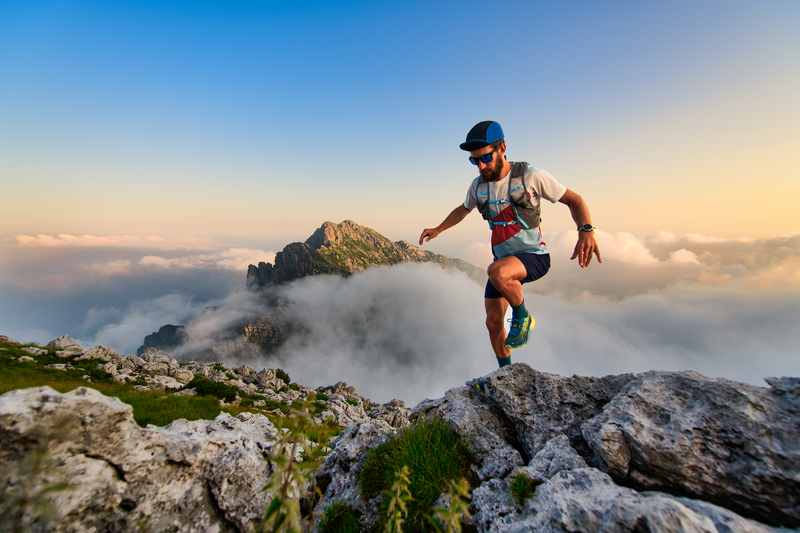
95% of researchers rate our articles as excellent or good
Learn more about the work of our research integrity team to safeguard the quality of each article we publish.
Find out more
MINI REVIEW article
Front. Cardiovasc. Med. , 26 May 2023
Sec. Cardiovascular Genetics and Systems Medicine
Volume 10 - 2023 | https://doi.org/10.3389/fcvm.2023.1151717
This article is part of the Research Topic Genomic Architecture of Cardiovascular Diseases, Volume II View all 6 articles
Most blood vessels are surrounded by adipose tissues known as perivascular adipose tissue (PVAT). Emerging experimental data have implicated the potential involvement of PVAT in the pathogenesis of cardiovascular disease: PVAT might be a source of inflammatory mediators under pathological conditions such as metabolic disorders, chronic inflammation, and aging, leading to vascular pathologies, while having vasculo-protective roles in a healthy state. PVAT has been also gaining attention in human disease conditions. Recent integrative omics approaches have greatly enhanced our understanding of the molecular mechanisms underlying the diverse functions of PVAT. This review summarizes recent progress in PVAT research and discusses the potential of PVAT as a target for the treatment of atherosclerosis.
Classically, the pathogenesis of atherosclerosis has been considered to begin with damage to the intima of blood vessels (1–3), and endothelial cells have been regarded as the primary therapeutic target (4–6). Recent research, in turn, has demonstrated that perivascular adipose tissue (PVAT), the connective tissue surrounding blood vessels, substantially contributes to vascular physiological responses in an outside-in manner (7). Under non-pathological conditions, PVAT maintains vascular homeostasis and prevents progression of vascular inflammation and atherosclerosis. However, under pathological conditions, such as metabolic disorders, chronic inflammation, and aging, PVAT becomes a source of inflammatory mediators that exacerbate atherosclerosis (8). While the analysis of atherosclerotic plaque at the single-cell level has been performed (9, 10), integrative omics approaches involving PVAT could provide insights into a greater understanding of the pathophysiology of atherosclerosis and the molecular basis of the diverse functions of PVAT. Recently, glucagon-like peptide-1 receptor (GLP1R) agonists and sodium-glucose cotransporter-2 (SGLT2) inhibitors demonstrated cardiovascular benefits that might not be directly attributed to glycemic control (11). These agents have been shown to alter the quantity and quality of human coronary PVAT and epicardial adipose tissue, where PVAT might be potentially involved in such pathologies. In this review, we summarize recent advances in PVAT research and discuss the role of PVAT as a therapeutic target for atherosclerosis.
Most arteries and veins with a diameter of 100 µm or greater are surrounded by PVAT, with a few exceptions, such as the cerebral arteries (12). PVAT is a connective tissue composed of adipocytes, preadipocytes, mesenchymal stem cells, fibroblasts, immune cells (macrophages, lymphocytes, and eosinophils), vascular cells, and nerves (13). PVAT has previously been referred to as epicardial adipose tissue (EAT), specifically pericoronary EAT for coronary arteries (11, 14), periaortic adipose tissue for the aorta (15), and visceral periadventitial adipose tissue for the mesenteric artery (16). Historically, PVAT was only considered a supportive tissue for blood vessels, but research conducted in 1991 on rat thoracic aorta revealed that vessels without PVAT had decreased reactivity to contractile stimuli such as epinephrine and electrical stimulation compared to vessels with PVAT, suggesting a potentially important role of PVAT in vascular reactivity (17).
Recent studies have classified adipose tissues into white and brown adipose tissue based on function and morphology in mammals (18, 19). Functionally, white adipose tissue mainly stores energy in the form of triglycerides in cells and, when needed, supplies it to the blood as free fatty acids. Morphologically, white adipose tissue has only a small amount of cytoplasm and monocular lipid droplets, whereas brown adipose tissue has multilocular lipid droplets and a high concentration of mitochondria expressing uncoupling protein 1 (UCP1), a protein located in the inner mitochondrial membrane that produces heat instead of adenosine triphosphate (20, 21). The characteristics of PVAT vary based on animal species and anatomic region. In rodents, the mesenteric artery is surrounded by white adipose tissue, the thoracic aorta by brown adipose-like tissue, and the abdominal aorta by adipose tissue with a mixture of white and brown adipocytes (22). The coronary arteries in mice have no surrounding adipose tissue, whereas in humans and large laboratory animals, such as rabbits and pigs, these vessels are all surrounded by PVAT (22). As noted above, the human coronary PVAT is contained within EAT (11, 14).
Recent studies have shown that adipose tissues function as endocrine organs (23). Adipocytes secrete a variety of cytokines, hormones, chemokines, and fatty acids referred to as adipokines (23). For example, PVAT secretes several pro-inflammatory factors such as interleukin-1β (IL-1β), interleukin-6 (IL-6), tumor necrosis factor-α (TNF-α), and monocyte chemotactic protein 1 (MCP-1), as well as anti-inflammatory factors such as adiponectin and nitric oxide (12, 14). Interestingly, the amount of adipokines secreted from PVAT does not correlate with the concentration of adipokines in plasma, suggesting the importance of the local autocrine or paracrine action of PVAT (14).
The removal of PVAT has been reported to significantly increase neointima formation after vascular injury, which was suppressed by transplanting healthy mouse subcutaneous adipose tissue, implying that PVAT has atheroprotective effects under non-pathological conditions (8). Consistently, selective deletion of peroxisome proliferator-activated receptor γ (PPARγ) specifically in vascular smooth muscle cells of mice has been reported to cause the loss of PVAT while simultaneously accelerating atherosclerosis (24), suggesting that the absence of healthy PVAT promotes the development of atherosclerosis. On the contrary, earlier studies have also shown that the pathological state of PVAT leads to atherosclerosis. High-fat and high-sucrose diet-induced obesity was found to cause inflammatory changes in the PVAT surrounding the femoral artery in mice, exacerbating the formation of atherosclerotic plaques after vascular injury (8). A study on the paired samples of EAT and subcutaneous adipose tissue collected during a coronary artery bypass graft surgery observed significantly higher levels of mRNA and protein expression of inflammatory cytokines (IL-1β, IL-6, MCP-1, and TNF-α) in EAT (14). These findings imply that PVAT in the pathological state promotes atherosclerosis and that PVAT is a source of inflammatory mediators in high-risk cardiac patients (14).
Catecholamine or cold stimulation has been shown to convert white adipose tissue into beige adipose tissue, which expresses UCP1 and resembles brown adipose tissue morphologically. This phenomenon is referred to as “browning” or “beiging” (25, 26). Peroxisome proliferator-activated receptor gamma coactivator 1-alpha (PPARGC1A) and PR domain-containing 16 (PRDM16) are known as critical regulators of beiging (27–30). Studies in mouse models of atherosclerosis have shown that in the early stage of atherosclerosis, the beiging of PVAT is induced and serves to prevent the development of atherosclerotic plaques (31). In contrast, in the late stage of atherosclerosis, activation of the transforming growth factor-β (TGF-β) signaling pathway leads to fibrosis of the PVAT adjacent to plaques, resulting in PVAT dysfunction, decreased UCP1 expression, and increased PVAT inflammation (31). Studies using PVAT stromal cells (PVASCs) have shown that PPARGC1A is highly expressed in young mice PVASCs, but PPARGC1A expression in PVASCs decreases with age, resulting in decreased brown adipogenic differentiation (32). Recently, a decrease in the beiging of thoracic PVAT has been observed in an aging hypertensive rat model and has been linked to vascular dysfunction (33). The age-related loss of beiging capacity in PVAT may contribute to age-related vascular dysfunction (Figure 1) (32, 33).
Figure 1. Role of perivascular adipose tissue (PVAT) in atherosclerosis. Acute vascular injury causes acute inflammation of PVAT (pro-inflammatory inside-out signaling) and triggers the process of “beiging” in PVAT as a protective mechanism. Beige PVAT then inhibits pathological vascular remodeling through the secretion of anti-inflammatory factors such as NRG4 (outside-in signaling). Chronic inflammation, metabolic disorder, or aging lead to PVAT dysfunction, promoting fibrosis adjacent to plaques and reducing the expression of beige adipose markers. Dysfunctional PVAT produces pro-inflammatory cytokines such as IL-1β and IL-6, exaggerating vascular inflammation (outside-in signaling). PVAT, perivascular adipose tissue; UCP1, uncoupling protein 1; NRG4, neuregulin 4; TGF-β, transforming growth factor-β; IL-1β, interleukin-1β; IL-6, interleukin-6.
We recently demonstrated that vascular injury induces PVAT beiging and suppresses vascular remodeling by modulating the inflammatory response (34). In a mouse model of vascular injury, macrophages accumulated in the PVAT and caused phenotypic changes of beiging. Silencing PRDM16, a key regulator to beiging, suppressed PVAT beiging and exacerbated inflammation and vascular remodeling following vascular injury. In contrast, the activation of PVAT beiging suppressed inflammation and pathological vascular remodeling via secretion of anti-inflammatory factors such as neuregulin 4 (NRG4), which critically regulate alternative macrophage activation. These findings suggest that PVAT beiging plays a pivotal anti-atherosclerotic role in the resolution of inflammation following vascular injury (Figure 1) (34).
Recent studies using comprehensive analyses of PVAT uncovered the developmental process of PVAT and its diverse functions. Through single-cell transcriptomic analysis of perinatal PVAT from mice, Angueira et al. identified several distinct cell types, such as preadipocytes and mesenchymal progenitor cells (35). Subsequent cell differentiation assays and genetic lineage tracing studies revealed that the formation of PVAT in the aorta is mediated by fibroblast progenitor cells. In adult mice, the aortic adventitia was found to lack fibroblastic preadipocytes but contain adipogenic smooth muscle cells that contribute to adipocyte formation. These findings were mirrored in human adult PVAT samples analyzed using single-nucleus RNA sequencing, which revealed presumptive fibroblastic and smooth muscle-like adipocyte progenitor cells (35).
Pan et al. identified a high degree of transcriptional heterogeneity within PVASCs in the murine thoracic PVAT through single-cell RNA sequencing analysis of primary cultured PVASCs (Sca1+/CD90+/CD146+/CD29+) (32). They identified 10–12 distinct subpopulations with diverse lineages, including adipogenic, epithelial, neural, and endothelial lineages. Gene ontology analysis revealed that pathways related to vascular development were enriched in PVASCs, indicating a potential role in vascular remodeling. Additionally, human PVASCs were found to be involved in vascular remodeling and neointima formation upon transplantation into the mouse carotid artery ligation model. Gu et al. used single-cell RNA sequencing to demonstrate that mesenchymal stem cells expressing CD29+/Sca1+/PLIN1−/PECAM1− (referred to as perivascular adipose tissue-derived stem cells, or PV-ADSCs) reside in PVAT and contribute to vascular remodeling through migration and smooth muscle cell differentiation (36). Using a vein graft model, the authors demonstrated that PV-ADSCs transplanted into the adventitia side of the vein graft significantly promote neointima formation through migration and differentiation to smooth muscle cells in vivo.
Our research group has recently discovered, through the utilization of bulk RNA sequencing, that PVAT undergoes beiging after acute vascular injury, which fine-tunes inflammatory response and thus vascular remodeling as a protective mechanism (34). Single-cell RNA sequencing further revealed that beige adipocytes abundantly express Nrg4 (34). NRG4 is a member of the NRG protein family that acts via the ErbB receptor tyrosine kinase, and the NRG4-ErbB4 pathway is reported to suppress inflammation in macrophages (37, 38). Consistently, Shi et al. reported that NRG4 can inhibit endothelial inflammation through the ErbB4-protein kinase B (Akt)-nuclear factor (NF)-κB pathway and protect against endothelial injury and atherosclerosis (39). These findings imply that the beiging of PVAT plays a crucial anti-atherosclerotic role in the resolution of inflammation following vascular injury, in part through the secretion of NRG4 (Figure 1) (34).
Inflammation plays a significant role in the development and progression of atherosclerosis (2, 40, 41). Recent studies have shown that the inflammatory response induced by vascular damage expands into PVAT. In the mouse femoral artery wire injury model and rat iliac artery balloon injury model, vascular injury significantly upregulated the expression of inflammatory cytokines in PVAT (42). In a study using EAT specimens from patients undergoing cardiac surgery, macrophage infiltration and inflammatory cytokine expression were seen to be higher in the EAT of patients with coronary artery disease than in that of patients without coronary artery disease. The ratio of M1 (inflammatory) to M2 (anti-inflammatory) macrophages was positively correlated with the severity of coronary artery disease (43). Inflammatory cells accumulate in perivascular tissues prior to accumulation in the vessels after vascular injury, and inflammatory cell infiltration occurs from the outside to the inside of the vessel (34, 42). Vascular endothelial growth factor (VEGF), derived from adventitial fibroblasts, contributes to neointimal hyperplasia through vasa vasorum proliferation by activating the VEGFR2-extracellular signal-regulated kinase (ERK)1/2 signaling pathway (44), suggesting that adventitial fibroblasts play an important role in the infiltration of inflammatory cells from the outer to the inner vasculature.
18F-fluorodeoxyglucose positron emission tomography is a useful tool for assessing inflammation in adipose tissue, but it is expensive and not available in all healthcare facilities (45, 46). Recently, a non-invasive computed tomography angiography (CTA) technique was developed to quantify PVAT inflammation caused by vascular damage (47). In this method, the CT marker fat attenuation index (FAI) was determined by analyzing human coronary PVAT (EAT) obtained during coronary artery bypass graft surgery and was found to reflect adipose tissue inflammation. FAI measurements pertaining to human coronary arteries have a potential for detecting inflammation in acute coronary syndromes and vulnerable atherosclerotic plaques with reasonable sensitivity and specificity (47). In addition, a study involving 3,912 patients who underwent coronary CTA found that peri-coronary FAI was able to predict cardiac risk more effectively than traditional coronary risk factors (48). Furthermore, peri-coronary FAI levels were significantly higher in patients with coronary spastic angina compared to those without, implying that perivascular inflammation may play a role in the development of coronary spasm (49). In patients with abdominal aortic aneurysms, high periaortic FAI levels were also found to be an independent predictor of aortic aneurysm progression (50). However, perivascular inflammation can be present even in the absence of plaque, raising the question of what comes first (51). It is challenging to discern between the inside-out vs. outside-in issues. This noninvasive approach to appraising PVAT inflammation by CTA may aid in predicting atherosclerotic stability and the development of cardiovascular events, but further evaluation is required to determine its efficacy.
We recently demonstrated that local activation of beiging in mouse femoral artery PVAT using a pluronic gel containing CL316243, a specific β3-adrenergic receptor agonist, suppressed inflammation and vascular remodeling after vascular injury (34). Another study confirmed that local injection of human PVASC, which overexpresses Ppargc1a to promote beiging, into the PVAT after ligation of mouse carotid arteries significantly reduced perivascular collagen deposition (32). These results suggest that the beiging activation of PVAT may be a promising therapeutic target for atherosclerosis. However, some research indicates that systemic activation of beiging exacerbates atherosclerosis via brown fat-mediated lipolysis (52), suggesting that the anti-atherosclerotic effects of beiging activation may differ depending on which adipose tissue is targeted for beiging.
Recent cardiovascular clinical trials have demonstrated that antidiabetic medications can provide cardiovascular benefits beyond glycemic control. GLP1R agonists, which are used to treat type 2 diabetes and obesity, have been shown to lower the incidence of cardiovascular death, myocardial infarction, and stroke (53–55). GLP1R agonists have been found to reduce EAT thickness more effectively than overall weight loss in patients with type 2 diabetes and obesity (56–58). Notably, GLP1R is expressed in the EAT but not in subcutaneous adipose tissue (59), suggesting that GLP1R agonists may directly impact PVAT/EAT. While studies in rats have indicated that GLP1R agonists stimulate the thermogenic activity of brown adipose tissue via hypothalamic AMP-activated protein kinase (60), they may also exert their effects through other pathways. Dozio et al. collected EAT from patients with coronary artery disease undergoing coronary artery bypass graft surgeries and performed microarray analysis to show that GLP1R expression in the EAT was positively correlated with the expression of genes that induce white-to-brown adipose tissue differentiation (61). GLP1R agonists have been proposed to target GLP1R in the EAT to inhibit local adipogenesis, enhance fat utilization, and induce brown adipose tissue differentiation (11, 61).
SGLT2 inhibitors, which are used to treat heart failure regardless of diabetes status, have been shown in cardiovascular outcome trials to reduce the risk of major adverse cardiovascular events, cardiovascular death, and heart failure (62, 63). SGLT2 inhibitors such as dapagliflozin and empagliflozin have significantly reduced the EAT thickness or volume in humans (64–69). In mice with diet-induced obesity, SGLT2 inhibition by empagliflozin promotes fat utilization and beiging of adipose tissue while decreasing inflammation and insulin resistance by polarizing macrophages in adipose tissue to an anti-inflammatory state (70–72). The SGLT2 inhibitor luseogliflozin suppresses neointimal hyperplasia after vascular injury in high-fat diet-fed mice, partly by suppressing macrophage platelet-derived growth factor subunit B expression in the PVAT (73). Further research is needed to determine the independent effects of SGLT2 inhibitors on human PVAT/EAT.
Establishing causality in the relationship between inflamed vessels and adjacent fat inflammation and signaling is challenging and may be confounded by outside-in signaling. While current evidence suggests a bidirectional relationship between these phenomena, further research is necessary to determine the directionality and underlying mechanisms. Additionally, while existing data from mouse studies supports the hypothesis that PVAT is involved in atherosclerosis, normal mice differ from humans in susceptibility to atherosclerosis and vascular remodeling. Therefore, we need to be careful when applying results obtained in mice to humans. Further research is necessary to establish the clinical relevance of PVAT and to identify potential therapeutic targets for human vascular disease. Finally, while the current data support the hypothesis that GLP1R agonists and SGLT2 inhibitors may target PVAT/EAT to exert cardiovascular protection, it is important to note that this conclusion is based on limited evidence and may be subject to alternative interpretations. Previous investigations have suggested that these agents can induce generalized weight loss, improve insulin sensitivity, reduce blood pressure, lower lipid levels, and decrease visceral and epicardial adiposity (62, 63, 74–76). To fully elucidate the mechanisms underlying these effects and their potential relevance to PVAT/EAT, further research is warranted.
Recent studies have shown that PVAT plays a crucial role in maintaining vascular homeostasis and preventing the development of atherosclerosis. Integrated omics analyses have revealed that PVAT undergoes a process known as “beiging” after acute vascular injury, which serves as a protective mechanism by inhibiting pathological vascular remodeling through the secretion of anti-inflammatory factors such as NRG4. However, chronic inflammation, metabolic disorder, or aging can lead to PVAT dysfunction, resulting in fibrosis adjacent to plaques and reduced PVAT beiging in the advanced stages of atherosclerosis. Future studies targeting PVAT will open a new avenue for treating atherosclerosis.
All authors listed have made a substantial, direct, and intellectual contribution to the work and have approved it for publication. All authors contributed to the article and approved the submitted version.
This work was supported by the Japan Society for the Promotion of Science KAKENHI (22K16097), Japan Heart Foundation Research Grant, Japan Foundation for Applied Enzymology, and MSD Life Science Foundation (to Y.A.).
The authors declare that the research was conducted in the absence of any commercial or financial relationships that could be construed as a potential conflict of interest.
All claims expressed in this article are solely those of the authors and do not necessarily represent those of their affiliated organizations, or those of the publisher, the editors and the reviewers. Any product that may be evaluated in this article, or claim that may be made by its manufacturer, is not guaranteed or endorsed by the publisher.
1. Ross R, Glomset JA. The pathogenesis of atherosclerosis. N Engl J Med. (1976) 295:369–77. doi: 10.1056/nejm197608122950707
2. Rader DJ, Daugherty A. Translating molecular discoveries into new therapies for atherosclerosis. Nature. (2008) 451:904–13. doi: 10.1038/nature06796
3. Silvestre-Roig C, Braster Q, Ortega-Gomez A, Soehnlein O. Neutrophils as regulators of cardiovascular inflammation. Nat Rev Cardiol. (2020) 17:327–40. doi: 10.1038/s41569-019-0326-7
4. Gimbrone MA Jr, García-Cardeña G. Endothelial cell dysfunction and the pathobiology of atherosclerosis. Circ Res. (2016) 118:620–36. doi: 10.1161/circresaha.115.306301
5. Libby P, Ridker PM, Hansson GK. Inflammation in atherosclerosis: from pathophysiology to practice. J Am Coll Cardiol. (2009) 54:2129–38. doi: 10.1016/j.jacc.2009.09.009
6. Aikawa M, Sugiyama S, Hill CC, Voglic SJ, Rabkin E, Fukumoto Y, et al. Lipid lowering reduces oxidative stress and endothelial cell activation in rabbit atheroma. Circulation. (2002) 106:1390–6. doi: 10.1161/01.cir.0000028465.52694.9b
7. Kim HW, Shi H, Winkler MA, Lee R, Weintraub NL. Perivascular adipose tissue and vascular perturbation/atherosclerosis. Arterioscler Thromb Vasc Biol. (2020) 40:2569–76. doi: 10.1161/ATVBAHA.120.312470
8. Takaoka M, Nagata D, Kihara S, Shimomura I, Kimura Y, Tabata Y, et al. Periadventitial adipose tissue plays a critical role in vascular remodeling. Circ Res. (2009) 105:906–11. doi: 10.1161/CIRCRESAHA.109.199653
9. Depuydt MAC, Prange KHM, Slenders L, Ord T, Elbersen D, Boltjes A, et al. Microanatomy of the human atherosclerotic plaque by single-cell transcriptomics. Circ Res. (2020) 127:1437–55. doi: 10.1161/CIRCRESAHA.120.316770
10. Emoto T, Yamamoto H, Yamashita T, Takaya T, Sawada T, Takeda S, et al. Single-cell RNA sequencing reveals a distinct immune landscape of myeloid cells in coronary culprit plaques causing acute coronary syndrome. Circulation. (2022) 145:1434–6. doi: 10.1161/CIRCULATIONAHA.121.058414
11. Iacobellis G. Epicardial adipose tissue in contemporary cardiology. Nat Rev Cardiol. (2022) 19:593–606. doi: 10.1038/s41569-022-00679-9
12. Henrichot E, Juge-Aubry CE, Pernin A, Pache JC, Velebit V, Dayer JM, et al. Production of chemokines by perivascular adipose tissue: a role in the pathogenesis of atherosclerosis? Arterioscler Thromb Vasc Biol. (2005) 25:2594–9. doi: 10.1161/01.ATV.0000188508.40052.35
13. Gil-Ortega M, Somoza B, Huang Y, Gollasch M, Fernandez-Alfonso MS. Regional differences in perivascular adipose tissue impacting vascular homeostasis. Trends Endocrinol Metab. (2015) 26:367–75. doi: 10.1016/j.tem.2015.04.003
14. Mazurek T, Zhang L, Zalewski A, Mannion JD, Diehl JT, Arafat H, et al. Human epicardial adipose tissue is a source of inflammatory mediators. Circulation. (2003) 108:2460–6. doi: 10.1161/01.CIR.0000099542.57313.C5
15. Police SB, Thatcher SE, Charnigo R, Daugherty A, Cassis LA. Obesity promotes inflammation in periaortic adipose tissue and angiotensin II-induced abdominal aortic aneurysm formation. Arterioscler Thromb Vasc Biol. (2009) 29:1458–64. doi: 10.1161/ATVBAHA.109.192658
16. Verlohren S, Dubrovska G, Tsang SY, Essin K, Luft FC, Huang Y, et al. Visceral periadventitial adipose tissue regulates arterial tone of mesenteric arteries. Hypertension. (2004) 44:271–6. doi: 10.1161/01.HYP.0000140058.28994.ec
17. Soltis EE, Cassis LA. Influence of perivascular adipose tissue on rat aortic smooth muscle responsiveness. Clin Exp Hypertens A. (1991) 13:277–96. doi: 10.3109/10641969109042063
18. Harms M, Seale P. Brown and beige fat: development, function and therapeutic potential. Nat Med. (2013) 19:1252–63. doi: 10.1038/nm.3361
19. Virtanen KA, Lidell ME, Orava J, Heglind M, Westergren R, Niemi T, et al. Functional brown adipose tissue in healthy adults. N Engl J Med. (2009) 360:1518–25. doi: 10.1056/NEJMoa0808949
20. Kajimura S, Spiegelman BM, Seale P. Brown and beige fat: physiological roles beyond heat generation. Cell Metab. (2015) 22:546–59. doi: 10.1016/j.cmet.2015.09.007
21. Wang W, Seale P. Control of brown and beige fat development. Nat Rev Mol Cell Biol. (2016) 17:691–702. doi: 10.1038/nrm.2016.96
22. Brown NK, Zhou Z, Zhang J, Zeng R, Wu J, Eitzman DT, et al. Perivascular adipose tissue in vascular function and disease: a review of current research and animal models. Arterioscler Thromb Vasc Biol. (2014) 34:1621–30. doi: 10.1161/ATVBAHA.114.303029
23. Skurk T, Alberti-Huber C, Herder C, Hauner H. Relationship between adipocyte size and adipokine expression and secretion. J Clin Endocrinol Metab. (2007) 92:1023–33. doi: 10.1210/jc.2006-1055
24. Chang L, Villacorta L, Li R, Hamblin M, Xu W, Dou C, et al. Loss of perivascular adipose tissue on peroxisome proliferator-activated receptor-gamma deletion in smooth muscle cells impairs intravascular thermoregulation and enhances atherosclerosis. Circulation. (2012) 126:1067–78. doi: 10.1161/CIRCULATIONAHA.112.104489
25. Wu J, Bostrom P, Sparks LM, Ye L, Choi JH, Giang AH, et al. Beige adipocytes are a distinct type of thermogenic fat cell in mouse and human. Cell. (2012) 150:366–76. doi: 10.1016/j.cell.2012.05.016
26. Sidossis L, Kajimura S. Brown and beige fat in humans: thermogenic adipocytes that control energy and glucose homeostasis. J Clin Invest. (2015) 125:478–86. doi: 10.1172/JCI78362
27. Dempersmier J, Sambeat A, Gulyaeva O, Paul SM, Hudak CS, Raposo HF, et al. Cold-inducible Zfp516 activates UCP1 transcription to promote browning of white fat and development of brown fat. Mol Cell. (2015) 57:235–46. doi: 10.1016/j.molcel.2014.12.005
28. Seale P, Bjork B, Yang W, Kajimura S, Chin S, Kuang S, et al. PRDM16 controls a brown fat/skeletal muscle switch. Nature. (2008) 454:961–7. doi: 10.1038/nature07182
29. Ohno H, Shinoda K, Spiegelman BM, Kajimura S. PPARgamma agonists induce a white-to-brown fat conversion through stabilization of PRDM16 protein. Cell Metab. (2012) 15:395–404. doi: 10.1016/j.cmet.2012.01.019
30. Cohen P, Levy JD, Zhang Y, Frontini A, Kolodin DP, Svensson KJ, et al. Ablation of PRDM16 and beige adipose causes metabolic dysfunction and a subcutaneous to visceral fat switch. Cell. (2014) 156:304–16. doi: 10.1016/j.cell.2013.12.021
31. Kim S, Lee ES, Lee SW, Kim YH, Lee CH, Jo DG, et al. Site-specific impairment of perivascular adipose tissue on advanced atherosclerotic plaques using multimodal nonlinear optical imaging. Proc Natl Acad Sci U S A. (2019) 116:17765–74. doi: 10.1073/pnas.1902007116
32. Pan XX, Ruan CC, Liu XY, Kong LR, Ma Y, Wu QH, et al. Perivascular adipose tissue-derived stromal cells contribute to vascular remodeling during aging. Aging Cell. (2019) 18:e12969. doi: 10.1111/acel.12969
33. Kong LR, Zhou YP, Chen DR, Ruan CC, Gao PJ. Decrease of perivascular adipose tissue browning is associated with vascular dysfunction in spontaneous hypertensive rats during aging. Front Physiol. (2018) 9:400. doi: 10.3389/fphys.2018.00400
34. Adachi Y, Ueda K, Nomura S, Ito K, Katoh M, Katagiri M, et al. Beiging of perivascular adipose tissue regulates its inflammation and vascular remodeling. Nat Commun. (2022) 13:5117. doi: 10.1038/s41467-022-32658-6
35. Angueira AR, Sakers AP, Holman CD, Cheng L, Arbocco MN, Shamsi F, et al. Defining the lineage of thermogenic perivascular adipose tissue. Nat Metab. (2021) 3(4):469–84. doi: 10.1038/s42255-021-00380-0
36. Gu W, Nowak WN, Xie Y, Le Bras A, Hu Y, Deng J, et al. Single-cell RNA-sequencing and metabolomics analyses reveal the contribution of perivascular adipose tissue stem cells to vascular remodeling. Arterioscler Thromb Vasc Biol. (2019) 39:2049–66. doi: 10.1161/ATVBAHA.119.312732
37. Schumacher MA, Hedl M, Abraham C, Bernard JK, Lozano PR, Hsieh JJ, et al. Erbb4 signaling stimulates pro-inflammatory macrophage apoptosis and limits colonic inflammation. Cell Death Dis. (2017) 8:e2622. doi: 10.1038/cddis.2017.42
38. Schumacher MA, Dennis IC, Liu CY, Robinson C, Shang J, Bernard JK, et al. NRG4-ErbB4 signaling represses proinflammatory macrophage activity. Am J Physiol Gastrointest Liver Physiol. (2021) 320:G990–G1001. doi: 10.1152/ajpgi.00296.2020
39. Shi L, Li Y, Xu X, Cheng Y, Meng B, Xu J, et al. Brown adipose tissue-derived Nrg4 alleviates endothelial inflammation and atherosclerosis in male mice. Nat Metab. (2022) 4:1573–90. doi: 10.1038/s42255-022-00671-0
40. Ruparelia N, Chai JT, Fisher EA, Choudhury RP. Inflammatory processes in cardiovascular disease: a route to targeted therapies. Nat Rev Cardiol. (2017) 14:133–44. doi: 10.1038/nrcardio.2016.185
41. Libby P, Ridker PM, Hansson GK. Progress and challenges in translating the biology of atherosclerosis. Nature. (2011) 473:317–25. doi: 10.1038/nature10146
42. Takaoka M, Suzuki H, Shioda S, Sekikawa K, Saito Y, Nagai R, et al. Endovascular injury induces rapid phenotypic changes in perivascular adipose tissue. Arterioscler Thromb Vasc Biol. (2010) 30:1576–82. doi: 10.1161/ATVBAHA.110.207175
43. Hirata Y, Tabata M, Kurobe H, Motoki T, Akaike M, Nishio C, et al. Coronary atherosclerosis is associated with macrophage polarization in epicardial adipose tissue. J Am Coll Cardiol. (2011) 58:248–55. doi: 10.1016/j.jacc.2011.01.048
44. Li XD, Hong MN, Chen J, Lu YY, Ye MQ, Ma Y, et al. Adventitial fibroblast-derived vascular endothelial growth factor promotes vasa vasorum-associated neointima formation and macrophage recruitment. Cardiovasc Res. (2020) 116:708–20. doi: 10.1093/cvr/cvz159
45. Christen T, Sheikine Y, Rocha VZ, Hurwitz S, Goldfine AB, Di Carli M, et al. Increased glucose uptake in visceral versus subcutaneous adipose tissue revealed by PET imaging. JACC Cardiovasc Imaging. (2010) 3:843–51. doi: 10.1016/j.jcmg.2010.06.004
46. Bucerius J, Mani V, Wong S, Moncrieff C, Izquierdo-Garcia D, Machac J, et al. Arterial and fat tissue inflammation are highly correlated: a prospective 18F-FDG PET/CT study. Eur J Nucl Med Mol Imaging. (2014) 41:934–45. doi: 10.1007/s00259-013-2653-y
47. Antonopoulos AS, Sanna F, Sabharwal N, Thomas S, Oikonomou EK, Herdman L, et al. Detecting human coronary inflammation by imaging perivascular fat. Sci Transl Med. (2017) 9(398):eaal2658. doi: 10.1126/scitranslmed.aal2658
48. Oikonomou EK, Marwan M, Desai MY, Mancio J, Alashi A, Hutt Centeno E, et al. Non-invasive detection of coronary inflammation using computed tomography and prediction of residual cardiovascular risk (the CRISP CT study): a post-hoc analysis of prospective outcome data. Lancet. (2018) 392:929–39. doi: 10.1016/S0140-6736(18)31114-0
49. Ueno H, Hoshino M, Sugiyama T, Kanaji Y, Nogami K, Horie T, et al. Pericoronary adipose tissue inflammation on coronary computed tomography in patients with vasospastic angina. JACC Cardiovasc Imaging. (2021) 14:511–2. doi: 10.1016/j.jcmg.2020.08.002
50. Yamaguchi M, Yonetsu T, Hoshino M, Sugiyama T, Kanaji Y, Yasui Y, et al. Clinical significance of increased computed tomography attenuation of periaortic adipose tissue in patients with abdominal aortic aneurysms. Circ J. (2021) 85:2172–80. doi: 10.1253/circj.CJ-20-1014
51. Rodriguez-Granillo GA, Carrascosa P. Detection of coronary inflammation. Lancet. (2019) 393:2198–9. doi: 10.1016/S0140-6736(19)30225-9
52. Sui W, Li H, Yang Y, Jing X, Xue F, Cheng J, et al. Bladder drug mirabegron exacerbates atherosclerosis through activation of brown fat-mediated lipolysis. Proc Natl Acad Sci U S A. (2019) 116:10937–42. doi: 10.1073/pnas.1901655116
53. Marso SP, Daniels GH, Brown-Frandsen K, Kristensen P, Mann JF, Nauck MA, et al. Liraglutide and cardiovascular outcomes in type 2 diabetes. N Engl J Med. (2016) 375:311–22. doi: 10.1056/NEJMoa1603827
54. Marso SP, Bain SC, Consoli A, Eliaschewitz FG, Jodar E, Leiter LA, et al. Semaglutide and cardiovascular outcomes in patients with type 2 diabetes. N Engl J Med. (2016) 375:1834–44. doi: 10.1056/NEJMoa1607141
55. Gerstein HC, Colhoun HM, Dagenais GR, Diaz R, Lakshmanan M, Pais P, et al. Dulaglutide and cardiovascular outcomes in type 2 diabetes (REWIND): a double-blind, randomised placebo-controlled trial. Lancet. (2019) 394:121–30. doi: 10.1016/S0140-6736(19)31149-3
56. Iacobellis G, Villasante Fricke AC. Effects of semaglutide versus dulaglutide on epicardial fat thickness in subjects with type 2 diabetes and obesity. J Endocr Soc. (2020) 4:bvz042. doi: 10.1210/jendso/bvz042
57. Morano S, Romagnoli E, Filardi T, Nieddu L, Mandosi E, Fallarino M, et al. Short-term effects of glucagon-like peptide 1 (GLP-1) receptor agonists on fat distribution in patients with type 2 diabetes mellitus: an ultrasonography study. Acta Diabetol. (2015) 52:727–32. doi: 10.1007/s00592-014-0710-z
58. Dutour A, Abdesselam I, Ancel P, Kober F, Mrad G, Darmon P, et al. Exenatide decreases liver fat content and epicardial adipose tissue in patients with obesity and type 2 diabetes: a prospective randomized clinical trial using magnetic resonance imaging and spectroscopy. Diabetes Obes Metab. (2016) 18:882–91. doi: 10.1111/dom.12680
59. Iacobellis G, Camarena V, Sant DW, Wang G. Human epicardial fat expresses glucagon-like peptide 1 and 2 receptors genes. Horm Metab Res. (2017) 49:625–30. doi: 10.1055/s-0043-109563
60. Beiroa D, Imbernon M, Gallego R, Senra A, Herranz D, Villarroya F, et al. GLP-1 agonism stimulates brown adipose tissue thermogenesis and browning through hypothalamic AMPK. Diabetes. (2014) 63:3346–58. doi: 10.2337/db14-0302
61. Dozio E, Vianello E, Malavazos AE, Tacchini L, Schmitz G, Iacobellis G, et al. Epicardial adipose tissue GLP-1 receptor is associated with genes involved in fatty acid oxidation and white-to-brown fat differentiation: a target to modulate cardiovascular risk? Int J Cardiol. (2019) 292:218–24. doi: 10.1016/j.ijcard.2019.04.039
62. Zinman B, Wanner C, Lachin JM, Fitchett D, Bluhmki E, Hantel S, et al. Empagliflozin, cardiovascular outcomes, and mortality in type 2 diabetes. N Engl J Med. (2015) 373:2117–28. doi: 10.1056/NEJMoa1504720
63. Wiviott SD, Raz I, Bonaca MP, Mosenzon O, Kato ET, Cahn A, et al. Dapagliflozin and cardiovascular outcomes in type 2 diabetes. N Engl J Med. (2019) 380:347–57. doi: 10.1056/NEJMoa1812389
64. Requena-Ibanez JA, Santos-Gallego CG, Rodriguez-Cordero A, Vargas-Delgado AP, Mancini D, Sartori S, et al. Mechanistic insights of empagliflozin in nondiabetic patients with HFrEF: from the EMPA-TROPISM study. JACC Heart Fail. (2021) 9:578–89. doi: 10.1016/j.jchf.2021.04.014
65. Iacobellis G, Gra-Menendez S. Effects of dapagliflozin on epicardial fat thickness in patients with type 2 diabetes and obesity. Obesity. (2020) 28:1068–74. doi: 10.1002/oby.22798
66. Sato T, Aizawa Y, Yuasa S, Kishi S, Fuse K, Fujita S, et al. The effect of dapagliflozin treatment on epicardial adipose tissue volume. Cardiovasc Diabetol. (2018) 17:6. doi: 10.1186/s12933-017-0658-8
67. Yagi S, Hirata Y, Ise T, Kusunose K, Yamada H, Fukuda D, et al. Canagliflozin reduces epicardial fat in patients with type 2 diabetes mellitus. Diabetol Metab Syndr. (2017) 9:78. doi: 10.1186/s13098-017-0275-4
68. Fukuda T, Bouchi R, Terashima M, Sasahara Y, Asakawa M, Takeuchi T, et al. Ipragliflozin reduces epicardial fat accumulation in non-obese type 2 diabetic patients with visceral obesity: a pilot study. Diabetes Ther. (2017) 8:851–61. doi: 10.1007/s13300-017-0279-y
69. Bouchi R, Terashima M, Sasahara Y, Asakawa M, Fukuda T, Takeuchi T, et al. Luseogliflozin reduces epicardial fat accumulation in patients with type 2 diabetes: a pilot study. Cardiovasc Diabetol. (2017) 16:32. doi: 10.1186/s12933-017-0516-8
70. Xu L, Nagata N, Nagashimada M, Zhuge F, Ni Y, Chen G, et al. SGLT2 inhibition by empagliflozin promotes fat utilization and browning and attenuates inflammation and insulin resistance by polarizing M2 macrophages in diet-induced obese mice. EBioMedicine. (2017) 20:137–49. doi: 10.1016/j.ebiom.2017.05.028
71. Xu L, Nagata N, Chen G, Nagashimada M, Zhuge F, Ni Y, et al. Empagliflozin reverses obesity and insulin resistance through fat browning and alternative macrophage activation in mice fed a high-fat diet. BMJ Open Diabetes Res Care. (2019) 7:e000783. doi: 10.1136/bmjdrc-2019-000783
72. Xu L, Xu C, Liu X, Li X, Li T, Yu X, et al. Empagliflozin induces white adipocyte browning and modulates mitochondrial dynamics in KK Cg-Ay/J mice and mouse adipocytes. Front Physiol. (2021) 12:745058. doi: 10.3389/fphys.2021.745058
73. Mori Y, Terasaki M, Hiromura M, Saito T, Kushima H, Koshibu M, et al. Luseogliflozin attenuates neointimal hyperplasia after wire injury in high-fat diet-fed mice via inhibition of perivascular adipose tissue remodeling. Cardiovasc Diabetol. (2019) 18:143. doi: 10.1186/s12933-019-0947-5
74. Rajeev SP, Cuthbertson DJ, Wilding JP. Energy balance and metabolic changes with sodium-glucose co-transporter 2 inhibition. Diabetes Obes Metab. (2016) 18:125–34. doi: 10.1111/dom.12578
75. Wilcox T, De Block C, Schwartzbard AZ, Newman JD. Diabetic agents, from metformin to SGLT2 inhibitors and GLP1 receptor agonists: JACC focus seminar. J Am Coll Cardiol. (2020) 75:1956–74. doi: 10.1016/j.jacc.2020.02.056
Keywords: perivascular adipose tissue, beiging, brown adipose tissue, inflammation, atherosclerosis, remodeling
Citation: Adachi Y, Ueda K and Takimoto E (2023) Perivascular adipose tissue in vascular pathologies—a novel therapeutic target for atherosclerotic disease?. Front. Cardiovasc. Med. 10:1151717. doi: 10.3389/fcvm.2023.1151717
Received: 26 January 2023; Accepted: 28 April 2023;
Published: 26 May 2023.
Edited by:
Neal Lee Weintraub, Augusta University, United StatesReviewed by:
Sheldon Litwin, Medical University of South Carolina, United States© 2023 Adachi, Ueda and Takimoto. This is an open-access article distributed under the terms of the Creative Commons Attribution License (CC BY). The use, distribution or reproduction in other forums is permitted, provided the original author(s) and the copyright owner(s) are credited and that the original publication in this journal is cited, in accordance with accepted academic practice. No use, distribution or reproduction is permitted which does not comply with these terms.
*Correspondence: Kazutaka Ueda dWVkYWstdGt5QHVtaW4uYWMuanA=
Disclaimer: All claims expressed in this article are solely those of the authors and do not necessarily represent those of their affiliated organizations, or those of the publisher, the editors and the reviewers. Any product that may be evaluated in this article or claim that may be made by its manufacturer is not guaranteed or endorsed by the publisher.
Research integrity at Frontiers
Learn more about the work of our research integrity team to safeguard the quality of each article we publish.