbar B, Martí R, et al. Patterns of statin use and cholesterol goal attainment in a high-risk cardiovascular population: a retrospective study of primary care electronic medical records. J Clin Lipidol. (2016) 10(1):134–42. doi: 10.1016/j.jacl.2015.10.007
23. Raggi P, Genest J, Giles JT, Rayner KJ, Dwivedi G, Beanlands RS, et al. Role of inflammation in the pathogenesis of atherosclerosis and therapeutic interventions. Atherosclerosis. (2018) 276:98–108. doi: 10.1016/j.atherosclerosis.2018.07.014
24. Xiang D, Li Y, Cao Y, Huang Y, Zhou L, Lin X, et al. Different effects of endothelial extracellular vesicles and LPS-induced endothelial extracellular vesicles on vascular smooth muscle cells: role of curcumin and its derivatives. Front Cardiovasc Med. (2021) 8:649352. Available at: doi: 10.3389/fcvm.2021.649352
25. Njock MS, Cheng HS, Dang LT, Nazari-Jahantigh M, Lau AC, Boudreau E, et al. Endothelial cells suppress monocyte activation through secretion of extracellular vesicles containing antiinflammatory microRNAs. Blood. (2015) 125(20):3202–12. doi: 10.1182/blood-2014-11-611046
26. Jansen F, Stumpf T, Proebsting S, Franklin BS, Wenzel D, Pfeifer P, et al. Intercellular transfer of miR-126-3p by endothelial microparticles reduces vascular smooth muscle cell proliferation and limits neointima formation by inhibiting LRP6. J Mol Cell Cardiol. (2017) 104:43–52. doi: 10.1016/j.yjmcc.2016.12.005
27. Gustafson D, Veitch S, Fish JE. Extracellular vesicles as protagonists of diabetic cardiovascular pathology. Front Cardiovasc Med. (2017) 4:71. doi: 10.3389/fcvm.2017.00071
28. van Niel G, Carter DRF, Clayton A, Lambert DW, Raposo G, Vader P. Challenges and directions in studying cell-cell communication by extracellular vesicles. Nat Rev Mol Cell Biol. (2022) 23(5):369–82. doi: 10.1038/s41580-022-00460-3
29. Milasan A, Tessandier N, Tan S, Brisson A, Boilard E, Martel C. Extracellular vesicles are present in mouse lymph and their level differs in atherosclerosis. J Extracell Vesicles. (2016) 5:31427. doi: 10.3402/jev.v5.31427
30. Mulcahy LA, Pink RC, Carter DRF. Routes and mechanisms of extracellular vesicle uptake. J Extracell Vesicles. (2014) 3:10.3402/jev.v3.24641. doi: 10.3402/jev.v3.24641
31. Théry C, Witwer KW, Aikawa E, Alcaraz MJ, Anderson JD, Andriantsitohaina R, et al. Minimal information for studies of extracellular vesicles 2018 (MISEV2018): a position statement of the international society for extracellular vesicles and update of the MISEV2014 guidelines. J Extracell Vesicles. (2018) 7(1):1535750. doi: 10.1080/20013078.2018.1535750
32. Brás IC, Khani MH, Riedel D, Parfentev I, Gerhardt E, van RC, et al. Ectosomes and exosomes are distinct proteomic entities that modulate spontaneous activity in neuronal cells. bioRxiv. (2021):2021.06.24.449731. doi: 10.1101/2021.06.24.449731v1
33. Kim JH, Lee CH, Baek MC. Dissecting exosome inhibitors: therapeutic insights into small-molecule chemicals against cancer. Exp Mol Med. (2022) 54(11):1833–43. doi: 10.1038/s12276-022-00898-7
34. Catalano M, O’Driscoll L. Inhibiting extracellular vesicles formation and release: a review of EV inhibitors. J Extracell Vesicles. (2020) 9(1):1703244. doi: 10.1080/20013078.2019.1703244
35. Bongiovanni L, Andriessen A, Wauben MHM, Nolte-’t Hoen ENM, de Bruin A. Extracellular vesicles: novel opportunities to understand and detect neoplastic diseases. Vet Pathol. (2021) 58(3):453–71. doi: 10.1177/0300985821999328
36. Santos JC, Lima NdS, Sarian LO, Matheu A, Ribeiro ML, Derchain SFM. Exosome-mediated breast cancer chemoresistance via miR-155 transfer. Sci Rep. (2018) 8(1):829. doi: 10.1038/s41598-018-19339-5
37. Felicetti F, De Feo A, Coscia C, Puglisi R, Pedini F, Pasquini L, et al. Exosome-mediated transfer of miR-222 is sufficient to increase tumor malignancy in melanoma. J Transl Med. (2016) 14(56):1–15. doi: 10.1186/s12967-016-0811-2
38. Sánchez CA, Andahur EI, Valenzuela R, Castellón EA, Fullá JA, Ramos CG, et al. Exosomes from bulk and stem cells from human prostate cancer have a differential microRNA content that contributes cooperatively over local and pre-metastatic niche. Oncotarget. (2016) 7(4):3993–4008. doi: 10.18632/oncotarget.6540
39. Akhmerov A, Parimon T. Extracellular vesicles, inflammation, and cardiovascular disease. Cells. (2022) 11(14):2229. doi: 10.3390/cells11142229
40. Buffolo F, Monticone S, Camussi G, Aikawa E. Role of extracellular vesicles in the pathogenesis of vascular damage. Hypertension. (2022) 79(5):863–73. doi: 10.1161/HYPERTENSIONAHA.121.17957
41. Huber J, Vales A, Mitulovic G, Blumer M, Schmid R, Witztum JL, et al. Oxidized membrane vesicles and blebs from apoptotic cells contain biologically active oxidized phospholipids that induce monocyte-endothelial interactions. Arterioscler Thromb Vasc Biol. (2002) 22(1):101–7. doi: 10.1161/hq0102.101525
42. Hill AF. Extracellular vesicles and neurodegenerative diseases. J Neurosci Off J Soc Neurosci. (2019) 39(47):9269–73. doi: 10.1523/JNEUROSCI.0147-18.2019
43. Cervenakova L, Saá P, Yakovleva O, Vasilyeva I, de Castro J, Brown P, et al. Are prions transported by plasma exosomes? Transfus Apher Sci Off J World Apher Assoc Off J Eur Soc Haemapheresis. (2016) 55(1):70–83. doi: 10.1016/j.transci.2016.07.013
44. Saá P, Yakovleva O, de Castro J, Vasilyeva I, De Paoli SH, Simak J, et al. First demonstration of transmissible spongiform encephalopathy-associated prion protein (PrPTSE) in extracellular vesicles from plasma of mice infected with mouse-adapted variant creutzfeldt-jakob disease by in vitro amplification. J Biol Chem. (2014) 289(42):29247–60. doi: 10.1074/jbc.M114.589564
45. Cheng L, Doecke JD, Sharples RA, Villemagne VL, Fowler CJ, Rembach A, et al. Prognostic serum miRNA biomarkers associated with Alzheimer’s disease shows concordance with neuropsychological and neuroimaging assessment. Mol Psychiatry. (2015) 20(10):1188–96. doi: 10.1038/mp.2014.127
46. Chong SY, Lee CK, Huang C, Ou YH, Charles CJ, Richards AM, et al. Extracellular vesicles in cardiovascular diseases: alternative biomarker sources, therapeutic agents, and drug delivery carriers. Int J Mol Sci. (2019) 20(13):3272. doi: 10.3390/ijms20133272
47. Martin-Ventura JL, Roncal C, Orbe J, Blanco-Colio LM. Role of extracellular vesicles as potential diagnostic and/or therapeutic biomarkers in chronic cardiovascular diseases. Front Cell Dev Biol. (2022) 10:81. doi: 10.3389/fcell.2022.813885
48. Elsharkasy OM, Nordin JZ, Hagey DW, de Jong OG, Schiffelers RM, Andaloussi SE, et al. Extracellular vesicles as drug delivery systems: why and how? Adv Drug Deliv Rev. (2020) 159:332–43. doi: 10.1016/j.addr.2020.04.004
49. Herrmann IK, Wood MJA, Fuhrmann G. Extracellular vesicles as a next-generation drug delivery platform. Nat Nanotechnol. (2021) 16(7):748–59. doi: 10.1038/s41565-021-00931-2
50. de Jong OG, Kooijmans SAA, Murphy DE, Jiang L, Evers MJW, Sluijter JPG, et al. Drug delivery with extracellular vesicles: from imagination to innovation. Acc Chem Res. (2019) 52(7):1761–70. doi: 10.1021/acs.accounts.9b00109
51. Zhang F, Guo J, Zhang Z, Duan M, Wang G, Qian Y, et al. Application of engineered extracellular vesicles for targeted tumor therapy. J Biomed Sci. (2022) 29(1):14. doi: 10.1186/s12929-022-00798-y
52. Niu W, Xiao Q, Wang X, Zhu J, Li J, Liang X, et al. A biomimetic drug delivery system by integrating grapefruit extracellular vesicles and doxorubicin-loaded heparin-based nanoparticles for glioma therapy. Nano Lett. (2021) 21(3):1484–92. doi: 10.1021/acs.nanolett.0c04753
53. Yong T, Zhang X, Bie N, Zhang H, Zhang X, Li F, et al. Tumor exosome-based nanoparticles are efficient drug carriers for chemotherapy. Nat Commun. (2019) 10(1):3838. doi: 10.1038/s41467-019-11718-4
54. Zhang J, Yuan ZF, Wang Y, Chen WH, Luo GF, Cheng SX, et al. Multifunctional envelope-type mesoporous silica nanoparticles for tumor-triggered targeting drug delivery. J Am Chem Soc. (2013) 135(13):5068–73. doi: 10.1021/ja312004m
55. Murphy DE, de Jong OG, Brouwer M, Wood MJ, Lavieu G, Schiffelers RM, et al. Extracellular vesicle-based therapeutics: natural versus engineered targeting and trafficking. Exp Mol Med. (2019) 51(3):1–12. doi: 10.1038/s12276-019-0223-5
56. Li M, Fang F, Sun M, Zhang Y, Hu M, Zhang J. Extracellular vesicles as bioactive nanotherapeutics: an emerging paradigm for regenerative medicine. Theranostics. (2022) 12(11):4879–903. doi: 10.7150/thno.72812
57. De Jong OG, Van Balkom BWM, Schiffelers RM, Bouten CVC, Verhaar MC. Extracellular vesicles: potential roles in regenerative medicine. Front Immunol. (2014) 5:608. doi: 10.3389/fimmu.2014.00608
58. Li Jw, Wei L, Han Z, Chen Z. Mesenchymal stromal cells-derived exosomes alleviate ischemia/reperfusion injury in mouse lung by transporting anti-apoptotic miR-21-5p. Eur J Pharmacol. (2019 ) 852:68–76. doi: 10.1016/j.ejphar.2019.01.022
59. Rad LM, Yumashev AV, Hussen BM, Jamad HH, Ghafouri-Fard S, Taheri M, et al. Therapeutic potential of microvesicles in cell therapy and regenerative medicine of ocular diseases with an especial focus on mesenchymal stem cells-derived microvesicles. Front Genet. (2022) 13:570. dodoi: 10.3389/fgene.2022.847679
60. Dickhout A, Koenen RR. Extracellular vesicles as biomarkers in cardiovascular disease; chances and risks. Front Cardiovasc Med. (2018) 5:113. doi: 10.3389/fcvm.2018.00113
61. Takov K, Yellon DM, Davidson SM. Comparison of small extracellular vesicles isolated from plasma by ultracentrifugation or size-exclusion chromatography: yield, purity and functional potential. J Extracell Vesicles. (2019) 8(1):1560809. doi: 10.1080/20013078.2018.1560809
62. Mol EA, Goumans MJ, Doevendans PA, Sluijter JPG, Vader P. Higher functionality of extracellular vesicles isolated using size-exclusion chromatography compared to ultracentrifugation. Nanomedicine Nanotechnol Biol Med. (2017) 13(6):2061–5. doi: 10.1016/j.nano.2017.03.011
63. van der Pol E, Coumans FaW, Grootemaat AE, Gardiner C, Sargent IL, Harrison P, et al. Particle size distribution of exosomes and microvesicles determined by transmission electron microscopy, flow cytometry, nanoparticle tracking analysis, and resistive pulse sensing. J Thromb Haemost JTH. (2014) 12(7):1182–92. doi: 10.1111/jth.12602
64. Karimi N, Cvjetkovic A, Jang SC, Crescitelli R, Hosseinpour Feizi MA, Nieuwland R, et al. Detailed analysis of the plasma extracellular vesicle proteome after separation from lipoproteins. Cell Mol Life Sci CMLS. (2018) 75(15):2873–86. doi: 10.1007/s00018-018-2773-4
65. Bernal-Mizrachi L, Jy W, Jimenez JJ, Pastor J, Mauro LM, Horstman LL, et al. High levels of circulating endothelial microparticles in patients with acute coronary syndromes. Am Heart J. (2003) 145(6):962–70. doi: 10.1016/S0002-8703(03)00103-0
66. Chiva-Blanch G, Bratseth V, Ritschel V, Andersen GØ, Halvorsen S, Eritsland J, et al. Monocyte-derived circulating microparticles (CD14+, CD14+/CD11b+ and CD14+/CD142+) are related to long-term prognosis for cardiovascular mortality in STEMI patients. Int J Cardiol. (2017) 227:876–81. doi: 10.1016/j.ijcard.2016.11.302
67. Bandu R, Oh JW, Kim KP. Mass spectrometry-based proteome profiling of extracellular vesicles and their roles in cancer biology. Exp Mol Med. (2019 51(3):1–10. doi: 10.1038/s12276-019-0218-2
68. Schey KL, Luther JM, Rose KL. Proteomics characterization of exosome cargo. Methods San Diego Calif. (2015) 87:75–82. doi: 10.1016/j.ymeth.2015.03.018
69. Turchinovich A, Drapkina O, Tonevitsky A. Transcriptome of extracellular vesicles: state-of-the-art. Front Immunol. (2019) 10:202. doi: 10.3389/fimmu.2019.00202
70. Nozaki T, Sugiyama S, Koga H, Sugamura K, Ohba K, Matsuzawa Y, et al. Significance of a multiple biomarkers strategy including endothelial dysfunction to improve risk stratification for cardiovascular events in patients at high risk for coronary heart disease. J Am Coll Cardiol. (2009) 54(7):601–8. doi: 10.1016/j.jacc.2009.05.022
71. Bayés-Genis A, Lanfear DE, de Ronde MWJ, Lupón J, Leenders JJ, Liu Z, et al. Prognostic value of circulating microRNAs on heart failure-related morbidity and mortality in two large diverse cohorts of general heart failure patients. Eur J Heart Fail. (2018) 20(1):67–75. doi: 10.1002/ejhf.984
72. van der Velde AR, Gullestad L, Ueland T, Aukrust P, Guo Y, Adourian A, et al. Prognostic value of changes in galectin-3 levels over time in patients with heart failure: data from CORONA and COACH. Circulation: Heart Failure. (2013) 6(2):219–26. doi: 10.1161/CIRCHEARTFAILURE.112.000129
73. Zhang X, Wan Y, Chata R, Brazzale A, Atherton JJ, Kostner K, et al. A pilot study to demonstrate diagnostic potential of galectin-3 levels in saliva. J Clin Pathol. (2016 69(12):1100–4. doi: 10.1136/jclinpath-2016-203631
74. Wang L, Liu J, Xu B, Liu YL, Liu Z. Reduced exosome miR-425 and miR-744 in the plasma represents the progression of fibrosis and heart failure. Kaohsiung J Med Sci. (2018v) 34(11):626–33. doi: 10.1016/j.kjms.2018.05.008
75. Wu T, Chen Y, Du Y, Tao J, Li W, Zhou Z, et al. Circulating exosomal miR-92b-5p is a promising diagnostic biomarker of heart failure with reduced ejection fraction patients hospitalized for acute heart failure. J Thorac Dis. (2018) 10(11):6211–20. doi: 10.21037/jtd.2018.10.52
76. Widera C, Gupta SK, Lorenzen JM, Bang C, Bauersachs J, Bethmann K, et al. Diagnostic and prognostic impact of six circulating microRNAs in acute coronary syndrome. J Mol Cell Cardiol. (2011) 51(5):872–5. doi: 10.1016/j.yjmcc.2011.07.011
77. de Hoog VC, Timmers L, Schoneveld AH, Wang JW, van de Weg SM, Sze SK, et al. Serum extracellular vesicle protein levels are associated with acute coronary syndrome. Eur Heart J Acute Cardiovasc Care. (2013) 2(1):53–60. doi: 10.1177/2048872612471212
78. von zur Muhlen C, Schiffer E, Zuerbig P, Kellmann M, Brasse M, Meert N, et al. Evaluation of urine proteome pattern analysis for its potential to reflect coronary artery atherosclerosis in symptomatic patients. J Proteome Res. (2009) 8(1):335–45. doi: 10.1021/pr800615t
79. Jansen F, Yang X, Proebsting S, Hoelscher M, Przybilla D, Baumann K, et al. MicroRNA expression in circulating microvesicles predicts cardiovascular events in patients with coronary artery disease. J Am Heart Assoc. (2014) 3(6):e001249. doi: 10.1161/JAHA.114.001249
80. Kanhai DA, Visseren FLJ, van der Graaf Y, Schoneveld AH, Catanzariti LM, Timmers L, et al. Microvesicle protein levels are associated with increased risk for future vascular events and mortality in patients with clinically manifest vascular disease. Int J Cardiol. (2013) 168(3):2358–63. doi: 10.1016/j.ijcard.2013.01.231
81. Gidlöf O, Smith JG, Miyazu K, Gilje P, Spencer A, Blomquist S, et al. Circulating cardio-enriched microRNAs are associated with long-term prognosis following myocardial infarction. BMC Cardiovasc Disord. (2013) 13:12. doi: 10.1186/1471-2261-13-12
82. Matsumoto S, Sakata Y, Suna S, Nakatani D, Usami M, Hara M, et al. Circulating p53-responsive MicroRNAs are predictive indicators of heart failure after acute myocardial infarction. Circ Res. (2013) 113(3):322–6. doi: 10.1161/CIRCRESAHA.113.301209
83. Núñez E, Fuster V, Gómez-Serrano M, Valdivielso JM, Fernández-Alvira JM, Martínez-López D, et al. Unbiased plasma proteomics discovery of biomarkers for improved detection of subclinical atherosclerosis. EBioMedicine. (2022) 76(103874):103874. doi: 10.1016/j.ebiom.2022.103874
84. Amabile N, Rautou PE, Tedgui A, Boulanger CM. Microparticles: key protagonists in cardiovascular disorders. Semin Thromb Hemost. (2010) 36(8):907–16. doi: 10.1055/s-0030-1267044
85. Dickhout A, Koenen RR. Extracellular vesicles as biomarkers in cardiovascular disease; chances and Risks. Front Cardiovasc Med. (2018) 5:113. doi: 10.3389/fcvm.2018.00113
86. Xiao Y, Zheng L, Zou X, Wang J, Zhong J, Zhong T. Extracellular vesicles in type 2 diabetes mellitus: key roles in pathogenesis, complications, and therapy. J Extracell Vesicles. (2019) 8(1):1625677. doi: 10.1080/20013078.2019.1625677
87. Huang C, Fisher KP, Hammer SS, Navitskaya S, Blanchard GJ, Busik JV. Plasma exosomes contribute to microvascular damage in diabetic retinopathy by activating the classical complement pathway. Diabetes. (2018) 67(8):1639–49. doi: 10.2337/db17-1587
88. Guo SC, Tao SC, Yin WJ, Qi X, Yuan T, Zhang CQ. Exosomes derived from platelet-rich plasma promote the re-epithelization of chronic cutaneous wounds via activation of YAP in a diabetic rat model. Theranostics. (2017) 7(1):81–96. doi: 10.7150/thno.16803
89. Hwang HS, Kim H, Han G, Lee JW, Kim K, Kwon IC, et al. Extracellular vesicles as potential therapeutics for inflammatory diseases. Int J Mol Sci. (2021) 22(11):5487. doi: 10.3390/ijms22115487
90. Pasquali L, Svedbom A, Srivastava A, Rosén E, Lindqvist U, Ståhle M, et al. Circulating microRNAs in extracellular vesicles as potential biomarkers for psoriatic arthritis in patients with psoriasis. J Eur Acad Dermatol Venereol JEADV. (2020) 34(6):1248–56. doi: 10.1111/jdv.16203
91. Wang ZY, Yan BX, Zhou Y, Chen XY, Zhang J, Cai SQ, et al. miRNA profiling of extracellular vesicles reveals biomarkers for psoriasis. J Invest Dermatol. (2021) 141(1):185–9. e4. doi: 10.1016/j.jid.2020.04.021
92. Suades R, Greco MF, Padró T, Badimon L. Extracellular vesicles as drivers of immunoinflammation in atherothrombosis. Cells. (2022) 11(11):1845. doi: 10.3390/cells11111845
93. Badimon L, Suades R, Arderiu G, Peña E, Chiva-Blanch G, Padró T. Microvesicles in atherosclerosis and angiogenesis: from bench to bedside and reverse. Front Cardiovasc Med. (2017) 4:77. doi: 10.3389/fcvm.2017.00077
94. Suades R, Padró T, Crespo J, Sionis A, Alonso R, Mata P, et al. Liquid biopsy of extracellular microvesicles predicts future Major ischemic events in genetically characterized familial hypercholesterolemia patients. Arterioscler Thromb Vasc Biol. (2019) 39(6):1172–81. doi: 10.1161/ATVBAHA.119.312420
95. Chironi G, Simon A, Hugel B, Del Pino M, Gariepy J, Freyssinet JM, et al. Circulating leukocyte-derived microparticles predict subclinical atherosclerosis burden in asymptomatic subjects. Arterioscler Thromb Vasc Biol. (2006) 26(12):2775–80. doi: 10.1161/01.ATV.0000249639.36915.04
96. Blood Task Force. (cited 2023 Mar 24). Available at: https://www.isev.org/blood-task-force
97. Badimon L, Padro T, Arderiu G, Vilahur G, Borrell-Pages M, Suades R. Extracellular vesicles in atherothrombosis: from biomarkers and precision medicine to therapeutic targets. Immunol Rev. (2022) 312(1):6–19. doi: 10.1111/imr.13127
98. Yang J, Shin TS, Kim JS, Jee YK, Kim YK. A new horizon of precision medicine: combination of the microbiome and extracellular vesicles. Exp Mol Med. (2022) 54(4):466–82. doi: 10.1038/s12276-022-00748-6
99. Sluijter JPG, Davidson SM, Boulanger CM, Buzás EI, de Kleijn DPV, Engel FB, et al. Extracellular vesicles in diagnostics and therapy of the ischaemic heart: position paper from the working group on cellular biology of the heart of the European society of cardiology. Cardiovasc Res. (2018) 114(1):19–34. doi: 10.1093/cvr/cvx211
100. Bobryshev YV, Killingsworth MC, Orekhov AN. Increased shedding of microvesicles from intimal smooth muscle cells in athero-prone areas of the human aorta: implications for understanding of the predisease stage. Pathobiology. (2013) 80(1):24–31. doi: 10.1159/000339430
101. Leroyer AS, Isobe H, Lesèche G, Castier Y, Wassef M, Mallat Z, et al. Cellular origins and thrombogenic activity of microparticles isolated from human atherosclerotic plaques. J Am Coll Cardiol. (2007) 49(7):772–7. doi: 10.1016/j.jacc.2006.10.053
102. Perrotta I, Aquila S. Exosomes in human atherosclerosis: an ultrastructural analysis study. Ultrastruct Pathol. (2016) 40(2):101–6. doi: 10.3109/01913123.2016.1154912
103. Goetzl EJ, Schwartz JB, Mustapic M, Lobach IV, Daneman R, Abner EL, et al. Altered cargo proteins of human plasma endothelial cell–derived exosomes in atherosclerotic cerebrovascular disease. FASEB J. (2017) 31(8):3689–94. doi: 10.1096/fj.201700149
104. Georgescu A, Simionescu M. Extracellular vesicles: versatile nanomediators, potential biomarkers and therapeutic agents in atherosclerosis and COVID-19-related thrombosis. Int J Mol Sci. (2021) 22(11):5967. doi: 10.3390/ijms22115967
105. Mayr M, Grainger D, Mayr U, Leroyer AS, Leseche G, Sidibe A, et al. Proteomics, metabolomics, and immunomics on microparticles derived from human atherosclerotic plaques. Circ Cardiovasc Genet. (2009) 2(4):379–88. doi: 10.1161/CIRCGENETICS.108.842849
106. Brodsky SV, Zhang F, Nasjletti A, Goligorsky MS. Endothelium-derived microparticles impair endothelial function in vitro. Am J Physiol Heart Circ Physiol. (2004) 286(5):H1910–1915. doi: 10.1152/ajpheart.01172.2003
107. Densmore JC, Signorino PR, Ou J, Hatoum OA, Rowe JJ, Shi Y, et al. Endothelium-derived microparticles induce endothelial dysfunction and acute lung injury. Shock. (2006) 26(5):464. doi: 10.1097/01.shk.0000228791.10550.36
108. Jansen F, Yang X, Franklin BS, Hoelscher M, Schmitz T, Bedorf J, et al. High glucose condition increases NADPH oxidase activity in endothelial microparticles that promote vascular inflammation. Cardiovasc Res. (2013) 98(1):94–106. doi: 10.1093/cvr/cvt013
109. He S, Wu C, Xiao J, Li D, Sun Z, Li M. Endothelial extracellular vesicles modulate the macrophage phenotype: potential implications in atherosclerosis. Scand J Immunol. (2018) 87(4):e12648. doi: 10.1111/sji.12648
110. Chang YJ, Li YS, Wu CC, Wang KC, Huang TC, Chen Z, et al. Extracellular MicroRNA-92a mediates endothelial cell-macrophage communication. Arterioscler Thromb Vasc Biol. (2019) 39(12):2492–504. doi: 10.1161/ATVBAHA.119.312707
111. Zheng B, Yin Wn, Suzuki T, Zhang Xh, Zhang Y, Song Ll, et al. Exosome-Mediated miR-155 transfer from smooth muscle cells to endothelial cells induces endothelial injury and promotes atherosclerosis. Mol Ther. (2017) 25(6):1279–94. doi: 10.1016/j.ymthe.2017.03.031
112. Hergenreider E, Heydt S, Tréguer K, Boettger T, Horrevoets AJG, Zeiher AM, et al. Atheroprotective communication between endothelial cells and smooth muscle cells through miRNAs. Nat Cell Biol. (2012) 14(3):249–56. doi: 10.1038/ncb2441
113. Zhao Y, Li Y, Luo P, Gao Y, Yang J, Lao KH, et al. XBP1 Splicing triggers miR-150 transfer from smooth muscle cells to endothelial cells via extracellular vesicles. Sci Rep. (2016) 6(1):28627. doi: 10.1038/srep28627
114. Tang N, Sun B, Gupta A, Rempel H, Pulliam L. Monocyte exosomes induce adhesion molecules and cytokines via activation of NF-κB in endothelial cells. FASEB J Off Publ Fed Am Soc Exp Biol. (2016) 30(9):3097–106. doi: 10.1096/fj.201600368RR
115. Aharon A, Tamari T, Brenner B. Monocyte-derived microparticles and exosomes induce procoagulant and apoptotic effects on endothelial cells. Thromb Haemost. (2008) 100(5):878–85. doi: 10.1160/TH07-11-0691
116. Wang JG, Williams JC, Davis BK, Jacobson K, Doerschuk CM, Ting JPY, et al. Monocytic microparticles activate endothelial cells in an IL-1β-dependent manner. Blood. (2011) 118(8):2366–74. doi: 10.1182/blood-2011-01-330878
117. Niu C, Wang X, Zhao M, Cai T, Liu P, Li J, et al. Macrophage foam cell–derived extracellular vesicles promote vascular smooth muscle cell migration and adhesion. J Am Heart Assoc Cardiovasc Cerebrovasc Dis. (2016) 5(10):e004099. doi: 10.1161/JAHA.116.004099
118. Distler JHW, Huber LC, Hueber AJ, Reich CF, Gay S, Distler O, et al. The release of microparticles by apoptotic cells and their effects on macrophages. Apoptosis Int J Program Cell Death. (2005) 10(4):731–41. doi: 10.1007/s10495-005-2941-5
119. Böing AN, Hau CM, Sturk A, Nieuwland R. Platelet microparticles contain active caspase 3. Platelets. (2008) 19(2):96–103. doi: 10.1080/09537100701777295
120. Abid Hussein MN, Nieuwland R, Hau CM, Evers LM, Meesters EW, Sturk A. Cell-derived microparticles contain caspase 3 in vitro and in vivo. J Thromb Haemost JTH. (2005) 3(5):888–96. doi: 10.1111/j.1538-7836.2005.01240.x
121. Pizzirani C, Ferrari D, Chiozzi P, Adinolfi E, Sandonà D, Savaglio E, et al. Stimulation of P2 receptors causes release of IL-1beta-loaded microvesicles from human dendritic cells. Blood. (2007) 109(9):3856–64. doi: 10.1182/blood-2005-06-031377
122. Sarkar A, Mitra S, Mehta S, Raices R, Wewers MD. Monocyte derived microvesicles deliver a cell death message via encapsulated caspase-1. PLOS ONE. (2009) 4(9):e7140. doi: 10.1371/journal.pone.0007140
123. Chatterjee V, Yang X, Ma Y, Cha B, Meegan JE, Wu M, et al. Endothelial microvesicles carrying src-rich cargo impair adherens junction integrity and cytoskeleton homeostasis. Cardiovasc Res. (2020) 116(8):1525–38. doi: 10.1093/cvr/cvz238
124. Mesri M, Altieri DC. Leukocyte microparticles stimulate endothelial cell cytokine release and tissue factor induction in a JNK1 signaling pathway. J Biol Chem. (1999) 274(33):23111–8. doi: 10.1074/jbc.274.33.23111
125. Robbins PD, Dorronsoro A, Booker CN. Regulation of chronic inflammatory and immune processes by extracellular vesicles. J Clin Invest. (2016) 126(4):1173–80. doi: 10.1172/JCI81131
126. Luquero A, Vilahur G, Crespo J, Badimon L, Borrell-Pages M. Microvesicles carrying LRP5 induce macrophage polarization to an anti-inflammatory phenotype. J Cell Mol Med. (2021) 25(16):7935–47. doi: 10.1111/jcmm.16723
127. Mause SF, von Hundelshausen P, Zernecke A, Koenen RR, Weber C. Platelet microparticles: a transcellular delivery system for RANTES promoting monocyte recruitment on endothelium. Arterioscler Thromb Vasc Biol. (2005) 25(7):1512–8. doi: 10.1161/01.ATV.0000170133.43608.37
128. Rautou PE, Leroyer AS, Ramkhelawon B, Devue C, Duflaut D, Vion AC, et al. Microparticles from human atherosclerotic plaques promote endothelial ICAM1-dependent monocyte adhesion and transendothelial migration. Circ Res. (2011) 108(3):335–43. doi: 10.1161/CIRCRESAHA.110.237420
129. Llorente-Cortés V, Otero-Viñas M, Camino-López S, Llampayas O, Badimon L. Aggregated low-density lipoprotein uptake induces membrane tissue factor procoagulant activity and microparticle release in human vascular smooth muscle cells. Circulation. (2004) 110(4):452–9. doi: 10.1161/01.CIR.0000136032.40666.3D
130. Vajen T, Benedikter BJ, Heinzmann ACA, Vasina EM, Henskens Y, Parsons M, et al. Platelet extracellular vesicles induce a pro-inflammatory smooth muscle cell phenotype. J Extracell Vesicles. (2017) 6(1):1322454. doi: 10.1080/20013078.2017.1322454
131. Furmanik M, Chatrou M, van Gorp R, Akbulut A, Willems B, Schmidt H, et al. Reactive oxygen-forming Nox5 links vascular smooth muscle cell phenotypic switching and extracellular vesicle-mediated vascular calcification. Circ Res. (2020) 127(7):911–27. doi: 10.1161/CIRCRESAHA.119.316159
132. Lozito TP, Tuan RS. Endothelial cell microparticles act as centers of matrix metalloproteinsase-2 (MMP-2) activation and vascular matrix remodeling. J Cell Physiol. (2012) 227(2):534–49. doi: 10.1002/jcp.22744
133. Vascotto SG, Beckham Y, Kelly GM. The zebrafish’s swim to fame as an experimental model in biology. Biochem Cell Biol. (1997) 75(5):479–85. doi: 10.1139/o97-081
134. Grunwald DJ, Eisen JS. Headwaters of the zebrafish — emergence of a new model vertebrate. Nat Rev Genet. (2002) 3(9):717–24. doi: 10.1038/nrg892
135. Scott A, Sueiro Ballesteros L, Bradshaw M (辻千里), Tsuji C, Power A, Lorriman J, et al. In vivo characterization of endogenous cardiovascular extracellular vesicles in larval and adult zebrafish. Arterioscler Thromb Vasc Biol. (2021) 41(9):2454–68. doi: 10.1161/ATVBAHA.121.316539
136. Verweij FJ, Revenu C, Arras G, Dingli F, Loew D, Pegtel DM, et al. Live tracking of inter-organ communication by endogenous exosomes in vivo. Dev Cell. (2019) 48(4):573–89. e4. doi: 10.1016/j.devcel.2019.01.004
137. McCann JV, Liu A, Musante L, Erdbrügger U, Lannigan J, Dudley AC. A miRNA signature in endothelial cell-derived extracellular vesicles in tumor-bearing mice. Sci Rep. (2019) 9(1):16743. doi: 10.1038/s41598-019-52466-1
138. Barlin M, Erdmann-Gilmore P, Mudd JL, Zhang Q, Seymour RW, Guo Z, et al. Proteins in tumor-derived plasma extracellular vesicles indicate tumor origin. Mol Cell Proteomics. (2023) 22(1):100476. Available at: https://www.mcponline.org/article/S1535-9476(22)00284-5/abstract doi: 10.1016/j.mcpro.2022.100476
139. Delgado-Peraza F, Nogueras-Ortiz CJ, Volpert O, Liu D, Goetzl EJ, Mattson MP, et al. Neuronal and astrocytic extracellular vesicle biomarkers in blood reflect brain pathology in mouse models of Alzheimer’s disease. Cells. (2021) 10(5):993. doi: 10.3390/cells10050993
140. Willis CM, Nicaise AM, Menoret A, Ryu JK, Mendiola AS, Jellison ER, et al. Extracellular vesicle fibrinogen induces encephalitogenic CD8+ T cells in a mouse model of multiple sclerosis. Proc Natl Acad Sci. (2019) 116(21):10488–93. doi: 10.1073/pnas.1816911116
141. Casella G, Colombo F, Finardi A, Descamps H, Ill-Raga G, Spinelli A, et al. Extracellular vesicles containing IL-4 modulate neuroinflammation in a mouse model of multiple sclerosis. Mol Ther. (2018) 26(9):2107–18. doi: 10.1016/j.ymthe.2018.06.024
142. Yang S, Liu P, Gao T, Song D, Zhao X, Li Y, et al. Every road leads to Rome: therapeutic effect and mechanism of the extracellular vesicles of human embryonic stem cell-derived immune and matrix regulatory cells administered to mouse models of pulmonary fibrosis through different routes. Stem Cell Res Ther. (2022) 13(1):163. doi: 10.1186/s13287-022-02839-7
143. Mohammed I, Ijaz S, Mokhtari T, Gholaminejhad M, Mahdavipour M, Jameie B, et al. Subventricular zone-derived extracellular vesicles promote functional recovery in rat model of spinal cord injury by inhibition of NLRP3 inflammasome complex formation. Metab Brain Dis. (2020) 35(5):809–18. doi: 10.1007/s11011-020-00563-w
144. Romanelli P, Bieler L, Heimel P, Škokić S, Jakubecova D, Kreutzer C, et al. Enhancing functional recovery through intralesional application of extracellular vesicles in a rat model of traumatic spinal cord injury. Front Cell Neurosci. (2022) 15:795008. doi: 10.3389/fncel.2021.795008
145. Gómez-Molina C, Sandoval M, Henzi R, Ramírez JP, Varas-Godoy M, Luarte A, et al. Small extracellular vesicles in rat Serum contain astrocyte-derived protein biomarkers of repetitive stress. Int J Neuropsychopharmacol. (2019) 22(3):232–46. doi: 10.1093/ijnp/pyy098
146. Guy R, Herman S, Benyamini H, Ben-Zur T, Kobo H, Pasmanik-Chor M, et al. Mesenchymal stem cell-derived extracellular vesicles as proposed therapy in a rat model of cerebral small vessel disease. Int J Mol Sci. (2022) 23(19):11211. doi: 10.3390/ijms231911211
147. Berger A, Araújo-Filho I, Piffoux M, Nicolás-Boluda A, Grangier A, Boucenna I, et al. Local administration of stem cell-derived extracellular vesicles in a thermoresponsive hydrogel promotes a pro-healing effect in a rat model of colo-cutaneous post-surgical fistula. Nanoscale. (2021) 13(1):218–32. doi: 10.1039/D0NR07349K
148. Khalaj K, Figueira RL, Antounians L, Gandhi S, Wales M, Montalva L, et al. Treatment with amniotic fluid stem cell extracellular vesicles promotes fetal lung branching and cell differentiation at canalicular and saccular stages in experimental pulmonary hypoplasia secondary to congenital diaphragmatic hernia. Stem Cells Transl Med. (2022) 11(10):1089–102. doi: 10.1093/stcltm/szac063
149. Ural EE, Toomajian V, Hoque Apu E, Veletic M, Balasingham I, Ashammakhi N, et al. Visualizing extracellular vesicles and their function in 3D tumor microenvironment models. Int J Mol Sci. (2021) 22(9):4784. doi: 10.3390/ijms22094784
150. Perez GI, Broadbent D, Zarea AA, Dolgikh B, Bernard MP, Withrow A, et al. In vitro and in vivo analysis of extracellular vesicle-mediated metastasis using a bright, red-shifted bioluminescent reporter protein. Adv Genet. (2022) 3(1):2100055. doi: 10.1002/ggn2.202100055
151. Ye Y, Shi Q, Yang T, Xie F, Zhang X, Xu B, et al. In vivo visualized tracking of tumor-derived extracellular vesicles using CRISPR-Cas9 system. Technol Cancer Res Treat. (2022) 21:15330338221085370. doi: 10.1177/15330338221085370
152. Nørgård MØ, Steffensen LB, Hansen DR, Füchtbauer EM, Engelund MB, Dimke H, et al. A new transgene mouse model using an extravesicular EGFP tag enables affinity isolation of cell-specific extracellular vesicles. Sci Rep. (2022) 12(1):496. doi: 10.1038/s41598-021-04512-0
153. McCann JV, Bischoff SR, Zhang Y, Cowley DO, Sanchez-Gonzalez V, Daaboul GD, et al. Reporter mice for isolating and auditing cell type-specific extracellular vesicles in vivo. Genes N Y N. (2020) 58(7):e23369. doi: 10.1038/s41598-021-04512-0
154. Li W, Wang J, Yin X, Shi H, Sun B, Ji M, et al. Construction of a mouse model that can be used for tissue-specific EV screening and tracing in vivo. Front Cell Dev Biol. (2022) 10:2279. doi: 10.3389/fcell.2022.1015841
155. Yoshimura A, Kawamata M, Yoshioka Y, Katsuda T, Kikuchi H, Nagai Y, et al. Generation of a novel transgenic rat model for tracing extracellular vesicles in body fluids. Sci Rep. (2016) 6(1):31172. doi: 10.1038/srep31172
156. Angelillo-Scherrer A, Weber C, Mause S. Leukocyte-Derived microparticles in vascular homeostasis. Circ Res. (2012) 110(2):356–69. doi: 10.1161/CIRCRESAHA.110.233403
157. Sarlon-Bartoli G, Bennis Y, Lacroix R, Piercecchi-Marti MD, Bartoli MA, Arnaud L, et al. Plasmatic level of leukocyte-derived microparticles is associated with unstable plaque in asymptomatic patients with high-grade carotid stenosis. J Am Coll Cardiol. (2013) 62(16):1436–41. doi: 10.1016/j.jacc.2013.03.078
158. Chen W, Schilperoort M, Cao Y, Shi J, Tabas I, Tao W. Macrophage-targeted nanomedicine for the diagnosis and treatment of atherosclerosis. Nat Rev Cardiol. (2022) 19(4):228–49. doi: 10.1038/s41569-021-00629-x
Keywords: extracellular vesicles, atherosclerosis, biomarkers, therapeutics, EV tracking models
Citation: Patel S, Guo MK, Abdul Samad M and Howe KL (2023) Extracellular vesicles as biomarkers and modulators of atherosclerosis pathogenesis. Front. Cardiovasc. Med. 10:1202187. doi: 10.3389/fcvm.2023.1202187
Received: 7 April 2023; Accepted: 20 April 2023;
Published: 26 May 2023.
Edited by:
Louis Maximilian Buja, University of Texas Health Science Center at Houston, United StatesReviewed by:
Jon Homeister, University of North Carolina at Chapel Hill, United States© 2023 Patel, Guo, Abdul Samad and Howe. This is an open-access article distributed under the terms of the Creative Commons Attribution License (CC BY). The use, distribution or reproduction in other forums is permitted, provided the original author(s) and the copyright owner(s) are credited and that the original publication in this journal is cited, in accordance with accepted academic practice. No use, distribution or reproduction is permitted which does not comply with these terms.
*Correspondence: Kathryn L. Howe a2F0aHJ5bi5ob3dlQHVobi5jYQ==