- 1Institute of Microcirculation, Chinese Academy of Medical Sciences & Peking Union Medical College, Beijing, China
- 2Graduate School, Chinese Academy of Medical Sciences & Peking Union Medical College, Beijing, China
Exosomes are biological small spherical lipid bilayer vesicles secreted by most cells in the body. Their contents include nucleic acids, proteins, and lipids. Exosomes can transfer material molecules between cells and consequently have a variety of biological functions, participating in disease development while exhibiting potential value as biomarkers and therapeutics. Growing evidence suggests that exosomes are vital mediators of vascular remodeling. Endothelial cells (ECs), vascular smooth muscle cells (VSMCs), inflammatory cells, and adventitial fibroblasts (AFs) can communicate through exosomes; such communication is associated with inflammatory responses, cell migration and proliferation, and cell metabolism, leading to changes in vascular function and structure. Essential hypertension (EH), atherosclerosis (AS), and pulmonary arterial hypertension (PAH) are the most common vascular diseases and are associated with significant vascular remodeling. This paper reviews the latest research progress on the involvement of exosomes in vascular remodeling through intercellular information exchange and provides new ideas for understanding related diseases.
Introduction
With an aging population, rapid urbanization, and risk factors such as smoking, sodium intake, elevated blood pressure, and obesity increasingly endangering cardiovascular health (1), cardiovascular disease is a major cause of morbidity and mortality worldwide (2, 3). Exploring the pathophysiology of cardiovascular disease is of great value in the search for new disease targets, biomarkers, and therapeutic approaches. Vascular remodeling is a complex adaptive process and a fundamental pathological process in cardiovascular disease. The vasculature responds to stimuli such as haemodynamic abnormalities and bioactive substances, which manifest as a series of structural and functional changes in the three components of the vascular wall, including cellular phenotypic transformation, cell migration and proliferation, and inflammatory cell infiltration (4). Communication between the adventitia, media, and intima through signals (5), such as signaling between endothelial cells (ECs) in the intima and vascular smooth muscle cells (VSMCs) in the media, is thought to be crucial for the initiation and development of pathological vascular remodeling (6); thus, vascular remodeling is a dynamic process involving the entire vessel wall.
Extracellular vesicles (EVs) are messengers of intercellular communication that carry and deliver a variety of signaling molecules, particularly proteins, mRNAs, and noncoding RNAs (7). Based on their biogenesis and size, EVs can be categorized into three main subtypes: exosomes (30–150 nm), microvesicles (100–1,000 nm), and apoptotic bodies (1,000–5,000 nm). Exosomes are intraluminal vesicles within multivesicular bodies (MVBs) that are generated by the endosomal sorting complex mechanism, microvesicles are vesicles produced by the outgrowth of cell membranes, and apoptotic bodies are fragments produced by cell collapse (8). Exosomes are capable of transmitting biological information between adjacent and distant cells (9) and are involved in the diagnosis and treatment of many diseases. Exosome-mediated intercellular communication is instrumental in maintaining homeostasis in the cardiovascular system (10, 11), and stem cell-derived exosomes have cardioprotective properties that balance efficacy and safety, indicating their potential therapeutic value (12, 13).
Vascular remodeling involves communication between various vessel wall cells in the vascular microenvironment. This article focuses on the typical pathophysiological processes of three common vascular diseases and explores the origin and targeting of exosomes and their functions in specific cases to summarize cellular interactions during vascular remodeling (Figure 1).
Origin and function of exosomes
Exosomes are small vesicles with lipid bilayer membranes of approximately 30–150 nm in diameter that can be released into the extracellular environment by a variety of cells, can transit through biological barriers and are extensively present in body fluids, including blood, urine, cerebrospinal fluid, and saliva (14–17). In 1985 Pan et al. (18) observed exosomes by immunoelectron microscopy and described them as small vesicles containing transferrin receptors secreted by sheep reticulocytes; most studies later concluded that exosomes are produced by the endosomal pathway (19, 20). The classic mechanism of exosome biogenesis is associated with the endosomal system; endocytosis is the initial step in which the plasma membrane invaginates into the cytoplasm to form early endosomes (EEs), which then mature into late endosomes (LEs). In EEs, NOTCH4 activation increases expression in RAB5A, whereas in the maturation process, NOTCH4 signaling is reduced, causing a reduction of RAB5A and increase in RAB7A (21, 22). Second inward invagination in LEs leads to the eventual formation of MVBs containing intraluminal vesicles (ILVs); the generation of MVBs and their intrinsic ILVs involve endosomal sorting complex required for transport (ESCRT)-dependent and ESCRT-independent machineries (23, 24). MVBs can be degraded in lysosomes or reach and fuse with the cell membrane in collaboration with microtubules, SNAREs (soluble N-ethylmaleimide-sensitive factor-attachment protein receptors), and Rab-GTPases, releasing ILVs known as exosomes into the extracellular environment (22, 25, 26). The exosomal contents are related to the location of the cell membrane and the local microenvironment at the time of exosome formation (27, 28), and exosomes also contain endogenous molecules from trans-Golgi network, endoplasmic reticulum (ER), and other organelles (29).
Many studies have shown that exosomes, microvesicles, and apoptotic bodies have distinct basic characteristics (30–34) (Table 1). However, the hypothesis that exosomes are produced only via the endosomal pathway is controversial. Pegtal et al. (35) suggested that exosomes are created by budding at both the plasma and endosome membranes, while Fordjour et al. (36) redirected CD63 from endosomes to the plasma membrane by eliminating its constitutive endocytosis signal in HEK293 cells and found that the budding of plasma membrane-localized CD63 remained strong, arguing against the ‘endosome-only’ hypothesis of exosome biogenesis. Due to methodological difficulties in separation and conflicting definitions, even though the term ‘exosomes’ is widely used, it has been suggested to be replaced by the term ‘small EVs (sEVs)’ according to the International Society for Extracellular Vesicles (ISEV) 2018 guidelines (37). Some EVs of nonendosomal origin have the same biophysical characteristics exhibited by exosomes (38), and it is technically difficult to effectively distinguish exosomes from other EVs, especially functional microvesicles (39). Some researchers consider ‘EV’ to be the preferred generic term, and some original studies investigated EVs, which equate to a mixture of exosomes and microvesicles. Therefore, in this review, the term ‘exosome’ is used if clarified in the referenced publication, and the term ‘EV’ is used if the differentiation is unclear.
In 1996, Raposo et al. (40) isolated exosomes from human and mouse B lymphocytes by differential centrifugation and found that the exosomes could induce antigen-specific MHC class II-restricted T-cell responses. In 2007, Valadi et al. (41) showed that exosomes can deliver a specific mRNA or miRNA to recipient cells and then modify recipient cell protein production and gene expression. Exosomes are important molecules for the transmission of information between cells and are no longer thought of as simply metabolic waste excreted by cells. Through autocrine, paracrine and endocrine communication between different tissues, exosomes regulate gene expression and cellular functions (42, 43) (Figure 2). Exosomes not only regulate physiological states such as tissue regeneration, immune surveillance, and stem cell plasticity (44–46) but also participate in the pathology of diseases such as cardiovascular diseases, neurodegenerative diseases and malignant tumors (47–49) and have potential value for precise diagnosis, prognosis assessment, and disease treatment.
Almost all types of eukaryotic cells, as well as platelets, produce exosomes. For instance, mesenchymal stem cells (MSCs) have powerful paracrine activity and can secrete numerous exosomes that have been implicated in tissue repair processes and shown to have promising curative effects on cardiovascular and liver diseases (50, 51). Macrophages are widely distributed in the body and can be polarized into M1 or M2 type macrophages, and exosomes released from macrophages can modulate inflammatory signals (52). In response to an appropriate trigger, such as the activation of specific receptors or the availability of signaling factors, exosomes carry molecules from donor cells and are released into the extracellular fluid. The envelopes of the majority of exosomes can remain unbroken, are diffusible in body fluids and transfer their contents to recipient cells in diverse ways, including direct fusion with the plasma membrane of the target cell, the formation of intercellular channels with target cells using gap junction proteins, and uptake by target cells via the endocytic pathway (53, 54), thereby regulating recipient cell function. Furthermore, exosomes are ideal natural nanocarriers for basic research and clinical applications because of their high biocompatibility, low immunogenicity, low toxicity, circulating stability and biological barrier permeability (55). Exosomes derived from dendritic cells can induce antigen-specific T-cell responses and hold great promise in cancer immunotherapy (56, 57). Exosomes that originate from MSCs can be administered via intravenous or intranasal delivery; are capable of crossing the blood–brain barrier, inhibiting chronic inflammation and promoting healing and have value in the treatment of brain injuries (58). Exosomes are also candidates for regenerative cardiovascular medicine because they have lower immunogenicity and tumorigenicity and are more stabilized in the circulation than stem cells (12).
Advances in understanding the role of exosomes in the pathophysiology of essential hypertension
Essential hypertension (EH) is one of the major risk factors for the global disease burden (59), and epidemiological investigations have shown that patients with blood pressure greater than 140/90 mmHg have significantly higher mortality rates (60). Guidelines assess patients with hypertension based on blood pressure values, as well as on multiple cardiovascular risk elements (61). The known pathophysiological alterations that occur in EH include activation of the renin-angiotensin-aldosterone system (RAAS), activation of the sympathetic nervous system (SNS), extracellular matrix imbalance, endothelial dysfunction, oxidative stress, inflammatory responses, and vascular stiffness (62). These abnormalities can work in synergy to accelerate the progression of vascular remodeling. Endothelial nitric oxide synthase (eNOS) function is uncoupled, and mitochondrial respiration produces large amounts of reactive oxygen species (ROS) (63). Then, excessive ROS production disrupts redox homeostasis, which can lead to impairment of antioxidant defense systems and a decrease in NO levels (64). In addition, the increased accumulation of ROS activates matrix metalloproteinases. The collagen and elastin content of the extracellular matrix of vascular smooth muscle is reduced, leading to vascular stiffness (65). Excessive activity of the RAAS and SNS induces structural and functional changes in VSMCs and ECs (66). Oxidative stress in the kidney and arterial wall is strongly associated with inflammatory cell infiltration, and in a salt-sensitive hypertension model, the renal tubular interstitium was infiltrated by lymphocytes and macrophages (67, 68). The intensity of inflammatory cell infiltration and the severity of blood pressure elevation are significantly correlated (69).
Exosomes and endothelial dysfunction
Increased platelet- and endothelium-derived EVs are markers of EC activation and vascular injury (70), and several studies have focused on the alterations and importance of platelet- and endothelium-derived EVs in hypertensive states (71–73). Bao et al. (74) showed that platelet-derived EVs (pEVs), which deliver miR-92a-3p to VSMCs after intimal injury, inhibit PTEN and promote activation of the Akt pathway and the production of Col8a1 in VSMCs, leading to the deposition of Col8a1 at the site of intimal injury and increased arterial wall stiffness. Wang et al. (75) discovered that dysfunctional ECs could also secrete miR-92a-rich EVs, leading to the contractile-to-synthetic phenotype change of VSMCs or causing EC dysfunction in a paracrine manner; that circulating miR-92a levels were increased in hypertensive patients and Ang II-induced hypertensive mice compared to healthy controls; and that human plasma levels of circulating miR-92a were positively correlated with pulse wave velocity (PWV), systolic blood pressure (SBP), and diastolic blood pressure (DBP) and inversely correlated with NO levels. MiR-92a induces vascular dysfunction and is involved in the pathology of endothelial injury and atherosclerosis. However, the correlation between pathological conditions and circulating or cellular levels of miR-92a remains inconclusive, and other works suggest that miR-92a may play a protective role by maintaining vascular homeostasis. MiR-92a expression was downregulated in elderly and aged B6D2F1 mice (a model of vascular aging), which led to age-related vascular dysfunction (76). Inflammatory cell-derived exosomes have also been implicated in EC dysfunction under hypertension. Osada-Oka et al. (77) showed that Ang II-induced macrophages released less of exosomal miR-17-3p, which increased the expression of the proinflammatory factor intracellular adhesion molecule-1 (ICAM-1) in ECs.
Shang et al. (78) revealed that exosomal miR-483-3p released from ECs had a protective effect on ECs. Human umbilical vein endothelial cells (HUVECs) were incubated with Ang II in vitro to simulate the pathophysiological state of hypertension. MiR-483 overexpression reversed Ang II-induced changes in angiotensin-converting enzyme 1 (ACE1), endothelin-1 (ET-1), connective tissue growth factor (CTGF) and transforming growth factor-β (TGF-β) levels in ECs. Telmisartan increased miR-483-3p levels in ECs. In addition, exosomal miR-483-3p released from ECs could also be taken up by SMCs, regulating their functions and reducing hypertension-induced vascular injury by downregulating the expression levels of ACE1, TGF-β and CTGF. MiR-483-3p was also shown to protect ECs against endothelial-mesenchymal transition and inflammation in a recent study by Esmerats et al. (79). Hypertension in its early stages may increase miR-483 levels in ECs due to compensatory mechanisms, but as the disease progresses, this negative feedback effect is suppressed, and increased dysfunction occurs in ECs and SMCs, eventually leading to a decrease in miR-483-3p levels; thus, serum miR-483-3p levels are significantly reduced in patients with long-term chronic hypertension (78).
Exosomes and the RAAS
Tong et al. (80) isolated cells from the thoracic aortas of Wistar-Kyoto (WKY) rats and spontaneously hypertensive rats (SHRs) and found that exosomes released from SHR adventitial fibroblasts (AFs) transferred ACE to VSMCs, thereby increasing Ang II levels within VSMCs and activating AT1R (Ang II [angiotensin II] type 1 receptor) to promote the migration of VSMCs. Subsequent studies demonstrated that miR-155-5p, which is derived from the EVs of AFs, protects the vasculature, while miR-135a-5p promotes structural changes in blood vessels. Compared to those in WKY rats, miR155-5p levels were reduced in EVs released from SHR extravascular fibroblasts. Intravenous delivery of EVs derived from WKY rats mediated miR155-5p transfer, thereby reducing ACE expression in VSMCs and the arterial blood of SHRs and attenuating vascular remodeling (81). In addition, elevated miR-135a-5p levels in the EVs of SHR AFs decreased FNDC5 expression in VSMCs (82). As FNDC5 can attenuate oxidative stress and inflammatory responses and inhibit the proliferation of VSMCs through the mitogen-activated protein kinase (MAPK) signaling pathway, miR-135a-5p delivery exacerbated VSMC proliferation and vascular remodeling (83, 84). Furthermore, Zou et al. (85) found that EVs from monocytes transferred miR-27a to ECs, decreased Mas receptor expression, reduced eNOS phosphorylation, impaired Ang-(1–7)-mediated vasodilation, and promoted hypertension.
Exosomes and vascular calcification
Vascular calcification (VC) is an important phenotype of vascular aging that manifests not only in EH but also in atherosclerosis, chronic kidney disease and diabetes mellitus (86). Under normal physiological conditions, VSMCs exhibit a contractile phenotype and regulate microenvironmental homeostasis by actively releasing exosomes carrying endogenous inhibitors of calcification, such as fetuin A, matrix Gla protein (MGP), osteoprotegerin (OPG) and bone morphogenetic protein-7 (BMP-7), thereby inhibiting the development of VC (87). However, in pathological states, factors related to osteogenic differentiation exert their effects. For example, lipopolysaccharide (LPS)-treated macrophage-derived EVs are enriched in proinflammatory cytokines and CAD (cis-aconitate decarboxylase), PAI-1 (plasminogen activator inhibitor-1), and Saa3 (serum amyloid A-3 protein), creating an inflammatory microenvironment for surrounding cells, and these exosomes significantly increase the calcification of VSMCs by increasing the expression of osteogenic markers (osterix and osteocalcin) and decreasing the expression of a contractile marker (alpha-smooth muscle actin) (88). The mechanism by which nicotine promotes VC in clinical patients was revealed by Petsophonsakul et al. (89), who demonstrated that nicotine-induced activation of α7 and α3 nicotinic acetylcholine receptor (nAChR) increased intracellular Ca2+ levels and initiated the calcification of VSMCs through increased NADPH oxidase 5 (Nox5) activity, leading to oxidative stress-mediated exosomes release. A positive feedback loop between Ca2+, Nox5 and ROS was established that promoted VC and correlated with the action of exosomes. Specifically, Nox5-mediated oxidative stress led to the excessive production of ROS, resulting in increased intracellular Ca2+ and the increased release of large amounts of EVs from VSMCs; these exosomes were shown to induce calcification of the extracellular matrix (ECM) and increase the calcification of recipient VSMCs. Ca2+ uptake by cells via exosomes or Ca2+ channels further contributed to an increase in Nox5 activity (90). Under hyperglycaemic conditions, EV miR-32 in macrophages was upregulated and delivered to VSMCs, promoting osteogenic differentiation and inhibiting autophagy in VSMCs via the miR-32/Mef2d/cGMP-PKG signaling pathway (91). Lipoprotein(a) stimulation led to an increase in the annexin level in the EV cargo of primary human smooth muscle cells (SMCs) and valvular interstitial cells (VICs), and these EVs readily aggregated in the collagen matrix and induced its mineralization, leading to the formation of microcalcifications that coalesced into macrocalcifications (92). Calcifying exosomes may be involved in the formation of ectopic microcalcifications in the ECM of human arterial walls. This possibility offers a new perspective that differs from the previous suggestion that exosomes entering VSMCs alter their phenotype. Notably, warfarin increased ER stress via the UPR-PERK-ATF4 pathway, which further induced Grp78 to be loaded into EVs as cargo and promoted exosome release (93). Deposition of EV Grp78 in the ECM and action of Grp78 on the surface of VSMCs, i.e., the promotion of calcium phosphate crystal formation on the collagen matrix, caused VSMC calcification (93). The traditional oral anticoagulant warfarin may promote VC, whilst inhibition of ER stress represents a new therapeutic possibility.
Exosomes may also improve VC in the context of pharmacological intervention or under other conditions. For example, curcumin (CUR) and exosomes secreted by VSMCs after CUR intervention attenuated VC. In vitro, CUR intervention resulted in high miR-92b-3p expression in both VSMCs and exosomes and delivery of miR-92b-3p to adjacent cells, significantly reducing expression of the transcription factor KLF4 and osteogenic factor RUNX2 in VSMCs; in addition, in a rat calcification model, CUR attenuated vitamin D3-induced VC by increasing miR-92b-3p expression and decreasing KLF4 expression in the aorta (94). Melatonin induced VSMCs to produce exosomes carrying miR-204 and miR-211, which inhibited BMP-2 expression in adjacent cells in a paracrine manner and attenuated VC and senescence (95). Mansour et al. (96) found that the GFOGER peptide may be a novel and promising therapeutic target for decreasing VC, as GFOGER peptide treatment modulated the EV protein composition and inhibited the expression of osteogenic markers (Runt-related transcription factor 2, the matrix-Gla protein, osteocalcin, and tissue non-specific ALP) in VSMCs. Exosomes secreted by MSCs pretreated with advanced glycation end product-bovine serum albumin (AGE-BSA) contained a high level of miR-146a, which was transferred to VSMCs and inhibited AGE-BSA-induced calcification in a thioredoxin-interacting protein (TXNIP)-dependent manner. Thus, miR-146a-carrying exosomes may be a potential therapeutic target in VC (97). AGE stimulation resulted in the enrichment of miR-126-5P in EC-derived exosomes, which were subsequently delivered to VSMCs where they downregulated BMPR1B expression, blocking the Smad1/5/9 signaling pathway and negatively regulating calcification in VSMCs (98). The potential role for miR-126-5P in improving media calcification in the vasculature has been suggested (98).
Exosomes and renal damage
Urinary albumin excretion (UAE) is an indicator of cardiovascular risk and renal damage in hypertensive patients, and Perez et al. (99) showed in an observational case–control study that plasma exosomal miR-126-3p and miR-26a-5p levels significantly differed between EH patients without UAE and healthy controls, and elevated plasma miR-126 levels were a marker of an increased risk of vascular injury and cardiovascular events and have potential value for early diagnosis. In addition, urinary and plasma exosomal miR-26a-5p and miR-222-3p levels were negatively correlated with UAE, and plasma exosomal miR-191-5p and miR-126-3p levels were positively correlated with the UAE rate in patients with EH plus UAE. MiR-26a-5p is an influential regulator of glomerular development and structure that protects kidney function in animal experiments (100), but its therapeutic value needs to be explored in depth. Riffo-Campos et al. (101) detected characteristic ncRNAs associated with hypertension-related UAE in urinary exosomes or plasma exosomes; among these ncRNAs were five lncRNAs (LINC02614, BAALC-AS1, FAM230B, LOC100505824, LINC01484) and miR-301a-3p, which play critical roles in regulating key pathways associated with filtration barrier integrity and tubular reabsorption and could be used to study the mechanisms of proteinuria and cardiovascular injury. Gonzalez et al. (102) studied urinary exosomal protein alterations in EH patients with renin-angiotensin system inhibition and found 21 proteins that may be associated with glycosaminoglycan degradation, coagulation and complement system abnormalities, and oxidative stress at 3 years of follow-up, and increases in urinary exosomal complement C3, complement C4a, and ceruloplasmin were predictive of de novo albuminuria in hypertensive patients.
Advances in understanding the role of exosomes in the pathophysiology of atherosclerosis
Atherosclerosis (AS) is a chronic inflammatory vascular disease caused by the accumulation of lipids in the vessel wall, which leads to plaque formation and narrowing of the vessel lumen (103). AS occurs in large and medium-sized arteries with high branching and turbulence, such as the common carotid and left coronary arteries, and is the pathological basis for many fatal cardiovascular diseases (104). During AS, changes in the phenotype of the vascular system occur, including EC dysfunction, VSMC proliferation and migration, inflammatory responses, and macrophage infiltration and polarization (105). Vascular interventions, such as angioplasty and vascular bypass grafting, are commonly used to correct coronary atherosclerotic disease. However, these vascular interventions may cause damage to the vessel wall (106), and these the transfer of exosomal miR-185 to ECs was found to impair reendothelialization after vascular injury by regulating the chemokine ligand 12/chemokine receptor 4 axis (CXCL12/CXCR4) axis (107), whereas exosomal miR-21-5p activated autophagy and promoted endothelial repair by inhibiting SIPA1L2 (signal-induced proliferation-associated 1 like 2) expression (108).
Exosomes and inflammation
Inflammation is a common mediator of many risk factors for AS, including levels of low-density lipoproteins and triglyceride-rich lipoproteins, as well as the altered behavior of cells of the artery wall. The ample preclinical implications of inflammation in AS have opened the door to many new therapeutic targets (109). Recent clinical trials have shown that targeting inflammation can reduce cardiovascular events even in individuals who have been treated with a full panel of effective standard therapies, with relevant anti-inflammatory drugs including canakinumab and colchicine (110, 111). Immune activation in AS concerns both innate and adaptive immunity, involving both immune cells and immune molecules (112). However, studies on intercellular exosome transfer have focused more on the relationship between innate immune cells and the vessel wall. In this section, we focus on the exosomes that cause arterial inflammation, which are mainly derived from inflammatory cells, ECs, and VSMCs.
Inflammatory cell-derived exosomes
The inflammatory response is a central part of AS, and the roles of inflammatory cells as donors or receptors of exosomes are worth exploring; several studies have confirmed that exosomes originating from inflammatory cells act on components of the vessel wall. Li et al. (113) showed that elevated levels of miR-185-3p in M1 macrophage-derived EVs increased lipid levels, inflammation, and oxidative stress in ApoE−/− mice, including increased levels of cholesterol (TC), triglyceride (TG), low density lipoprotein cholesterin (LDL-C), malonaldehyde (MDA), interleukin (IL)-1β, IL-17A, and IL-23 and decreased activities of glutathione peroxidase (GSH-PX), catalase (CAT), and superoxide dismutase (SOD). MiR-185-3p affected ECs, reduces small mothers against decapentaplegic 7 (Smad7) expression, inhibited EC proliferation, and exacerbated AS development in ApoE−/− mice. Other studies have shown that miR-185 induces oxidative stress-related EC death (114). Lin et al. (115) showed that the transfer of dendritic cell-derived exosomal miR-203-3p into bone marrow-derived macrophages (BMDMs) inhibited cathepsin S expression, reduced inflammatory responses, and attenuated AS progression in mice.
MiR-146a is a hallmark molecule in cardiovascular disease research, but its role in AS is controversial. Su et al. (116) showed that EVs secreted by macrophages in plaques that phagocytosed oxidized low-density lipoprotein (ox-LDL) had elevated levels of miR-146a and were delivered to macrophages to inhibit the expression of the target genes IGF2BP1 (insulin-like growth factor 2 mRNA-binding protein 1) and HuR (human antigen R or ELAV-like RNA-binding protein 1), reducing macrophage migration and promoting macrophage retention in the vessel wall. This study suggested that miR-146a in EVs accelerated the development of AS by reducing macrophage motility. However, conversely, it has been shown that miR-146a inhibits nuclear factor-κB (NF-κB) and MAPK signaling pathways by targeting TRAF6 (TNF receptor-associated factor 6), IRAK1/2 (interleukin 1 receptor-associated kinase 1 or 2), and HuR (117). Limiting the inflammatory response in various cells has been shown to reduce vascular senescence and can prevent and treat AS (118–120). It has also been shown that exosomes do not have the same effects in cells with the same receptor that are in different states. For instance, Zhong et al. (121) studied the effects of exosomal miR-146a secretion from dendritic cells. At 12 h after the first effects of exosomes from mature dendritic cells (mDC-Exos) on HUVECs were observed, the expression of adhesion molecules increased, including vascular cell adhesion molecule 1 (VCAM-1), ICAM-1 and E-selectin. Then, HUVECs were treated with mDC-Exos again for 6 h after a 24 h recovery period, and the HUVECs were shown to be resistant to the second stimulation after initial stimulation with mDC-Exos. Changes in miRNAs in HUVECs were detected, and a significant increase in miR-146a was observed. Ultimately, it was confirmed that DC-derived exosomes delivered miR-146a to HUVECs and that miR-146a protected HUVECs against secondary stimulation by inhibiting IRAK, suggesting that exosomes contribute to a negative feedback loop that regulates endothelial inflammation.
EC-derived exosomes
Exosomes secreted by ECs have regulatory effects on inflammatory cells. He et al. (122) showed that miR-155 was enriched in EVs released from ECs under ox-LDL induction and was subsequently transferred to THP1 cells, and these vesicles enhanced monocyte activation by shifting the monocyte/macrophage balance from the anti-inflammatory M2 type to the proinflammatory M1 type. KLF2 was found to regulate inflammation-associated miR-155 in ECs and in EVs derived from ECs. Mice that received EVs derived from KLF2-transfected ECs showed reduced AS lesions, reduced proinflammatory M1 macrophages, and increased anti-inflammatory M2 macrophages, which was at least partially caused by reduced expression of inflammation-associated miR-155. In contrast, EC-derived miR-92a could be transported to macrophages via EVs and reduce KLF4 levels, leading to the AS phenotype in macrophages and the formation of AS lesions (123). Wang et al. (124) demonstrated that miR-92a expression was upregulated through the ROCK/STAT3 (RhoA/Rho-associated coiledcoil forming kinase/signal transducer and activator of transcription 3) and MLCK (myosin light chain kinase)/STAT3 pathways in the AS disease state and that increased miR-92a levels reduced expression of the target gene KLF4, suggesting that the inhibition of miR-92a expression could improve vascular function. Xu et al. (125) showed that CD137-modified ECs were activated by CD137 signaling, significantly increased IL-6 levels in EC exosomes, and promoted Th17-cell responses by upregulating Akt/NF-κB signaling. High levels of IL-6 in EC-derived exosomes form a vicious cycle with the differentiation of CD4+ T cells toward Th17 cells, promoting the formation of AS plaques. Zhang et al. (126) infected HUVECs with an ET-1-overexpressing adenovirus (AdET-1) and observed that exosomal miR-33 levels produced by HUVECs transfected with AdET-1 were upregulated and directly affected macrophages, reducing nuclear receptor 4A (NR4A) expression and leading to proinflammatory macrophage gene activation. The harmful role of ET-1 in the development of AS is partly dependent on the regulation of macrophage polarization, which is mediated by the miR-33/NR4A axis.
VSMC-derived exosomes
The role of VSMCs in initiating AS and propagating lesion inflammation has been viewed as rather important, including through secretion of cytokines, acquisition of inflammatory cell-like characteristics, and foam cell formation (127). Calcium phosphate (CaP) particle deposition has been found in a number of inflammatory diseases, including AS, and small CaP particles are closely associated with VSMCs in atherosclerotic fibrous caps, affecting the proinflammatory signaling of VSMCs (128). CaP particles stimulated activation of spleen tyrosine kinase (SYK) and caspase-1 in VSMCs, leading to increased release of VSMC-derived exosomal IL-1β, highlighting the proinflammatory and pro-calcification potential of microcalcifications and VSMCs (128). Furthermore, EVs from senescent VSMCs could influence the cytokine milieu by modulating immune cell activity. Based on unbiased proteomic analysis of EVs derived from VMSCs, EVs released from senescent VSMCs induced secretion of IL-17, interferon-γ (INF-γ) and IL-10 by T cells and tumor necrosis factor-α (TNF-α) by monocytes; moreover, senescent EVs affected the differentiation of monocytes and favored mixed M1/M2 polarization with proinflammatory characteristics (129). Another study demonstrated that when VSMCs expressed more proprotein convertase subtilisin/kexin type 9 (PCSK9), the released EVs carried a proinflammatory phenotype, which reduced the migratory capacity of macrophages (130). Specifically, exposure to VSMC-EVs resulted in a significant increase in the gene expression of IL-6, VCAM-1, ET-1, ICAM-1 and E-selectin in ECs as well as a significant increase in the gene expression of CCL2 (MCP-1/chemokine (C-C motif) ligand 2), IL-1α, IL-1β, IL-6 and IL-8 in macrophages (130). Activation of ECs occurs during the initiation phase of AS, and understanding the effect of exosomes released from VSMCs on ECs may provide clues for AS treatment. VSMC-derived exosomes mediated the transfer of KLF5-induced miR-155 from VSMCs to ECs, which destroyed tight junctions and the integrity of endothelial barriers, leading to increased endothelial permeability and dysfunction (131). Blockage of the exosome-mediated transfer of miR-155 between these two cell types might serve as a therapeutic target for AS (131). The antihypertensive tripeptide Leu-Ser-Trp (LSW) could increase miRNA-145 loading in VSMC-derived EVs and attenuated ox-LDL-induced endothelial dysfunction via miRNA-145 delivery, which was internalized into ECs and downregulated PDCD4 (programmed cell death protein 4) expression (132).
Exosomes from other cells
Several studies have elucidated the possible cellular and molecular mechanisms by which dyslipidaemia promotes atherogenesis, which is associated with inflammation and EC activation. Xie et al. (133) proposed that exosomes from visceral adipose tissue in obese mice regulated the transformation and polarization of macrophage-derived foam cells, thereby promoting the progression of AS. KLF4 interacts with the coactivator p300 to regulate NF-κB activity and attenuate the expression of VCAM1 and plasminogen activator inhibitor-1 (PAI1), thereby inducing an anti-adhesive, anti-thrombotic state (134). Jiang et al. (135) showed that steatotic hepatocyte-derived EVs delivered miR-1 to ECs. MiR-1 downregulated KLF4 expression in ECs, leading to NF-κB activation and elevated expression of adhesion molecules in ECs, promoting endothelial inflammation and AS. Moreover, miR-1 has been shown to directly bind and inhibit KLF4 expression in SMCs (136). Chrysin attenuated the release of exosomal miR-92a and upregulated KLF2 expression in human coronary artery endothelial cells (HCAECs) to protect against AS (137). MiR-25-3p could also alleviate endothelial inflammation, as Yao et al. (138) showed that thrombin-induced peripheral platelet-derived exosomes (PLT-Exos) carrying miR-25-3p could be endocytosed by coronary vascular endothelial cells (CVECs) and downregulated α-smooth muscle actin by reducing Adam10 expression, inhibiting the TNF-α/NF-κB signaling pathway and collagen I a1, collagen III a1, triglyceride, total cholesterol, IL-1β, IL-6, and TNF-α levels and thereby inhibiting ox-LDL-induced inflammation and lipid deposition in CVECs. There is also a correlation between the status of donor cells and the function of the exosomes they release. Liu et al. (139, 140) suggested that perivascular adipose tissue (PVAT)-derived exosomal miR-382-5p reduced the formation of macrophage foam cells, which could be protective against AS. Exosomal miR-382-5p downregulated the expression of SR-A (scavenger receptor A) to reduce cholesterol uptake in macrophages. In addition, the overexpression of ABCA1 (ATP-binding cassette transporter A1) or ABGA1 (ATP-binding cassette transporter G1) was induced to promote cholesterol efflux. This antiatherogenic effect might be mediated by the upstream regulation of PPARγ (peroxisome proliferator-activated receptor γ). The use of PVAT-derived exosomes as a promising preventative and therapeutic strategy for AS warrants further investigation. In contrast, PVAT exosomal miR-382-5p levels were reduced in patients with coronary atherosclerotic heart disease (140). However, in obese and diabetic conditions, PVAT was shown to be dysfunctional and lose its protective effect, instead secreting proinflammatory adipokines that induce endothelial dysfunction and inflammatory cell infiltration, thus promoting AS (141, 142).
Exosomes and cell migration
The presence of a substantial number of intimal VSMCs, such as those involved in the formation of fibrous caps, has been suggested as evidence that VSMC migration and proliferation from the media play an important role in atherogenesis (143). Burtenshaw et al. found that phosphorylation of ERK and Akt was increased in VSMCs treated with foam cell-derived EVs (FC-EVs) and stimulated VSMC migration, and the researchers speculated by proteomic analysis that proteins in EVs might have played a role in this effect (144). In addition, FC-EVs could transfer integrins β1 and α5 to the surface of VSMCs and promote cell adhesion (144). Macrophage-derived EVs containing miR-19b-3p accelerated the migration and promotion of VSMCs by targeting JAZF1, which promoted the development of AS lesions in ApoE−/− mice (145). Zhu et al. (136) showed that increased levels of exosomal miR-21-3p in nicotine-treated macrophages were transmitted to VSMCs and accelerated AS progression. MiR-21-3p binding sites were present in the 3′-UTR of PTEN, and miR-21-3p promoted the migration and proliferation of VSMCs by inhibiting PTEN. Ren et al. (146) showed that M1 macrophage-derived exosomal miR-186-5p levels were increased in response to ox-LDL stimulation and acted on VSMCs; miR-186-5p downregulated SHIP2 expression, enhancing cell viability, invasiveness, and the phosphatidylinositol-3-kinase/protein kinase B/mammalian target of rapamycin (PI3K/Akt/mTOR) pathway. Inhibition of the mTOR pathway has been shown to alleviate plaque progression and play a protective role against AS (147). Other researchers identified that miR-222 originating from M1 macrophage-derived exosomes triggered functional changes in VSMCs and confirmed that miR-222 played a key role in promoting VSMC proliferation and migration by targeting cyclin-dependent kinase inhibitor 1B (CDKN1B) and cyclin-dependent kinase inhibitor 1C (CDKN1C) in vitro (148). Li et al. (149) demonstrated that inflammatory CD137 signaling decreased the anti-inflammatory effects of ten-eleven translocation 2 (TET2) transfer from ECs to VSMCs, thereby accelerating VSMC proliferation and migration in vitro and neointimal formation in vivo. PVAT was shown to produce EVs containing miR-221-3p, which are subsequently taken up by neighboring VSMCs, leading to dramatically enhanced VSMC proliferation and migration (150). Mechanistically, EV-mediated miRNA-221-3p transport suppressed the downstream target PGC-1α (peroxisome proliferator–activated receptor γ coactivator 1α), a known metabolic modulator of VSMCs (150).
Co-incubation of oxidized low-density lipoprotein (ox-LDL) with human mononuclear cell lines (THP-1) can model foam cells in AS pathology. In vitro, exosomal lncRNA LIPCAR could be transferred from ox-LDL-treated THP-1 cells to VSMCs, resulting in the upregulation of CDK2 and PCNA, which remarkably promoted the viability and migration of VSMCs (151). Another study showed that miR-106a-3p was increased in exosomes from ox-LDL-induced THP-1 cells and could promote cell proliferation and repress cell apoptosis of VSMCs (152). It was proposed that exosome-derived miR-106a-3p could directly bind CASP9 and repress the caspase signaling pathway in VSMCs (152). In contrast, studies suggesting that exosomes may inhibit VSMC migration are uncommon. Exosomal LINC01005 from ox-LDL-treated ECs promoted VSMC phenotype switching, proliferation, and migration by regulating the miR-128-3p/KLF4 axis (153). KLF4 silencing and miR-128-3p mimic alone abrogated the ox-LDL-Exo-mediated promotion of VSMC phenotype switching, proliferation, and migration (153).
Exosomes, plaque instability and thrombosis
Hyperglycaemia is known to increase haemopoiesis in the bone marrow, thereby increasing the levels of monocytes and neutrophils, which readily enter the lesions of AS-susceptible mice and increase the burden on macrophages, accelerating AS (154). Compared to nondiabetic AS plaques, diabetic plaques are prone to becoming vulnerable AS plaques with abundant foam cells, larger necrotic core sizes, a greater extent of SMC, higher levels of macrophage apoptosis, and increased infiltration of inflammatory cells (155). Bouchareychas et al. (156) showed that exosomes from BMDMs exposed to high concentrations of glucose increased the number of hematopoietic and myeloid cells in the circulation of ApoE−/− mice, leading to an increase in the number of macrophages in the lesion, as well as an increase in the area of apoptosis and AS progression. The mechanism involved the delivery of miR-486-5p via BMDM-derived exosomes to macrophages and a significant reduction in ABCA1 mRNA expression in macrophages. Liu et al. (157) also demonstrated that miR-486-5p reduced ABCA1 expression in THP-1 macrophages. Wang et al. (158) showed that insulin-resistant adipocytes increased the vulnerability of aortic plaques in diabetic ApoE−/− mice by secreting exosomes containing sonic hedgehog (shh). The factor shh activated intracellular pathways by binding the Patched (Ptch) receptor on recipient cells (HUVECs and murine aortic endothelial cells), increasing the expression levels of TNF-α, IL-1β, IL-6, vascular endothelial growth factor A (VEGF-A), ICAM-1, matrix metalloproteinase-2 (MMP2), and MMP9 in plaques and promoting vasa vasorum (VV) angiogenesis. The VV is an important entry pathway for immune cells, promoting the development of chronic inflammatory and necrotic cores and leading to plaque instability (159).
VSMC necrosis and apoptosis play progressive roles in plaque instability and AS lesions, and the migration of VSMCs accelerates AS progression despite the possible role of these cells in stabilizing the fibrous cap (160). Wang et al. (161) showed that macrophage-derived EVs carrying miR-503-5p inhibited the proliferation, migration and angiogenesis of HCAECs while promoting the proliferation and migration of human coronary artery smooth muscle cells (HCASMCs) by downregulating Smad7, smurf1, and smurf2 and elevating TGF-β1, thereby exacerbating AS. In VSMCs, exosomal miR-223, miR-339, and miR-21 from thrombin-activated platelets inhibited the proliferation of VSMCs by reducing the expression of PDGF receptors. Because reduced PDGF receptor expression was also observed in VSMCs surrounding thrombotic areas in vivo, these miRNAs may be biomarkers for predicting atherosclerotic thrombosis (162). Another study showed that platelet-derived exosomes inhibited thrombogenesis in AS by reducing macrophage CD36-dependent lipid loading and inhibiting platelet aggregation (163).
Advances in understanding the role of exosomes in the pathophysiology of pulmonary arterial hypertension
Pulmonary arterial hypertension (PAH), which is predominant in women and is defined as a resting mean pulmonary artery pressure (mPAP) greater than or equal to 25 mmHg, is a chronic and progressive cardiopulmonary disease associated with persistent perivascular inflammation, vascular remodeling and right heart failure (164–166). The activation of complement, particularly through alternative pathways, plays a key role in the early inflammatory and proliferative response in PAH (167). Irreversible remodeling of the pulmonary vascular bed is a major cause of increased mean pulmonary arterial pressure (mPAP) in PAH, and right ventricular function is a major determinant of clinical outcomes and survival in patients with PAH (168, 169). Pulmonary artery plexiform lesions are characteristic of PAH and are characterized by marked capillary hyperplasia (170). Nongenetic abnormalities associated with pulmonary hypertension include endothelial dysfunction, SMC metabolic reprogramming, perivascular inflammation, platelet and coagulation dysfunction, and extracellular matrix remodeling (168, 171–174). The pathogenesis of PAH is also associated with mutations in components of the BMP pathway, including bone morphogenetic protein receptor 2 (BMPR2), BMP9, ACVRL1, ENG, or SMAD9 (175–177), among others. Impaired BMPR2 signaling can lead to accelerated cell proliferation and mitochondrial dysfunction and promote PAH (178–180). Mutations in the BMPR2 gene are strongly associated with the development of familial PAH (FPAH) and idiopathic PAH (IPAH) (181, 182), and PAH patients with BMPR2 mutations typically have a younger onset, exhibit increased disease severity and elevated mPAP and pulmonary vascular resistance, have a poorer response to acute vasodilatory testing and are at greater risk of death (183).
Exosomes and the BMP pathway
There is proof that BMPR2 is closely linked to miRNAs in the pathological alterations associated with PAH. A decline in BMPR-2 signaling in patients with hereditary PAH (HPAH) was shown to cause a decrease in miR-27 in ECs and an increase in translationally controlled tumor protein (TCTP) expression, resulting in EC resistance to apoptosis in patients with HPAH (184). In IPAH patients and animal models of PAH, the BMPR2 protein may be decreased even when the gene is normally expressed, indicating that the inhibition of BMPR2 transcription by miRNAs plays an important role in the pathogenesis of PAH. The depletion of miR-20a could restore BMPR2 signaling and attenuate the development of hypoxic PAH (185). IPAH patients with BMPR2 mutations have significantly more exosomes released from blood outgrowth ECs (BOECs) and contain more TCTP, which is transferred to pulmonary arterial smooth muscle cells (PASMCs), decreases caspase-3 activity and contributes to cell resistance to apoptosis (186). TCTP has potential value for diagnosing or assessing the severity of PAH. Zhang et al. (187) showed that exosomal miR-191 released from adipose-derived mesenchymal stem cells (ASCs) regulated the proliferation of pulmonary artery endothelial cells (PAECs) in vitro, and higher miR-191 levels were associated with faster PAEC proliferation and vice versa. In addition, miR-191 overexpression has been shown to be associated with PAH (188). MiR-191 antagonists reduced right ventricular systolic pressure (RVSP), increased BMPR2 levels in MCT-PAH rats and relieved PAH. The miR-191 inhibitor may have potential therapeutic value by preventing BMPR2 degradation to improve PAH. Zhang et al. (189) treated MCT-induced PAH mice with of MSC-derived exosomes via tail vein injection for 3 weeks and concluded that MSC-derived exosomes significantly reduced RVSP and the right ventricular hypertrophy index and attenuated pulmonary vascular remodeling and pulmonary fibrosis in vivo. In vivo and in vitro experiments confirmed that MSC-derived exosomes could regulate the Wnt5a/BMP signaling pathway in PAECs and PASMCs, including upregulating the expression of Wnt5a, Wnt11, BMPR2, BMP4, and BMP9 and downregulating the expression of β-catenin, cyclin D1, and TGF-β1.
Exosomes and cancer-like metabolism
In PAH and cancer, mitochondrial metabolism and redox signaling are reversibly disordered, creating a pseudohypoxic redox state characterized by normoxic decreases in ROS, a shift from oxidative to glycolytic metabolism, and hypoxia-inducible factor-1α (HIF-1α) activation (190, 191), which lead to sustained ATP production, uncontrolled cell growth and cellular resistance to apoptosis (192–194). Kumar et al. (195) showed that AFs obtained from humans with PAH and calves with hypoxia-induced PAH secreted exosomes containing complement C3, which mediated BMDM activation toward a proinflammatory and metabolically altered phenotype in vitro, and the latter was characterized by increased aerobic glycolysis and the accumulation of succinic acid. Mitochondrial dysfunction in PAH might cause alterations in circulating exosomes, i.e., low expression of the proliferator-activated receptors gamma-coactivator 1-alpha and sirtuin 1 in exosomes, and circulating exosomes affect on other organs, such as the brain (196). Researchers observed upregulated expression of manganese SOD, downregulated expression of HIF-1α and the preservation of antioxidant enzyme expression in the brain, explaining the potential role of exosomes in PAH and antioxidant defense mechanisms in protecting cells from disease progression (196, 197). As hypoxia exposure increases, sirtuin 4 (SIRT4) gene expression also increases, and Hogan et al. (198) showed that the administration of MSC-secreted exosomes to PASMCs inhibited SIRT4 and pyruvate dehydrogenase kinase 4 (PDK4) expression, upregulated pyruvate dehydrogenase (PDH) and glutamate dehydrogenase 1 (GLUD1) expression levels, allowed glutamine and pyruvate to enter the TCA cycle and improved mitochondrial dysfunction associated with PAH. Other studies have also suggested that some exosomes may contain mitochondrial material (38, 199), but their association with PAH-related cancer-like metabolism remains to be further investigated.
Exosomes and cell proliferation
Intercellular communication between ECs and other components of the pulmonary vascular wall is a critical signature of PAH pathogenesis (168). In an in vitro healing assay, Deng et al. (200) demonstrated the involvement of miR-143 in intercellular communication between PASMCs and PAECs, as miR-143 induced the migration of PAECs and reduced the death of PAECs. In a mouse hypoxia model, miR-143-3p expression was upregulated in the whole lung and right ventricle. Furthermore, histopathological and haemodynamic data collected under hypoxic conditions showed significantly reduced pulmonary vascular remodeling in miR-143−/− mice compared to wild-type mice. The expression levels of miR-143-3p and miR-143-5p were also significantly upregulated in PASMCs from PAH patients compared to healthy controls. Anti-miR-143 treatment reversed hypoxia-induced RVSP elevation and right ventricular hypertrophy and reduced vascular remodeling in mice. Zhang et al. (201) showed that hypoxia increased the secretion of exosomes from PAECs to exert their proliferative effects on recipient PAECs in a paracrine or autocrine manner, which was attributed at least partially to the enrichment of lipid-peroxidizing enzyme 15-lipoxygenase2 (15-LO2) in exosomes, which then activated the STAT3 signaling pathway. Aliotta et al. (202) showed that exosomal miRNAs that were commonly increased in MCT-PAH mice and IPAH patients included the miR-17-92 cluster, miR-21 and miR-145. Among them, the miR-17-92 cluster (including miR-17, miR-18a, miR-19a, miR-20a, miR-19b-1, and miR-92-1) is a well-characterized family of miRNAs that are known to play an important role in the regulation of cell proliferation and apoptosis (202). Khandagale et al. (203) confirmed that the expression of miR-486-5p and miR-26a-5p was altered in circulating exosomes from the plasma of PAH patients and found that overexpression of miR-486-5p and downregulation of miR-26a-5p induced the proliferation of PAECs as well as the transcription and protein expression of VEGF, partly by targeting NF-κB signaling. However, some studies on myocardial infarction and cancer have shown that PTEN, PIK3R1, and MMPs may be downstream target genes of miR-26a and miR-486-5p (204–207).
Uncontrolled EC proliferation is the main contributor to the characteristic plexiform lesions of PAH, but it is undeniable that medial thickening caused by SMC proliferation is also involved in vascular remodeling in PAH (208); however, there have been few relevant exosomal studies. In vitro, miR-181 and miR-324 in exosomes released by KLF2-overexpressing PAECs were found to be elevated and transmitted to PASMCs, in which they alleviated hypoxia and platelet-derived growth factor (PDGF)-induced cell proliferation (209). MiR-181a-5p and miR-324-5p act together to attenuate pulmonary vascular remodeling, and their actions are mediated by Notch4, ETS1 and other key regulators of vascular homoeostasis (209). Researchers have demonstrated that therapeutic supplementation with miR-181a-5p and miR-324-5p reduced proliferative and angiogenic responses in patient-derived cells and attenuated disease progression in PAH mice (209). Zhang et al. (210) identified a role for the HIF-1-let-7b-ACE2 axis: miR-let-7b expression was elevated in rat lungs under chronic hypoxic conditions, and in vitro and in vivo experiments showed that let-7b promoted PAH by inhibiting ACE2 expression and thus inducing PASMC proliferation and migration. Nevertheless, in contrast to the above, Wang et al. (211) concluded that let-7b-5p, miR-92b-3p and miR-100-5p in human neural stem cell line (ReNcell)-derived EVs reduced vascular remodeling and RVSP in Su/Hx mice. Chen et al. (212) found that PVEC-derived EVs contained both miR-210-3P, which promoted the proliferation of PASMCs, and miR-3,057-5P, 212-5P and 34C-3P, which inhibited the proliferation of PASMCs; however, it is noteworthy that these EVs are more likely to be microvesicles than exosomes.
Pulmonary vascular ECs in PAH release specific populations of exosomes and can induce PAH alterations in healthy lungs; a positive feedback loop that propagates vascular remodeling may exist, and although it is difficult to completely reverse PAH (202), MSC-derived exosomes provide hope. Aliotta et al. (202) showed that miRNAs isolated from MSC-derived exosomes had increased levels of anti-proliferative and anti-inflammatory miRNAs, including miRs-34a, −122, −124, and −127. Other studies confirmed that MSC-derived exosomes or EVs could improve PAH by inhibiting cell proliferation and inflammatory responses. Lee et al. (213) showed that intravenous injection of MSC-derived exosomes inhibited vascular remodeling and hypoxic pulmonary hypertension. Mechanistically, MSC-derived exosomes suppressed the hypoxic activation of STAT3 and the upregulation of the miR-17 superfamily of microRNA clusters, whereas they increased lung levels of miR-204, which had pleiotropic protective effects on the lung and suppressed PAH by inhibiting the hyperproliferative pathway. MSC-derived EVs also induced macrophage polarization toward the M2 type and partially inhibited the inflammatory response of macrophages (134). Klinger et al. (214) studied a Sugen5416/hypoxia-induced PAH rat model and showed that MSC-EVs reduced macrophage recruitment, promoted a shift from an inflammatory to a reparative macrophage phenotype, promoted angiogenesis, and reduced the expression of inflammatory cytokines such as IL-6, macrophage inflammatory protein 2 (MIP-2) and TNF-α. The researchers (214) also noted that miR-196b levels were hundreds of times higher in MSC-EVs than in fibroblast-derived EVs, that HOXA5 expression was increased in PAH patients and that miR-196b reduced HOXA5 expression (215, 216). However, other studies have shown that HOXA5 can inhibit the release of inflammatory factors from macrophages (217). Therefore, it is unclear whether miR-196b plays a role in the reversal of PAH mediated by MSC-EVs. Several reports on lung injury have shown that MSC-EVs reduced macrophage recruitment in lung tissue, reduced macrophage expression of proinflammatory cytokines associated with the development of PAH, such as IL-6, MIP-2, and TNF-α, limited alveolar injury in animal models of acute lung injury and attenuated PAH in animal models of bronchopulmonary dysplasia (134, 214). In addition, Li et al. (218) showed that miR-150 prevented hypoxia-induced pulmonary vascular remodeling, fibrosis, and abnormal proliferation in PASMCs and PAECs.
Engineered exosomes
As described above, multiple studies have shown that MSC-derived exosomes or EVs can alleviate vascular remodeling. EVs can be isolated from MSCs from various sources and can be stored efficiently, safely and easily. MSC-EVs are now known to have substantial therapeutic benefits in a range of animal disease models, and some effects have been clearly shown to be as potent as those observed in response to whole-cell MSC administration (219). Researchers have acknowledged the therapeutic value of exosomes; however, there are some challenges and confusion regarding the use of natural exosomes in disease treatment, including (220) (1) how to determine and optimize the route of administration, biodistribution, and potential toxicity of exosomes; (2) how to obtain a more comprehensive understanding of the characteristics of exosome contents to clarify the formulation and dosing regimen when using them; and (3) how to improve the targeting and accumulation of exosomes in specific organs and tissues. As a result, engineered exosomes have emerged. In addition to stem cells, cancer cells and HEK293T cells are often used as donor cells for engineered exosomes. Cancer cell-derived exosomes may have some tropism for tumors and are therefore mostly used in the field of tumor therapy (221, 222). HEK293T cells release exosomes that are almost completely nontoxic and free of immunogenicity, and the safety and efficacy of the HEK293T cell exosome drug delivery platform have been demonstrated (223, 224). Other studies have used exosomes from blood (plasma or serum), milk or plants, so the identity of the donor cells is not clear. Engineered exosomes are mainly modified by exosome content modification and exosome membrane surface modifications, which improve biological potency and targeting, enable trajectory monitoring and reduce nonspecific uptake (49) (Figure 3).
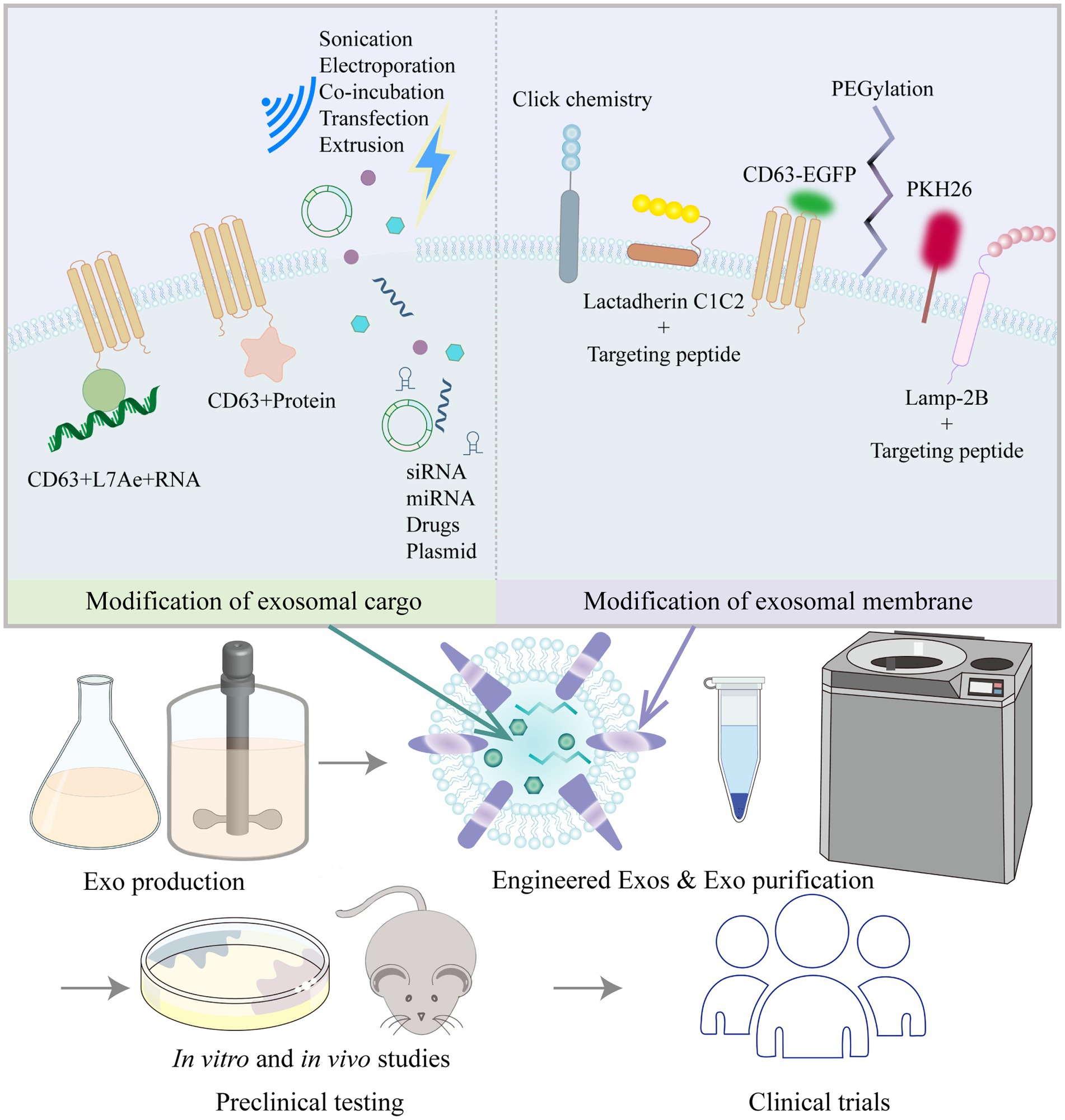
Figure 3. Common strategies to modify exosomes (Exos) and process of moving Exos toward clinical application.
Exosome cargo modification is mainly performed to improve the biological potency of exosomes by loading them with active pharmaceutical ingredients before or after exosome isolation. Prior to exosome isolation, active packaging is mostly used to package target delivery molecules into exosomes by the transfection of target genes into cells via plasmids or by coexpression with exosomal membrane proteins (LAMP-2B, CD9, CD63, CD81, etc.). Chen et al. (225) transfected Lv-miR-125b into BMSCs and then collected and purified the exosomes. BMSC-Exos-miR-125b (50 μg) were injected adjacent to the coronary ligation zone in a rat model of myocardial ischaemia–reperfusion injury, which significantly increased cell viability, decreased the apoptotic ratio, downregulated Bax and caspase-3 levels, upregulated Bcl-2 expression, and decreased IL-1β, IL-6, TNF-α, and SIRT7 levels. Han et al. (226) transfected a miR-675 mimic into human umbilical cord MSCs, then isolated the exosomes and applied a silk fibroin hydrogel to encapsulate the exosomes. Laser Doppler perfusion imaging showed that delivery of miR-675-overexpressing engineered exosomes via a silk fibroin hydrogel improved blood perfusion in the ischaemic hind limb of senescent mice. By means of active packaging, it was possible to successfully load not only small molecules such as miRNAs, agomirs and antagomirs to the exosome cargo but also large molecules such as mRNAs and proteins. Kojima et al. (227) constructed the EXOtic device using HEK293T cells as exosome-producing cells. The researchers cotransfected the potential RNA packaging device (CD63 + archaeal ribosomal protein L7Ae + catalase mRNA) and a cytosolic delivery helper (connexin 43 S368A mutant) together with the exosome production booster and a reporter coding for encoding nluc mRNA bearing C/Dbox(es) in its 3′-UTR, as well as the targeting module RVG-Lamp2b, which was reported to target exosomes to the brain by binding the nicotinic acetylcholine receptor (CHRNA7). By analyzing the expression levels of several markers (GFAP, Iba1, TNFα, CD11b), the engineered exosomes containing peroxidase were shown to attenuate 6-hydroxydopamine (6-OHDA)-induced neuroinflammation in mice, providing a new strategy for the treatment of Parkinson’s disease.
After the isolation of exosomes, passive packaging approaches have been mostly used to package target delivery molecules into exosomes by electroporation, sonication, co-incubation, extrusion, and freeze–thaw cycles, etc. Through passive packaging approaches, some studies have successfully added small molecules such as siRNAs, miRNAs or drugs to exosome cargoes. Zhao et al. (228) used incubation and extrusion to load siS100A4 into exosomes, which were designed to have high affinity for the lung and exert prominent gene silencing effects, significantly inhibiting lung metastasis in a mouse model of postoperative breast cancer tumor growth. Sun et al. (229) isolated exosomes from plasma and introduced miRNA-21 analogs into the exosomes by electroporation. The modified exosomes were then injected via the tail vein into a doxorubicin-induced cardiotoxicity mouse model, and ultrasound-targeted microbubble disruption was used to facilitate exosome delivery to the cardiac region. Echocardiography confirmed that exosomal miRNA-21 delivery improved doxorubicin-induced cardiac dysfunction. Kim et al. (230) carried out in vivo trials of non-small cell lung cancer treatment by loading paclitaxel into macrophage-derived exosomes by ultrasound treatment. Kalani et al. (231) loaded CUR into embryonic stem cell exosomes (MESC-Exos) by repeated rapid freeze–thawing. After intranasal administration, the modified exosomes alleviated inflammation, reduced astrocytic GFAP expression, and reduced neuronal NeuN expression in the cerebral cortex and hippocampus of mice with middle cerebral artery ischaemia–reperfusion injury. Qu et al. (232) applied saturated solution incubation to load dopamine into blood exosomes, and the modified exosomes could transport drugs across the blood–brain barrier via transferrin receptor-mediated endocytosis, resulting in a more than 15-fold increase in brain distribution of dopamine, which is important for the targeted treatment of Parkinson’s disease and other central nervous system disorders.
Exosomal membrane surfaces have been modified in a variety of ways, mainly for the purpose of improving targeting and tracking. Xu et al. (233) fused MSC-binding peptide E7 to the exosomal membrane protein Lamp-2b, giving exosomes the ability to target synovial fluid-derived MSCs. KGN delivered by these modified exosomes was able to efficiently enter SF-MSCs and strongly induce chondrogenic differentiation while protecting chondrocytes from degeneration. Wang et al. (234) genetically modified the parent cells of EVs by fusing the ischaemic myocardium-targeting peptide CSTSMLKAC with the EV membrane protein Lamp-2b to enhance the specificity and efficiency of EVs in targeting the ischaemic myocardium. Tian et al. (235) isolated EVs from a human neural progenitor cell line and constructed a recombinant fusion protein with neurological targeting properties on EV membranes: an arginine-glycine-aspartate (RGD)-4C peptide (ACDCRGDCFC) fused to the phosphatidylserine (PS)-binding domain of lactamycin-C1C2. For in vivo validation in middle cerebral artery occlusion (MCAO) mice, RGD-C1C2-binding EVs (RGD-EVs) were injected via the tail vein, and the modified EVs were shown to effectively target ischaemic brain lesions and strongly inhibit the inflammatory response. Polyethylene glycol modification increased the circulation time of exosomes and reduced their uptake by nonspecific cells (236). Aminoethylbenzamide-polyethylene glycol-modified exosomes (AA-PEG-exoPTX) carrying paclitaxel (PTX) had high drug loading and strong accumulation capacity in cancer cells after systemic administration, showing high anticancer efficacy in a mouse model of metastatic lung cancer (230). Different techniques for exosome labeling include fluorescent labeling, bioluminescent labeling and radioisotope labeling. Lipophilic dyes include Exoria, PKH67, PKH26, carbocyanine dyes (DiI, DiO), FM4-64, and others (237–239). Each dye has its own characteristics; for example, PKH-26 has a long half-life (238), and Exoria is suitable for labeling MSC-EVs (237). Fluorescent dyes are embedded in the bilayers of exosomes with noncovalent bonds; however, these dyes can form aggregates or micelles in aqueous solutions in similar proportions to exosomes, which may give misleading information in absorption experiments (240). Targeted metabolic labeling techniques and click chemistry approaches can likewise aid exosome tracing. Angiogenesis is characterized by the over-expresses ανβ3 integrin, which plays a key role in endothelial cell survival, migration and angiogenic growth, and ανβ3 integrin can be specifically bound by a peptide containing the RGD sequence. RGD-labeled exosomes containing unnatural monosaccharide derivatives (Ac4ManNAz) were delivered into HUVECs in a receptor-mediated manner, which revealed that unnatural sialic acids with azide groups were artificially generated on the cell surface. The fluorescent dye DBCO-Cy3 (dibenzylcyclooctyne-cyanine3) was strongly and specifically bound to the unnatural sialic acids outside of the cell surface by copper-free click chemistry for glycan imaging (241, 242). Click chemistry has also been used to improve exosome targeting, and thiol-maleimide cross-linking is one of the most classical and established click chemistry methods. To allow prostate cancer cells to efficiently recognize and internalize exosomes, Han et al. (243) used the thiol-maleimide cross-linking reaction to generate E3 aptamer-PEG-cholesterol adducts to modify the exosome surface. In addition, labeling could be achieved by generating CD63EGFP fusion proteins, such as by cotransfecting stable cell lines using the PCL6-CD63EGFP plasmid, which resulted in CD63-EGFP fusion protein-tagged exosomes, were then analyzed by serial two-photon tomography (STP) and anti-EGFP antibody staining (244, 245). Among the bioluminescent markers, exosomal surface-expressed reporter proteins such as Gaussia luciferase, firefly luciferase or kidneyworm luciferase produced bioluminescence when their substrates, such as cecropin (CTZ), were added (246). Radioisotope labels such as 131I, 99mTc, and 111In-oxine are often used to label exosomes and allow the imaging of deeper tissues due to their tissue penetration potential, and these labels are commonly used for assessing exosome biodistribution in isolated organs (247, 248).
Preclinical studies and clinical trials involving exosomes or EVs continue to emerge. According to a systematic review in 2021, more than half of preclinical studies on EV-delivered drugs in the last decade have focused on cancer therapy, and 12.7% involved cardiovascular disease (247). We searched ‘exosome’ or ‘extracellular vesicle’ at https://www.clinicaltrials.gov, and 191 related trials are active and recruiting. However, it is important to note that there is a lack of quality control standards, as well as evaluation criteria for the use of engineered exosomes for drug delivery or disease treatment (249).
Conclusion
Exosomes are widely involved in the development and progression of EH, AS and PAH and play an influential role in vascular remodeling. Exosomal miRNAs hold particular promise for the development of new tools for the diagnosis and treatment of vascular diseases.
Clinical samples from diseased and healthy groups are sources of exosomes, and abnormally increased and decreased biomolecules are screened based on databases or by high-throughput sequencing and proteomics analysis. Such studies are common and have identified valuable markers through the validation of clinical specimens. For example, low plasma levels of exosomal miR-150 (27) and miR-483 (250) and high levels of miR-596 (251) in patients with PAH suggested a poor prognosis; DQ593039 correlated significantly with diagnostic parameters in patients with CTEPH, and this piRNA showed potential value as a noninvasive screening agent (252). In practice, however, we do not know the donor cells, recipient cells, or specific mechanisms of abnormal exosomes in these disease groups, so further studies may be needed to elucidate the mechanisms of these markers in molecular, cellular, and animal models. This review has summarized the most recent and well-documented studies (Table 2 and Figure 4), which are fundamental to our understanding of exosomes, the development of vascular diseases, and the exploration of potential therapeutic approaches. Only by understanding the roles of these markers can we accurately use exosomes for disease diagnosis and monitoring.
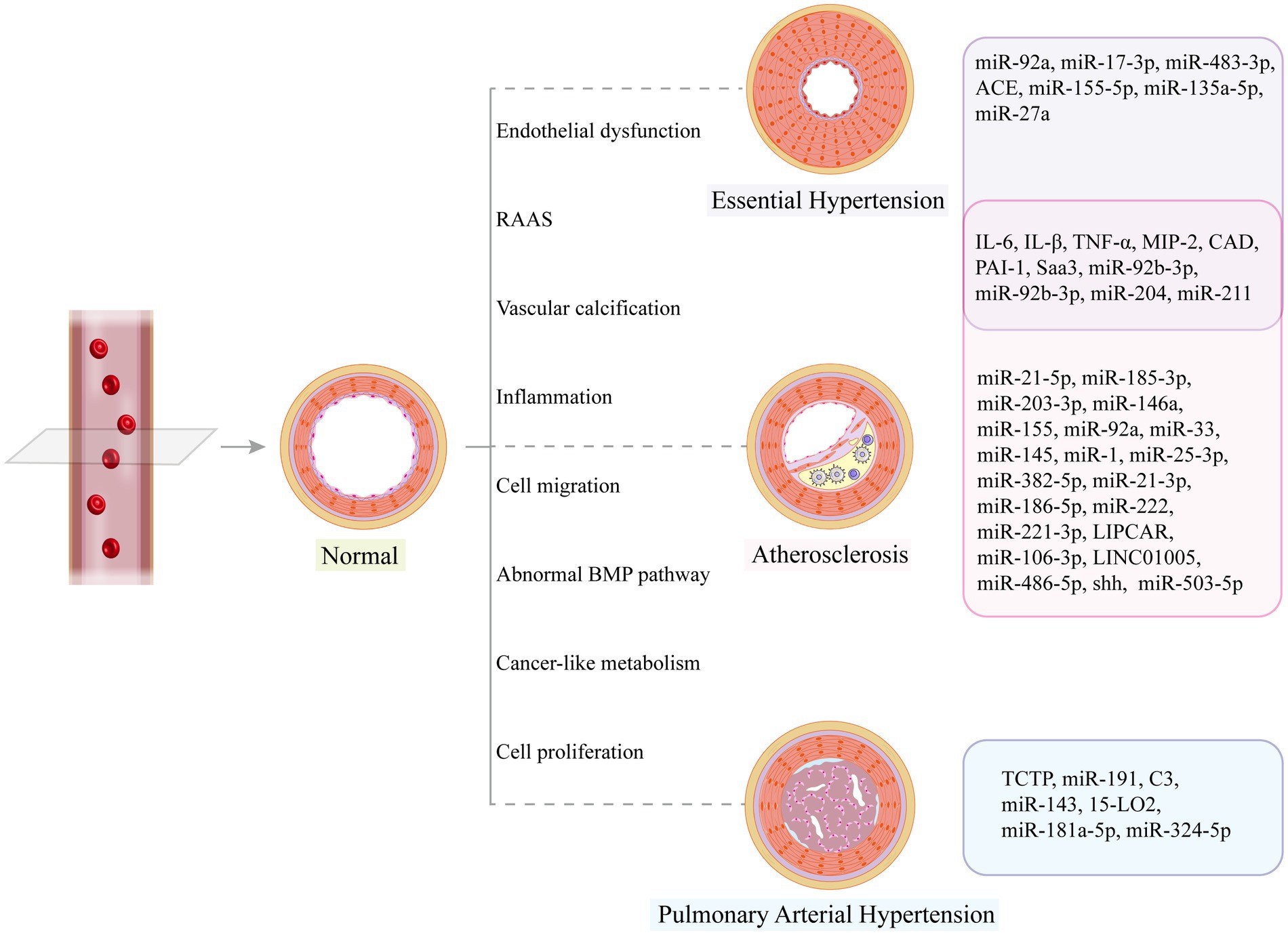
Figure 4. Vascular structural changes may occur in disease states, and related exosome cargos can promote or alleviate vascular remodeling.
Stem cell therapy has become a promising strategy for the treatment of vascular diseases, yet stem cell transplantation is often complicated by graft-versus-host problems (55, 253, 254). Paracrine signaling via exosomes can take advantage of stem cell therapy while avoiding graft-induced complications (255), and the reversal of arterial muscularization and ventricular hypertrophy via exosomes has been reported (213). However, providing a safe and effective targeted therapeutic approach still requires further evidence of the feasibility and positive effects of exosomes in the treatment of vascular-related diseases.
Author contributions
YR wrote the manuscript and produced the figures. HZ reviewed the manuscript. All authors contributed to the article and approved the submitted version.
Funding
This work was supported by CAMS Initiative for Innovative Medicine (CAMS-I2M) [2016-I2M-3-006].
Acknowledgments
The authors would like to thank AJE Customer Service (www.aje.cn/services/) for the English editing and review services.
Conflict of interest
The authors declare that the research was conducted in the absence of any commercial or financial relationships that could be construed as a potential conflict of interest.
Publisher’s note
All claims expressed in this article are solely those of the authors and do not necessarily represent those of their affiliated organizations, or those of the publisher, the editors and the reviewers. Any product that may be evaluated in this article, or claim that may be made by its manufacturer, is not guaranteed or endorsed by the publisher.
References
1.Smith, SC, Collins, A, Ferrari, R, Holmes, DR, Logstrup, S, McGhie, DV, et al. Our time: a call to save preventable death from cardiovascular disease (heart disease and stroke). Eur Heart J. (2012) 33:2910–6. doi: 10.1093/eurheartj/ehs313
2.Yusuf, S, Joseph, P, Rangarajan, S, Islam, S, Mente, A, Hystad, P, et al. Modifiable risk factors, cardiovascular disease, and mortality in 155 722 individuals from 21 high-income, middle-income, and low-income countries (PURE): a prospective cohort study. Lancet. (2020) 395:795–808. doi: 10.1016/S0140-6736(19)32008-2
3.Roth, GA, Johnson, C, Abajobir, A, Abd-Allah, F, Abera, SF, Abyu, G, et al. Global, regional, and national burden of cardiovascular diseases for 10 causes, 1990 to 2015. J Am Coll Cardiol. (2017) 70:1–25. doi: 10.1016/j.jacc.2017.04.052
4.Gibbons, GH, and Dzau, VJ. The emerging concept of vascular remodeling. N Engl J Med. (1994) 330:1431–8. doi: 10.1056/NEJM199405193302008
5.Bortman, SM, and Losordo, DW. Dynamics of vascular remodeling: an overview and bibliography. J Thromb Thrombolysis. (1996) 3:71–86. doi: 10.1007/BF00226415
6.Méndez-Barbero, N, Gutiérrez-Muñoz, C, and Blanco-Colio, LM. Cellular crosstalk between endothelial and smooth muscle cells in vascular wall remodeling. Int J Mol Sci. (2021) 22:7284. doi: 10.3390/ijms22147284
7.Ibrahim, A, and Marbán, E. Exosomes: fundamental biology and roles in cardiovascular physiology. Annu Rev Physiol. (2016) 78:67–83. doi: 10.1146/annurev-physiol-021115-104929
8.Mathieu, M, Martin-Jaular, L, Lavieu, G, and Théry, C. Specificities of secretion and uptake of exosomes and other extracellular vesicles for cell-to-cell communication. Nat Cell Biol. (2019) 21:9–17. doi: 10.1038/s41556-018-0250-9
9.Tetta, C, Ghigo, E, Silengo, L, Deregibus, MC, and Camussi, G. Extracellular vesicles as an emerging mechanism of cell-to-cell communication. Endocrine. (2013) 44:11–9. doi: 10.1007/s12020-012-9839-0
10.Xiong, F, Mao, R, Zhao, R, Zhang, L, Tan, K, Liu, C, et al. Plasma exosomal S1PR5 and CARNS1 as potential non-invasive screening biomarkers of coronary heart disease. Front Cardiovasc Med. (2022) 9:845673. doi: 10.3389/fcvm.2022.845673
11.Fan, J, Ren, M, and He, Y. Diagnostic and therapeutic properties of exosomes in cardiac fibrosis. Front Cell Dev Biol. (2022) 10:931082. doi: 10.3389/fcell.2022.931082
12.Hade, MD, Suire, CN, and Suo, Z. Mesenchymal stem cell-derived exosomes: applications in regenerative medicine. Cells. (2021) 10:1959. doi: 10.3390/cells10081959
13.Xiong, Y, Tang, R, Xu, J, Jiang, W, Gong, Z, Zhang, L, et al. Tongxinluo-pretreated mesenchymal stem cells facilitate cardiac repair via exosomal transfer of miR-146a-5p targeting IRAK1/NF-κB p65 pathway. Stem Cell Res Ther. (2022) 13:289. doi: 10.1186/s13287-022-02969-y
14.Street, JM, Barran, PE, Mackay, CL, Weidt, S, Balmforth, C, Walsh, TS, et al. Identification and proteomic profiling of exosomes in human cerebrospinal fluid. J Transl Med. (2012) 10:5. doi: 10.1186/1479-5876-10-5
15.Chen, C, Skog, J, Hsu, C-H, Lessard, RT, Balaj, L, Wurdinger, T, et al. Microfluidic isolation and transcriptome analysis of serum microvesicles. Lab Chip. (2010) 10:505–11. doi: 10.1039/B916199F
16.Pisitkun, T, Shen, R-F, and Knepper, MA. Identification and proteomic profiling of exosomes in human urine. Proc Natl Acad Sci U S A. (2004) 101:13368–73. doi: 10.1073/pnas.0403453101
17.Lin, J, Li, J, Huang, B, Liu, J, Chen, X, Chen, X-M, et al. Exosomes: novel biomarkers for clinical diagnosis. ScientificWorldJournal. (2015) 2015:657086. doi: 10.1155/2015/657086
18.Pan, BT, Teng, K, Wu, C, Adam, M, and Johnstone, RM. Electron microscopic evidence for externalization of the transferrin receptor in vesicular form in sheep reticulocytes. J Cell Biol. (1985) 101:942–8. doi: 10.1083/jcb.101.3.942
19.Edgar, JR. Q&A: what are exosomes, exactly? BMC Biol. (2016) 14:46. doi: 10.1186/s12915-016-0268-z
20.Wang, J, Wu, F, Liu, C, Dai, W, Teng, Y, Su, W, et al. Exosomes released from rabies virus-infected cells may be involved in the infection process. Virol Sin. (2019) 34:59–65. doi: 10.1007/s12250-019-00087-3
21.Jin, K, Wen, Z, Wu, B, Zhang, H, Qiu, J, Wang, Y, et al. NOTCH-induced rerouting of endosomal trafficking disables regulatory T cells in vasculitis. J Clin Invest. (2021) 131:136042. doi: 10.1172/JCI136042
22.Neves, KB, Rios, FJ, Sevilla-Montero, J, Montezano, AC, and Touyz, RM. Exosomes and the cardiovascular system: role in cardiovascular health and disease. J Physiol. (2022). doi: 10.1113/JP282054
23.Xu, R, Greening, DW, Zhu, H-J, Takahashi, N, and Simpson, RJ. Extracellular vesicle isolation and characterization: toward clinical application. J Clin Invest. (2016) 126:1152–62. doi: 10.1172/JCI81129
24.Schmidt, O, and Teis, D. The ESCRT machinery. Curr Biol. (2012) 22:R116–20. doi: 10.1016/j.cub.2012.01.028
25.Henning, RJ. Cardiovascular exosomes and MicroRNAs in cardiovascular physiology and pathophysiology. J Cardiovasc Transl Res. (2021) 14:195–212. doi: 10.1007/s12265-020-10040-5
26.Ostrowski, M, Carmo, NB, Krumeich, S, Fanget, I, Raposo, G, Savina, A, et al. Rab27a and Rab27b control different steps of the exosome secretion pathway. Nat Cell Biol. (2010) 12:19–30. doi: 10.1038/ncb2000
27.Willms, E, Johansson, HJ, Mäger, I, Lee, Y, Blomberg, KEM, Sadik, M, et al. Cells release subpopulations of exosomes with distinct molecular and biological properties. Sci Rep. (2016) 6:22519. doi: 10.1038/srep22519
28.Colombo, M, Raposo, G, and Théry, C. Biogenesis, secretion, and intercellular interactions of exosomes and other extracellular vesicles. Annu Rev Cell Dev Biol. (2014) 30:255–89. doi: 10.1146/annurev-cellbio-101512-122326
29.Saadi, E, Tal, S, and Barki-Harrington, L. Substrate-inactivated cyclooxygenase-2 is disposed of by exosomes through the ER-Golgi pathway. Biochem J. (2018) 475:3141–51. doi: 10.1042/BCJ20180530
30.Sahoo, S, Adamiak, M, Mathiyalagan, P, Kenneweg, F, Kafert-Kasting, S, and Thum, T. Therapeutic and diagnostic translation of extracellular vesicles in cardiovascular diseases: roadmap to the clinic. Circulation. (2021) 143:1426–49. doi: 10.1161/CIRCULATIONAHA.120.049254
31.Han, L, Lam, EW-F, and Sun, Y. Extracellular vesicles in the tumor microenvironment: old stories, but new tales. Mol Cancer. (2019) 18:59. doi: 10.1186/s12943-019-0980-8
32.Vader, P, Breakefield, XO, and Wood, MJA. Extracellular vesicles: emerging targets for cancer therapy. Trends Mol Med. (2014) 20:385–93. doi: 10.1016/j.molmed.2014.03.002
33.Jaiswal, R, and Sedger, LM. Intercellular vesicular transfer by exosomes, microparticles and oncosomes - implications for cancer biology and treatments. Front Oncol. (2019) 9:125. doi: 10.3389/fonc.2019.00125
34.Zhang, H, Freitas, D, Kim, HS, Fabijanic, K, Li, Z, Chen, H, et al. Identification of distinct nanoparticles and subsets of extracellular vesicles by asymmetric flow field-flow fractionation. Nat Cell Biol. (2018) 20:332–43. doi: 10.1038/s41556-018-0040-4
35.Pegtel, DM, and Gould, SJ. Exosomes. Annu Rev Biochem. (2019) 88:487–514. doi: 10.1146/annurev-biochem-013118-111902
36.Fordjour, FK, Daaboul, GG, and Gould, SJ. A shared pathway of exosome biogenesis operates at plasma and endosome membranes. Cell Biol [Preprint]. (2019). doi: 10.1101/545228
37.Théry, C, Witwer, KW, Aikawa, E, Alcaraz, MJ, Anderson, JD, Andriantsitohaina, R, et al. Minimal information for studies of extracellular vesicles 2018 (MISEV2018): a position statement of the International Society for Extracellular Vesicles and update of the MISEV2014 guidelines. J Extracell Vesicles. (2018) 7:1535750. doi: 10.1080/20013078.2018.1535750
38.Kowal, J, Arras, G, Colombo, M, Jouve, M, Morath, JP, Primdal-Bengtson, B, et al. Proteomic comparison defines novel markers to characterize heterogeneous populations of extracellular vesicle subtypes. Proc Natl Acad Sci U S A. (2016) 113:E968–77. doi: 10.1073/pnas.1521230113
39.Yang, D, Zhang, W, Zhang, H, Zhang, F, Chen, L, Ma, L, et al. Progress, opportunity, and perspective on exosome isolation - efforts for efficient exosome-based theranostics. Theranostics. (2020) 10:3684–707. doi: 10.7150/thno.41580
40.Raposo, G, Nijman, HW, Stoorvogel, W, Liejendekker, R, Harding, CV, Melief, CJ, et al. B lymphocytes secrete antigen-presenting vesicles. J Exp Med. (1996) 183:1161–72. doi: 10.1084/jem.183.3.1161
41.Valadi, H, Ekström, K, Bossios, A, Sjöstrand, M, Lee, JJ, and Lötvall, JO. Exosome-mediated transfer of mRNAs and microRNAs is a novel mechanism of genetic exchange between cells. Nat Cell Biol. (2007) 9:654–9. doi: 10.1038/ncb1596
42.Mori, MA, Ludwig, RG, Garcia-Martin, R, Brandão, BB, and Kahn, CR. Extracellular miRNAs: from biomarkers to mediators of physiology and disease. Cell Metab. (2019) 30:656–73. doi: 10.1016/j.cmet.2019.07.011
43.Asare-Werehene, M, Nakka, K, Reunov, A, Chiu, C-T, Lee, W-T, Abedini, MR, et al. The exosome-mediated autocrine and paracrine actions of plasma gelsolin in ovarian cancer chemoresistance. Oncogene. (2020) 39:1600–16. doi: 10.1038/s41388-019-1087-9
44.Bjørge, IM, Kim, SY, Mano, JF, Kalionis, B, and Chrzanowski, W. Extracellular vesicles, exosomes and shedding vesicles in regenerative medicine - a new paradigm for tissue repair. Biomater Sci. (2017) 6:60–78. doi: 10.1039/c7bm00479f
45.Plebanek, MP, Angeloni, NL, Vinokour, E, Li, J, Henkin, A, Martinez-Marin, D, et al. Pre-metastatic cancer exosomes induce immune surveillance by patrolling monocytes at the metastatic niche. Nat Commun. (2017) 8:1319. doi: 10.1038/s41467-017-01433-3
46.Yin, S, Ji, C, Wu, P, Jin, C, and Qian, H. Human umbilical cord mesenchymal stem cells and exosomes: bioactive ways of tissue injury repair. Am J Transl Res. (2019) 11:1230–40.
47.Zarà, M, Amadio, P, Campodonico, J, Sandrini, L, and Barbieri, SS. Exosomes in cardiovascular diseases. Diagnostics. (2020) 10:E943. doi: 10.3390/diagnostics10110943
48.Polanco, JC, Li, C, Durisic, N, Sullivan, R, and Götz, J. Exosomes taken up by neurons hijack the endosomal pathway to spread to interconnected neurons. Acta Neuropathol Commun. (2018) 6:10. doi: 10.1186/s40478-018-0514-4
49.Zhang, X, Zhang, H, Gu, J, Zhang, J, Shi, H, Qian, H, et al. Engineered extracellular vesicles for cancer therapy. Adv Mater. (2021) 33:e2005709. doi: 10.1002/adma.202105655
50.Zheng, H, Liang, X, Han, Q, Shao, Z, Zhang, Y, Shi, L, et al. Hemin enhances the cardioprotective effects of mesenchymal stem cell-derived exosomes against infarction via amelioration of cardiomyocyte senescence. J Nanobiotechnology. (2021) 19:332. doi: 10.1186/s12951-021-01077-y
51.Lin, F, Chen, W, Zhou, J, Zhu, J, Yao, Q, Feng, B, et al. Mesenchymal stem cells protect against ferroptosis via exosome-mediated stabilization of SLC7A11 in acute liver injury. Cell Death Dis. (2022) 13:271. doi: 10.1038/s41419-022-04708-w
52.Bouchareychas, L, Duong, P, Covarrubias, S, Alsop, E, Phu, TA, Chung, A, et al. Macrophage exosomes resolve atherosclerosis by regulating Hematopoiesis and inflammation via MicroRNA cargo. Cell Rep. (2020) 32:107881. doi: 10.1016/j.celrep.2020.107881
53.Maas, SLN, Breakefield, XO, and Weaver, AM. Extracellular vesicles: unique intercellular delivery vehicles. Trends Cell Biol. (2017) 27:172–88. doi: 10.1016/j.tcb.2016.11.003
54.Soares, AR, Martins-Marques, T, Ribeiro-Rodrigues, T, Ferreira, JV, Catarino, S, Pinho, MJ, et al. Gap junctional protein Cx43 is involved in the communication between extracellular vesicles and mammalian cells. Sci Rep. (2015) 5:13243. doi: 10.1038/srep13243
55.Lin, Y, Zhu, W, and Chen, X. The involving progress of MSCs based therapy in atherosclerosis. Stem Cell Res Ther. (2020) 11:216. doi: 10.1186/s13287-020-01728-1
56.Pitt, JM, André, F, Amigorena, S, Soria, J-C, Eggermont, A, Kroemer, G, et al. Dendritic cell-derived exosomes for cancer therapy. J Clin Invest. (2016) 126:1224–32. doi: 10.1172/JCI81137
57.Wang, Y, Xiang, Y, Xin, VW, Wang, X-W, Peng, X-C, Liu, X-Q, et al. Dendritic cell biology and its role in tumor immunotherapy. J Hematol Oncol. (2020) 13:107. doi: 10.1186/s13045-020-00939-6
58.Dabrowska, S, Andrzejewska, A, Lukomska, B, and Janowski, M. Neuroinflammation as a target for treatment of stroke using mesenchymal stem cells and extracellular vesicles. J Neuroinflammation. (2019) 16:178. doi: 10.1186/s12974-019-1571-8
59.Lim, SS, Vos, T, Flaxman, AD, Danaei, G, Shibuya, K, Adair-Rohani, H, et al. A comparative risk assessment of burden of disease and injury attributable to 67 risk factors and risk factor clusters in 21 regions, 1990-2010: a systematic analysis for the global burden of disease study 2010. Lancet. (2012) 380:2224–60. doi: 10.1016/S0140-6736(12)61766-8
60.Lewington, S, Clarke, R, Qizilbash, N, Peto, R, and Collins, R. Prospective studies collaboration. Age-specific relevance of usual blood pressure to vascular mortality: a meta-analysis of individual data for one million adults in 61 prospective studies. Lancet. (2002) 360:1903–13. doi: 10.1016/s0140-6736(02)11911-8
61.Bakris, G, Ali, W, and Parati, G. ACC/AHA versus ESC/ESH on hypertension guidelines: JACC guideline comparison. J Am Coll Cardiol. (2019) 73:3018–26. doi: 10.1016/j.jacc.2019.03.507
62.Tan, PPS, Hall, D, Chilian, WM, Chia, YC, Mohd Zain, S, Lim, HM, et al. Exosomal microRNAs in the development of essential hypertension and its potential as biomarkers. Am J Physiol Heart Circ Physiol. (2021) 320:H1486–97. doi: 10.1152/ajpheart.00888.2020
63.Lubos, E, Handy, DE, and Loscalzo, J. Role of oxidative stress and nitric oxide in atherothrombosis. Front Biosci. (2008) 13:5323–44. doi: 10.2741/3084
64.Togliatto, G, Lombardo, G, and Brizzi, MF. The future challenge of reactive oxygen species (ROS) in hypertension: from bench to bed side. Int J Mol Sci. (2017) 18:E1988. doi: 10.3390/ijms18091988
65.Guzik, TJ, and Touyz, RM. Oxidative stress, inflammation, and vascular aging in hypertension. Hypertension. (2017) 70:660–7. doi: 10.1161/HYPERTENSIONAHA.117.07802
66.Manrique, C, Lastra, G, Gardner, M, and Sowers, JR. The renin angiotensin aldosterone system in hypertension: roles of insulin resistance and oxidative stress. Med Clin North Am. (2009) 93:569–82. doi: 10.1016/j.mcna.2009.02.014
67.Solak, Y, Afsar, B, Vaziri, ND, Aslan, G, Yalcin, CE, Covic, A, et al. Hypertension as an autoimmune and inflammatory disease. Hypertens Res. (2016) 39:567–73. doi: 10.1038/hr.2016.35
68.Heijnen, BF, Van Essen, H, Schalkwijk, CG, Janssen, BJ, and Struijker-Boudier, HA. Renal inflammatory markers during the onset of hypertension in spontaneously hypertensive rats. Hypertens Res. (2014) 37:100–9. doi: 10.1038/hr.2013.99
69.Rodriguez-Iturbe, B, Zhan, C-D, Quiroz, Y, Sindhu, RK, and Vaziri, ND. Antioxidant-rich diet relieves hypertension and reduces renal immune infiltration in spontaneously hypertensive rats. Hypertension. (2003) 41:341–6. doi: 10.1161/01.HYP.0000052833.20759.64
70.Preston, RA, Jy, W, Jimenez, JJ, Mauro, LM, Horstman, LL, Valle, M, et al. Effects of severe hypertension on endothelial and platelet microparticles. Hypertension. (2003) 41:211–7. doi: 10.1161/01.HYP.0000049760.15764.2D
71.Wu, Q, Yuan, X, Li, B, Han, R, Zhang, H, and Xiu, R. Integrated exosomal miRNA and transcriptome analysis of brain microvascular endothelial cells in spontaneously hypertensive rats. Hypertens Res. (2020) 43:90–8. doi: 10.1038/s41440-019-0345-0
72.Hu, S-S, Zhang, H-G, Zhang, Q-J, and Xiu, R-J. Circulating CD62P small microparticles levels are increased in hypertension. Int J Clin Exp Pathol. (2014) 7:5324–6.
73.Wang, J-M, Su, C, Wang, Y, Huang, Y-J, Yang, Z, Chen, L, et al. Elevated circulating endothelial microparticles and brachial-ankle pulse wave velocity in well-controlled hypertensive patients. J Hum Hypertens. (2009) 23:307–15. doi: 10.1038/jhh.2008.137
74.Bao, H, Li, Z-T, Xu, L-H, Su, T-Y, Han, Y, Bao, M, et al. Platelet-derived extracellular vesicles increase Col8a1 secretion and vascular stiffness in intimal injury. Front Cell Dev Biol. (2021) 9:641763. doi: 10.3389/fcell.2021.641763
75.Wang, C, Wu, H, Xing, Y, Ye, Y, He, F, Yin, Q, et al. Endothelial-derived extracellular microRNA-92a promotes arterial stiffness by regulating phenotype changes of vascular smooth muscle cells. Sci Rep. (2022) 12:344. doi: 10.1038/s41598-021-04341-1
76.Hazra, S, Henson, GD, Morgan, RG, Breevoort, SR, Ives, SJ, Richardson, RS, et al. Experimental reduction of miR-92a mimics arterial aging. Exp Gerontol. (2016) 83:165–70. doi: 10.1016/j.exger.2016.08.007
77.Osada-Oka, M, Shiota, M, Izumi, Y, Nishiyama, M, Tanaka, M, Yamaguchi, T, et al. Macrophage-derived exosomes induce inflammatory factors in endothelial cells under hypertensive conditions. Hypertens Res. (2017) 40:353–60. doi: 10.1038/hr.2016.163
78.Shang, F, Guo, X, Chen, Y, Wang, C, Gao, J, Wen, E, et al. Endothelial MicroRNA-483-3p is hypertension-protective. Oxidative Med Cell Longev. (2022) 2022:3698219. doi: 10.1155/2022/3698219
79.Fernandez Esmerats, J, Villa-Roel, N, Kumar, S, Gu, L, Salim, MT, Ohh, M, et al. Disturbed flow increases UBE2C (ubiquitin E2 ligase C) via loss of miR-483-3p, inducing aortic valve calcification by the pVHL (von Hippel-Lindau protein) and HIF-1α (hypoxia-inducible factor-1α) pathway in endothelial cells. Arterioscler Thromb Vasc Biol. (2019) 39:467–81. doi: 10.1161/ATVBAHA.118.312233
80.Tong, Y, Ye, C, Ren, X-S, Qiu, Y, Zang, Y-H, Xiong, X-Q, et al. Exosome-mediated transfer of ACE (angiotensin-converting enzyme) from adventitial fibroblasts of spontaneously hypertensive rats promotes vascular smooth muscle cell migration. Hypertension. (2018) 72:881–8. doi: 10.1161/HYPERTENSIONAHA.118.11375
81.Ren, X-S, Tong, Y, Qiu, Y, Ye, C, Wu, N, Xiong, X-Q, et al. MiR155-5p in adventitial fibroblasts-derived extracellular vesicles inhibits vascular smooth muscle cell proliferation via suppressing angiotensin-converting enzyme expression. J Extracell Vesicles. (2020) 9:1698795. doi: 10.1080/20013078.2019.1698795
82.Tong, Y, Ye, C, Zheng, F, Bo, J-H, Wu, L-L, Han, Y, et al. Extracellular vesicle-mediated miR135a-5p transfer in hypertensive rat contributes to vascular smooth muscle cell proliferation via targeting FNDC5. Vasc Pharmacol. (2021) 140:106864. doi: 10.1016/j.vph.2021.106864
83.Liu, T-Y, Xiong, X-Q, Ren, X-S, Zhao, M-X, Shi, C-X, Wang, J-J, et al. FNDC5 alleviates hepatosteatosis by restoring AMPK/mTOR-mediated autophagy, fatty acid oxidation, and lipogenesis in mice. Diabetes. (2016) 65:3262–75. doi: 10.2337/db16-0356
84.Rabiee, F, Lachinani, L, Ghaedi, S, Nasr-Esfahani, MH, Megraw, TL, and Ghaedi, K. New insights into the cellular activities of Fndc5/Irisin and its signaling pathways. Cell Biosci. (2020) 10:51. doi: 10.1186/s13578-020-00413-3
85.Zou, X, Wang, J, Chen, C, Tan, X, Huang, Y, Jose, PA, et al. Secreted monocyte miR-27a, via mesenteric arterial mas receptor-eNOS pathway, causes hypertension. Am J Hypertens. (2020) 33:31–42. doi: 10.1093/ajh/hpz112
86.Yang, W, Zou, B, Hou, Y, Yan, W, Chen, T, and Qu, S. Extracellular vesicles in vascular calcification. Clin Chim Acta. (2019) 499:118–22. doi: 10.1016/j.cca.2019.09.002
87.Qin, Z, Liao, R, Xiong, Y, Jiang, L, Li, J, Wang, L, et al. A narrative review of exosomes in vascular calcification. Ann Transl Med. (2021) 9:579. doi: 10.21037/atm-20-7355
88.Yaker, L, Tebani, A, Lesueur, C, Dias, C, Jung, V, Bekri, S, et al. Extracellular vesicles from LPS-treated macrophages aggravate smooth muscle cell calcification by propagating inflammation and oxidative stress. Front Cell Dev Biol. (2022) 10:823450. doi: 10.3389/fcell.2022.823450
89.Petsophonsakul, P, Burgmaier, M, Willems, B, Heeneman, S, Stadler, N, Gremse, F, et al. Nicotine promotes vascular calcification via intracellular Ca2+−mediated, Nox5-induced oxidative stress, and extracellular vesicle release in vascular smooth muscle cells. Cardiovasc Res. (2022) 118:2196–210. doi: 10.1093/cvr/cvab244
90.Furmanik, M, Chatrou, M, van Gorp, R, Akbulut, A, Willems, B, Schmidt, H, et al. Reactive oxygen-forming Nox5 links vascular smooth muscle cell phenotypic switching and extracellular vesicle-mediated vascular calcification. Circ Res. (2020) 127:911–27. doi: 10.1161/CIRCRESAHA.119.316159
91.Cao, J, Chen, C, Chen, Q, Gao, Y, Zhao, Z, Yuan, Q, et al. Extracellular vesicle miR-32 derived from macrophage promotes arterial calcification in mice with type 2 diabetes via inhibiting VSMC autophagy. J Transl Med. (2022) 20:307. doi: 10.1186/s12967-022-03502-8
92.Rogers, MA, Atkins, SK, Zheng, KH, Singh, SA, Chelvanambi, S, Pham, TH, et al. Lipoprotein(a) induces vesicular cardiovascular calcification revealed with single-extracellular vesicle analysis. Front Cardiovasc Med. (2022) 9:778919. doi: 10.3389/fcvm.2022.778919
93.Furmanik, M, van Gorp, R, Whitehead, M, Ahmad, S, Bordoloi, J, Kapustin, A, et al. Endoplasmic reticulum stress mediates vascular smooth muscle cell calcification via increased release of Grp78 (glucose-regulated protein, 78 kDa)-loaded extracellular vesicles. Arterioscler Thromb Vasc Biol. (2021) 41:898–914. doi: 10.1161/ATVBAHA.120.315506
94.Chen, C, Li, Y, Lu, H, Liu, K, Jiang, W, Zhang, Z, et al. Curcumin attenuates vascular calcification via the exosomal miR-92b-3p/KLF4 axis. Exp Biol Med. (2022) 247:1420–32. doi: 10.1177/15353702221095456
95.Xu, F, Zhong, J-Y, Lin, X, Shan, S-K, Guo, B, Zheng, M-H, et al. Melatonin alleviates vascular calcification and ageing through exosomal miR-204/miR-211 cluster in a paracrine manner. J Pineal Res. (2020) 68:e12631. doi: 10.1111/jpi.12631
96.Mansour, A, Darwiche, W, Yaker, L, Da Nascimento, S, Gomila, C, Rossi, C, et al. GFOGER peptide modifies the protein content of extracellular vesicles and inhibits vascular calcification. Front Cell Dev Biol. (2020) 8:589761. doi: 10.3389/fcell.2020.589761
97.Wang, Y, Ma, W-Q, Zhu, Y, Han, X-Q, and Liu, N. Exosomes derived from mesenchymal stromal cells pretreated with advanced glycation end product-bovine serum albumin inhibit calcification of vascular smooth muscle cells. Front Endocrinol. (2018) 9:524. doi: 10.3389/fendo.2018.00524
98.Guo, B, Shan, S-K, Xu, F, Lin, X, Li, F-X-Z, Wang, Y, et al. Protective role of small extracellular vesicles derived from HUVECs treated with AGEs in diabetic vascular calcification. J Nanobiotechnol. (2022) 20:334. doi: 10.1186/s12951-022-01529-z
99.Perez-Hernandez, J, Riffo-Campos, AL, Ortega, A, Martinez-Arroyo, O, Perez-Gil, D, Olivares, D, et al. Urinary- and plasma-derived exosomes reveal a distinct MicroRNA signature associated with albuminuria in hypertension. Hypertension. (2021) 77:960–71. doi: 10.1161/HYPERTENSIONAHA.120.16598
100.Zhu, X-Y, Ebrahimi, B, Eirin, A, Woollard, JR, Tang, H, Jordan, KL, et al. Renal vein levels of MicroRNA-26a are lower in the poststenotic kidney. J Am Soc Nephrol. (2015) 26:1378–88. doi: 10.1681/ASN.2014030248
101.Riffo-Campos, AL, Perez-Hernandez, J, Ortega, A, Martinez-Arroyo, O, Flores-Chova, A, Redon, J, et al. Exosomal and plasma non-coding RNA signature associated with urinary albumin excretion in hypertension. Int J Mol Sci. (2022) 23:823. doi: 10.3390/ijms23020823
102.Gonzalez-Calero, L, Martínez, PJ, Martin-Lorenzo, M, Baldan-Martin, M, Ruiz-Hurtado, G, de la Cuesta, F, et al. Urinary exosomes reveal protein signatures in hypertensive patients with albuminuria. Oncotarget. (2017) 8:44217–31. doi: 10.18632/oncotarget.17787
103.Doran, AC. Inflammation resolution: implications for atherosclerosis. Circ Res. (2022) 130:130–48. doi: 10.1161/CIRCRESAHA.121.319822
104.Virani, SS, Alonso, A, Aparicio, HJ, Benjamin, EJ, Bittencourt, MS, Callaway, CW, et al. Heart disease and stroke statistics-2021 update: a report from the American Heart Association. Circulation. (2021) 143:e254–743. doi: 10.1161/CIR.0000000000000950
105.Checkouri, E, Blanchard, V, and Meilhac, O. Macrophages in atherosclerosis, first or second row players? Biomedicine. (2021) 9:1214. doi: 10.3390/biomedicines9091214
106.Suwanabol, PA, Kent, KC, and Liu, B. TGF-β and restenosis revisited: a Smad link. J Surg Res. (2011) 167:287–97. doi: 10.1016/j.jss.2010.12.020
107.Si, Y, Liu, F, Wang, D, Fang, C, Tang, X, Guo, B, et al. Exosomal transfer of miR-185 is controlled by hnRNPA2B1 and impairs re-endothelialization after vascular injury. Front Cell Dev Biol. (2021) 9:619444. doi: 10.3389/fcell.2021.619444
108.Ke, X, Liao, Z, Luo, X, Chen, J-Q, Deng, M, Huang, Y, et al. Endothelial colony-forming cell-derived exosomal miR-21-5p regulates autophagic flux to promote vascular endothelial repair by inhibiting SIPL1A2 in atherosclerosis. Cell Commun Signal. (2022) 20:30. doi: 10.1186/s12964-022-00828-0
109.Soehnlein, O, and Libby, P. Targeting inflammation in atherosclerosis - from experimental insights to the clinic. Nat Rev Drug Discov. (2021) 20:589–610. doi: 10.1038/s41573-021-00198-1
110.Nidorf, SM, Fiolet, ATL, Mosterd, A, Eikelboom, JW, Schut, A, Opstal, TSJ, et al. Colchicine in patients with chronic coronary disease. N Engl J Med. (2020) 383:1838–47. doi: 10.1056/NEJMoa2021372
111.Ridker, PM, Everett, BM, Thuren, T, MacFadyen, JG, Chang, WH, Ballantyne, C, et al. Antiinflammatory therapy with canakinumab for atherosclerotic disease. N Engl J Med. (2017) 377:1119–31. doi: 10.1056/NEJMoa1707914
112.Gisterå, A, and Hansson, GK. The immunology of atherosclerosis. Nat Rev Nephrol. (2017) 13:368–80. doi: 10.1038/nrneph.2017.51
113.Li, K, Cui, M, Zhang, K, Wang, G, and Zhai, S. M1 macrophages-derived extracellular vesicles elevate microRNA-185-3p to aggravate the development of atherosclerosis in ApoE−/− mice by inhibiting small mothers against decapentaplegic 7. Int Immunopharmacol. (2021) 90:107138. doi: 10.1016/j.intimp.2020.107138
114.Zhang, D, Lee, H, Cao, Y, Dela Cruz, CS, and Jin, Y. miR-185 mediates lung epithelial cell death after oxidative stress. Am J Physiol Lung Cell Mol Physiol. (2016) 310:L700–10. doi: 10.1152/ajplung.00392.2015
115.Lin, B, Xie, W, Zeng, C, Wu, X, Chen, A, Li, H, et al. Transfer of exosomal microRNA-203-3p from dendritic cells to bone marrow-derived macrophages reduces development of atherosclerosis by downregulating Ctss in mice. Aging. (2021) 13:15638–58. doi: 10.18632/aging.103842
116.Nguyen, M-A, Karunakaran, D, Geoffrion, M, Cheng, HS, Tandoc, K, Perisic Matic, L, et al. Extracellular vesicles secreted by atherogenic macrophages transfer MicroRNA to inhibit cell migration. Arterioscler Thromb Vasc Biol. (2018) 38:49–63. doi: 10.1161/ATVBAHA.117.309795
117.Cao, Q, Wu, J, Wang, X, and Song, C. Noncoding RNAs in vascular aging. Oxidative Med Cell Longev. (2020) 2020:7914957–14. doi: 10.1155/2020/7914957
118.Yang, K, He, YS, Wang, XQ, Lu, L, Chen, QJ, Liu, J, et al. MiR-146a inhibits oxidized low-density lipoprotein-induced lipid accumulation and inflammatory response via targeting toll-like receptor 4. FEBS Lett. (2011) 585:854–60. doi: 10.1016/j.febslet.2011.02.009
119.Li, K, Ching, D, Luk, FS, and Raffai, RL. Apolipoprotein E enhances microRNA-146a in monocytes and macrophages to suppress nuclear factor-κB-driven inflammation and atherosclerosis. Circ Res. (2015) 117:e1–e11. doi: 10.1161/CIRCRESAHA.117.305844
120.Cheng, HS, Besla, R, Li, A, Chen, Z, Shikatani, EA, Nazari-Jahantigh, M, et al. Paradoxical suppression of atherosclerosis in the absence of microRNA-146a. Circ Res. (2017) 121:354–67. doi: 10.1161/CIRCRESAHA.116.310529
121.Zhong, X, Gao, W, Wu, R, Liu, H, and Ge, J. Dendritic cell exosome-shuttled miRNA146a regulates exosome-induced endothelial cell inflammation by inhibiting IRAK-1: a feedback control mechanism. Mol Med Rep. (2019) 20:5315–23. doi: 10.3892/mmr.2019.10749
122.He, S, Wu, C, Xiao, J, Li, D, Sun, Z, and Li, M. Endothelial extracellular vesicles modulate the macrophage phenotype: potential implications in atherosclerosis. Scand J Immunol. (2018) 87:e12648. doi: 10.1111/sji.12648
123.Chang, Y-J, Li, Y-S, Wu, C-C, Wang, K-C, Huang, T-C, Chen, Z, et al. Extracellular MicroRNA-92a mediates endothelial cell-macrophage communication. Arterioscler Thromb Vasc Biol. (2019) 39:2492–504. doi: 10.1161/ATVBAHA.119.312707
124.Wang, J, Zhang, C, Li, C, Zhao, D, Li, S, Ma, L, et al. MicroRNA-92a promotes vascular smooth muscle cell proliferation and migration through the ROCK/MLCK signalling pathway. J Cell Mol Med. (2019) 23:3696–710. doi: 10.1111/jcmm.14274
125.Xu, L, Geng, T, Zang, G, Bo, L, Liang, Y, Zhou, H, et al. Exosome derived from CD137-modified endothelial cells regulates the Th17 responses in atherosclerosis. J Cell Mol Med. (2020) 24:4659–67. doi: 10.1111/jcmm.15130
126.Zhang, J, Zhao, W-S, Xu, L, Wang, X, Li, X-L, and Yang, X-C. Endothelium-specific endothelin-1 expression promotes pro-inflammatory macrophage activation by regulating miR-33/NR4A axis. Exp Cell Res. (2021) 399:112443. doi: 10.1016/j.yexcr.2020.112443
127.Frismantiene, A, Philippova, M, Erne, P, and Resink, TJ. Smooth muscle cell-driven vascular diseases and molecular mechanisms of VSMC plasticity. Cell Signal. (2018) 52:48–64. doi: 10.1016/j.cellsig.2018.08.019
128.Dautova, Y, Kapustin, AN, Pappert, K, Epple, M, Okkenhaug, H, Cook, SJ, et al. Calcium phosphate particles stimulate interleukin-1β release from human vascular smooth muscle cells: a role for spleen tyrosine kinase and exosome release. J Mol Cell Cardiol. (2018) 115:82–93. doi: 10.1016/j.yjmcc.2017.12.007
129.Głuchowska, A, Cysewski, D, Baj-Krzyworzeka, M, Szatanek, R, Węglarczyk, K, Podszywałow-Bartnicka, P, et al. Unbiased proteomic analysis of extracellular vesicles secreted by senescent human vascular smooth muscle cells reveals their ability to modulate immune cell functions. Geroscience. (2022) 44:2863–84. doi: 10.1007/s11357-022-00625-0
130.Greco, MF, Rizzuto, AS, Zarà, M, Cafora, M, Favero, C, Solazzo, G, et al. PCSK9 confers inflammatory properties to extracellular vesicles released by vascular smooth muscle cells. Int J Mol Sci. (2022) 23:13065. doi: 10.3390/ijms232113065
131.Zheng, B, Yin, W-N, Suzuki, T, Zhang, X-H, Zhang, Y, Song, L-L, et al. Exosome-mediated miR-155 transfer from smooth muscle cells to endothelial cells induces endothelial injury and promotes atherosclerosis. Mol Ther. (2017) 25:1279–94. doi: 10.1016/j.ymthe.2017.03.031
132.Song, T, Zhou, M, Li, W, Zheng, L, Wu, J, and Zhao, M. Tripeptide Leu-Ser-Trp regulates the vascular endothelial cells phenotype switching by mediating the vascular smooth muscle cells-derived small extracellular vesicles packaging of miR-145. Molecules. (2022) 27:7025. doi: 10.3390/molecules27207025
133.Xie, Z, Wang, X, Liu, X, Du, H, Sun, C, Shao, X, et al. Adipose-derived exosomes exert proatherogenic effects by regulating macrophage foam cell formation and polarization. J Am Heart Assoc. (2018) 7:e007442. doi: 10.1161/JAHA.117.007442
134.Henao Agudelo, JS, Braga, TT, Amano, MT, Cenedeze, MA, Cavinato, RA, Peixoto-Santos, AR, et al. Mesenchymal stromal cell-derived microvesicles regulate an internal pro-inflammatory program in activated macrophages. Front Immunol. (2017) 8:881. doi: 10.3389/fimmu.2017.00881
135.Jiang, F, Chen, Q, Wang, W, Ling, Y, Yan, Y, and Xia, P. Hepatocyte-derived extracellular vesicles promote endothelial inflammation and atherogenesis via microRNA-1. J Hepatol. (2020) 72:156–66. doi: 10.1016/j.jhep.2019.09.014
136.Zhu, J, Liu, B, Wang, Z, Wang, D, Ni, H, Zhang, L, et al. Exosomes from nicotine-stimulated macrophages accelerate atherosclerosis through miR-21-3p/PTEN-mediated VSMC migration and proliferation. Theranostics. (2019) 9:6901–19. doi: 10.7150/thno.37357
137.Lin, C-M, Wang, B-W, Pan, C-M, Fang, W-J, Chua, S-K, Cheng, W-P, et al. Chrysin boosts KLF2 expression through suppression of endothelial cell-derived exosomal microRNA-92a in the model of atheroprotection. Eur J Nutr. (2021) 60:4345–55. doi: 10.1007/s00394-021-02593-1
138.Yao, Y, Sun, W, Sun, Q, Jing, B, Liu, S, Liu, X, et al. Platelet-derived Exosomal MicroRNA-25-3p inhibits coronary vascular endothelial cell inflammation through Adam10 via the NF-κB Signaling pathway in ApoE−/− mice. Front Immunol. (2019) 10:2205. doi: 10.3389/fimmu.2019.02205
139.Liu, Y, Sun, Y, Lin, X, Zhang, D, Hu, C, Liu, J, et al. Perivascular adipose-derived exosomes reduce foam cell formation by regulating expression of cholesterol transporters. Front Cardiovasc Med. (2021) 8:697510. doi: 10.3389/fcvm.2021.697510
140.Liu, Y, Sun, Y, Lin, X, Zhang, D, Hu, C, Liu, J, et al. Perivascular adipose-derived exosomes reduce macrophage foam cell formation through miR-382-5p and the BMP4-PPARγ-ABCA1/ABCG1 pathways. Vasc Pharmacol. (2022) 143:106968. doi: 10.1016/j.vph.2022.106968
141.Liu, Y, Sun, Y, Hu, C, Liu, J, Gao, A, Han, H, et al. Perivascular adipose tissue as an indication, contributor to, and therapeutic target for atherosclerosis. Front Physiol. (2020) 11:615503. doi: 10.3389/fphys.2020.615503
142.Qi, X-Y, Qu, S-L, Xiong, W-H, Rom, O, Chang, L, and Jiang, Z-S. Perivascular adipose tissue (PVAT) in atherosclerosis: a double-edged sword. Cardiovasc Diabetol. (2018) 17:134. doi: 10.1186/s12933-018-0777-x
143.Bennett, MR, Sinha, S, and Owens, GK. Vascular smooth muscle cells in atherosclerosis. Circ Res. (2016) 118:692–702. doi: 10.1161/CIRCRESAHA.115.306361
144.Niu, C, Wang, X, Zhao, M, Cai, T, Liu, P, Li, J, et al. Macrophage foam cell-derived extracellular vesicles promote vascular smooth muscle cell migration and adhesion. J Am Heart Assoc. (2016) 5:e004099. doi: 10.1161/JAHA.116.004099
145.Wang, Q, Dong, Y, and Wang, H. microRNA-19b-3p-containing extracellular vesicles derived from macrophages promote the development of atherosclerosis by targeting JAZF1. J Cell Mol Med. (2022) 26:48–59. doi: 10.1111/jcmm.16938
146.Ren, L, Chen, S, Yao, D, and Yan, H. OxLDL-stimulated macrophage exosomes promote proatherogenic vascular smooth muscle cell viability and invasion via delivering miR-186-5p then inactivating SHIP2 mediated PI3K/AKT/mTOR pathway. Mol Immunol. (2022) 146:27–37. doi: 10.1016/j.molimm.2022.02.018
147.Tarantino, G, and Capone, D. Inhibition of the mTOR pathway: a possible protective role in coronary artery disease. Ann Med. (2013) 45:348–56. doi: 10.3109/07853890.2013.770333
148.Wang, Z, Zhu, H, Shi, H, Zhao, H, Gao, R, Weng, X, et al. Exosomes derived from M1 macrophages aggravate neointimal hyperplasia following carotid artery injuries in mice through miR-222/CDKN1B/CDKN1C pathway. Cell Death Dis. (2019) 10:422. doi: 10.1038/s41419-019-1667-1
149.Li, B, Zang, G, Zhong, W, Chen, R, Zhang, Y, Yang, P, et al. Activation of CD137 signaling promotes neointimal formation by attenuating TET2 and transferrring from endothelial cell-derived exosomes to vascular smooth muscle cells. Biomed Pharmacother. (2020) 121:109593. doi: 10.1016/j.biopha.2019.109593
150.Li, X, Ballantyne, LL, Yu, Y, and Funk, CD. Perivascular adipose tissue-derived extracellular vesicle miR-221-3p mediates vascular remodeling. FASEB J. (2019) 33:12704–22. doi: 10.1096/fj.201901548R
151.Hu, N, Zeng, X, Tang, F, and Xiong, S. Exosomal long non-coding RNA LIPCAR derived from oxLDL-treated THP-1 cells regulates the proliferation of human umbilical vein endothelial cells and human vascular smooth muscle cells. Biochem Biophys Res Commun. (2021) 575:65–72. doi: 10.1016/j.bbrc.2021.08.053
152.Liu, Y, Zhang, W-L, Gu, J-J, Sun, Y-Q, Cui, H-Z, Bu, J-Q, et al. Exosome-mediated miR-106a-3p derived from ox-LDL exposed macrophages accelerated cell proliferation and repressed cell apoptosis of human vascular smooth muscle cells. Eur Rev Med Pharmacol Sci. (2020) 24:7039–50. doi: 10.26355/eurrev_202006_21697
153.Zhang, Z, Yi, D, Zhou, J, Zheng, Y, Gao, Z, Hu, X, et al. Exosomal LINC01005 derived from oxidized low-density lipoprotein-treated endothelial cells regulates vascular smooth muscle cell phenotypic switch. Biofactors. (2020) 46:743–53. doi: 10.1002/biof.1665
154.Kanter, JE, Hsu, C-C, and Bornfeldt, KE. Monocytes and macrophages as protagonists in vascular complications of diabetes. Front Cardiovasc Med. (2020) 7:10. doi: 10.3389/fcvm.2020.00010
155.Burke, AP, Kolodgie, FD, Zieske, A, Fowler, DR, Weber, DK, Varghese, PJ, et al. Morphologic findings of coronary atherosclerotic plaques in diabetics: a postmortem study. Arterioscler Thromb Vasc Biol. (2004) 24:1266–71. doi: 10.1161/01.ATV.0000131783.74034.97
156.Bouchareychas, L, Duong, P, Phu, TA, Alsop, E, Meechoovet, B, Reiman, R, et al. High glucose macrophage exosomes enhance atherosclerosis by driving cellular proliferation & hematopoiesis. iScience. (2021) 24:102847. doi: 10.1016/j.isci.2021.102847
157.Liu, D, Zhang, M, Xie, W, Lan, G, Cheng, H-P, Gong, D, et al. MiR-486 regulates cholesterol efflux by targeting HAT1. Biochem Biophys Res Commun. (2016) 472:418–24. doi: 10.1016/j.bbrc.2015.11.128
158.Wang, F, Chen, F-F, Shang, Y-Y, Li, Y, Wang, Z-H, Han, L, et al. Insulin resistance adipocyte-derived exosomes aggravate atherosclerosis by increasing vasa vasorum angiogenesis in diabetic ApoE−/− mice. Int J Cardiol. (2018) 265:181–7. doi: 10.1016/j.ijcard.2018.04.028
159.Langheinrich, AC, Kampschulte, M, Buch, T, and Bohle, RM. Vasa vasorum and atherosclerosis - Quid novi? Thromb Haemost. (2007) 97:873–9. doi: 10.1160/TH06-12-0742
160.Yeh, C-C, Wu, J-Y, Lee, G-L, Wen, H-T, Lin, P, and Kuo, C-C. Vanadium derivative exposure promotes functional alterations of VSMCs and consequent atherosclerosis via ROS/p38/NF-κB-mediated IL-6 production. Int J Mol Sci. (2019) 20:E6115. doi: 10.3390/ijms20246115
161.Wang, Y, Xu, Z, Wang, X, Zheng, J, Peng, L, Zhou, Y, et al. Extracellular-vesicle containing miRNA-503-5p released by macrophages contributes to atherosclerosis. Aging. (2021) 13:12239–57. doi: 10.18632/aging.103855
162.Tan, M, Yan, H-B, Li, J-N, Li, W-K, Fu, Y-Y, Chen, W, et al. Thrombin stimulated platelet-derived exosomes inhibit platelet-derived growth factor receptor-Beta expression in vascular smooth muscle cells. Cell Physiol Biochem. (2016) 38:2348–65. doi: 10.1159/000445588
163.Srikanthan, S, Li, W, Silverstein, RL, and McIntyre, TM. Exosome poly-ubiquitin inhibits platelet activation, downregulates CD36 and inhibits pro-atherothombotic cellular functions. J Thromb Haemost. (2014) 12:1906–17. doi: 10.1111/jth.12712
164.Runo, JR, and Loyd, JE. Primary pulmonary hypertension. Lancet. (2003) 361:1533–44. doi: 10.1016/S0140-6736(03)13167-4
165.Humbert, M, Guignabert, C, Bonnet, S, Dorfmüller, P, Klinger, JR, Nicolls, MR, et al. Pathology and pathobiology of pulmonary hypertension: state of the art and research perspectives. Eur Respir J. (2019) 53:1801887. doi: 10.1183/13993003.01887-2018
166.Rroji, M, Cafka, M, Seferi, S, Seiti, J, Barbullushi, M, and Goda, A. The potential effect of cardiac function on pulmonary hypertension, other risk factors, and its impact on survival in dialysis patients. Int Urol Nephrol. (2021) 53:343–51. doi: 10.1007/s11255-020-02655-z
167.Frid, MG, McKeon, BA, Thurman, JM, Maron, BA, Li, M, Zhang, H, et al. Immunoglobulin-driven complement activation regulates proinflammatory remodeling in pulmonary hypertension. Am J Respir Crit Care Med. (2020) 201:224–39. doi: 10.1164/rccm.201903-0591OC
168.Guignabert, C, Tu, L, Girerd, B, Ricard, N, Huertas, A, Montani, D, et al. New molecular targets of pulmonary vascular remodeling in pulmonary arterial hypertension: importance of endothelial communication. Chest. (2015) 147:529–37. doi: 10.1378/chest.14-0862
169.Vonk Noordegraaf, A, Chin, KM, Haddad, F, Hassoun, PM, Hemnes, AR, Hopkins, SR, et al. Pathophysiology of the right ventricle and of the pulmonary circulation in pulmonary hypertension: an update. Eur Respir J. (2019) 53:1801900. doi: 10.1183/13993003.01900-2018
170.Galambos, C, Sims-Lucas, S, Abman, SH, and Cool, CD. Intrapulmonary bronchopulmonary anastomoses and plexiform lesions in idiopathic pulmonary arterial hypertension. Am J Respir Crit Care Med. (2016) 193:574–6. doi: 10.1164/rccm.201507-1508LE
171.Hu, Y, Chi, L, Kuebler, WM, and Goldenberg, NM. Perivascular inflammation in pulmonary arterial hypertension. Cells. (2020) 9:E2338. doi: 10.3390/cells9112338
172.Tuder, RM, Archer, SL, Dorfmüller, P, Erzurum, SC, Guignabert, C, Michelakis, E, et al. Relevant issues in the pathology and pathobiology of pulmonary hypertension. J Am Coll Cardiol. (2013) 62:D4–D12. doi: 10.1016/j.jacc.2013.10.025
173.Vrigkou, E, Tsangaris, I, Bonovas, S, Kopterides, P, Kyriakou, E, Konstantonis, D, et al. Platelet and coagulation disorders in newly diagnosed patients with pulmonary arterial hypertension. Platelets. (2019) 30:646–51. doi: 10.1080/09537104.2018.1499890
174.Thenappan, T, Chan, SY, and Weir, EK. Role of extracellular matrix in the pathogenesis of pulmonary arterial hypertension. Am J Physiol Heart Circ Physiol. (2018) 315:H1322–31. doi: 10.1152/ajpheart.00136.2018
175.Aldred, MA, Comhair, SA, Varella-Garcia, M, Asosingh, K, Xu, W, Noon, GP, et al. Somatic chromosome abnormalities in the lungs of patients with pulmonary arterial hypertension. Am J Respir Crit Care Med. (2010) 182:1153–60. doi: 10.1164/rccm.201003-0491OC
176.Thenappan, T, Ormiston, ML, Ryan, JJ, and Archer, SL. Pulmonary arterial hypertension: pathogenesis and clinical management. BMJ. (2018) 360:j5492. doi: 10.1136/bmj.j5492
177.Wang, X-J, Lian, T-Y, Jiang, X, Liu, S-F, Li, S-Q, Jiang, R, et al. Germline BMP9 mutation causes idiopathic pulmonary arterial hypertension. Eur Respir J. (2019) 53:1801609. doi: 10.1183/13993003.01609-2018
178.Hurst, LA, Dunmore, BJ, Long, L, Crosby, A, Al-Lamki, R, Deighton, J, et al. TNFα drives pulmonary arterial hypertension by suppressing the BMP type-II receptor and altering NOTCH signalling. Nat Commun. (2017) 8:14079. doi: 10.1038/ncomms14079
179.Diebold, I, Hennigs, JK, Miyagawa, K, Li, CG, Nickel, NP, Kaschwich, M, et al. BMPR2 preserves mitochondrial function and DNA during reoxygenation to promote endothelial cell survival and reverse pulmonary hypertension. Cell Metab. (2015) 21:596–608. doi: 10.1016/j.cmet.2015.03.010
180.Long, L, Ormiston, ML, Yang, X, Southwood, M, Gräf, S, Machado, RD, et al. Selective enhancement of endothelial BMPR-II with BMP9 reverses pulmonary arterial hypertension. Nat Med. (2015) 21:777–85. doi: 10.1038/nm.3877
181.Aldred, MA, Vijayakrishnan, J, James, V, Soubrier, F, Gomez-Sanchez, MA, Martensson, G, et al. BMPR2 gene rearrangements account for a significant proportion of mutations in familial and idiopathic pulmonary arterial hypertension. Hum Mutat. (2006) 27:212–3. doi: 10.1002/humu.9398
182.Cogan, JD, Pauciulo, MW, Batchman, AP, Prince, MA, Robbins, IM, Hedges, LK, et al. High frequency of BMPR2 exonic deletions/duplications in familial pulmonary arterial hypertension. Am J Respir Crit Care Med. (2006) 174:590–8. doi: 10.1164/rccm.200602-165OC
183.Evans, JDW, Girerd, B, Montani, D, Wang, X-J, Galiè, N, Austin, ED, et al. BMPR2 mutations and survival in pulmonary arterial hypertension: an individual participant data meta-analysis. Lancet Respir Med. (2016) 4:129–37. doi: 10.1016/S2213-2600(15)00544-5
184.Lavoie, JR, Ormiston, ML, Perez-Iratxeta, C, Courtman, DW, Jiang, B, Ferrer, E, et al. Proteomic analysis implicates translationally controlled tumor protein as a novel mediator of occlusive vascular remodeling in pulmonary arterial hypertension. Circulation. (2014) 129:2125–35. doi: 10.1161/CIRCULATIONAHA.114.008777
185.Brock, M, Samillan, VJ, Trenkmann, M, Schwarzwald, C, Ulrich, S, Gay, RE, et al. AntagomiR directed against miR-20a restores functional BMPR2 signalling and prevents vascular remodelling in hypoxia-induced pulmonary hypertension. Eur Heart J. (2014) 35:3203–11. doi: 10.1093/eurheartj/ehs060
186.Ferrer, E, Dunmore, BJ, Hassan, D, Ormiston, ML, Moore, S, Deighton, J, et al. A potential role for exosomal translationally controlled tumor protein export in vascular remodeling in pulmonary arterial hypertension. Am J Respir Cell Mol Biol. (2018) 59:467–78. doi: 10.1165/rcmb.2017-0129OC
187.Zhang, C, Wang, P, Mohammed, A, Zhou, Z, Zhang, S, Ni, S, et al. Function of adipose-derived mesenchymal stem cells in monocrotaline-induced pulmonary arterial hypertension through miR-191 via regulation of BMPR2. Biomed Res Int. (2019) 2019:2858750. doi: 10.1155/2019/2858750
188.Wei, C, Henderson, H, Spradley, C, Li, L, Kim, I-K, Kumar, S, et al. Circulating miRNAs as potential marker for pulmonary hypertension. PLoS One. (2013) 8:e64396. doi: 10.1371/journal.pone.0064396
189.Zhang, Z, Ge, L, Zhang, S, Wang, J, Jiang, W, Xin, Q, et al. The protective effects of MSC-EXO against pulmonary hypertension through regulating Wnt5a/BMP signalling pathway. J Cell Mol Med. (2020) 24:13938–48. doi: 10.1111/jcmm.16002
190.Archer, SL, Gomberg-Maitland, M, Maitland, ML, Rich, S, Garcia, JGN, and Weir, EK. Mitochondrial metabolism, redox signaling, and fusion: a mitochondria-ROS-HIF-1alpha-Kv1.5 O2-sensing pathway at the intersection of pulmonary hypertension and cancer. Am J Physiol Heart Circ Physiol. (2008) 294:H570–8. doi: 10.1152/ajpheart.01324.2007
191.Fijalkowska, I, Xu, W, Comhair, SAA, Janocha, AJ, Mavrakis, LA, Krishnamachary, B, et al. Hypoxia inducible-factor1alpha regulates the metabolic shift of pulmonary hypertensive endothelial cells. Am J Pathol. (2010) 176:1130–8. doi: 10.2353/ajpath.2010.090832
192.Peng, H, Xiao, Y, Deng, X, Luo, J, Hong, C, and Qin, X. The warburg effect: a new story in pulmonary arterial hypertension. Clin Chim Acta. (2016) 461:53–8. doi: 10.1016/j.cca.2016.07.017
193.Chettimada, S, Gupte, R, Rawat, D, Gebb, SA, McMurtry, IF, and Gupte, SA. Hypoxia-induced glucose-6-phosphate dehydrogenase overexpression and -activation in pulmonary artery smooth muscle cells: implication in pulmonary hypertension. Am J Physiol Lung Cell Mol Physiol. (2015) 308:L287–300. doi: 10.1152/ajplung.00229.2014
194.Rafikova, O, Srivastava, A, Desai, AA, Rafikov, R, and Tofovic, SP. Recurrent inhibition of mitochondrial complex III induces chronic pulmonary vasoconstriction and glycolytic switch in the rat lung. Respir Res. (2018) 19:69. doi: 10.1186/s12931-018-0776-1
195.Kumar, S, Frid, MG, Zhang, H, Li, M, Riddle, S, Brown, RD, et al. Complement-containing small extracellular vesicles from adventitial fibroblasts induce proinflammatory and metabolic reprogramming in macrophages. JCI Insight. (2021) 6:e148382. doi: 10.1172/jci.insight.148382
196.Corssac, GB, Bonetto, JP, Campos-Carraro, C, Cechinel, LR, Zimmer, A, Parmeggiani, B, et al. Pulmonary arterial hypertension induces the release of circulating extracellular vesicles with oxidative content and alters redox and mitochondrial homeostasis in the brains of rats. Hypertens Res. (2021) 44:918–31. doi: 10.1038/s41440-021-00660-y
197.Sekar, D. Extracellular vesicles are involved in oxidative stress and mitochondrial homeostasis in pulmonary arterial hypertension. Hypertens Res. (2021) 44:1028–9. doi: 10.1038/s41440-021-00672-8
198.Hogan, SE, Rodriguez Salazar, MP, Cheadle, J, Glenn, R, Medrano, C, Petersen, TH, et al. Mesenchymal stromal cell-derived exosomes improve mitochondrial health in pulmonary arterial hypertension. Am J Physiol Lung Cell Mol Physiol. (2019) 316:L723–37. doi: 10.1152/ajplung.00058.2018
199.Phinney, DG, Di Giuseppe, M, Njah, J, Sala, E, Shiva, S, St Croix, CM, et al. Mesenchymal stem cells use extracellular vesicles to outsource mitophagy and shuttle microRNAs. Nat Commun. (2015) 6:8472. doi: 10.1038/ncomms9472
200.Deng, L, Blanco, FJ, Stevens, H, Lu, R, Caudrillier, A, McBride, M, et al. MicroRNA-143 activation regulates smooth muscle and endothelial cell crosstalk in pulmonary arterial hypertension. Circ Res. (2015) 117:870–83. doi: 10.1161/CIRCRESAHA.115.306806
201.Zhang, M, Xin, W, Ma, C, Zhang, H, Mao, M, Liu, Y, et al. Exosomal 15-LO2 mediates hypoxia-induced pulmonary artery hypertension in vivo and in vitro. Cell Death Dis. (2018) 9:1022. doi: 10.1038/s41419-018-1073-0
202.Aliotta, JM, Pereira, M, Wen, S, Dooner, MS, Del Tatto, M, Papa, E, et al. Exosomes induce and reverse monocrotaline-induced pulmonary hypertension in mice. Cardiovasc Res. (2016) 110:319–30. doi: 10.1093/cvr/cvw054
203.Khandagale, A, Corcoran, P, Nikpour, M, Isaksson, A, Wikström, G, Siegbahn, A, et al. MircoRNA in extracellular vesicles from patients with pulmonary arterial hypertension alters endothelial angiogenic response. Int J Mol Sci. (2022) 23:11964. doi: 10.3390/ijms231911964
204.Li, Q, Xu, Y, Lv, K, Wang, Y, Zhong, Z, Xiao, C, et al. Small extracellular vesicles containing miR-486-5p promote angiogenesis after myocardial infarction in mice and nonhuman primates. Sci Transl Med. (2021) 13:eabb0202. doi: 10.1126/scitranslmed.abb0202
205.Zhu, Y, Lu, Y, Zhang, Q, Liu, J-J, Li, T-J, Yang, J-R, et al. MicroRNA-26a/b and their host genes cooperate to inhibit the G1/S transition by activating the pRb protein. Nucleic Acids Res. (2012) 40:4615–25. doi: 10.1093/nar/gkr1278
206.Tian, F, Wang, J, Ouyang, T, Lu, N, Lu, J, Shen, Y, et al. MiR-486-5p serves as a good biomarker in nonsmall cell lung cancer and suppresses cell growth with the involvement of a target PIK3R1. Front Genet. (2019) 10:688. doi: 10.3389/fgene.2019.00688
207.Sun, X-H, Wang, X, Zhang, Y, and Hui, J. Exosomes of bone-marrow stromal cells inhibit cardiomyocyte apoptosis under ischemic and hypoxic conditions via miR-486-5p targeting the PTEN/PI3K/AKT signaling pathway. Thromb Res. (2019) 177:23–32. doi: 10.1016/j.thromres.2019.02.002
208.Hassoun, PM. Pulmonary arterial hypertension. N Engl J Med. (2021) 385:2361–76. doi: 10.1056/NEJMra2000348
209.Sindi, HA, Russomanno, G, Satta, S, Abdul-Salam, VB, Jo, KB, Qazi-Chaudhry, B, et al. Therapeutic potential of KLF2-induced exosomal microRNAs in pulmonary hypertension. Nat Commun. (2020) 11:1185. doi: 10.1038/s41467-020-14966-x
210.Zhang, R, Su, H, Ma, X, Xu, X, Liang, L, Ma, G, et al. MiRNA let-7b promotes the development of hypoxic pulmonary hypertension by targeting ACE2. Am J Physiol Lung Cell Mol Physiol. (2019) 316:L547–57. doi: 10.1152/ajplung.00387.2018
211.Wang, J, Hu, L, Huang, H, Yu, Y, Wang, J, Yu, Y, et al. CAR (CARSKNKDC) peptide modified ReNcell-derived extracellular vesicles as a novel therapeutic agent for targeted pulmonary hypertension therapy. Hypertension. (2020) 76:1147–60. doi: 10.1161/HYPERTENSIONAHA.120.15554
212.Chen, T, Sun, MR, Zhou, Q, Guzman, AM, Ramchandran, R, Chen, J, et al. Extracellular vesicles derived from endothelial cells in hypoxia contribute to pulmonary artery smooth muscle cell proliferation in-vitro and pulmonary hypertension in mice. Pulm Circ. (2022) 12:e12014. doi: 10.1002/pul2.12014
213.Lee, C, Mitsialis, SA, Aslam, M, Vitali, SH, Vergadi, E, Konstantinou, G, et al. Exosomes mediate the cytoprotective action of mesenchymal stromal cells on hypoxia-induced pulmonary hypertension. Circulation. (2012) 126:2601–11. doi: 10.1161/CIRCULATIONAHA.112.114173
214.Klinger, JR, Pereira, M, Del Tatto, M, Brodsky, AS, Wu, KQ, Dooner, MS, et al. Mesenchymal stem cell extracellular vesicles reverse sugen/hypoxia pulmonary hypertension in rats. Am J Respir Cell Mol Biol. (2020) 62:577–87. doi: 10.1165/rcmb.2019-0154OC
215.Mo, J-S, Park, Y-R, and Chae, S-C. MicroRNA 196B regulates HOXA5, HOXB6 and GLTP expression levels in colorectal cancer cells. Pathol Oncol Res. (2019) 25:953–9. doi: 10.1007/s12253-018-0399-3
216.Yuan, Y, Lin, D, Feng, L, Huang, M, Yan, H, Li, Y, et al. Upregulation of miR-196b-5p attenuates BCG uptake via targeting SOCS3 and activating STAT3 in macrophages from patients with long-term cigarette smoking-related active pulmonary tuberculosis. J Transl Med. (2018) 16:284. doi: 10.1186/s12967-018-1654-9
217.Cao, W, Zhang, T, Feng, R, Xia, T, Huang, H, Liu, C, et al. Hoxa5 alleviates obesity-induced chronic inflammation by reducing ER stress and promoting M2 macrophage polarization in mouse adipose tissue. J Cell Mol Med. (2019) 23:7029–42. doi: 10.1111/jcmm.14600
218.Li, Y, Ren, W, Wang, X, Yu, X, Cui, L, Li, X, et al. MicroRNA-150 relieves vascular remodeling and fibrosis in hypoxia-induced pulmonary hypertension. Biomed Pharmacother. (2019) 109:1740–9. doi: 10.1016/j.biopha.2018.11.058
219.Rani, S, Ryan, AE, Griffin, MD, and Ritter, T. Mesenchymal stem cell-derived extracellular vesicles: toward cell-free therapeutic applications. Mol Ther. (2015) 23:812–23. doi: 10.1038/mt.2015.44
220.de Abreu, RC, Fernandes, H, da Costa Martins, PA, Sahoo, S, Emanueli, C, and Ferreira, L. Native and bioengineered extracellular vesicles for cardiovascular therapeutics. Nat Rev Cardiol. (2020) 17:685–97. doi: 10.1038/s41569-020-0389-5
221.Garofalo, M, Villa, A, Crescenti, D, Marzagalli, M, Kuryk, L, Limonta, P, et al. Heterologous and cross-species tropism of cancer-derived extracellular vesicles. Theranostics. (2019) 9:5681–93. doi: 10.7150/thno.34824
222.Emam, SE, Abu Lila, AS, Elsadek, NE, Ando, H, Shimizu, T, Okuhira, K, et al. Cancer cell-type tropism is one of crucial determinants for the efficient systemic delivery of cancer cell-derived exosomes to tumor tissues. Eur J Pharm Biopharm. (2019) 145:27–34. doi: 10.1016/j.ejpb.2019.10.005
223.Zhu, X, Badawi, M, Pomeroy, S, Sutaria, DS, Xie, Z, Baek, A, et al. Comprehensive toxicity and immunogenicity studies reveal minimal effects in mice following sustained dosing of extracellular vesicles derived from HEK293T cells. J Extracell Vesicles. (2017) 6:1324730. doi: 10.1080/20013078.2017.1324730
224.Wang, C, Li, N, Li, Y, Hou, S, Zhang, W, Meng, Z, et al. Engineering a HEK-293T exosome-based delivery platform for efficient tumor-targeting chemotherapy/internal irradiation combination therapy. J Nanobiotechnology. (2022) 20:247. doi: 10.1186/s12951-022-01462-1
225.Chen, Q, Liu, Y, Ding, X, Li, Q, Qiu, F, Wang, M, et al. Bone marrow mesenchymal stem cell-secreted exosomes carrying microRNA-125b protect against myocardial ischemia reperfusion injury via targeting SIRT7. Mol Cell Biochem. (2020) 465:103–14. doi: 10.1007/s11010-019-03671-z
226.Han, C, Zhou, J, Liu, B, Liang, C, Pan, X, Zhang, Y, et al. Delivery of miR-675 by stem cell-derived exosomes encapsulated in silk fibroin hydrogel prevents aging-induced vascular dysfunction in mouse hindlimb. Mater Sci Eng C. (2019) 99:322–32. doi: 10.1016/j.msec.2019.01.122
227.Kojima, R, Bojar, D, Rizzi, G, Hamri, GC-E, El-Baba, MD, Saxena, P, et al. Designer exosomes produced by implanted cells intracerebrally deliver therapeutic cargo for Parkinson’s disease treatment. Nat Commun. (2018) 9:1305. doi: 10.1038/s41467-018-03733-8
228.Zhao, L, Gu, C, and Gan, Y. Exosome-mediated siRNA delivery to suppress postoperative breast cancer metastasis. J Control Release. (2020) 318:1–15. doi: 10.1016/j.jconrel.2019.12.005
229.Sun, W, Zhao, P, Zhou, Y, Xing, C, Zhao, L, Li, Z, et al. Ultrasound targeted microbubble destruction assisted exosomal delivery of miR-21 protects the heart from chemotherapy associated cardiotoxicity. Biochem Biophys Res Commun. (2020) 532:60–7. doi: 10.1016/j.bbrc.2020.05.044
230.Kim, MS, Haney, MJ, Zhao, Y, Yuan, D, Deygen, I, Klyachko, NL, et al. Engineering macrophage-derived exosomes for targeted paclitaxel delivery to pulmonary metastases: in vitro and in vivo evaluations. Nanomedicine. (2018) 14:195–204. doi: 10.1016/j.nano.2017.09.011
231.Kalani, A, Chaturvedi, P, Kamat, PK, Maldonado, C, Bauer, P, Joshua, IG, et al. Curcumin-loaded embryonic stem cell exosomes restored neurovascular unit following ischemia-reperfusion injury. Int J Biochem Cell Biol. (2016) 79:360–9. doi: 10.1016/j.biocel.2016.09.002
232.Qu, M, Lin, Q, Huang, L, Fu, Y, Wang, L, He, S, et al. Dopamine-loaded blood exosomes targeted to brain for better treatment of Parkinson’s disease. J Control Release. (2018) 287:156–66. doi: 10.1016/j.jconrel.2018.08.035
233.Xu, X, Liang, Y, Li, X, Ouyang, K, Wang, M, Cao, T, et al. Exosome-mediated delivery of kartogenin for chondrogenesis of synovial fluid-derived mesenchymal stem cells and cartilage regeneration. Biomaterials. (2021) 269:120539. doi: 10.1016/j.biomaterials.2020.120539
234.Wang, X, Chen, Y, Zhao, Z, Meng, Q, Yu, Y, Sun, J, et al. Engineered exosomes with ischemic myocardium-targeting peptide for targeted therapy in myocardial infarction. J Am Heart Assoc. (2018) 7:e008737. doi: 10.1161/JAHA.118.008737
235.Tian, T, Cao, L, He, C, Ye, Q, Liang, R, You, W, et al. Targeted delivery of neural progenitor cell-derived extracellular vesicles for anti-inflammation after cerebral ischemia. Theranostics. (2021) 11:6507–21. doi: 10.7150/thno.56367
236.Kooijmans, S, Fliervoet, LAL, van der Meel, R, Fens, MHAM, Heijnen, HFG, van Bergen en Henegouwen, PMP, et al. PEGylated and targeted extracellular vesicles display enhanced cell specificity and circulation time. J Control Release. (2016) 224:77–85. doi: 10.1016/j.jconrel.2016.01.009
237.Tertel, T, Schoppet, M, Stambouli, O, Al-Jipouri, A, James, PF, and Giebel, B. Imaging flow cytometry challenges the usefulness of classically used extracellular vesicle labeling dyes and qualifies the novel dye Exoria for the labeling of mesenchymal stromal cell-extracellular vesicle preparations. Cytotherapy. (2022) 24:619–28. doi: 10.1016/j.jcyt.2022.02.003
238.Tian, Y, Li, S, Song, J, Ji, T, Zhu, M, Anderson, GJ, et al. A doxorubicin delivery platform using engineered natural membrane vesicle exosomes for targeted tumor therapy. Biomaterials. (2014) 35:2383–90. doi: 10.1016/j.biomaterials.2013.11.083
239.Kamerkar, S, LeBleu, VS, Sugimoto, H, Yang, S, Ruivo, CF, Melo, SA, et al. Exosomes facilitate therapeutic targeting of oncogenic KRAS in pancreatic cancer. Nature. (2017) 546:498–503. doi: 10.1038/nature22341
240.Tario, JD, Humphrey, K, Bantly, AD, Muirhead, KA, Moore, JS, and Wallace, PK. Optimized staining and proliferation modeling methods for cell division monitoring using cell tracking dyes. J Vis Exp. (2012):e4287. doi: 10.3791/4287
241.Wang, J, Li, W, Lu, Z, Zhang, L, Hu, Y, Li, Q, et al. The use of RGD-engineered exosomes for enhanced targeting ability and synergistic therapy toward angiogenesis. Nanoscale. (2017) 9:15598–605. doi: 10.1039/C7NR04425A
242.Roberts-Dalton, HD, Cocks, A, Falcon-Perez, JM, Sayers, EJ, Webber, JP, Watson, P, et al. Fluorescence labelling of extracellular vesicles using a novel thiol-based strategy for quantitative analysis of cellular delivery and intracellular traffic. Nanoscale. (2017) 9:13693–706. doi: 10.1039/C7NR04128D
243.Han, Q, Xie, QR, Li, F, Cheng, Y, Wu, T, Zhang, Y, et al. Targeted inhibition of SIRT6 via engineered exosomes impairs tumorigenesis and metastasis in prostate cancer. Theranostics. (2021) 11:6526–41. doi: 10.7150/thno.53886
244.Görgens, A, Bremer, M, Ferrer-Tur, R, Murke, F, Tertel, T, Horn, PA, et al. Optimisation of imaging flow cytometry for the analysis of single extracellular vesicles by using fluorescence-tagged vesicles as biological reference material. J Extracell Vesicles. (2019) 8:1587567. doi: 10.1080/20013078.2019.1587567
245.Zhou, F, Ebea, P, Mutai, E, Wang, H, Sukreet, S, Navazesh, S, et al. Small extracellular vesicles in milk cross the blood-brain barrier in murine cerebral cortex endothelial cells and promote dendritic complexity in the hippocampus and brain function in C57BL/6J mice. Front Nutr. (2022) 9:838543. doi: 10.3389/fnut.2022.838543
246.Salunkhe, S, Dheeraj,, Basak, M, Chitkara, D, and Mittal, A. Surface functionalization of exosomes for target-specific delivery and in vivo imaging & tracking: strategies and significance. J Control Release. (2020) 326:599–614. doi: 10.1016/j.jconrel.2020.07.042
247.Martinez, E, de Castilla, P, Tong, L, Huang, C, Sofias, AM, Pastorin, G, et al. Extracellular vesicles as a drug delivery system: a systematic review of preclinical studies. Adv Drug Deliv Rev. (2021) 175:113801. doi: 10.1016/j.addr.2021.05.011
248.Rashid, MH, Borin, TF, Ara, R, Angara, K, Cai, J, Achyut, BR, et al. Differential in vivo biodistribution of 131I-labeled exosomes from diverse cellular origins and its implication for theranostic application. Nanomedicine. (2019) 21:102072. doi: 10.1016/j.nano.2019.102072
249.Ahn, S-H, Ryu, S-W, Choi, H, You, S, Park, J, and Choi, C. Manufacturing therapeutic exosomes: from bench to industry. Mol Cells. (2022) 45:284–90. doi: 10.14348/molcells.2022.2033
250.Zhang, J, He, Y, Yan, X, Chen, S, He, M, Lei, Y, et al. MicroRNA-483 amelioration of experimental pulmonary hypertension. EMBO Mol Med. (2020) 12:e11303. doi: 10.15252/emmm.201911303
251.Huang, Y, Wang, Z-G, Tang, L, Gong, S-G, Sun, Y-Y, Wang, L, et al. Plasma exosomal miR-596: a novel biomarker predicts survival in patients with idiopathic pulmonary artery hypertension. J Int Med Res. (2021) 49:3000605211002379. doi: 10.1177/03000605211002379
252.Lipps, C, Northe, P, Figueiredo, R, Rohde, M, Brahmer, A, Krämer-Albers, E-M, et al. Non-invasive approach for evaluation of pulmonary hypertension using extracellular vesicle-associated small non-coding RNA. Biomol Ther. (2019) 9:E666. doi: 10.3390/biom9110666
253.Foster, WS, Suen, CM, and Stewart, DJ. Regenerative cell and tissue-based therapies for pulmonary arterial hypertension. Can J Cardiol. (2014) 30:1350–60. doi: 10.1016/j.cjca.2014.08.022
254.Komanduri, KV, Couriel, D, and Champlin, RE. Graft-versus-host disease after allogeneic stem cell transplantation: evolving concepts and novel therapies including photopheresis. Biol Blood Marrow Transplant. (2006) 12:1–6. doi: 10.1016/j.bbmt.2005.11.003
Keywords: exosomes, extracellular vesicles, miRNA, vascular remodeling, essential hypertension, atherosclerosis, pulmonary arterial hypertension
Citation: Ren Y and Zhang H (2023) Emerging role of exosomes in vascular diseases. Front. Cardiovasc. Med. 10:1090909. doi: 10.3389/fcvm.2023.1090909
Edited by:
Raquel Guillamat-Prats, LMU Munich University Hospital, GermanyReviewed by:
Shiyou Chen, University of Missouri, United StatesAlexander N. Kapustin, AstraZeneca, United Kingdom
Copyright © 2023 Ren and Zhang. This is an open-access article distributed under the terms of the Creative Commons Attribution License (CC BY). The use, distribution or reproduction in other forums is permitted, provided the original author(s) and the copyright owner(s) are credited and that the original publication in this journal is cited, in accordance with accepted academic practice. No use, distribution or reproduction is permitted which does not comply with these terms.
*Correspondence: Honggang Zhang, emhhbmdoZzE5NjYxMjZAMTYzLmNvbQ==
†ORCID: Yi Ren https://orcid.org/0000-0001-5219-8889
Honggang Zhang https://orcid.org/0000-0002-6837-8443