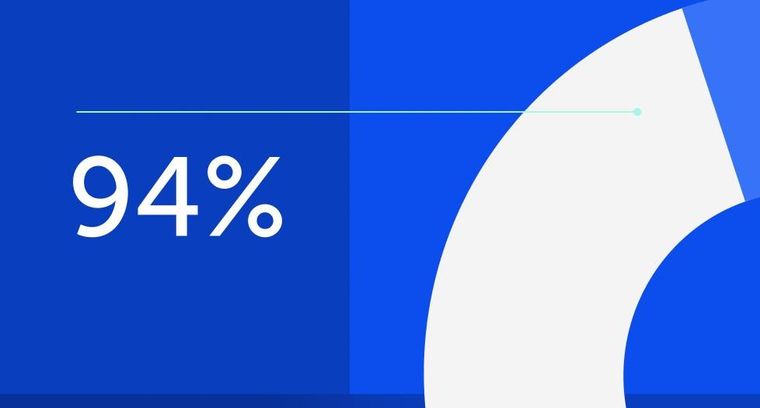
94% of researchers rate our articles as excellent or good
Learn more about the work of our research integrity team to safeguard the quality of each article we publish.
Find out more
REVIEW article
Front. Cardiovasc. Med., 21 April 2023
Sec. Sex and Gender in Cardiovascular Medicine
Volume 10 - 2023 | https://doi.org/10.3389/fcvm.2023.1072042
This article is part of the Research TopicThe Role of Sex in Coronary Artery DiseaseView all 8 articles
Stress is an important risk factor for modern chronic diseases, with distinct influences in males and females. The sex specificity of the mammalian stress response contributes to the sex-dependent development and impacts of coronary artery disease (CAD). Compared to men, women appear to have greater susceptibility to chronic forms of psychosocial stress, extending beyond an increased incidence of mood disorders to include a 2- to 4-fold higher risk of stress-dependent myocardial infarction in women, and up to 10-fold higher risk of Takotsubo syndrome—a stress-dependent coronary-myocardial disorder most prevalent in post-menopausal women. Sex differences arise at all levels of the stress response: from initial perception of stress to behavioural, cognitive, and affective responses and longer-term disease outcomes. These fundamental differences involve interactions between chromosomal and gonadal determinants, (mal)adaptive epigenetic modulation across the lifespan (particularly in early life), and the extrinsic influences of socio-cultural, economic, and environmental factors. Pre-clinical investigations of biological mechanisms support distinct early life programming and a heightened corticolimbic-noradrenaline-neuroinflammatory reactivity in females vs. males, among implicated determinants of the chronic stress response. Unravelling the intrinsic molecular, cellular and systems biological basis of these differences, and their interactions with external lifestyle/socio-cultural determinants, can guide preventative and therapeutic strategies to better target coronary heart disease in a tailored sex-specific manner.
The mammalian stress response modulates whole body physiology and behaviour to enhance survival in the face of acute environmental threats. Unfortunately, prolonged or repetitive activation of this survival response—via a diversity of psychosocial, economic and environmental stressors prevalent in modern society—detrimentally impacts physiology, mood and behaviour to promote major diseases “plaguing” modern populations. These psychosocial stress dependent diseases include major depressive disorder (MDD) (1), obesity, metabolic syndrome and diabetes (2), osteoporosis (3), cancers (4) and cardiovascular disease (CVD) (5). They are also frequently co- or multi-morbid, consistent with these “diseases of modernity” sharing mechanistic networks (6). Modern stress-dependent syndemics are also emerging, with attention to stress and CVD linked synergistic conditions (7, 8), and most recently to stress and the COVID-19 mental health syndemic (9–11).
A primary focus in stress research has been the development of mood disorders, and though the mechanistic basis of stress-dependent MDD remains elusive, these studies provide a (growing) catalogue of the biological influences of chronic stress, including mechanisms likely participating in the positive relationship between stress and CAD. These include changes in nervous and endocrine control of the cardiovascular system, whole body metabolism, immuno-inflammatory function and gut biology, together with affective/behavioural responses that reinforce cardiometabolic disease development (including inactivity, hyperphagia and biased selection of palatable sugar- and fat-rich foods). Critically, the mammalian stress response and its systemic influences are highly sex-dependent, a fundamental yet still under-studied basis for sex specific disease risks and outcomes (12).
Psychosocial and other forms of stress are powerful “unconventional” risk factors for CVD (5). Indeed, animal studies suggest stress may be a stronger determinant of coronary disease than cholesterol and associated lipid levels (13). This pathogenic influence was initially flagged by Selye shortly after his pioneering work defining stress, postulating that chronic stress may be linked to cardiovascular disease (14). Since that time the cardiovascular impacts of stress have been interrogated in pre-clinical, clinical and epidemiological investigations. The latter identify significant influences of diverse stressors on CVD. Psychosocial stress promotes atherosclerosis, CAD and acute myocardial infarction (AMI) (15–17). The INTERHEART study, for example, found risk of AMI was more than 2-fold higher in people reporting “permanent stress” (18). Both personal and work stressors have been linked to up to a 50% increase in CAD (5). The Stockholm Heart Program (SHEEP study group) found AMI patients were more likely to have high level work stress (high work demands vs. low control) (19). The Whitehall II study of British civil servants revealed a >2-fold increase in CVD risk in men experiencing a mismatch between work effort and reward (20), and 1.4-fold increased CAD risk in both men and women experiencing job insecurity (21). Analysis of Jackson Heart Study data indicates a 2.4-fold increased risk of CAD with medium to high level financial stress (an association largely explained by depression, together with smoking and diabetes) (22). Non-obstructive forms of coronary disease are also significantly related to psychological stress and a “distressed” personality (23).
Acute episodes of mental stress, more commonly encountered than chronic forms of stress, are also strongly linked to incidence of cardiovascular events (24, 25). Indeed, the pathological influences of acute “trigger” stressors can appear stronger than pro-disease effects of chronic stress (24). For example, earthquake has been linked to up to a 5-fold increase in sudden cardiac death (26). Acute emotional upset is frequently reported by patients in the hrs immediately prior to AMI (27, 28). Analysis of INTERHEART data suggests emotional upset increases the risk of AMI almost 2.5-fold (27), while meta-analysis supports an almost 5-fold increase in risk of infarction or acute coronary syndrome (ACS) within 2 h of an acute anger episode (29). While still incompletely understood, the pathophysiology of spontaneous coronary artery dissection (SCAD)—an important determinant of AMI and sudden death—may also involve acute psychosocial or physical stress (30, 31).
The associations between stress and chronic disease development are strongly sex-dependent, however the importance of sex in these linkages remains understudied. Recognising the need to address this historic paucity of research, investigators have belatedly focussed attention on sex differences (32), including in stress biology and its influence on cardiovascular or mood disorder development (33). Although females may be conferred some protection against CAD development, this is evident prior to menopause (34), whereas post-menopausal women may be at greater risk of AMI than men and suffer greater morbidity and mortality (35). Both short- (36) and long-term outcomes (37) appear worsened in women, though these differences may reflect in part higher age and co-morbidities (38) and are minimised in high-quality clinical settings (39). Nonetheless, considerable evidence indicates that short- and long- term outcomes in acute coronary syndromes are consistently worse in young to middle-aged women relative to age-matched men (40, 41). A greater prevalence of anxiety and depression in women also reduces adherence to cardiac rehabilitation (42), contributing to worsened long-term outcomes. Sex differences in stress biology contribute to these biased outcomes. Indeed, while psychological stress significantly predicts coronary events in women, this is less evident in men (17). Women are at particularly heightened risk of stress-dependent SCAD (30, 31) and myocardial infarction (43,44), together with Takotsubo syndrome—a coronary-myocardial disorder induced by profound stress (45, 46). A higher female prevalence of heart failure with preserved ejection fraction (HfpEF) adds further support to the importance of sex in coronary related disorders, given the involvement of microvascular dysfunction and inflammation in this condition (47).
In this review we briefly outline the mammalian stress response and consider how it differs fundamentally between the sexes, before discussing the mechanistic basis of psychosocial stress-dependent heart disease and evidence these processes are also highly sex-dependent.
Physical and psychological stimuli threatening or perceived to threaten wellbeing (stressors) induce a systemic stress response—a centrally controlled, integrated adaptation aimed at maintaining homeostasis and enhancing survival (Figure 1). This well conserved mammalian stress response involves 2 primary central mediators—the hypothalamic-pituitary-adrenal (HPA) axis and the autonomic nervous system (ANS). The renin-angiotensin-aldosterone system (RAAS) is also involved, linking stress to regulation of blood pressure and volume.
Figure 1. The mammalian stress response, and roles of sympathetic nervous system (SNS) and hypothalamic-pituitary-adrenal (HPA) axis. The SNS releases (1) noradrenaline from the splanchnic nerve (originating from sympathetic chain ganglia) and (2b) Adrenaline from the adrenal medulla [through activation of the sympathomedullary pathway (2a)]. Activation of the HPA axis involves release of corticotropin-releasing hormone (CRH) and other “releasing” hormones [including arginine vasopressin (AVP)] from paraventricular nucleus of hypothalamus (3). The release of CRH, AVP and other hormones acts on the anterior pituitary, resulting in release of β-endorphin (4) and adrenocorticotropin-releasing hormone (ACTH) (5). ACTH acts on the adrenal cortex to promote synthesis and release of glucocorticoid hormones; cortisol in humans and corticosterone in rodents (6). NB: solid line with arrow = act on; dashed line with arrow = release of; solid line = description; solid line with circle = zoom in.
The paraventricular nucleus (PVN) of the hypothalamus is the first brain region to respond to a real or perceived stressor, in turn promoting HPA axis and sympathetic nervous system (SNS) activities (Figure 1). Release of noradrenaline from peripheral nerves and adrenaline from the adrenal medulla is coupled with a decline in parasympathetic nervous system (PNS) activity, contributing to an autonomic imbalance. While normally tightly controlled, prolonged or chronic stress and HPA activation stimulates additional brain regions, including the amygdala and medulla oblongata, and leads to a hyper-stimulation of the PVN and the HPA axis. Hypothalamic neurons are stimulated to synthesize corticotropin releasing hormone (CRH) and arginine vasopressin (AVP). At the hypothalamic-pituitary unit of the axis, CRH is released into the hypophyseal portal system, with adrenocorticotropic hormone (ACTH) cleaved from its pro-opiomelanocortin precursor, simultaneously generating β-endorphin. This surge in ACTH stimulates adrenal cortical cells to release glucocorticoids and adrenal androgens (Figure 1). The primary glucocorticoid is species dependent—cortisol in humans and corticosterone in rodents—though functions and activities are similar.
Importantly, the initial perception of stress, activation of these stress pathways and their subsequent influences on physiology and behaviour appear to be highly sex-dependent. Transcriptomic profiling of brain responses to stress is informative regarding the extent of these differences (48, 49), which appear to be of a similar magnitude to the differences between rodent and human biology (50). Disentangling the integrated elements underlying the sex-specific stress response remains a major challenge. Sex differences involve a complex interplay between proximate biological mechanisms, including chromosomal and gonadal determinants of nervous system structure and function (51–53) that act developmentally and post-developmentally (54), molecular transduction of life history via epigenetic control across the lifespan (particularly in early life) (55, 56), and the influences of (sex-biased) socio-cultural (57), economic and environmental factors. Women may generally experience higher degrees of background stress in day-to-day life, for example as a result of ongoing inequities in unpaid domestic work and other stressors (58–61). In addition, social support and ranking substantially influence stress resilience and reactivity, however such effects differ considerably between the sexes (62, 63). Indeed, as argued by Cohen et al. (57), social construction of gender roles may largely explain sex differences in psychosocial stress reactivity.
Nonetheless, the mammalian stress response itself appears to be characterised by sex-specific features across organisational levels (64): from the initial perception and processing of stressors; to differing fear, cognitive and coping responses; functionality and reactivity of integrated HPA axis, sympatho-adrenergic and inflammatory pathways (including the locus coeruleus-noradrenaline-neuroinflammatory axis); neurotrophin signalling and neuroplasticity; and signalling via multiple endocrine and neurotransmitter systems. The gut microbiome, the gut-brain axis and its involvement in stress-related disease, also appear sex-dependent, as are the influences of early life programming/epigenetic control, and the conditioning effects of prior stress. Our own recent work supports greater biological stress or allostatic load in female vs. male rodents subjected to chronic social stress, with evidence of greater coronary dysfunction, anxiety-like behaviour, weight loss and inflammation in females (12). However, mixed findings emerge from different animal studies, including evidence females are more susceptible to neuroendocrine and behavioural disruption (and selectively more sensitive to the cardiovascular/autonomic effects of homotypic stress) while males are more vulnerable to somatic effects of chronic stress (65).
Since advantageous psychological traits differ between males and females during human evolution, Darwinian sexual selection provides a mechanistic basis for the emergence of behavioural/cognitive differences between the sexes (66). This process selects for traits that increase competitiveness in acquiring and fertilising mates. A general pattern is that females, with slower reproduction rates, invest more in parenting and mate selection, and less in intra-sexual competition for mates; while males with a faster reproduction rate invest less in parenting/mate selection, and more in competition. In Palaeolithic life females shared care of infants, food gathering and female-female social interactions, whereas males had differing roles and pressures, including social isolation for extended periods. Females thus invest in developing social support, and attracting supportive mates for reproduction, while males engage in rank behaviour to enhance access to multiple mates, acquire resources and maintain social status (67). Intra-sexual competition for mates favours aggression/territoriality. Sex-dependent responses to prenatal stress may also have an evolutionary origin: it may be adaptive for females to be more alert to dangers, thus more stress responsive (potentially predisposing to stress-related disorders) (68). As detailed below, males and females differ substantially in their initial perceptions of stress, stress reactivities, and cognitive and coping responses.
Differing perception of stress, and heightened reactivity, arousal and negative valence may contribute to the sex-dependence of stress-related disease development. Distinct cognitive and coping responses influence stress resilience and systemic outcomes.
What is perceived as a threat or stressor can be highly diverse at an individual level, influenced by extrinsic environmental (social, cultural, economic) and intrinsic (chromosomal, gonadal) determinants, together with the epigenetic transduction of life history and experience. Evidence has shown that females have a generally greater perception of stress [and also pain (69)], which may influence disease processes (70–73). Interestingly, while perceived stress and coping have been linked to stress-inflammatory function, there is also evidence of an inverse relationship in Japanese men (74, 75). The differing stress perception may contribute to hyperarousal, a maladaptive state leading to agitation, restlessness and cognitive disruption. A core feature of stress-related psychiatric disorders, hyperarousal is more pronounced in women than men (76, 77).
Fear conditioning and extinction, important in mitigating effects of repeated stressors, is mediated in animals and humans by circuitry linking prefrontal cortex and amygdala (78, 79). Fear responses and these neuronal circuits differ between the sexes (80–82), which may reflect differing neurogenesis responses (83), and predispose to a heightened stress reactivity in females.
Cognitive responses to stress influence outcomes, with evidence improved cognitive function reduces inflammatory reactivity. For example, Shields et al. (84), shows better cognitive control reduces salivary cytokine levels in response to a video stressor. Sex significantly influences cognitive strategies (85). Cognitive responses to stress differ between the sexes, involving in part gonadal hormone differences (86–88). There is evidence stress may impair decision making to a greater extent in females than males (89).
Determinants of sex-dependent stress responses encompass sex-specific genetic interactions (90), sex hormones (91) and associated endocrine changes (92). Animal studies confirm the importance of both gonadal and genetic sex (90), for example employing the four-core genotype mouse model (95, 96). Sex also oppositely affects gene modules related to stress (96), a finding also evidenced in humans (97). Immuno-inflammatory reactivity differs, with female-specific changes in peripheral (93) and central inflammation (94), and links between inflammation and behaviour (93, 98). Overall, differing behavioural and physiological influences of stress may be explained by sex differences in brain architecture and circuitry, the function and reactivity of the HPA (99, 100) and a locus coeruleus–noradrenergic-neuroinflammatory axis (101), together with neurotrophin signalling and the influences of early life experience and adversity (among other factors).
Recent neuroimaging studies support sexual dimorphisms in brain structure. The most agreed-upon difference is the macroscopic observation of a larger brain volume in men (102, 103), even after accounting for body size difference (103–105). Differences are also evident with respect to cortical and sub-cortical regions, including larger planum temporale and Sylvian fissure in males (106, 107), whereas a larger hippocampus, superior temporal cortex, Broca's area and caudate are observed in females (108, 109). Women also appear to have a proportionally higher grey:white matter ratio than men (110, 111). Relevant region-specific differences are also evident. For example, higher grey matter percentages or grey:white ratios are evident within the dorsolateral prefrontal cortex (112), superior temporal gyrus (112), and parietal lobe (104, 113) of the female brain. How such architecture differences might influence the stress response is unclear, however there is evidence linking stress resilience to neuro-anatomy, for example differences in limbic region structure and connectivity (114–116). A reduced hippocampal volume is also characteristic of stress-related depression in both men and women (117, 118).
Differences within specific neural circuits underlying emotional control and expression may be important: regions associated with negative valence, including the amygdala, frontal cortex and hippocampus, exhibit significant sex differences (119, 120). The magnitude of corticolimbic responses to emotional stimuli may also differ between sexes. Domes et al. (121) found that initial aversive stimuli increased amygdala activity to a greater extent in women than men. Stevens et al. (122) also observed sex differences in the amygdala response to emotional stimuli, with women favouring negative and men positive emotion. Negatively valanced words activate left perirhinal cortex and hippocampus in women and the right supramarginal gyrus in men (123). Garret et al. (124) found that chronic stress induces opposing dendritic atrophy and hypertrophy in the frontal cortex of male and female rats, respectively. Stressed females also exhibit longer and more complex dendrites in basolateral projecting neurons (125), an outcome not evident in males (126). These regional activity and circuitry differences contribute to sex specific stress axis activity and responses.
Prominent sex differences emerge in the HPA axis response to stress (127). In healthy participants, baseline cortisol levels may be comparable in women and men (128, 129), although this is challenged by recent reports of higher baseline concentrations in women (130, 131). This may be related in part to higher background levels of stress in women (59–61). Women with stress-related MDD also have higher cortisol levels than male patients (133). Rodent studies support both higher basal and stress-induced corticosterone levels in females vs. males (134–136), with females secreting higher corticosterone in response to either physical or psychological stressors (135, 137–148). Enhanced corticosterone responses in females involve both a more rapid elevation in the initial minutes of stress exposure (143, 149, 150), and a more sustained elevation in corticosterone levels (135, 149, 151). Sex differences in the corticosterone response are paralleled by higher stress-dependent ACTH levels in females (134, 144, 145, 149, 152, 153).
Differing glucocorticoid responses suggest generally increased stress axis reactivity in females (154, 155). This is also reflected in evidence of increased neuroinflammatory reactivity in females (156, 157). However, the simplistic notion that increased corticosterone/cortisol levels may underpin sex differences in behavioural outcomes and resilience must be tempered by evidence females may be more resistant to the effects of corticosterone (158–160). Moreover, the biological response to cortisol or corticosterone is itself highly sex-dependent, including distinct behavioural, neuroendocrine and miRNA responses (160, 161). Although corticosterone levels predict stress-dependent behavioural changes in males, this may not be the case in females (162).
Multiple mechanisms could contribute to differing HPA axis responses in females vs. males. Sex hormones influence HPA axis function (99, 154, 163–165), including up-regulation of hypothalamic CRH, which has oestrogen and androgen response elements in its genetic promoter region. There is also evidence of an interaction between oestrogen and corticosteroid binding globulin in differing sex-dependent stress reactivity (166). Considerable evidence supports a greater reactivity of the locus coeruleus (LC) norepinephrine system in females (101), important in hyperarousal (167–169) and stress-inflammatory activation. The LC of adult female rats is larger and contains more noradrenaline containing neurons than in males (170–172) and may receive greater synaptic input than in male rats (101). Increased LC-noradrenaline axis reactivity in females may involve: gonadal hormone up-regulation of hypothalamic CRH, which has oestrogen and androgen response elements within its gene promoter region; increased noradrenaline content, involving oestrogen-dependent up-regulation of synthesis/down-regulation of degradation (101); differing locus coeruleus neuronal number/ structure and dendritic morphology, promoting reactivity to psychosocial stimuli (173); differing trafficking of the CRH receptor, which may not desensitise as effectively in females (174, 175); and biased CRH signalling via CRH may increase HPA reactivity in females, with distinct receptor-effector coupling in each sex (175, 176).
An increased inflammatory reactivity to stress in females may arise as a result heightened LC-noradrenaline axis (coupled with reduced PNS) activity. Inflammatory challenge itself induces distinct behavioural outcomes in females (177–180). However, experimental findings are again equivocal, including evidence: of similar behavioural outcomes (sickness behaviour) in the face of differing levels of neuroinflammation (181); that sex differences in neuroinflammation do not underlie diverging affective-like behaviours; and that differences in inflammatory markers are not linked to differing corticosterone levels (182). There is also evidence for differing pro- and anti-inflammatory influences of other regulatory systems in males vs. females [e.g., cannabinoid receptor signalling (183)], complicating interpretation. Nonetheless, an array of findings provide support for the thesis that differing stress reactivity and disease development in females involves at least in part greater stress-inflammatory activation (184).
Experiential factors significantly influence stress resilience in later life, and these effects may differ between the sexes (185). Early life programming of stress reactivity and associated disease risks are sex-dependent, involving shifts in neuro-immune and other functions (180, 186–190), together with differences in neurogenesis/plasticity (88, 180, 190). There is evidence adverse childhood experience may have a greater impact on adaptive responses to stressors in later life in women vs. men (191). Dimorphic influences of chronic stress in later life may thus involve both prior programming effects and extant sex differences. Sex dependent influences of early life stress and palatable food intake include reportedly select hyperphagia/weight gain in females vs. metabolic programming (adrenal growth and declining adiponectin) in males (192). Prior experience of controllable stress also has a conditioning or pro-resilience effect, protecting against subsequent stress. However, animal studies suggest this benefit is effective in males and not females, potentially reflecting differing neuroplasticity (193). Other recent work indicates that early life influences synaptic organization/excitability in the neonatal amygdala in a sex dependent manner, governing anxiety and fear responses in later life (194, 195).
Differing neurogenesis and plasticity responses are evident in males and females, with both early life (196) and adult (197) stressors. Experimental studies support sex specific changes in neurogenesis with chronic restraint (198, 199) and social stress (197), for example. Among neurotrophins, much attention has focused on brain-derived neurotropic factor (BDNF) in stress-dependent disorders, and there is evidence central nervous system (CNS) BDNF signalling is selectively or more substantially disrupted in stressed females than males (200–203). This dimorphism may involve epigenetic (201) and inflammatory (203) mechanisms. Despite a focus on BDNF in CNS responses and affective outcomes, recent evidence implicates BDNF signalling in thrombosis and CAD (204, 205).
While relatively poorly studied, sex differences in pyrimidine nucleotide metabolism and related sirtuin signalling may be an important determinant of differing resilience to and outcomes from chronic stress in males and females. Stress responses, mitochondrial energy state and metabolic homeostasis, inflammation, oxidative stress, cell senescence and longevity are all sensitive to pyrimidine nucleotides and sirtuin signalling. Nicotinamide adenine dinucleotide (NAD+) is an essential pyrimidine nucleotide that serves as a cofactor for several hundred metabolic enzymes (206). NAD+ also plays a key role in regulating 3 major groups of NAD+ consuming enzymes: sirtuins, poly-ADP-ribose polymerases (PARPs), and CD38/157 ectoenzymes. Intracellular levels of NAD+ are reduced in tissues of physiologically aged animals (207, 208) and humans (209–211). Low NAD+ is linked to metabolic and age-related disorders (212), while a reduced NAD+/NADH redox state may promote cardiomyopathy (213). Chronic catecholamine stress in vitro reduces cellular NAD+ levels together with PARP-1 expression and activity (214), and chronic stress dependent behavioural changes in animal models have been linked to a NAD+–sirtuin-1 pathway (215). Maternal separation stress also modifies whole body NAD+ metabolism, with consistently elevated N-methylnicotinamide excretion consistent with net NAD+ catabolism (216). Endoplasmic reticulum stress can also repress central quinolinic acid phosphoribosyl transferase transcription, leading to a build-up of the NMDA receptor agonist and excitotoxin, increased oxidative stress and inflammation, and depletion in NAD+ levels, culminating in cell death (217, 218).
Supporting casual roles for NAD+ in stress-related disorders, nicotinamide mononucleotide supplementation reduces depressive-like behaviour in association with improved NAD+ levels, sirtuin-3 activity and mitochondrial energy metabolism in a model of chronic corticosterone stress (219). Schroeder and colleagues document dysregulation of NAD+ generation in the brains of chronic prenatally stressed mice, with NAD+ therapy preventing axonal degeneration, cognitive and depressive outcomes (220). The same NAD+ stabilising therapy counters aberrant maternal care behaviours arising with chronic gestational stress (221).
The sirtuins are at least partly responsible for the effects of NAD+ on responses to stress. Sirtuins have been reported to induce stress resistance in lower organisms and mammals (222). Sirtuin-1 is regulated by NAD+-dependent PARP-1 and modulates stress responses and resilience, with effects on inflammation, oxidative stress, mitochondrial genesis and metabolism, endothelial dysfunction, coagulopathy, cell senescence and longevity (223, 224). They also play a cardinal role in improving glucose metabolism and insulin secretion (225, 226) via increasing NAD+ levels (227). Stress related reductions in NAD+ therefore have broad implications for these sirtuin sensitive processes. However, stress also directly modifies sirtuin expression and results in inhibitory post-translational modifications, with these changes linked to neurodegenerative and other age-related diseases (228). Oxidative stress and CAD risk factors such as cigarette smoke are known to post-translationally inhibit sirtuin-1 and enhance degradation.
Support for the important of sirtuin-1 in chronic stress resilience includes a significant association between depression and a SIRT1 gene variant identified in a genome-wide sequencing study within a large population of Chinese women (229). Animal studies reveal reductions in hippocampal sirtuin-1 with chronic stress (230–232) and show that pharmacologic or genetic inhibition of sirtuin-1 increases stress-dependent behavioural changes and dendritic atrophy, while activation blocks these outcomes (231). Sirtuin-1 protection against these chronic stress effects may involve a microglial shift toward the anti-inflammatory M2 phenotype (233). Long-term cognitive impairment in response to chronic unpredictable stress in adolescent mice is also associated with increased cortical expression of the 50 kD vs. 110 kD sirtuin-1 isoforms and respective mRNAs (234). Other investigations identify roles for sirtuin-1 dependent pathways in protecting against stress-dependent behavioural dysfunction (235), while the beneficial CNS and behavioural effects of environmental enrichment (236) and hydrogen sulphide (237) have both been linked to sirtuin-1 dependent signalling in rat models of chronic unpredictable stress. The ability of resveratrol treatment to improve chronic stress resilience and outcomes is also linked to changes in central sirtuin-1 signalling (238). On the other hand, Ferland et al. (239), show select infusion of the sirtuin-1 inhibitor sirtinol into the dentate gyrus prevents chronic stress-dependent changes in stress kinase signalling and histone acetylation, corresponding with improved behavioural outcomes. This agrees with evidence sirtuin-1 inhibition may enhances neuroprotection against stress-related depressive behaviour, inflammation and oxidative stress (246). Regional differences in sirtuin changes are important, and may explain differing outcomes. As opposed to a fall n hippocampal sirtuin-1 and sirtuin-3, chronic stress reportedly increases sirtuin-1 in nucleus accumbens, and both pharmacological sirtuin-1 activation or sirtuin-1 overexpression within this region increases depression- and anxiety-like behaviours, while sirtuin-1 antagonist infusion counters these changes (240).
Chronic stress dependent reductions in hippocampal sirtuin-2 are also relevant, with associated depressive outcomes and impaired neurogenesis countered by hippocampal sirtuin-2 over-expression and mimicked by sirtuin-2 inhibition (241). Zhang et al. report that depressive outcomes in chronically stressed mice are associated with CDK5 mediated phosphorylation of sirtuin-2, and are blocked either by gene deletion of sirtuin-2 or inhibition of this phosphorylation process (242). Depressive-like symptoms in rats, and associated oxidative stress and telomere shortening may also involve inhibition of sirtuin-3 and a resultant decline anti-oxidant enzyme activity (243).
The importance of sex in stress-dependent changes in pyrimidine metabolism and sirtuin signalling are not well studied. However, a handful of studies support sex differences in NAD+ metabolism, and a number have revealed sex specific aspects of sirtuin signalling and stress responses in different tissues. Schwarzmann et al. (244) recently tested for sex differences in NAD+ and the NAD+ redox ratio in 91 men and 114 women between 18 and 83 years of age. Despite no significant differences in total plasma NAD+, a higher NAD+/NADH ratio was evident in women vs. men, a difference that declined with age. Thus, the ratio was higher in adult but not elderly women compared to age-matched men. The authors speculate there may be less cellular NAD+ release via connexin 43 hemichannels and/or increased NAD+ consumption via enzymes such as CD38 in men. In contrast, Yang et al. (2022) report higher circulating NAD+ levels in men than women in a study of 1,518 participants from the Jidong community, 18 years of age and over (245). Breton et al. (246), found no sex differences in blood NAD+ levels in a smaller French study, although there was a trend to an age-dependent decline in NAD+ in males and not females. Relationships between NAD+, sex hormones, and stress have yet to be explored in detail.
A larger, albeit still limited, body of evidence exists regarding sex and sirtuins, which play a role in differing susceptibilities of males and females to CVD (247) and other disorders. Analysis of human ventricular tissue from young and old subjects shows a sex- and age-dependent decline in cardiac sirtuin-1 and -3 expression in females but not males, consistent with declining mitochondrial antioxidant defences and increasing inflammation in female (not male) hearts (248). This may reflect reductions in oestrogenic induction of sirtuins in females. Sirtuin-1 induces sexually dimorphic effects on depressive behaviours in mice, with deletion in forebrain excitatory neurons inducing a depression-like phenotype specifically in male mice (249). Down-regulation of sirtuin-1 contributes to ovariectomy -induced arterial senescence and atherosclerosis in female apolipoprotein (Apo)E−/− mice, whereas oestrogen or a selective oestrogen receptor modulator up-regulate sirtuin-1 and counter these changes (250). Thus, protection against vascular dysfunction and atherosclerosis in females may involve the activity of an oestrogen/sirtuin-1 axis.
Sex-dependent sirtuin-3 signalling produces distinct effects on energy metabolism, coronary and myocardial diastolic function, and tissue stress resistance. Sirtuin-3 depletion in arcuate pro-opiomelanocortin neurons—critical regulators of metabolism—induces a negative energy balance (decreased body weight and adiposity, increased energy expenditure) specifically in male mice on a normal diet, and not in males fed a high fat diet or females on either regimen (251). Such effects may contribute to differing risks of obesity or weight loss with chronic stress in males and females. Sex-specific involvement of endothelial sirtuin-3 in determining blood pressure, coronary flow reserve and diastolic function in female vs. male mice (252) suggests age- or disease related coronary and diastolic dysfunction in females may involve reductions in this signalling. Sex differences in the stress resistance of other tissues is also related to sex-dependent sirtuin-3 levels and activity, which are increased with estradiol and reduced by testosterone (253).
While focussing on elements of the stress axis and response, other sex differences likely influence the responses to/impacts of acute and chronic stressors. These include differences in monoamine, gamma-aminobutyric acid (GABA), neuropeptide Y (NPY) and adipokine signalling, together with gut biology.
Central serotonin activity may be differentially stress responsive in males and females. For example, social stress reportedly decreases action potential frequency in serotoninergic nerves of males while increasing frequency in females (254). Females also appear sensitised to the behavioural effects of serotonin deficiency (255).
The sensitivity of the dopamine system to acute and repeated stressors may be greater in females compared to males (256). Different models of stress are associated with sex-dependent changes in dopamine signalling: the forced swim test increases dopaminergic activity in prefrontal cortex and hippocampus in males compared to females, while chronic mild stress decreases dopaminergic activity in the prefrontal cortex of females (257).
Differences in GABA signalling may also participate in sex-dependent stress reactivity and resilience. Differing behavioural effects of chronic unpredictable stress in male and female mice have been linked to selective changes in pre-synaptic GABA activity in females (258). Others report greater down-regulation of the GABA neuronal marker somatostatin in women with MDD, that somatostatin and GABA-synthesizing enzymes are sensitive to X-chromosome polymorphisms, and that genetic sex modulates both GABA related gene expression and anxiety behaviours (259). Stress dependent up-regulation of GABA related genes is also evidenced in male but not female rats (260).
Neuropeptide Y, linked to and regulating HPA and LC-noradrenaline axis activities, may play a role in the sex-dependent stress response. Studies in rodents support lower central NPY levels in females, including in specific stress-sensitive regions (261). However, there is little information on the sex dependence of NPY within the LC. Gonadal hormones (and fluctuations in their levels) may be important in this regard, with oestrogen increasing NPY neuronal numbers, and hippocampal NPY transcription and release (262–264). Gene deletion studies indicate NPY has a greater role in anxiety behaviours in males vs. females (265, 266). Moreover, the anxiolytic effect of exogenous NPY is evident in males and not females (267). Stress-dependent regional changes in NPY levels also differ between the sexes, though age is an important factor (261). Interestingly, NPY levels increase with age in women (268, 269) while declining in males (270–272).
Sex-dependent roles for adipokines warrant study. Chronic stress may differentially modify ghrelin levels and signalling in male and female mice (273), and there is also evidence social isolation stress preferentially inhibits central leptin signalling in female vs. male rats (274). In contrast, restraint stress selectively increases hypothalamus leptin in females (275). We recently report a fall in circulating leptin in socially stressed male but not female mice (12). Increases in leptin, potentially reflecting emerging leptin-resistance, are linked to detrimental cardio-metabolic outcomes (276, 277), and have been linked to disturbed ANS control and reduced heart rate variability (278). Leptin levels appear to be generally higher in women than in men (279, 280).
Adiponectin levels are also higher in females vs. males (279, 281, 282), though this may reflect differing body compositions (279). Adiponectin levels may decline with chronic stress (283), an effect likely to favour cardiac hypertrophy. Adiponectin was recently shown to limit cardiac sympathetic and myocardial remodelling after infarction in dogs (284). Sex differences in adiponectin responses to stress have yet to be examined in detail. Analysis of adiponectin and PTSD in woman supports a protective role for higher circulating adiponectin (285). Oestradiol may increase adiponectin levels in association with improved mitochondrial biogenesis in skeletal muscle (286), a sex dependent protective path potentially extending to the heart. Oestradiol and testosterone have also been shown to induce opposing stimulatory vs. inhibitory effects on mitochondrial biogenesis and adiponectin levels in white adipose tissue (287).
Differences in cell death will influence the response to chronic forms of stress. Previous studies report sex differences in cell death pathways, for example in response to ischemic stress (288). Although caspase-dependent pathways play a dominant role in the female brain, caspase-independent processes associated with activation of PARPs are likely to play a more important role in the male brain (289–293). This is supported by the finding that gene knockdown of PARP-1 reduces infarction volume in the male brain but increases cell death in PARP-1 knockout females (294). Similarly, neuroprotective effects of an NAD+ precursor and endogenous PARP inhibitor are evident in male mice but not in females (294). Sex differences in the neuroprotective response has been previously reported in a murine model for reperfusion injury but not in an embolic clot model (295). It is likely that sex differences exist in the links between cellular energy homeostasis and cell death processes.
Finally, the gut microbiome and gut-brain axis are key players in stress related diseases including cardiovascular, metabolic and mood disorders (296–298), and are sex-dependent and sensitive to gonadal hormones (299–304). Indeed, an oestrogen-gut microbiome axis is implicated in sex and oestrogen sensitive chronic disease (305).
While a summary only of extensive sex differences within the mammalian stress response, it is clear from this overview that the biology of stress (and its influences on health and disease) must be viewed as distinct in males and females. Focussed research is thus essential in identifying and understanding these mechanistic processes within each sex. A historic view of cardioprotection in women vs. men belies evidence of worsened CVD outcomes in women (306–308), and a relative increase in CVD risk in females over recent decades vs. reductions in males (309). Younger women have a particularly poor prognosis in ACS (310). Stress-dependent disorders, including obstructive and non-obstructive CAD and myocardial infarction (30, 31, 43, 44, 311, 312) and Takotsubo cardiomyopathy (45, 46, 313, 314), are highly sex-dependent, consistent with distinct stress biology. Acute and chronic stressors may influence CAD development and coronary events via multiple sex sensitive mechanisms. However, much remains to be unravelled regarding the relationships between sex, stress and CAD.
While sustained or repetitive stress promotes the development of chronic diseases including CAD, acute mental stress is also strongly linked to cardiovascular events (24, 25), acting as a potent trigger (24, 26, 315). This acute response may involve shifts in vascular structure and control, inflammation and thrombosis. Acute stress increases vasoconstrictor activity and sympathetic tone (316, 317). An almost instantaneous elevation in cardiac loads (heart rate, arterial pressure) with acute stressors (318) will simultaneously increase ischaemic vulnerability. Episodes of emotional upset or anger are a risk factor for SCAD (30, 31), are common in the hours immediately prior to AMI (28, 319), and appear to profoundly increase risk of acute coronary events (29). Autonomic changes during panic attacks have been linked to significant defects in myocardial perfusion (59), and sympathetic activation is implicated in SCAD (30, 31). Experimentally, acute mental stress induces features of AMI in hypercholesterolemic ApoE−/− mice (60), an effect initially linked to endothelin signalling. However, more recent work supports acute stress-dependent plaque destabilisation (61), mechanistically linked to local noradrenaline activation of endothelial adhesion molecule expression and chemokine release.
Acute stress can increase platelet reactivity, coagulation, and fibrinolytic processes to ultimately enhance thrombosis (320). Platelet activation increases with acute stress (321–323), though there is also evidence platelet glycoprotein (GP)Ib and GPIIb/IIIa expression and function are insensitive to acute stress in healthy subjects (324, 325). These effects appear to be more robust in those with CAD (325, 326). Acute stress also induces a relative hyper-activation of the coagulation cascade, with evidence of reduced fibrinolytic activation in CVD patients compared with healthy controls (327–329). In terms of sex differences, acute stress has been shown to selectively enhance FVIIa activity in men (330) vs. increased t-PA activity in women (331).
These acute outcomes involve both glucocorticoid and catecholamine signalling. Platelet activation and aggregation are increased with glucocorticoid administration in healthy subjects (332, 333), and acute catecholamine administration also stimulates thrombopoiesis and platelet activity (via α2-adrenoceptors) (322, 334). On the other hand, adrenaline and β2-AR agonists may induce a pro-coagulant state (335, 336), and sympathetic activation acts via β2-adrencoepotors to induce endothelial release of FVIII, vWF, and t-PA (322, 337). Increased β-adrenoceptor activity with acute stress may contribute to endothelial dysfunction (338, 339), however this role requires further investigation. The impacts of these glucocorticoid and catecholamine mediated effects may be enhanced by acute stress-dependent inflammation and endothelial dysfunction.
Inflammation is rapidly provoked by acute experimental (340, 341) and real-life stressors, including bereavement, natural disasters, and sporting events (342–344), and may promote coronary ischaemia (345). Increased circulating IL-6 parallels fibrin formation and markers of coagulation, for example (346). The inflammatory response to acute stress may also be exaggerated in those with CAD (347). Acute stress appears to induce endothelial dysfunction, though findings from human studies are mixed (348). Some report increased perfusion and flow-mediated dilation in response to acute stress in healthy males and females (349), while others report vasoconstriction and impaired flow-mediated dilation (350–352). The latter inhibitory effect may be eliminated by inhibiting cortisol release (350), consistent with evidence cortisol reduces vascular eNOS signalling (353, 354). This likely involves a suppressive glucocorticoid response element in the eNOS promoter region (355). Cortisol may also increase release of the potent vasoconstrictor endothelin to further reduce vascular conductance (356, 357). Human studies confirm increased endothelin levels in response to acute stress (358, 359), and a reduction in associated endothelial dysfunction with endothelin antagonism (360).
The importance of sex in the cardiovascular impacts of acute stress remain relatively understudied. Females are at a substantially heightened risk of acute mental stress related AMI (Table 1). This stress induced ischaemia (361), and associated transient endothelial dysfunction (362), both predict major cardiovascular events in those with CAD. Vaccarino et al. determined a 4-fold higher risk of mental stress induced ischaemia in women ≤50 years of age compared with men of similar age or older (43). More recent work identifies a 2-fold greater risk of mental stress induced myocardial ischaemia in young women vs. men, with a relationship between peripheral vascular function and ischaemia in women only (44). This is congruent with evidence endothelial function and vascular reactivity to mental stress predict major adverse cardiac events in women but not men (363). These observations collectively suggest greater propensity to microvascular dysfunction and ischaemia in women. Women are also at much greater risk of SCAD and resultant perfusion defects, a response linked to acute emotional and (to a lesser extent) physical stress (30, 31).
There is evidence acute stress exerts distinct effects on mediators of coagulation and fibrinolysis in males and females (330, 331). Greater sympathetic activity in the hearts of women (364), particularly in the apical region of the left ventricle, may be relevant to relative myocardial sensitivity to acute stress, and is consistent with the sex dependence of Takotsubo cardiomyopathy and the apical changes in this disorder. The acute coronary response to mental stress differs between sexes: Martin et al. (365) found that reactive hyperaemia with mental stress is lower in women vs. men, in association with greater endothelial dysfunction and reduced reactivity. Mehta et al. (366) found that women with coronary vascular dysfunction also exhibit greater peripheral vasoconstriction in response to acute mental stress.
Repetitive or chronic stress promotes disease via a broad disruption of homeostasis and adaptability (367, 368), incurring a significant biological cost or allostatic load (369). Shared biological networks may transduce chronic stress to multiple co-morbid disease states (6). Coronary artery disease is promoted by integrated influences of autonomic, neuroendocrine and immuno-inflammatory dysregulation on metabolism and lipid handling, thrombosis/haemostasis, and heart and vessel function and structure (Figure 2). Associated behavioural/affective impacts of chronic stress interact with these biological determinants to significantly increase risk of CAD, together with commonly co-morbid conditions including MDD and diabetes (6) (Figure 2). However, the stress response and its behavioural and cardiovascular influences are significantly sex-dependent (370). Indeed, fundamental adaptive mechanisms that provide resilience to stress differ between the sexes (371, 372).
Figure 2. The stress response and its effects on body systems and CVD risk. Chronic stress activation of the HPA axis and SNS activity result in elevations in glvuocorticoids (GC) and catecholamines, and reduced PNS activity. Resultant behavoural/affective outcomes, immuo-inflammatory dysregulation, metabolic and cardiovascular effects collectively favour development of CVD.
Chronic stress strongly predicts maladaptive coping or avoidance responses to a wide variety of provocations, including decreased social interactions, physical inactivity, altered sleep and dietary patterns (373, 374). These outcomes favour emergence of cardio-metabolic disorders. Behavioural drives of disease involve and are compounded by the influences of stress on neuroendocrine control, metabolic homeostasis and lipid handling, inflammation, and gut biology. Importantly, sex differences in coping strategies guide distinct behavioural responses to stress. The literature highlights a greater impact on eating and sleep patterns, together with immuno-inflammatory function in females vs. males.
The basis of sex-dependent behavioural responses to stress is complex. External psycho-social, cultural and life history factors all contribute to differences in stress-related behaviours and outcomes. Gonadal hormones play an important role (375), and sex differences in stress-related mood disorders emerge after puberty (376). Significant variability in gonadal hormone levels in women has also been implicated in differing incidence of stress-related disease (377). Androgens negatively regulate the HPA axis and reduce stress-dependent behavioural changes (378). In contrast, estrogen receptor (ER)α activity promotes HPA axis activity, increasing stress-dependent ACTH and corticosterone in male and female rodents (379, 380) and reducing negative feedback control of the axis (381). This is associated with anxiogenic influences of ERα activity (379, 382), although opposing behavioural effects of ERα activity are reported (383, 384), linked to reproductive history. Interestingly, anxiety-like behaviours are increased by ERα deletion in males (385) but not females (386). However, absence of these regulatory receptors over the life of knockout models complicates interpretation. Nonetheless, associations between ERα gene variants and anxiety and depressive disorders in humans (387–389) support the importance of sex hormones in the response to chronic stress.
Unfortunately, outcomes from animal studies, particularly in rodents, are equivocal and in some cases opposed to observations in humans. Studies in rats report reduced anxiety-like (390–392) and depression-like (393, 394) behaviours in females vs. males, suggesting greater female resilience. Others report no differences or increased anxiety- and depressive-like behaviours in female vs. male rats (395, 396). Similarly variable outcomes are evident in murine studies (397, 398), however there is evidence of greater biological or behavioural disruption in stressed female vs. male mice (399, 400), consistent with our own recent observations (12).
Inflammatory challenge induces “sickness behaviours” that closely mimic elements of stress dependent mood disorders, often cited evidence for key involvement of low-grade inflammation in the behavioural/affective impacts of chronic stress (401, 402). Interactions between inflammation and mood disorders (403, 404) have been highlighted in large population studies (405) and meta-analysis (406). A bi-directional interaction exists between inflammation and mood disorders, a positive feedback characteristic of stress-related disease (407, 408). Circulating cytokines communicate with the brain through both neural and humoral routes, influencing central control of mood and behaviour (409, 410). The biological mechanisms of cytokine-induced behavioural changes have been well studied in animal models, for example employing synthetic double-stranded RNA (poly I:C) or lipopolysaccharide (LPS) challenge to induce anxiety- (411) and depressive-like behaviour (412), respectively. Although chronic exposure may ultimately induce immune tolerance, prolonged changes in the brain and periphery emerge. For example, chronic LPS treatment induces sustained microglial activation (413), and persistent poly I:C exposure induces tumour necrosis factor (TNF)-α dependent neuroinflammation and alters expression of memory associated genes in frontal cortex (FC) and hippocampus (414).
The behavioural influences of inflammation are sex-dependent (177). Experimental evidence supports an enhanced immuno-inflammatory reactivity in women (415–417), although comparable outcomes in men and women have also been reported (418, 419). Studies in animal models provide support for greater neuroinflammatory reactivity in females vs. males (420). Distinct outcomes from in vitro vs. in vivo studies are relevant, confirming critical involvement of systemic (in vivo) mechanisms in the expression of sex differences. For example, higher female immunoreactivity is less evident in ex vivo models (421), with opposing evidence of greater reactivity in isolated immune cells from men vs. women (422, 423). This highlights the importance of assessing the complex biological influences of stress in vivo within an integrated systems biology framework (6).
The temporal pattern of behavioural response is also relevant in terms of sex differences. Engler et al. (181) assessed behavioural/affective effects of low level LPS challenge and reported similar mood, anxiety and fatigue levels in men and women over the initial 6 h of immune challenge, consistent with other reports (416, 424–426). However, sickness behaviours 24 h following LPS may be greater in women vs. men (427), suggesting a progressive emergence of sex dependent behavioural outcomes after the initial inflammatory response and peak cytokine changes. That said, there are also reports of greater depressive symptoms during the initial inflammatory response in women (93, 428).
Dietary patterns are changed with stress, in a manner generally promoting the development of CAD (429). This “emotional eating behaviour” (430) includes spontaneous binging and hyperphagia together with increased consumption of fatty foods, sweet foods and snacks (vs. decreased consumption of fruits and vegetables). It is well established that elevations in CRH and adrenaline with acute stress suppress appetite to induce a negative energy balance. Conversely, extended release of cortisol or corticosterone (with chronic stress) more generally increases appetite and motivation to eat (431) and modifies adiposity and fat distribution (432)—although not all studies agree (433). As with addictive drug behaviours, hyper-palatable food consumption with chronic stress is linked to increased mesolimbic dopaminergic system activity (434).
Unfortunately, few studies directly examine the importance of biological sex in determining stress-dependent eating patterns. Meta-analysis of links between stress and metabolic syndrome evidences a differing stress-metabolism relationship in females vs. males, suggestive of greater susceptibility of females to harmful stress-dependent behaviours (such as insomnia, physical inactivity and disordered eating) (435). Additionally, an analysis of relationships between BMI and post-traumatic stress disorder in Iraq and Afghanistan veterans identified sex effects, including preferential links between persistent obesity and PTSD in men vs. depression in women (implicating distinct sex-specific mediators of stress related weight gain). Women may experience more stress-dependent eating than men (436), and pre-clinical studies support sex-dependent patterns of stress feeding. For example, Anversa et al. (437)., investigated stress-induced binge-eating in food restricted and unrestricted mice, with results showing that males only displayed binge-like eating behaviour under food restricted conditions, whereas this behaviour was evident in ad libitum-fed females. This suggest potentially greater hedonic or reward behaviour in females, whereas male eating may be strongly dependent upon or reflects a homeostatic response to metabolic challenge. Nonetheless, opposing effects of stress are also reported, with chronic stress reducing food consumption in both mice (438) and rats (439), though dietary changes again appear more prominent in females. Temporal differences may be important: Pare et al. (439) found that food consumption was consistently reduced with increasing stress chronicity in females, whereas changes were transient (recovering to baseline) in male animals over the same period. Nonetheless, with only a 5-day duration of “chronic” stress in this study, outcomes may involve significant influences of acute stress.
Reductions in physical activity with stress favour development of metabolic and cardiovascular disorders. Increased physical activity, in turn, is cardioprotective and also anti-depressant. Indeed, physical fitness or aerobic capacity may be a dominant determinant of chronic disease risk (conversely health and longevity) (440). Levels of physical activity are reduced in chronic stress or stress-related mood disorders, explaining in part the link between stress/MDD and CVD (441). Similarly, chronic stress reduces running activity in mice (442). Anxiety sensitivity, a learned cognitive trait predisposing individuals to fearful misinterpretations of internal processes and sensations (443–445), is also associated with declining physical activity and fitness (446–448), together with cardiovascular risks including hypertension (449, 450), high cholesterol (451), atherosclerosis and arterial stiffening (452). A study by DeWolfe (453)., identified a significant, indirect effect of sex on physical activity in anxiety sensitivity (454), with female students reporting less activity and greater anxiety sensitivity than males. While Moshier et al. (373) found no sex differences in the AS-exercise relationship, it was noted that women have significantly less engagement with exercise than men (thus may be at greater risk of physical inactivity).
Dysregulated sleep, including more frequent awakening and inability to fall into deep sleep, may be an important link between chronic stress and CVD (455). Under normal conditions, the HPA axis and SNS are suppressed in the initial stages of sleep, with activity increasing close to maximum circadian rhythm immediately after waking. The HPA axis and SNS influence the overall amount of rapid eye movement sleep (456), and chronic stress is associated with nyctohemeral activation of these systems, increasing the release of CRH, ACTH, cortisol/corticosterone, noradrenaline, and adrenaline (457), disrupting circadian clock genes in peripheral organs, and inducing nocturia, chronic insomnia and increased fatigue (458).
Excitatory orexins may play an important role in dysregulated sleep. Generated in the lateral hypothalamic regions, orexin neurons project to all brain regions. Of particular relevance, orexins influence regions associated with arousal, such as the locus coeruleus, to regulate responses to stressful stimuli (459). Effects of central orexin administration are well documented in animal models, supporting involvement in stress-mediated behaviour (460) and the dysregulation of HPA axis (461, 462) and SNS function (463, 464). Although it is clear acute stress up-regulates orexins, effects of repeated or chronic stress are less well defined: predictable (homotypic) chronic stressors generate conflicting outcomes (465–467), while unpredictable (heterotypic) chronic stressors up-regulate orexins (468). Lower orexin function may be indicative of stress resilience (469) or habituation (470). Examining sex-effects in rats, Grafe et al. found that females exhibit significantly less habituation to repeated restraint stress than do males, in association with higher levels of orexin neuronal activity (471).
Effects of chronic stress on neuroendocrine/autonomic function and inflammation interact to disturb whole body and organ specific metabolism. With the perception of stressful conditions, HPA axis and SNS activities and mediators increase energy supply via catabolic influences, liberating energy substrates such as glucose, amino acids, glycerol and fatty acids. However, prolonged activity with chronic stress can promote obesity, insulin-resistance, diabetes and metabolic syndrome. Psychosocial stress is a significant risk factor for excess weight gain (472) and obesity (473), associated with increasing adiposity (474), redistribution of fat tissue and accumulation of abdominal fat (475, 476). Exposure of healthy (non-obese) young men to long term stress increases abdominal obesity and dyslipidaemia (477). Chronic psychological stress in patients with stable ischaemic heart disease is similarly associated with higher body fat and detrimental lipid changes (478). Community-based studies also demonstrate a tendency for obese individuals to experience greater levels of stress-related disorders (479). Moreover, chronic stress may selectively promote particularly problematic visceral fat accumulation rather than subcutaneous fat, which is associated with low CAD risk and mortality (480). Mediators of stress-dependent metabolic dysfunction are noted below, together with potential sex effects.
The link between glucocorticoids and metabolic disruption has been well established, exemplified in the increased weight gain, and visceral adiposity in Cushing's syndrome (481) and exogenous corticosteroid exposure (482). The link between glucocorticoid dysregulation and metabolic abnormalities is highlighted in mood disorders, with hypercortisolemic (and not normo-cortisolemic) depression associated with increased visceral fat (483). Increased glucocorticoid levels induce visceral fat accumulation through increases in dietary fat intake and hydrolysis of circulating triglycerides by lipoprotein lipase (484). Glucocorticoids also stimulate hepatic gluconeogenesis and inhibit glucose handling skeletal muscle and adipose tissue, promoting insulin-resistance (485, 486). There is evidence elevations in glucocorticoids contribute to insulin-resistance via increases in pancreatic islet proliferation and volume, insulin secretion capacity, and islet chaperone expression (487). Interestingly, despite anabolic effects on adipose and overall mass in humans, catabolic effects of glucocorticoids appear maintained in other tissues, with reductions in bone mineral density and lean body mass (488). These catabolic effects are more prominent in rodents vs. humans, with reductions of body weight in response to corticosterone intake (489) or repeated dexamethasone injections (490). However, adipose mass may nonetheless increase relative to overall body mass (489, 491).
The SNS is important in the integrated regulation of energy expenditure and intake to maintain long-term energy balance. Clinical and pre-clinical studies provide broad support for the involvement of sympathetic over-activity in development of obesity and metabolic syndrome (492), and stress-dependent metabolic dysregulation. Baseline sympathoadrenal activity has been shown to predict increases in body weight and development of insulin-resistance in an 18-year longitudinal study (493). Insulin-resistance and dyslipidaemia in a rat model of metabolic syndrome are worsened by chronic restraint stress, in association with increased noradrenaline levels and countered by β-adrenoceptor blockade (494). There is also evidence the greater effects of stress on LDL cholesterol and triglycerides in hypertensives vs. normotensives involve increased noradrenaline activity (495). Sympathetically mediated vasoconstriction may also functionally antagonize insulin-dependent glycaemic control via reductions in tissue blood flow, contributing to the development of insulin-resistance (496). There is evidence for an important interaction between SNS and NPY activities in development of obesity and metabolic disease. For example, Kuo and colleagues (497) report that stress dependent NPY release from sympathetic nerves promotes abdominal obesity and development of metabolic syndrome (including impaired glucose tolerance, hyperlipidaemia, hypertension, and increased concentrations of insulin, leptin and resistin). Outcomes in NPY over-expressing mice are also consistent with a role for altered SNS activity in NPY-dependent obesity and metabolic syndrome (498).
Immuno-inflammatory activation in brain and periphery are a feature of chronic stress (499), with indirect effects on metabolism and regulatory systems throughout the body (500). Chronic low-grade inflammation induces insulin-resistance (together with endothelial dysfunction) (501) and may directly facilitate diet-induced obesity (502). A bi-directional positive feedback is evidenced in studies of dietary obesity in mice (503), with increased hypothalamic and hippocampal inflammation participating in a vicious feed-forward cycle of CNS dysfunction (504). This may involve increased blood brain barrier permeability, reactive glial cytokine production and circulating pro-inflammatory adipokines (505). In the periphery, members of the interleukin (IL)-1 family influence insulin-resistance and metabolic inflammation in obesity-associated disorders (506–508). Modulators of IL-1, NLRP6 and NLRP3 inflammasomes negatively regulate non-alcoholic fatty liver disease and steatohepatitis progression and contribute to aspects of metabolic syndrome (509). The inflammatory transcription factor NFκB has emerged as an important metabolic regulator, with enhanced hepatic activity observed in high fat-fed mice (510, 511). As detailed further below, pro-inflammatory cytokines contribute significantly to vascular dysfunction and atherosclerotic disease (512).
Stress-dependent dyslipidemia similarly favours development of CAD. Chronic stress promotes an unfavourable pro-atherogenic lipid profile in humans (513) and animal models (514–517). This may include elevations in circulating cholesterol, low-density lipoproteins (LDLs), very-low density lipoproteins (VLDL) and triglycerides, and reductions in high-density lipoproteins (HDLs). Chronic mild stress in rats increases total, LDL and VLDL cholesterol together with triglyceride levels (and atherogenic index) without influencing HDL cholesterol (518). Increased sympathetic activity with stress, acting via β1, β2 or β3-adrenergic receptors in white adipose tissue, promotes lipolysis (519). Increased VLDL and decreased HDL levels, and facilitation of LDL entry into blood vessel walls, initiates and promotes the atherosclerotic process (520).
Further work is needed to reveal the effects and importance of sex in stress-dependent metabolic dysregulation and disease. Nonetheless, there is evidence of sex specific changes in metabolism and lipid profiles. For example, animal studies suggest stress-induced changes in metabolic rate may be greater in females than males (521), which may reflect oestrogenic attenuation of sympathoadrenal and HPA responsiveness (522). The anabolic effects of glucocorticoids are more prominent in male compared with female mice, potentially involving oestrogenic protection and differing corticosteroid receptor expression/sensitivity in females (523). This may contribute to a greater relative risk of stress-dependent obesity and metabolic disturbance in females vs. males. It has been reported that chronic restraint stress reduces abdominal fat deposition in male rats while increasing fat in females (524). On the other hand, chronic social stress may induce weight loss specifically in female and not male mice (12).
Studies confirm sex differences in circulating lipid profiles under both stress and non-stressed conditions. Pre-menopausal females exhibit higher circulating HDL levels while males have higher triglyceride levels, with lipoprotein profiles converging after menopause (525). Reduced risk of CVD in premenopausal women has been attributed this higher concentration of HDL cholesterol (526). However, there is also evidence recurrent episodes of stress may eliminate this benefit in women (527). This agrees with evidence risk of stress-related disorders is differentially linked to lower HDL-cholesterol levels in women vs. lower LDL-cholesterol levels in men (528). Similarly, HDL-cholesterol is a more significant CVD risk factor in women, while LDL-C is more significant in men (529). Studies in rats indicate acute and chronic stress increase plasma cholesterol levels to a greater extent in ovariectomized females (compared with intact females or males), and that metabolic risk is influenced more by acute stress in males vs. chronic stress in females (530). Prenatal stress also exerts sex-specific effects on metabolism, although findings are mixed. This includes evidence of a female specific elevation in cholesterol levels, and a male specific fall in body weight in prenatally stressed mice (531), whereas others report a male specific increase in cholesterol and triglycerides (532).
Chronic stress induces endothelial dysfunction and detrimental vascular remodelling (533–535), effects that increase vascular resistance and favour local O2 supply:demand imbalances, hypertension, development of atherosclerosis, and acute coronary events. Stress-dependent endothelial dysfunction, linked to adverse cardiovascular outcomes in patients with CAD (362), arises with diverse stressors in both humans and animal models. For example, flow-mediated, endothelium-dependent vasodilation is impaired with chronic sleep deprivation and exam stress in healthy male college students (536), and with chronic carer stress in the elderly (537). The coronary microvascular impact of mental stress appears solely endothelium-dependent and is mirrored in peripheral vascular responses (538). Experimental studies support reduced nitric oxide (NO) bioavailability and vasodilatation (potentiating α-adrenoceptor-mediated vasoconstriction) in association with increased oxidative stress in animal models of chronic stress (518, 539–541). However, there is also evidence of reduced endothelium-dependent hyperpolarization-like relaxation in microvessels from rats subjected to chronic mild stress (542). Reduced endothelial-dependent dilation in arterial tissue from chronically stressed rats has also been linked to shifts in central (cortical) glutamate signalling (543), revealing a potential neurobiological mechanism of stress-dependent endothelial dysfunction. Intima and media hypertrophy arise in association with increased α-adrenoceptor vasoreactivity in rats exposed to chronic unpredictable stress (518). Arterial blood pressure also increases in models of chronic stress, in association with endothelial dysfunction, oxidative stress and increased angiotensin II levels (541). Causal involvement of RAAS activity is supported by beneficial effects of ramipril and losartan. Chronic stress may excessively activate the RAAS (544) and increase arterial sensitivity to angiotensin (545). Chronic elevations in angiotensin II also induce inflammation, endothelial dysfunction and senescence (546, 547), while angiotensin receptor antagonism protects against the effects of chronic stress (548). Sympathetic activity may indirectly promote endothelial dysfunction via its stimulatory effects on RAAS activity (549), together with inflammation and oxidative stress.
Glucocorticoids play a causal role in endothelial dysfunction, whereas involvement of sympatho-adrenergic activity is less clear and potentially indirect (533). Nonetheless, vasoconstrictor activity of catecholamines is consistently augmented with chronic stress, which likely contributes to hypertension and coronary abnormalities. Increases in cortisol inhibit endothelium-dependent vasodilation (550), reducing forearm blood flow responses to acetylcholine in healthy men for example (551). Cortisol directly reduces human endothelial eNOS expression (552), consistent with presence of a glucocorticoid response element in the eNOS promoter. Confirming the importance of glucocorticoids, effects of mental stress on flow-mediated vasodilation are negated by inhibition of cortisol production (350).
Involvement of the low-grade inflammation characteristic of chronic stress is indirectly supported by observations in humans and pre-clinical models. Cytokines reduce endothelial-dependent dilation in human veins in vivo (553), and acute inflammation with vaccine challenge in healthy volunteers is associated with pronounced endothelial dysfunction (554). As for cortisol, cytokines also down-regulate endothelial nitric oxide synthase (eNOS) in human coronary endothelium (555), and arterial endothelium of other species (556).
Chronic psycho-social stress promotes development of essential hypertension (496, 557–559), a major risk for CAD and infarction (560). Recent secondary analysis of the Isfahan Cohort Study (561), for example, indicates high stress levels increase the likelihood of hypertension by ∼40%, with significant links between hypertension and job conflict, job security, personal conflict, sexual and daily life in both sexes (independent of socioeconomic/lifestyle covariates), together with financial problems in males. Stress-dependent sympathetic activity promotes vessel remodelling and vasoconstriction (562), which with endothelial dysfunction and altered neuroendocrine and autonomic control, collectively favour development of hypertension (496, 585). Chronic stress impairs baroreceptor sensitivity and baroreflex function to increasing arterial pressure in humans (563, 564), while increased glucocorticoid levels are also associated with and increase the risk of hypertension (565, 566). Hypertension is additionally supported by associated neuroinflammation (567) and increases in renal renin and pituitary AVP secretion (568). Inhibition of neuroinflammation counters hypertension in different experimental models (569–572), and the hypertensive effects of angiotensin II/RAAS activity have also been linked to microglial activation and inflammatory cytokines within the PVN (573).
The coronary and hypertensive influences of chronic stress are significantly dependent upon biological sex. Indeed, it is clear that coronary physiology and syndromes must be understood and managed in a targeted sex-specific manner (574–576). Importantly, women have greater microvascular density and baseline coronary perfusion (intermediate between healthy and CAD subjects) than men (577, 578), with less macro or obstructive CAD (579, 580). Evidence suggests women are more prone to coronary microvascular dysfunction than men (580, 581), including stress-dependent infarction (43, 44). Coronary microvascular dysfunction is also linked to sex-dependent Takotsubo cardiomyopathy (45) and heart failure with preserved ejection fraction (HFpEF) (582), both significantly more prevalent in women. Interestingly, recent work shows that a reduced coronary flow reserve in women is predicted by a blunted heart rate reserve, indicative of involvement of higher sympathetic activity in differing coronary outcomes (583). We recently reported that chronic social stress differentially increases coronary resistance in the hearts of female mice, while reducing resistance in males (12). Consistent with increased propensity to stress-dependent ischaemia in women (43, 44), the basis of this coronary dimorphism is unclear. However, female mice did exhibit greater inflammation than males, which impairs endothelial-dependent control in different vascular beds (553, 554).
Age and neuroendocrine changes are important interacting factors regarding sex effects on vascular and blood pressure control. For example, baroreflex sensitivity is higher in pre-menopausal women than either age-matched men or post-menopausal women (584). Oestrogen replacement therapy also increases baroreceptor sensitivity in postmenopausal women, attenuating onset and progression of cardiovascular disease and accelerating recovery from cardiovascular events (585, 586). Age-dependent elevations in sympathetic activity are more pronounced in women, while vagal tone and baroreflex sensitivity decline with age (587–589). Endothelial function falls progressively with age in men yet is relatively preserved in pre-menopausal women, before subsequently declining (590). This pattern is congruent with vasoprotection via gonadal hormones: endothelial function is reduced by prolonged gonadotropin-releasing hormone agonism (suppressing oestrogen generation) or acute gonadotropin-releasing hormone antagonism, and improved by hormone replacement in women (591); and endothelial function in young men is similarly impaired by aromatase inhibition to limit oestrogen generation (592). Stress-dependent increases in arterial pressure in ovariectomized rats are also reduced with hormone replacement, in association with increased vascular eNOS expression (593). Such observations support a protective role for oestrogen-sensitive vascular eNOS in limiting cardiovascular stress reactivity. Age dependent arterial stiffening is also more prominent in women, contributing to reduced baroreceptor firing and baroreflex sensitivity in older women vs. men (594). These age dependent reductions in baroreflex sensitivity may involve changes in peripheral afferent and/or efferent pathway control of the baroreflex system and sinus node function (594), with changes more dramatic in women compared to men (595).
Influences of testosterone on endothelial dysfunction are less clear: there is some evidence endothelial dysfunction is linked to declining testosterone levels (596, 597), although the opposite has also been reported (598). Meta-analysis indicates no significant associations between testosterone treatment and endothelial function, however studies are limited and outcomes mixed (599). There is also little research on the relationship between testosterone and endothelial function in women, however there is evidence of an association between low testosterone and endothelial dysfunction in post-menopausal oophorectomized women (600). Worboys et al. (601), also report that 6 weeks of testosterone supplementation in post-menopausal women receiving estradiol therapy improves both endothelial-dependent and -independent vasodilation. On the other hand, pre-clinical studies suggest potentially detrimental effects of testosterone on endothelial function (602), including inhibition of the vascular benefits of oestrogen (603). A higher blood pressure in male compared with female spontaneously hypertensive rats has been linked to testosterone sensitivity (and oestrogen insensitivity) of vasodilatory prostanoid generation, associated with sex differences in renal oxidative stress, heme oxygenase- 1 and arachidonic acid metabolism (604, 605). Consistent with a male propensity to stress-induced hypertension, chronic stress selectively increases arterial pressure in borderline hypertensive male but not female rats (606).
Chronic stress is strongly pro-atherogenic, reflecting integrated impacts of stress-dependent dyslipidaemia, vascular dysfunction/remodelling, thrombosis, hypertension, oxidative stress and low-grade inflammation, together with more direct atherogenic influences of SNS and HPA axis dysregulation. Chronic stress has been estimated to be a stronger independent risk factor for atherosclerosis (and intimal and media thickness) in an animal model of disease than total or LDL cholesterol (13). The broadly pro-inflammatory milieu induced by acute and chronic stress and SNS overactivity is pro-atherogenic (607), and the makeup, inflammatory profile and stability of atherosclerotic plaques is stress-sensitive. Chronic stress shifts plaques towards an unstable phenotype, with increased leukocyte and matrix metalloproteinase (608, 609) vs. reduced smooth muscle and collagen contents (634), and attrition of the fibrous cap (608). Plaque destabilisation in response to acute stress in ApoE−/− mice (335) involves noradrenaline stimulation of endothelial adhesion molecule expression and chemokine release. Mast cells also participate in plaque destabilisation together with coronary vasospasm (610), are activated by chronic stress and corticotropin-releasing hormone, and are increased in CAD patients where they provoke acute coronary events (611–613). They have been shown to promote plaque destabilisation in animals subjected to acute stress (614), while chronic and early life stresses also increase mast cell numbers (615–617). However, involvement in chronic stress related atherogenesis awaits focussed study.
Sympathetic activity is an important mediator, with strong atherogenic potency of catecholamines (618, 619) readily demonstrable in animal studies (620, 621), even in the absence of altered blood pressure and cholesterol levels (619). Increased blood pressure reactivity to mental stress, governed by SNS activity, is also positively associated with development of atherosclerosis (622). Sympathetic activity stimulates splenic hematopoietic progenitor cell proliferation and myeloid cell development (623), and leukocytes express a more pro-inflammatory transcriptional profile (624), with increased expression of C-reactive protein, IL-1, IL-6 and TNF (625). Ablation of SNS activity with 6-hydroxydopamine reportedly counters the up-regulation of endothelial adhesion molecules in models of AMI (626).
Glucocorticoids are also pro-atherogenic (627). Pre-clinical studies confirm pro-atherosclerotic effects of cortisol/corticosterone in different models (628, 629). Priming by prior exposure to either glucocorticoids or noradrenaline also sensitises monocytes/macrophages to subsequent inflammatory challenge (630), effectively amplifying the effects of repeated stress. Stress and SNS-dependent RAAS activation additionally promotes atherosclerosis (656, 657). Angiotensin II activation of type 1 receptors increases leukocyte adhesion molecule and inflammatory mediator expression in endothelial cells (631), which possess glucocorticoid receptors that render them directly sensitive to HPA axis activity (632). Increased arteriolar leukocyte adhesion in response to angiotensin-II has been linked to TNF-α dependent signalling (633). Experimental studies also support involvement of pro-inflammatory toll-like receptor 4 and nuclear factor-kappaB signalling in the atherogenic effects of stress in ApoE−/− mice (634), and a role for oxidative stress (and hyperlipidaemia) in stress related atherosclerosis (662). Endothelial dysfunction and abnormal NO signalling, reflecting in part the influences of inflammation and oxidative stress, also play an important role (663). Altered reverse cholesterol transport (RCT) could also participate, though this has received relatively little research attention. There is evidence chronic stress augments the inhibitory influences of high-fat feeding on RCT (635), although these investigators found no influence of stress alone on markers of RCT.
There is evidence of significant influences of sex on stress related atherogenesis (Table 1), however focussed research is needed. For example, Chumaeva et al. report that, in the context of the syndrome of vital exhaustion (characterized by fatigue and irritability), only men with reduced arterial elasticity show an increased risk of atherosclerosis in early life, with no such risk in women with either high or low arterial compliance (636). While inconclusive, the authors suggest this may reflect better coping with stressful risk factors in women than men. Shivpuri et al. (637), also found evidence of sex-dependent influences of stress on inflammation in the Multi-ethnic Study of Atherosclerosis. Experimental studies also identify sex-dependent influences of early life stress on inflammation and mast cell activity: early weaning stress increases mast cell number and activity in pigs to a significantly greater degree in females vs. males (616), consistent with evidence repeated maternal separation stress selectively increases hippocampal mast cell numbers in females and not males (617). Such observations implicate mast cells as an understudied mediator of the sex-specific effects of early life adversity.
Thrombosis links chronic stress, mood disorders and CAD (638). Increased platelet–leukocyte aggregates are evident in CAD patients, and platelet activity is increased by high level stress and in people prone to stress-related depression (639). Mental stress induces prolonged elevations in pro-inflammatory platelet activity (321), and mental stress induced ischaemia is associated with increased platelet aggregation (640). Animal models similarly evidence increased thrombosis in response to chronic stress (641, 642). Increases in thrombopoiesis and platelet activity with stress involve both glucocorticoid and sympathetic influences (and associated inflammatory/ROS responses), together with potential roles for serotonin (643) and brain-derived neurotrophic factor (BDNF) signalling (204, 644). The sympathoadrenal activation with chronic forms of stress primarily enhances coagulation, while fibrinolysis may be additionally influenced by acute stressors (322, 645, 646). This may reflect a pro-survival response aimed at limiting blood loss in threatening fight-or-flight settings. However, such effects can in the longer term promote CAD and risk of acute coronary events (647, 648).
Platelet levels, activity and aggregation are all increased with glucocorticoid administration (332, 333), and under conditions of chronically elevated cortisol (649). Increased thrombin-induced platelet aggregation with high work stress is linked to elevations in cortisol (650). There is also experimental evidence ACTH promotes arterial thrombosis, with ACTH (yet not cortisol) acutely amplifying agonist-induced platelet aggregation (651). Associated SNS activity is also involved in stress-axis dependent thrombosis, with animal studies confirming key involvement in the pro-coagulant effects of chronic stress (652). Exposure to either stress or catecholamine increases thrombopoiesis, platelet reactivity and GPIb, GPIIb-IIIa complex and P-selectin expression (322, 334, 646, 653) in an α2-adrenoceptor dependent manner. In vitro studies confirm that adrenaline increases platelet activation/aggregation and clot formation via α2a-adrenoceptor activity (654).
Serotonergic signalling may also participate in stress-dependent platelet activity and thrombosis. Serotonin mediated platelet activation is enhanced in people with stress-related MDD (655), and an early review of the literature (688) highlighted varied evidence of hyperactive platelet serotonin receptor signalling in depressive patients which may increase risk of thromboembolic events. Subsequent studies confirm increased platelet serotonin receptor signalling and reactivity in response to acute or chronic stress (640, 656). Recent work also shows that depression is associated with higher serotonin receptor density in CAD patients, with increased platelet serotonin reactivity in depressed patients with minor adverse cardiac events (657).
Signalling by the neurotrophin BDNF, and specifically a BDNFVal66met polymorphism, may additionally link platelet function to stress and MDD (204). Chronic stress disrupts BDNF signalling, which is considered an important determinant of stress resilience and mental health outcomes (658). Recent work shows that BDNFVal66Met mice have increased propensity to thrombosis, with sub-chronic stress (7 day restraint stress) inducing a pro-thrombotic phenotype (659). This includes increased leukocyte and platelet numbers, heightened platelet responses, and increased platelet/leukocyte aggregates, P-selectin and GPIIbIIIa expression, and arterial tissue factor activity. More recent experimental evidence supports involvement of increased platelet α2-adrenoceptor signalling: the pro-thrombotic phenotype in BDNFMet/Met mice is linked to platelet over-expression of α2A-adrenoceptors, involves noradrenaline and is rescued by select α2A-adrenoceptor antagonism; and platelets from homozygous BDNFMet/Met CAD patients are similarly over-reactive and over-express α2A-adrenoceptors (205).
Additional pro-thrombotic influences of chronic stress include increased reactive oxygen species (ROS) and nicotinamide adenine dinucleotide phosphate (NADPH) oxidase dependent platelet activity (660), and dipeptidyl peptidase-4 expression (694), which may promote both oxidative stress and von Willebrand factor cleavage by ADAMTS13). However, stress-dependent elevations in dipeptidyl peptidase-4 may accelerate atherosclerosis via a multiplicity of mechanisms (661).
The importance of sex on the association between stress related disease and platelet function has been somewhat neglected. A preliminary analysis of sex effects in the platelet-MDD association by Izzi and colleagues (662) evidences sex-dependent links between depressive symptoms and both platelet volume and variability in size. In a cohort of patients with stable IHD, Samad et al. found evidence of increased platelet sensitivities to both serotonin and adrenaline in women compared with men, together with greater mental stress induced ischaemia and collagen-stimulated platelet aggregation (640) (Table 1).
Just as vascular control and structure are altered, myocardial function and makeup are significantly modified with chronic stress. Autonomic dysfunction and a shift towards sympathetic vs. parasympathetic activity underlie a hallmark of chronic stress and associated depression—reduced heart rate variability (HRV). These autonomic changes are responsible for impaired baroreflex control of cardiac function (and blood pressure) (563). Animal studies link susceptibility to chronic stress to increased sympathetic tone and impaired baroreflex control (663). Angiotensin II signalling may play some role in these autonomic and baroreflex responses to chronic stress (664). Increased sympathetic tone and impaired baroreflex control, in turn, favours coronary and cardiac dysfunction, hypertension and cardiac hypertrophy. This is consistent with evidence a low HRV predicts the onset of (665) [and falls further with (666)] hypertension, and a relationship between low HRV and left ventricular hypertrophy (667, 668), although the latter may vary significantly with race (669). A reduced HRV significantly increases the risk of a cardiac event: analysis of Framingham Heart Study data reveals a low HRV increases risk of an acute cardiac event over a mean follow-up of 3.5 years by ∼1.5 fold (670). Reductions in HRV following AMI or CABG are also linked to worsened outcomes (671, 672). However, despite generally reflecting the balance of sympathetic and parasympathetic influences in cardiovascular control, it should be noted that the mechanistic basis of the relationship between HRV and coronary or myocardial disease remains to be firmly established, and may well be indirect—changes in HRV may serve as a secondary biomarker of chronic stress, which influences CAD risk and outcomes via diverse mechanisms parallel to SNS activity.
Together with altered cardiovascular control, prolonged sympathetic activity and associated inflammation, and shifts in adipokine and other endocrine signalling, promote cardiac hypertrophy and failure. Hypertrophy renders the myocardium susceptible to O2 supply:demand imbalance and is a risk factor for CAD development and cardiovascular morbidity and mortality. Chronic stress also exacerbates pressure overload dependent heart failure in animal models, in association with shifts in cardiac apoptosis and fibrosis (673). The relationships between low HRV and left ventricular mass (667, 668) is consistent with involvement of autonomic imbalance in hypertrophy. Supporting causal involvement, recent experimental work shows that reinstating HRV reverses detrimental remodelling in an ovine model of heart failure (674).
Altered adipokine signalling may also play some role. Adiponectin is cardioprotective, limiting hypertrophy and heart failure (675, 676) and protecting against sympathetic and myocardial remodelling after infarction (284). Adiponectin levels are generally repressed with chronic stress (283), potentially facilitating stress-dependent dysfunction and hypertrophy. However, early life stress is reported to specifically increase adiponectin levels in female and not male rats (192), reflecting a potentially protective sex dependent response. Interestingly, adiponectin dependent signalling has also been linked to fear extinction, with reduced adiponectin associated with emergence of post-traumatic stress disorder (285, 677). Leptin signalling is also modified with chronic stress, including elevated circulating levels linked to evolving leptin resistance. However, while leptin can be protective, for example limiting hypertrophy and apoptosis, increased levels are strongly linked to CAD risk and poor outcomes (675, 676). Increased leptin levels with stress may thus predispose to CAD development, mortality and morbidity. Early life stress may induce sex dependent changes in leptin, including evidence of a selective fall in leptin in male and not female rats (678). However, the roles of leptin in stress-dependent disease remain unclear (675, 676, 679) and require further study, particularly in terms of the effects of sex.
Oestrogen may protect against cardiac hypertrophy/failure, while post-menopausal women may become sensitised to these outcomes. The protective effects of oestrogen in CAD and maladaptive hypertrophy may involve multiple mechanisms, including influences on sympathetic activity and endothelium-dependent and -independent control of microvascular function (680), lipid handling and profiles, and key transcriptional regulators such as the nuclear factor of activated T-cells (NFATs) (681). Experimental studies show oestrogen does protect against cardiovascular dysfunction in models of Takotsubo syndrome (682, 683), and counters cardiomyopathy in female rodents exposed to chronic catecholamine stress (682). Others report that oestrogenic protection against stress-dependent cardiomyopathy may involve increased β2AR–Gαs signalling activity and reduced catecholamine levels (684).
Both HFpEF and Takotsubo cardiomyopathy are of interest in terms of sex-dependent CVD and stress. Increased immuno-inflammatory reactivity to stress in women is congruent with proposed involvement of coronary vascular inflammation and dysfunction in female linked HFpEF (47, 582). Similarly, Takotsubo cardiomyopathy is thought to involve excessive sympathetic drive (45, 685), and its dominance in post-menopausal women is consistent with enhanced stress-dependent disruption of autonomic control in females as a result of reduced oestrogenic protection (685).
Women are 4- to 10-fold more likely to suffer Takotsubo than men (46), although there is evidence males may suffer greater in-hospital complications (686). The most well-established mechanism in Takotsubo cardiomyopathy is sympathetic over-activation, which involves increased noradrenaline release (and potentially changes in myocardial sensitivity) (45, 685). The effects of stress on the LC-noradrenaline axis, which enhances HPA activity and release of adrenal adrenaline/noradrenaline into the circulation, are sex-dependent (101). Locally released catecholamines may also participate, and may be more damaging than those transported in the circulation (687). Increased myocardial sensitivity to catecholamines in females—particularly within the left ventricular apex—could underlie apical changes characteristic of Takotsubo syndrome (364). Coronary vascular dysfunction may also contribute, with reductions in apical perfusion documented (688). Sympathetic activity favours coronary dysfunction (689), and associated inflammation (690) contributes to endothelial damage. Release of vasoconstrictors and related ROS generation may also participate in coronary vascular changes (691). Thus, both the stress-related sympathetic activity and coronary dysfunction implicated in Takotsubo cardiomyopathy are sex-dependent. This is congruent with the strong sex-dependence of stress related SCAD, also potentially involving excess sympathetic activation (30, 31). Mitochondrial dysfunction may in turn be exacerbated by these autonomic and vascular changes, and there is evidence of an impaired myocardial energy state in Takotsubo cardiomyopathy (692). Though inflammation is also implicated (693, 694), it is interesting to note that that the limited information available on sex differences in the disorder—largely from a series of studies in Japanese populations—supports lower C-reactive protein and leukocyte levels in female patients (46). An extremely limited comparison of cardiac gene expression in a single male patient (>70 years of age) and single female patient (>80 years of age) suggests greater changes in mitochondria related genes in the male compared with greater ECM-receptor and -integrin interaction genes in the female patient (695). However, whether such a difference might be causal, and robust across the sexes, remains to be tested. Such findings nonetheless further evidence of distinct disease processes in males and females.
Stress increases myocardial vulnerability to arrhythmias and coronary ischaemia (696–700). Studies in mice also suggest that mild chronic stress may interact synergistically with a western diet to worsen myocardial ischaemic injury, together with metabolic homeostasis and anxiety-like behaviour (701). We more recently report that chronic modes of social or unpredictable mild stress worsen myocardial ischemic tolerance in male mice, with cardiac outcomes correlating with circulating noradrenaline and leptin levels, and cortical and hippocampal expression of monoamine and inflammatory genes (702). However, we also report that chronic social stress reduces myocardial ischaemic tolerance in male and not female mice, in associated with a sex-specific decline in ventricular expression of genes involved in energy metabolism, mitochondrial biogenesis and cardioprotection (12). While still poorly studied (703), there is also evidence chronic stress inhibits cardioprotective survival kinase activation (704). Our unpublished findings indicate that chronic restraint stress in mice prevents the protective phospho-activation of myocardial protein kinase B (AKT) in response to preconditioning stimuli. Information on the sex dependence of these cardiac effects is lacking.
Mitochondria are responsive to stress (705), and dysfunction is linked to CVD, MDD and chronic stress (706–710). Mitochondrial dysfunction may be a common link between chronic stress and multiple diseases (711). Chronic stress inhibits mitochondrial respiration in nervous tissue of animal models (712), with a link between duration of stress and degree of inhibition (713). Stress effects involve in part direct glucocorticoid responses, with mitochondria possessing glucocorticoid receptors together with sex hormone receptors/transcription factors, providing sensitivity to endocrine function and sex (714, 715). While the sex dependence of mitochondrial dysfunction in CAD awaits detailed analysis, sex does influence cardiac mitochondrial responses to stress (705) and sex differences are evident in mitochondrial roles in stress-related mental disorders (716). Experimental studies also demonstrate broad “mito-protective” functions of oestrogens (717–719), which directly enhance mitochondrial oxidative phosphorylation (720, 721). Apparent resilience of female cardiac mitochondria to acute stressors has been linked to direct effects of oestrogen (705). Others link improved ischaemic tolerance in female mouse hearts to oestrogen dependent improvements in mitochondrial connexin-43 content and phosphorylation (722). There is evidence mitochondrial dysfunction (in brain tissue) is more sensitive to oestrogen than testosterone deficiency (723). While cardiac mitochondrial dysfunction is enhanced by both testosterone (724) or oestrogen deficiency (725) in insulin-resistant male and female rats, there is evidence sex hormone deprivation is more likely to induce cardiac mitochondrial dysfunction in healthy and insulin-resistant male vs. female mice (726). Pereira et al. (727), recently found that reduced maternal nutrition in a baboon model dysregulates foetal cardiac mitochondria in a sex specific manner, which could contribute to sex-dependent programming of adult cardiac dysfunction. Similarly, Louwagie et al. found that foetal exposure to maternal glucolipotoxicity modifies metabolism, propensity to cell death and risk of adult heart disease via mitochondrial mechanisms in a sex-dependent manner (suggestive of improved mitochondrial quality control in females, and greater vulnerability in adult males) (728).
Differing responses to chronic forms of psychosocial stress contribute to sex-dependent CAD and its impacts, however significant knowledge gaps exist in terms of the interactions between sex, stress and disease. This demands an increased focus on sex biology and dependent disease mechanisms. Evolutionary, socio-cultural and intrinsic biological factors all interact in generating differing perceptions of stress, and the biological transduction of stress to different behavioural, emotional and physiological outcomes. This distinct stress biology involves molecular, structural and functional differences within the HPA axis and ANS, together with immuno-inflammatory, neurotrophin and endocrine function, pyrimidine metabolism and sirtuin signalling, among other players. Baseline myocardial and coronary physiological differences may interact with these distinct stress responses in the sex-dependent development of cardiovascular disorders, particularly those linked to coronary vascular (dys)function. Despite these clear biological differences, the daily experience of stress remains biased by socio-cultural and economic factors, and stress axis reactivity and responses are similarly influenced by such external determinants. Significant advances in the prevention of chronic disease in women can thus be made at fundamental socio-economic levels. At the same time, greater understanding of sex and stress biology can better inform approaches to CAD prevention (targeting sex specific risk profiles) and therapy (targeting sex dependent biological mechanisms) in both men and women.
TH, JH, NJCS and NB reviewed the literature and contributed to the preparation of this manuscript. All authors contributed to the article and approved the submitted version.
The authors declare that the research was conducted in the absence of any commercial or financial relationships that could be construed as a potential conflict of interest.
All claims expressed in this article are solely those of the authors and do not necessarily represent those of their affiliated organizations, or those of the publisher, the editors and the reviewers. Any product that may be evaluated in this article, or claim that may be made by its manufacturer, is not guaranteed or endorsed by the publisher.
CAD, coronary artery disease; MDD, major depressive disorder; CVD, cardiovascular disorder; AMI, acute myocardial infarction; SHEEP, Stockholm Heart Program; HPA, hypothalamic -pituitary- adrenal; ANS, autonomic nervous system; RAAS, renin-angiotensin-aldosterone; CRH, corticotropin-releasing hormone; AVP, arginine vasopressin; ACTH, adrenocorticotropin-releasing hormone; LC, locus coerulus; BDNF, brain derived neurotropic factor; CNS, central nervous system, NAD+, nicotinamide adenine dinucleotide; PARP, poly-ADP-ribose polymerase; GABA, gamma-aminobutyric acid; NPY, neuropeptide y; GP, glycoprotein; ER, estrogen receptor; LPS, lipopolysaccharide; TNF, tumour necrosis factor; FC, frontal cortex; IL, interleukin; LDL, low-density lipoproteins; VLDL, very-low density lipoproteins; HDL, high-density lipoproteins; NO, nitric oxide; eNOS, endothelial nitric oxide synthase; HFpEF, heart failure with preserved ejection fraction; Apo, apolipoprotein; ROS, reactive oxygen species; NADPH, nicotinamide adenine dinucleotide phosphate; HRV, heart rate variability; NFATs, nuclear factor of activated T-cells; AKT, protein kinase B.
1. Cruz-Pereira JS, Rea K, Nolan YM, O'Leary OF, Dinan TG, Cryan JF. Depression's unholy trinity: dysregulated stress, immunity, and the microbiome. Annu Rev Psychol. (2020) 71:49–78. doi: 10.1146/annurev-psych-122216-011613
2. Surwit RS, Schneider MS, Feinglos MN. Stress and diabetes mellitus. Diabetes Care. (1992) 15(10):1413–22. doi: 10.2337/diacare.15.10.1413
3. Azuma K, Adachi Y, Hayashi H, Kubo KY. Chronic psychological stress as a risk factor of osteoporosis. J UOEH. (2015) 37(4):245–53. doi: 10.7888/juoeh.37.245
4. Dai S, Mo Y, Wang Y, Xiang B, Liao Q, Zhou M, et al. Chronic stress promotes cancer development. Front Oncol. (2020) 10:1492. doi: 10.3389/fonc.2020.01492
5. Steptoe A, Kivimäki M. Stress and cardiovascular disease. Nat Rev Cardiol. (2012) 9(6):360–70. doi: 10.1038/nrcardio.2012.45
6. Stapelberg NJC, Neumann DL, Shum D, Headrick JP. Health, pre-disease and critical transition to disease in the psycho-immune-neuroendocrine network: are there distinct states in the progression from health to major depressive disorder? Physiol Behav. (2019) 198:108–19. doi: 10.1016/j.physbeh.2018.10.014
7. Slagboom MN, Reis R, Tsai AC, Büchner FL, van Dijk DJA, Crone MR. Psychological distress, cardiometabolic diseases and musculoskeletal pain: a cross-sectional, population-based study of syndemic ill health in a Dutch fishing village. J Glob Health. (2021) 11:04029. doi: 10.7189/jogh.11.04029
8. Freedland KE, Skala JA, Carney RM, Steinmeyer BC, Rich MW. Psychosocial syndemics and multimorbidity in patients with heart failure. J Psychiatr Brain Sci. (2021) 6:e210006. doi: 10.20900/jpbs.20210006
9. Zhou X, Nguyen-Feng VN, Wamser-Nanney R, Lotzin A. Racism, posttraumatic stress symptoms, and racial disparity in the U.S. COVID-19 syndemic. Behav Med. (2022) 48(2):85–94. doi: 10.1080/08964289.2021.2006131
10. Duby Z, Bunce B, Fowler C, Bergh K, Jonas K, Dietrich JJ, et al. Intersections between COVID-19 and socio-economic mental health stressors in the lives of South African adolescent girls and young women. Child Adolesc Psychiatry Ment Health. (2022) 16(1):23. doi: 10.1186/s13034-022-00457-y
11. Azevedo KJ, Kalvesmaki AF, Riendeau RP, Sweet PA, Holmes SM. Leveraging anthropological expertise to respond to the COVID-19 global mental health syndemic. Am Anthropol. (2022. doi: 10.1111/aman.13747
12. Helman TJ, Headrick JP, Vider J, Peart JN, Stapelberg NJC. Sex-specific behavioral, neurobiological, and cardiovascular responses to chronic social stress in mice. J Neurosci Res. (2022) 100(11):2004–27. doi: 10.1002/jnr.25115
13. Meng LB, Qi R, Xu L, Chen Y, Yu Z, Guo P, et al. The more critical murderer of atherosclerosis than lipid metabolism: chronic stress. Lipids Health Dis. (2018) 17(1):143. doi: 10.1186/s12944-018-0795-4
15. Rozanski A, Blumenthal JA, Kaplan J. Impact of psychological factors on the pathogenesis of cardiovascular disease and implications for therapy. Circulation. (1999) 99(16):2192–217. doi: 10.1161/01.cir.99.16.2192
16. Tawakol A, Ishai A, Takx RA, Figueroa AL, Ali A, Kaiser Y, et al. Relation between resting amygdalar activity and cardiovascular events: a longitudinal and cohort study. Lancet. (2017) 389(10071):834–45. doi: 10.1016/S0140-6736(16)31714-7
17. Pimple P, Lima BB, Hammadah M, Wilmot K, Ramadan R, Levantsevych O, et al. Psychological distress and subsequent cardiovascular events in individuals with coronary artery disease. J Am Heart Assoc. (2019) 8(9):e011866. doi: 10.1161/JAHA.118.011866
18. Rosengren A, Hawken S, Ounpuu S, Sliwa K, Zubaid M, Almahmeed WA, et al. Association of psychosocial risk factors with risk of acute myocardial infarction in 11119 cases and 13648 controls from 52 countries (the INTERHEART study): case-control study. Lancet. (2004) 364(9438):953–62. doi: 10.1016/S0140-6736(04)17019-0
19. Theorell T, Tsutsumi A, Hallquist J, Reuterwall C, Hogstedt C, Fredlund P, et al. Decision latitude, job strain, and myocardial infarction: a study of working men in Stockholm. The SHEEP study group. Stockholm heart epidemiology program. Am J Public Health. (1998) 88(3):382–8. doi: 10.2105/ajph.88.3.382
20. Bosma H, Peter R, Siegrist J, Marmot M. Two alternative job stress models and the risk of coronary heart disease. Am J Public Health. (1998) 88(1):68–74. doi: 10.2105/ajph.88.1.68
21. Ferrie JE, Kivimäki M, Shipley MJ, Davey Smith G, Virtanen M. Job insecurity and incident coronary heart disease: the whitehall II prospective cohort study. Atherosclerosis. (2013) 227(1):178–81. doi: 10.1016/j.atherosclerosis.2012.12.027
22. Moran KE, Ommerborn MJ, Blackshear CT, Sims M, Clark CR. Financial stress and risk of coronary heart disease in the Jackson heart study. Am J Prev Med. (2019) 56(2):224–31. doi: 10.1016/j.amepre.2018.09.022
23. Mommersteeg PM, Arts L, Zijlstra W, Widdershoven JW, Aarnoudse W, Denollet J. Impaired health Status, psychological distress, and personality in women and men with nonobstructive coronary artery disease: sex and gender differences: the TWIST (tweesteden mild stenosis) study. Circ Cardiovasc Qual Outcomes. (2017) 10(2):e003387. doi: 10.1161/CIRCOUTCOMES.116.003387
24. Kivimäki M, Steptoe A. Effects of stress on the development and progression of cardiovascular disease. Nat Rev Cardiol. (2018) 15(4):215–29. doi: 10.1038/nrcardio.2017.189
25. Mittleman MA, Mostofsky E. Physical, psychological and chemical triggers of acute cardiovascular events: preventive strategies. Circulation. (2011) 124(3):346–54. doi: 10.1161/CIRCULATIONAHA.110.968776
26. Leor J, Poole WK, Kloner RA. Sudden cardiac death triggered by an earthquake. N Engl J Med. (1996) 334(7):413–9. doi: 10.1056/NEJM199602153340701
27. Smyth A, O’Donnell M, Lamelas P, Teo K, Rangarajan S. Yusuf S; INTERHEART investigators. Physical activity and anger or emotional upset as triggers of acute myocardial infarction: the INTERHEART study. Circulation. (2016) 134(15):1059–67. doi: 10.1161/CIRCULATIONAHA.116.023142
28. Edmondson D, Newman JD, Whang W, Davidson KW. Emotional triggers in myocardial infarction: do they matter? Eur Heart J. (2013) 34(4):300–6. doi: 10.1093/eurheartj/ehs398
29. Mostofsky E, Penner EA, Mittleman MA. Outbursts of anger as a trigger of acute cardiovascular events: a systematic review and meta-analysis. Eur Heart J. (2014) 35(21):1404–10. doi: 10.1093/eurheartj/ehu033
30. Hayes SN, Kim ESH, Saw J, Adlam D, Arslanian-Engoren C, Economy KE, et al.; American heart association council on peripheral vascular disease; council on clinical cardiology; council on cardiovascular and stroke nursing; council on genomic and precision medicine; and stroke council. Spontaneous coronary artery dissection: current state of the science: a scientific statement from the American heart association. Circulation. (2018) 137(19):e523–557. doi: 10.1161/CIR.0000000000000564
31. Khiatah B, Jazayeri S, Yamamoto N, Burt T, Frugoli A, Brooks DL. Cardiovascular disease in women: a review of spontaneous coronary artery dissection. Medicine (Baltimore). (2022) 101(38):e30433. doi: 10.1097/MD.0000000000030433
32. Woitowich NC, Beery A, Woodruff T. A 10-year follow-up study of sex inclusion in the biological sciences. eLife. (2020) 9:e56344. doi: 10.7554/eLife.56344
33. Rechlin RK, Splinter TFL, Hodges TE, Albert AY, Galea LAM. An analysis of neuroscience and psychiatry papers published from 2009 to 2019 outlines opportunities for increasing discovery of sex differences. Nat Commun. (2022) 13(1):2137. doi: 10.1038/s41467-022-29903-3
34. Mascitelli L, Pezzetta F. Cardiovascular protection in premenopausal women. Arch Intern Med. (2005) 165(1):120. doi: 10.1001/archinte.165.1.120-a
35. Gorodeski GI. Update on cardiovascular disease in post-menopausal women. Best Pract Res Clin Obstet Gynaecol. (2002) 16(3):329–55. doi: 10.1053/beog.2002.0282
36. Cenko E, Yoon J, Kedev S, Stankovic G, Vasiljevic Z, Krljanac G, et al. Sex differences in outcomes after STEMI: effect modification by treatment strategy and age. JAMA Intern Med. (2018) 178(5):632–39. doi: 10.1001/jamainternmed.2018.0514
37. Zachura M, Wilczek K, Janion M, Gąsior M, Gierlotka M, Sadowski M. Long-term outcomes in men and women with ST-segment elevation myocardial infarction and incomplete reperfusion after a primary percutaneous coronary intervention: a 2-year follow-up. Coron Artery Dis. (2019) 30(3):171–76. doi: 10.1097/MCA.0000000000000703
38. Pain TE, Jones DA, Rathod KS, Gallagher SM, Knight CJ, Mathur A, et al. Influence of female sex on long-term mortality after acute coronary syndromes treated by percutaneous coronary intervention: a cohort study of 7304 patients. Coron Artery Dis. (2013) 24(3):183–90. doi: 10.1097/MCA.0b013e32835d75f0
39. Ghadri JR, Sarcon A, Jaguszewski M, Diekmann J, Bataiosu RD, Hellermann J, et al. Gender disparities in acute coronary syndrome: a closing gap in the short-term outcome. J Cardiovasc Med (Hagerstown). (2015) 16(5):355–62. doi: 10.2459/JCM.0000000000000248
40. Kumbhani DJ, Shishehbor MH, Willis JM, Karim S, Singh D, Bavry AA, et al. Influence of gender on long-term mortality in patients presenting with non-ST-elevation acute coronary syndromes undergoing percutaneous coronary intervention. Am J Cardiol. (2012) 109(8):1087–91. doi: 10.1016/j.amjcard.2011.11.044
41. Vaccarino V, Krumholz HM, Yarzebski J, Gore JM, Goldberg RJ. Sex differences in 2-year mortality after hospital discharge for myocardial infarction. Ann Intern Med. (2001) 134(3):173–81. doi: 10.7326/0003-4819-134-3-200102060-00007
42. McGrady A, McGinnis R, Badenhop D, Bentle M, Rajput M. Effects of depression and anxiety on adherence to cardiac rehabilitation. J Cardiopulm Rehabil Prev. (2009) 29(6):358–64. doi: 10.1097/HCR.0b013e3181be7a8f
43. Vaccarino V, Wilmot K, Al Mheid I, Ramadan R, Pimple P, Shah AJ, et al. Sex differences in mental stress-induced myocardial ischemia in patients with coronary heart disease. J Am Heart Assoc. (2016) 5(9):e003630. doi: 10.1161/JAHA.116.003630
44. Vaccarino V, Sullivan S, Hammadah M, Wilmot K, Al Mheid I, Ramadan R, et al. Mental stress-induced-myocardial ischemia in young patients with recent myocardial infarction: sex differences and mechanisms. Circulation. (2018) 137(8):794–805. doi: 10.1161/CIRCULATIONAHA.117.030849
45. Lyon AR, Citro R, Schneider B, Morel O, Ghadri JR, Templin C, et al. Pathophysiology of takotsubo syndrome: jACC state-of-the-art review. J Am Coll Cardiol. (2021) 77(7):902–21. doi: 10.1016/j.jacc.2020.10.060
46. Murakami T, Komiyama T, Kobayashi H, Ikari Y. Gender differences in takotsubo syndrome. Biology (Basel). (2022) 11(5):653. doi: 10.3390/biology11050653
47. Lam CSP, Arnott C, Beale AL, Chandramouli C, Hilfiker-Kleiner D, Kaye DM, et al. Sex differences in heart failure. Eur Heart J. (2019) 40(47):3859–3868c. doi: 10.1093/eurheartj/ehz835
48. Labonté B, Engmann O, Purushothaman I, Menard C, Wang J, Tan C, et al. Sex-specific transcriptional signatures in human depression. Nat Med. (2017) 23(9):1102–11. doi: 10.1038/nm.4386
49. Karisetty BC, Khandelwal N, Kumar A, Chakravarty S. Sex difference in mouse hypothalamic transcriptome profile in stress-induced depression model. Biochem Biophys Res Commun. (2017) 486(4):1122–8. doi: 10.1016/j.bbrc.2017.04.005
50. Scarpa JR, Fatma M, Loh YE, Traore SR, Stefan T, Chen TH, et al. Shared transcriptional signatures in Major depressive disorder and mouse chronic stress models. Biol Psychiatry. (2020) 88(2):159–68. doi: 10.1016/j.biopsych.2019.12.029
51. Arnold AP. Rethinking sex determination of non-gonadal tissues. Curr Top Dev Biol. (2019) 134:289–315. doi: 10.1016/bs.ctdb.2019.01.003
52. Arnold AP. Sexual differentiation of brain and other tissues: five questions for the next 50 years. Horm Behav. (2020) 120:104691. doi: 10.1016/j.yhbeh.2020.104691
53. McCarthy MM, Arnold AP. Reframing sexual differentiation of the brain. Nat Neurosci. (2011) 14(6):677–83. doi: 10.1038/nn.2834
54. Leitner N, Ben-Shahar Y. The neurogenetics of sexually dimorphic behaviors from a postdevelopmental perspective. Genes Brain Behav. (2020) 19(2):e12623. doi: 10.1111/gbb.12623
55. McCarthy MM, Nugent BM. At the frontier of epigenetics of brain sex differences. Front Behav Neurosci. (2015) 9:221. doi: 10.3389/fnbeh.2015.00221
56. Forger NG. Epigenetic mechanisms in sexual differentiation of the brain and behaviour. Philos Trans R Soc Lond B Biol Sci. (2016) 371(1688):20150114. doi: 10.1098/rstb.2015.0114
57. Cohen S, Murphy MLM, Prather AA. Ten surprising facts about stressful life events and disease risk. Annu Rev Psychol. (2019) 70:577–97. doi: 10.1146/annurev-psych-010418-102857
58. Ervin J, Taouk Y, Alfonzo LF, Hewitt B, King T. Gender differences in the association between unpaid labour and mental health in employed adults: a systematic review. Lancet Public Health. (2022) 7(9):e775–786. doi: 10.1016/S2468-2667(22)00160-8
59. Harryson L, Strandh M, Hammarström A. Domestic work and psychological distress–what is the importance of relative socioeconomic position and gender inequality in the couple relationship? PLoS One. (2012) 7(6):e38484. doi: 10.1371/journal.pone.0038484
60. Harryson L, Novo M, Hammarström A. Is gender inequality in the domestic sphere associated with psychological distress among women and men? Results from the northern Swedish cohort. J Epidemiol Community Health. (2012) 66(3):271–6. doi: 10.1136/jech.2010.109231
61. Maeda E, Nomura K, Hiraike O, Sugimori H, Kinoshita A, Osuga Y. Domestic work stress and self-rated psychological health among women: a cross-sectional study in Japan. Environ Health Prev Med. (2019) 24(1):75. doi: 10.1186/s12199-019-0833-5
62. LeClair KB, Chan KL, Kaster MP, Parise LF, Burnett CJ, Russo SJ. Individual history of winning and hierarchy landscape influence stress susceptibility in mice. eLife. (2021) 10:e71401. doi: 10.7554/eLife.71401
63. Karamihalev S, Brivio E, Flachskamm C, Stoffel R, Schmidt MV, Chen A. Social dominance mediates behavioral adaptation to chronic stress in a sex-specific manner. eLife. (2020) 9:e58723. doi: 10.7554/eLife.58723
64. Bangasser DA, Wicks B. Sex-specific mechanisms for responding to stress. J Neurosci Res. (2017) 95(1–2):75–82. doi: 10.1002/jnr.23812
65. Vieira JO, Duarte JO, Costa-Ferreira W, Morais-Silva G, Marin MT, Crestani CC. Sex differences in cardiovascular, neuroendocrine and behavioral changes evoked by chronic stressors in rats. Prog Neuropsychopharmacol Biol Psychiatry. (2018) 81:426–37. doi: 10.1016/j.pnpbp.2017.08.014
66. Byrd-Craven J, Geary DC. Biological and evolutionary contributions to developmental sex differences. Reprod Biomed Online. (2007) 15(Suppl 2):12–22. doi: 10.1016/s1472-6483(10)60545-7
67. Archer J. The reality and evolutionary significance of human psychological sex differences. Biol Rev Camb Philos Soc. (2019) 94(4):1381–415. doi: 10.1111/brv.12507
68. Glover V, Hill J. Sex differences in the programming effects of prenatal stress on psychopathology and stress responses: an evolutionary perspective. Physiol Behav. (2012) 106(5):736–40. doi: 10.1016/j.physbeh.2012.02.011
69. Mogil JS. Qualitative sex differences in pain processing: emerging evidence of a biased literature. Nat Rev Neurosci. (2020) 21(7):353–65. doi: 10.1038/s41583-020-0310-6
70. Tuovinen N, Yalcin-Siedentopf N, Welte AS, Siedentopf CM, Steiger R, Gizewski ER, et al. Neurometabolite correlates with personality and stress in healthy emerging adults: a focus on sex differences. Neuroimage. (2022) 247:118847. doi: 10.1016/j.neuroimage.2021.118847
71. Graves BS, Hall ME, Dias-Karch C, Haischer MH, Apter C. Gender differences in perceived stress and coping among college students. PLoS One. (2021) 16(8):e0255634. doi: 10.1371/journal.pone.0255634
72. Xu X, Bao H, Strait K, Spertus JA, Lichtman JH, D'Onofrio G, et al. Sex differences in perceived stress and early recovery in young and middle-aged patients with acute myocardial infarction. Circulation. (2015) 131(7):614–23. doi: 10.1161/CIRCULATIONAHA.114.012826
73. Yalcin-Siedentopf N, Pichler T, Welte AS, Hoertnagl CM, Klasen CC, Kemmler G, et al. Sex matters: stress perception and the relevance of resilience and perceived social support in emerging adults. Arch Womens Ment Health. (2021) 24(3):403–11. doi: 10.1007/s00737-020-01076-2
74. Shimanoe C, Hara M, Nishida Y, Nanri H, Otsuka Y, Horita M, et al. Coping strategy and social support modify the association between perceived stress and C-reactive protein: a longitudinal study of healthy men and women. Stress. (2018) 21(3):237–46. doi: 10.1080/10253890.2018.1435638
75. Shimanoe C, Otsuka Y, Hara M, Nanri H, Nishida Y, Nakamura K, et al. Gender-specific associations of perceived stress and coping strategies with C-reactive protein in middle-aged and older men and women. Int J Behav Med. (2014) 21(5):821–32. doi: 10.1007/s12529-013-9341-y
76. American Psychiatric Association. DSM-5 Task force. Diagnostic and statistical manual of mental disorders: DSM-5. Washington, DC, USA: American Psychiatric Association (2013).
77. Coker AL, Weston R, Creson DL, Justice B, Blakeney P. PTSD Symptoms among men and women survivors of intimate partner violence: the role of risk and protective factors. Violence Vict. (2005) 20(6):625–43. doi: 10.1891/0886-6708.20.6.625
78. Gilboa A, Shalev A, Loar L, Lester H, Louzoun Y, Chisin R, et al. Functional connectivity of the prefrontal cortex and the amygdala in posttraumatic stress disorder. Biol Psychiatry. (2004) 55(3):263–72. doi: 10.1016/j.biopsych.2003.08.004
79. Knapska E, Macias M, Mikosz M, Nowak A, Owczarek D, Wawrzyniak M, et al. Functional anatomy of neural circuits regulating fear and extinction. Proc Natl Acad Sci U S A. (2012) 109(42):17093–8. doi: 10.1073/pnas.1202087109
80. Baran SE, Armstrong CE, Niren DC, Hanna JJ, Conrad CD. Chronic stress and sex differences on the recall of fear conditioning and extinction. Neurobiol Learn Mem. (2009) 91(3):323–32. doi: 10.1016/j.nlm.2008.11.005
81. Farrell MR, Sengelaub DR, Wellman CL. Sex differences and chronic stress effects on the neural circuitry underlying fear conditioning and extinction. Physiol Behav. (2013) 122:208–15. doi: 10.1016/j.physbeh.2013.04.002
82. Gruene TM, Roberts E, Thomas V, Ronzio A, Shansky RM. Sex-specific neuroanatomical correlates of fear expression in prefrontal-amygdala circuits. Biol Psychiatry. (2015) 78(3):186–93. doi: 10.1016/j.biopsych.2014.11.014
83. Drummond KD, Waring ML, Faulkner GJ, Blewitt ME, Perry CJ, Kim JH. Hippocampal neurogenesis mediates sex-specific effects of social isolation and exercise on fear extinction in adolescence. Neurobiol Stress. (2021) 15:100367. doi: 10.1016/j.ynstr.2021.100367
84. Shields GS, Kuchenbecker SY, Pressman SD, Sumida KD, Slavich GM. Better cognitive control of emotional information is associated with reduced pro-inflammatory cytokine reactivity to emotional stress. Stress. (2016) 19(1):63–8. doi: 10.3109/10253890.2015.1121983
85. Qiu LR, Germann J, Spring S, Alm C, Vousden DA, Palmert MR, et al. Hippocampal volumes differ across the mouse estrous cycle, can change within 24 h, and associate with cognitive strategies. Neuroimage. (2013) 83:593–8. doi: 10.1016/j.neuroimage.2013.06.074
86. Luine V, Gomez J, Beck K, Bowman R. Sex differences in chronic stress effects on cognition in rodents. Pharmacol Biochem Behav. (2017) 152:13–9. doi: 10.1016/j.pbb.2016.08.005
87. Korol DL, Pisani SL. Estrogens and cognition: friends or foes?: an evaluation of the opposing effects of estrogens on learning and memory. Horm Behav. (2015) 74:105–15. doi: 10.1016/j.yhbeh.2015.06.017
88. Hill RA, Klug M, Kiss Von Soly S, Binder MD, Hannan AJ, van den Buuse M. Sex-specific disruptions in spatial memory and anhedonia in a “two hit” rat model correspond with alterations in hippocampal brain-derived neurotrophic factor expression and signaling. Hippocampus. (2014) 24(10):1197–211. doi: 10.1002/hipo.22302
89. Lei H, Mochizuki Y, Chen C, Hagiwara K, Hirotsu M, Matsubara T, et al. Sex difference in the weighting of expected uncertainty under chronic stress. Sci Rep. (2021) 11(1):8700. doi: 10.1038/s41598-021-88155-1
90. Barko K, Paden W, Cahill KM, Seney ML, Logan RW. Sex-Specific effects of stress on mood-related gene expression. Mol Neuropsychiatry. (2019) 5(3):162–75.31312637
91. Ter Horst JP, de Kloet ER, Schächinger H, Oitzl MS. Relevance of stress and female sex hormones for emotion and cognition. Cell Mol Neurobiol. (2012) 32(5):725–35. doi: 10.1007/s10571-011-9774-2
92. Handa RJ, Burgess LH, Kerr JE, O'Keefe JA. Gonadal steroid hormone receptors and sex differences in the hypothalamo-pituitary-adrenal axis. Horm Behav. (1994) 28(4):464–76. doi: 10.1006/hbeh.1994.1044
93. Moieni M, Irwin MR, Jevtic I, Olmstead R, Breen EC, Eisenberger NI. Sex differences in depressive and socioemotional responses to an inflammatory challenge: implications for sex differences in depression. Neuropsychopharmacology. (2015) 40(7):1709–16. doi: 10.1038/npp.2015.17
94. Hanamsagar R, Bilbo SD. Sex differences in neurodevelopmental and neurodegenerative disorders: focus on microglial function and neuroinflammation during development. J Steroid Biochem Mol Biol. (2016) 160:127–33. doi: 10.1016/j.jsbmb.2015.09.039
95. Arnold AP, Chen X. What does the “four core genotypes” mouse model tell us about sex differences in the brain and other tissues? Front Neuroendocrinol. (2009) 30(1):1–9. doi: 10.1016/j.yfrne.2008.11.001
96. Paden W, Barko K, Puralewski R, Cahill KM, Huo Z, Shelton MA, et al. Sex differences in adult mood and in stress-induced transcriptional coherence across mesocorticolimbic circuitry. Transl Psychiatry. (2020) 10:59. doi: 10.1038/s41398-020-0742-9
97. Seney ML, Huo Z, Cahill K, French L, Puralewski R, Zhang J, et al. Opposite molecular signatures of depression in men and women. Biol Psychiatry. (2018) 84(1):18–27. doi: 10.1016/j.biopsych.2018.01.017
98. Birur B, Amrock EM, Shelton RC, Li L. Sex differences in the peripheral immune system in patients with depression. Front Psychiatry. (2017) 8:108. doi: 10.3389/fpsyt.2017.00108
99. Oyola MG, Handa RJ. Hypothalamic-pituitary-adrenal and hypothalamic-pituitary-gonadal axes: sex differences in regulation of stress responsivity. Stress. (2017) 20(5):476–94. doi: 10.1080/10253890.2017.1369523
100. Heck AL, Handa RJ. Sex differences in the hypothalamic-pituitary-adrenal axis’ response to stress: an important role for gonadal hormones. Neuropsychopharmacology. (2019) 44(1):45–58. doi: 10.1038/s41386-018-0167-9
101. Bangasser DA, Wiersielis KR, Khantsis S. Sex differences in the locus coeruleus-norepinephrine system and its regulation by stress. Brain Res. (2016) 1641(Pt B):177–88. doi: 10.1016/j.brainres.2015.11.021
102. Chen X, Sachdev PS, Wen W, Anstey KJ. Sex differences in regional gray matter in healthy individuals aged 44–48 years: a voxel-based morphometric study. Neuroimage. (2007) 36(3):691–9. doi: 10.1016/j.neuroimage.2007.03.063
103. Cosgrove KP, Mazure CM, Staley JK. Evolving knowledge of sex differences in brain structure, function, and chemistry. Biol Psychiatry. (2007) 62(8):847–55. doi: 10.1016/j.biopsych.2007.03.001
104. Nopoulos P, Flaum M, O'Leary D, Andreasen NC. Sexual dimorphism in the human brain: evaluation of tissue volume, tissue composition and surface anatomy using magnetic resonance imaging. Psychiatry Res. (2000) 98(1):1–13. doi: 10.1016/s0925-4927(99)00044-x
105. Lüders E, Steinmetz H, Jäncke L. Brain size and grey matter volume in the healthy human brain. Neuroreport. (2002) 13(17):2371–4. doi: 10.1097/01.wnr.0000049603.85580.da
106. Kulynych JJ, Vladar K, Jones DW, Weinberger DR. Gender differences in the normal lateralization of the supratemporal cortex: mRI surface-rendering morphometry of heschl's gyrus and the planum temporale. Cereb Cortex. (1994) 4(2):107–18. doi: 10.1093/cercor/4.2.107
107. Leonard CM, Towler S, Welcome S, Halderman LK, Otto R, Eckert MA, et al. Size matters: cerebral volume influences sex differences in neuroanatomy. Cereb Cortex. (2008) 18(12):2920–31. doi: 10.1093/cercor/bhn052
108. Harasty J, Double KL, Halliday GM, Kril JJ, McRitchie DA. Language-associated cortical regions are proportionally larger in the female brain. Arch Neurol. (1997) 54(2):171–6. doi: 10.1001/archneur.1997.00550140045011
109. Filipek PA, Richelme C, Kennedy DN, Caviness VS Jr. The young adult human brain: an MRI-based morphometric analysis. Cerebral Cortex. (1994) 4(4):344–60. 1093/cercor/4.4.344 7950308
110. Allen JS, Damasio H, Grabowski TJ, Bruss J, Zhang W. Sexual dimorphism and asymmetries in the gray-white composition of the human cerebrum. Neuroimage. (2003) 18(4):880–94. doi: 10.1016/s1053-8119(03)00034-x
111. Goldstein JM, Seidman LJ, Horton NJ, Makris N, Kennedy DN, Caviness VS Jr, et al. Normal sexual dimorphism of the adult human brain assessed by in vivo magnetic resonance imaging. Cereb Cortex. (2001) 11(6):490–7. doi: 10.1093/cercor/11.6.490
112. Schlaepfer TE, Harris GJ, Tien AY, Peng L, Lee S, Pearlson GD. Structural differences in the cerebral cortex of healthy female and male subjects: a magnetic resonance imaging study. Psychiatry Res. (1995) 61(3):129–35. doi: 10.1016/0925-4927(95)02634-a
113. Koscik T, O'Leary D, Moser DJ, Andreasen NC, Nopoulos P. Sex differences in parietal lobe morphology: relationship to mental rotation performance. Brain Cogn. (2009) 69(3):451–9. doi: 10.1016/j.bandc.2008.09.004
114. Drysdale AT, Grosenick L, Downar J, Dunlop K, Mansouri F, Meng Y, et al. Erratum: resting-state connectivity biomarkers define neurophysiological subtypes of depression. Nat Med. (2017) 23(2):264. doi: 10.1038/nm0217-264d
115. Greicius MD, Flores BH, Menon V, Glover GH, Solvason HB, Kenna H, et al. Resting-state functional connectivity in major depression: abnormally increased contributions from subgenual cingulate cortex and thalamus. Biol Psychiatry. (2007) 62(5):429–37. doi: 10.1016/j.biopsych.2006.09.020
116. Koolschijn PC, van Haren NE, Lensvelt-Mulders GJ, Hulshoff Pol HE, Kahn RS. Brain volume abnormalities in major depressive disorder: a meta-analysis of magnetic resonance imaging studies. Hum Brain Mapp. (2009) 30(11):3719–35. doi: 10.1002/hbm.20801
117. MacQueen GM, Campbell S, McEwen BS, Macdonald K, Amano S, Joffe RT, et al. Course of illness, hippocampal function, and hippocampal volume in major depression. Proc Natl Acad Sci U S A. (2003) 100(3):1387–92. doi: 10.1073/pnas.0337481100
118. Sheline YI, Wang PW, Gado MH, Csernansky JG, Vannier MW. Hippocampal atrophy in recurrent major depression. Proc Natl Acad Sci U S A. (1996) 93(9):3908–13. doi: 10.1073/pnas.93.9.3908
119. Phan KL, Wager TD, Taylor SF, Liberzon I. Functional neuroimaging studies of human emotions. CNS Spectr. (2004) 9(4):258–66. doi: 10.1017/S1092852900009196
120. Sabatinelli D, Fortune EE, Li Q, Siddiqui A, Krafft C, Oliver WT, et al. Emotional perception: meta-analyses of face and natural scene processing. Neuroimage. (2011) 54(3):2524–33. doi: 10.1016/j.neuroimage.2010.10.011
121. Domes G, Schulze L, Böttger M, Grossmann A, Hauenstein K, Wirtz PH, et al. The neural correlates of sex differences in emotional reactivity and emotion regulation. Hum Brain Mapp. (2010) 31(5):758–69. doi: 10.1002/hbm.20903
122. Stevens JS, Hamann S. Sex differences in brain activation to emotional stimuli: a meta-analysis of neuroimaging studies. Neuropsychologia. (2012) 50(7):1578–93. doi: 10.1016/j.neuropsychologia.2012.03.011
123. Hofer A, Siedentopf CM, Ischebeck A, Rettenbacher MA, Verius M, Felber S, et al. Sex differences in brain activation patterns during processing of positively and negatively valenced emotional words. Psychol Med. (2007) 37(1):109–19. doi: 10.1017/S0033291706008919
124. Garrett JE, Wellman CL. Chronic stress effects on dendritic morphology in medial prefrontal cortex: sex differences and estrogen dependence. Neuroscience. (2009) 162(1):195–207. doi: 10.1016/j.neuroscience.2009.04.057
125. Shansky RM, Hamo C, Hof PR, Lou W, McEwen BS, Morrison JH. Estrogen promotes stress sensitivity in a prefrontal cortex-amygdala pathway. Cereb Cortex. (2010) 20(11):2560–7. doi: 10.1093/cercor/bhq003
126. Shansky RM, Hamo C, Hof PR, McEwen BS, Morrison JH. Stress-induced dendritic remodeling in the prefrontal cortex is circuit specific. Cereb Cortex. (2009) 19(10):2479–84. doi: 10.1093/cercor/bhp003
127. Handa RJ, McGivern RF. Sex differences: stress response. In: reference module in neuroscience and biobehavioral psychology. Amsterdam, Netherlands: Elsevier (2017).
128. Uhart M, Chong RY, Oswald L, Lin PI, Wand GS. Gender differences in hypothalamic-pituitary-adrenal (HPA) axis reactivity. Psychoneuroendocrinology. (2006) 31(5):642–52. doi: 10.1016/j.psyneuen.2006.02.003
129. Kirschbaum C, Kudielka BM, Gaab J, Schommer NC, Hellhammer DH. Impact of gender, menstrual cycle phase, and oral contraceptives on the activity of the hypothalamus-pituitary-adrenal axis. Psychosom Med. (1999) 61(2):154–62. doi: 10.1097/00006842-199903000-00006
130. Larsson CA, Gullberg B, Råstam L, Lindblad U. Salivary cortisol differs with age and sex and shows inverse associations with WHR in Swedish women: a cross-sectional study. BMC Endocr Disord. (2009) 9:16. doi: 10.1186/1472-6823-9-16
131. Strewe C, Moser D, Buchheim JI, Gunga HC, Stahn A, Crucian BE, et al. Sex differences in stress and immune responses during confinement in Antarctica. Biol Sex Differ. (2019) 10(1):20. doi: 10.1186/s13293-019-0231-0
132. Harryson L, Strandh M, Hammarström A. Domestic work and psychological distress–what is the importance of relative socioeconomic position and gender inequality in the couple relationship? PLoS One. (2012) 7(6):e38484. doi: 10.1371/journal.pone.0038484
133. Peeters F, Nicholson NA, Berkhof J. Cortisol responses to daily events in major depressive disorder. Psychosom Med. (2003) 65(5):836–41. doi: 10.1097/01.PSY.0000088594.17747.2E
134. Viau V, Bingham B, Davis J, Lee P, Wong M. Gender and puberty interact on the stress-induced activation of parvocellular neurosecretory neurons and corticotropin-releasing hormone messenger ribonucleic acid expression in the rat. Endocrinology. (2005) 146(1):137–46. doi: 10.1210/en.2004-0846
135. Weinstock M, Razin M, Schorer-Apelbaum D, Men D, McCarty R. Gender differences in sympathoadrenal activity in rats at rest and in response to footshock stress. Int J Dev Neurosci. (1998) 16(3–4):289–95. doi: 10.1016/S0736-5748(98)00021-5
136. Bowman R, MacLusky NJ, Sarmiento Y, Frankfurt M, Gordon M, Luine VN. Sexually dimorphic effects of prenatal stress on cognition, hormonal responses and central neurotransmitters. Endocrinology. (2004) 145:3778–87. doi: 10.1210/en.2003-1759
137. Aloisi AM, Ceccarelli I, Lupo C. Behavioural and hormonal effects of restraint stress and formalin test in male and female rats. Brain Res Bull. (1998) 47(1):57–62. doi: 10.1016/s0361-9230(98)00063-x
138. Goel N, Bale TL. Organizational and activational effects of testosterone on masculinization of female physiological and behavioral stress responses. Endocrinology. (2008) 149(12):6399–405. doi: 10.1210/en.2008-0433
139. Goel N, Bale TL. Sex differences in the serotonergic influence on the hypothalamic-pituitary-adrenal stress axis. Endocrinology. (2010) 151(4):1784–94. doi: 10.1210/en.2009-1180
140. Mitsushima D, Masuda J, Kimura F. Sex differences in the stress-induced release of acetylcholine in the hippocampus and corticosterone from the adrenal cortex in rats. Neuroendocrinology. (2003) 78(4):234–40. doi: 10.1159/000073707
141. Frederic F, Oliver C, Wollman E, Delhaye-Bouchaud N, Mariani J. IL-1 and LPS induce a sexually dimorphic response of the hypothalamo-pituitary-adrenal axis in several mouse strains. Eur Cytokine Netw. (1993) 4(5):321–9.8117933
142. Goel N, Plyler KS, Daniels D, Bale TL. Androgenic influence on serotonergic activation of the HPA stress axis. Endocrinology. (2011) 152(5):2001–10. doi: 10.1210/en.2010-0964
143. Kant GJ, Lenox RH, Bunnell BN, Mougey EH, Pennington LL, Meyerhoff JL. Comparison of stress response in male and female rats: pituitary cyclic AMP and plasma prolactin, growth hormone and corticosterone. Psychoneuroendocrinology. (1983) 8(4):421–8. doi: 10.1016/0306-4530(83)90021-5
144. Kitay JI. Sex differences in adrenal cortical secretion in the rat. Endocrinology. (1961) 68:818–24. doi: 10.1210/endo-68-5-818
145. Rivier C. Female rats release more corticosterone than males in response to alcohol: influence of circulating sex steroids and possible consequences for blood alcohol levels. Alcohol Clin Exp Res. (1993) 17(4):854–9. doi: 10.1111/j.1530-0277.1993.tb00853.x
146. Rivier C. Gender, sex steroids, corticotropin-releasing factor, nitric oxide, and the HPA response to stress. Pharmacol Biochem Behav. (1999) 64(4):739–51. doi: 10.1016/s0091-3057(99)00148-3
147. Spinedi E, Suescun MO, Hadid R, Daneva T, Gaillard RC. Effects of gonadectomy and sex hormone therapy on the endotoxin-stimulated hypothalamo-pituitary-adrenal axis: evidence for a neuroendocrine-immunological sexual dimorphism. Endocrinology. (1992) 131:2430–36. doi: 10.1210/endo.131.5.1330501
148. Aoki M, Shimozuru M, Kikusui T, Takeuchi Y, Mori Y. Sex differences in behavioral and corticosterone responses to mild stressors in ICR mice are altered by ovariectomy in peripubertal period. Zoolog Sci. (2010) 27(10):783–9. doi: 10.2108/zsj.27.783
149. Iwasaki-Sekino A, Mano-Otagiri A, Ohata H, Yamauchi N, Shibasaki T. Gender differences in corticotropin and corticosterone secretion and corticotropin-releasing factor mRNA expression in the paraventricular nucleus of the hypothalamus and the central nucleus of the amygdala in response to footshock stress or psychological stress in rats. Psychoneuroendocrinology. (2009) 34(2):226–37. doi: 10.1016/j.psyneuen.2008.09.003
150. Livezey GT, Miller JM, Vogel WH. Plasma norepinephrine, epinephrine and corticosterone stress responses to restraint in individual male and female rats, and their correlations. Neurosci Lett. (1985) 62(1):51–6. doi: 10.1016/0304-3940(85)90283-6
151. Heinsbroek RP, Van Haaren F, Feenstra MG, Endert E, Van de Poll NE. Sex- and time-dependent changes in neurochemical and hormonal variables induced by predictable and unpredictable footshock. Physiol Behav. (1991) 49(6):1251–6. doi: 10.1016/0031-9384(91)90359-v
152. Rivier C. Stimulatory effect of interleukin-1 beta on the hypothalamic-pituitary-adrenal axis of the rat: influence of age, gender and circulating sex steroids. J Endocrinol. (1994) 140:365–72. doi: 10.1677/joe.0.1400365
153. Seale JV, Wood SA, Atkinson HC, Bate E, Lightman SL, Ingram CD, et al. Gonadectomy reverses the sexually diergic patterns of circadian and stress-induced hypothalamic-pituitary-adrenal axis activity in male and female rats. J Neuroendocrinol. (2004) 16:516–24. doi: 10.1111/j.1365-2826.2004.01195.x
154. Kajantie E, Phillips DI. The effects of sex and hormonal status on the physiological response to acute psychosocial stress. Psychoneuroendocrinology. (2006) 31(2):151–78. doi: 10.1016/j.psyneuen.2005.07.002
155. Babb JA, Masini CV, Day HE, Campeau S. Sex differences in activated corticotropin-releasing factor neurons within stress-related neurocircuitry and hypothalamic-pituitary-adrenocortical axis hormones following restraint in rats. Neuroscience. (2013) 234:40–52. doi: 10.1016/j.neuroscience.2012.12.051
156. Martinez-Muniz GA, Wood SK. Sex differences in the inflammatory consequences of stress: implications for pharmacotherapy. J Pharmacol Exp Ther. (2020) 375(1):161–74. doi: 10.1124/jpet.120.266205
157. Bekhbat M, Neigh GN. Sex differences in the neuro-immune consequences of stress: focus on depression and anxiety. Brain Behav Immun. (2018) 67:1–12. doi: 10.1016/j.bbi.2017.02.006
158. Kokras N, Dalla C, Sideris AC, Dendi A, Mikail HG, Antoniou K, et al. Behavioral sexual dimorphism in models of anxiety and depression due to changes in HPA axis activity. Neuropharmacology. (2012) 62(1):436–45. doi: 10.1016/j.neuropharm.2011.08.025
159. Mekiri M, Gardier AM, David DJ, Guilloux JP. Chronic corticosterone administration effects on behavioral emotionality in female c57bl6 mice. Exp Clin Psychopharmacol. (2017) 25(2):94–104. doi: 10.1037/pha0000112
160. Kaplowitz ET, Savenkova M, Karatsoreos IN, Romeo RD. Somatic and neuroendocrine changes in response to chronic corticosterone exposure during adolescence in male and female rats. J Neuroendocrinol. (2016) 28(2):12336. doi: 10.1111/jne.12336
161. Corrales WA, Silva JP, Parra CS, Olave FA, Aguayo FI, Román-Albasini L, et al. Sex-Dependent changes of miRNA levels in the hippocampus of adrenalectomized rats following acute corticosterone administration. ACS Chem Neurosci. (2021) 12(16):2981–3001. doi: 10.1021/acschemneuro.0c00762
162. Kokras N, Krokida S, Varoudaki TZ, Dalla C. Do corticosterone levels predict female depressive-like behavior in rodents? J Neurosci Res. (2021) 99(1):324–31. doi: 10.1002/jnr.24686
163. Puder JJ, Freda PU, Goland RS, Wardlaw SL. Estrogen modulates the hypothalamic-pituitary-adrenal and inflammatory cytokine responses to endotoxin in women. J Clin Endocrinol Metab. (2001) 86(6):2403–8. doi: 10.1210/jcem.86.6.7528
164. Naninck EF, Lucassen PJ, Bakker J. Sex differences in adolescent depression: do sex hormones determine vulnerability? J Neuroendocrinol. (2011) 23(5):383–92. doi: 10.1111/j.1365-2826.2011.02125.x
165. Young E, Korszun A. Sex, trauma, stress hormones and depression. Mol Psychiatry. (2010) 15(1):23–8. doi: 10.1038/mp.2009.94
166. Minni AM, de Medeiros GF, Helbling JC, Duittoz A, Marissal-Arvy N, Foury A, et al. Role of corticosteroid binding globulin in emotional reactivity sex differences in mice. Psychoneuroendocrinology. (2014) 50:252–63. doi: 10.1016/j.psyneuen.2014.07.029
167. O'Donnell T, Hegadoren KM, Coupland NC. Noradrenergic mechanisms in the pathophysiology of post-traumatic stress disorder. Neuropsychobiology. (2004) 50(4):273–83. doi: 10.1159/000080952
168. Gold PW, Chrousos GP. Organization of the stress system and its dysregulation in melancholic and atypical depression: high vs low CRH/NE states. Mol Psychiatry. (2002) 7(3):254–75. doi: 10.1038/sj.mp.4001032
169. Koob GF. Corticotropin-releasing factor, norepinephrine, and stress. Biol Psychiatry. (1999) 46(9):1167–80. doi: 10.1016/s0006-3223(99)00164-x
170. Guillamón A, de Blas MR, Segovia S. Effects of sex steroids on the development of the locus coeruleus in the rat. Brain Res. (1988) 468(2):306–10. doi: 10.1016/0165-3806(88)90143-5
171. Luque JM, de Blas MR, Segovia S, Guillamón A. Sexual dimorphism of the dopamine-beta-hydroxylase-immunoreactive neurons in the rat locus ceruleus. Brain Res Dev Brain Res. (1992) 67(2):211–5. doi: 10.1016/0165-3806(92)90221-H
172. Pinos H, Collado P, Rodríguez-Zafra M, Rodríguez C, Segovia S, Guillamón A. The development of sex differences in the locus coeruleus of the rat. Brain Res Bull. (2001) 56(1):73–8. doi: 10.1016/S0361-9230(01)00540-8
173. Bangasser DA, Zhang X, Garachh V, Hanhauser E, Valentino RJ. Sexual dimorphism in locus coeruleus dendritic morphology: a structural basis for sex differences in emotional arousal. Physiol Behav. (2011) 103(3–4):342–51. doi: 10.1016/j.physbeh.2011.02.037
174. Bangasser DA, Curtis A, Reyes BA, Bethea TT, Parastatidis I, Ischiropoulos H, et al. Sex differences in corticotropin-releasing factor receptor signaling and trafficking: potential role in female vulnerability to stress-related psychopathology. Mol Psychiatry. (2010) 15(9):877; 896–904. doi: 10.1038/mp.2010.66
175. Valentino RJ, Van Bockstaele E, Bangasser D. Sex-specific cell signaling: the corticotropin-releasing factor receptor model. Trends Pharmacol Sci. (2013) 34(8):437–44. doi: 10.1016/j.tips.2013.06.004
176. Valentino RJ, Bangasser D, Van Bockstaele EJ. Sex-biased stress signaling: the corticotropin-releasing factor receptor as a model. Mol Pharmacol. (2013) 83(4):737–45. doi: 10.1124/mol.112.083550
177. Lasselin J, Lekander M, Axelsson J, Karshikoff B. Sex differences in how inflammation affects behavior: what we can learn from experimental inflammatory models in humans. Front Neuroendocrinol. (2018) 50:91–106. doi: 10.1016/j.yfrne.2018.06.005
178. Mello BSF, Chaves Filho AJM, Custódio CS, Cordeiro RC, Miyajima F, de Sousa FCF, et al. Sex influences in behavior and brain inflammatory and oxidative alterations in mice submitted to lipopolysaccharide-induced inflammatory model of depression. J Neuroimmunol. (2018) 320:133–42. doi: 10.1016/j.jneuroim.2018.04.009
179. Millett CE, Phillips BE, Saunders EFH. The sex-specific effects of LPS on depressive-like behavior and oxidative stress in the hippocampus of the mouse. Neuroscience. (2019) 399:77–88. doi: 10.1016/j.neuroscience.2018.12.008
180. Brydges NM, Moon A, Rule L, Watkin H, Thomas KL, Hall J. Sex specific effects of pre-pubertal stress on hippocampal neurogenesis and behaviour. Transl Psychiatry. (2018) 8(1):271. doi: 10.1038/s41398-018-0322-4
181. Engler H, Benson S, Wegner A, Spreitzer I, Schedlowski M, Elsenbruch S. Men and women differ in inflammatory and neuroendocrine responses to endotoxin but not in the severity of sickness symptoms. Brain Behav Immun. (2016) 52:18–26. doi: 10.1016/j.bbi.2015.08.013
182. Pyter LM, Kelly SD, Harrell CS, Neigh GN. Sex differences in the effects of adolescent stress on adult brain inflammatory markers in rats. Brain Behav Immun. (2013) 30:88–94. doi: 10.1016/j.bbi.2013.01.075
183. De Meij J, Alfanek Z, Morel L, Decoeur F, Leyrolle Q, Picard K, et al. Microglial cannabinoid type 1 receptor regulates brain inflammation in a sex-specific manner. Cannabis Cannabinoid Res. (2021) 6(6):488–507. doi: 10.1089/can.2020.0170
184. Slavich GM, Sacher J. Stress, sex hormones, inflammation, and major depressive disorder: extending social signal transduction theory of depression to account for sex differences in mood disorders. Psychopharmacology (Berl). (2019) 236(10):3063–79. doi: 10.1007/s00213-019-05326-9
185. Fallon IP, Tanner MK, Greenwood BN, Baratta MV. Sex differences in resilience: experiential factors and their mechanisms. Eur J Neurosci. (2020) 52(1):2530–47. doi: 10.1111/ejn.14639
186. Bekhbat M, Howell PA, Rowson SA, Kelly SD, Tansey MG, Neigh GN. Chronic adolescent stress sex-specifically alters central and peripheral neuro-immune reactivity in rats. Brain Behav Immun. (2019) 76:248–57. doi: 10.1016/j.bbi.2018.12.005
187. Gilman SE, Cherkerzian S, Buka SL, Hahn J, Hornig M, Goldstein JM. Prenatal immune programming of the sex-dependent risk for major depression. Transl Psychiatry. (2016) 6(5):e822. doi: 10.1038/tp.2016.91
188. Goldstein JM, Holsen L, Huang G, Hammond BD, James-Todd T, Cherkerzian S, et al. Prenatal stress-immune programming of sex differences in comorbidity of depression and obesity/metabolic syndrome. Dialogues Clin Neurosci. (2016) 18(4):425–36. doi: 10.31887/DCNS.2016.18.4/jgoldstein
189. Goldstein JM, Cherkerzian S, Seidman LJ, Donatelli JA, Remington AG, Tsuang MT, et al. Prenatal maternal immune disruption and sex-dependent risk for psychoses. Psychol Med. (2014) 44(15):3249–61. doi: 10.1017/S0033291714000683
190. Zitman FM, Richter-Levin G. Age and sex-dependent differences in activity, plasticity and response to stress in the dentate gyrus. Neuroscience. (2013) 249:21–30. doi: 10.1016/j.neuroscience.2013.05.030
191. Tyra AT, Cook TE, Young DA, Hurley PE, Oosterhoff BJ, John-Henderson NA, et al. Adverse childhood experiences, sex, and cardiovascular habituation to repeated stress. Biol Psychol. (2021) 165:108175. doi: 10.1016/j.biopsycho.2021.108175
192. Krolow R, Noschang C, Arcego DM, Huffell AP, Marcolin ML, Benitz AN, et al. Sex-specific effects of isolation stress and consumption of palatable diet during the prepubertal period on metabolic parameters. Metab Clin Exp. (2013) 62(9):1268–78. doi: 10.1016/j.metabol.2013.04.009
193. Baratta MV, Gruene TM, Dolzani SD, Chun LE, Maier SF, Shansky RM. Controllable stress elicits circuit-specific patterns of prefrontal plasticity in males, but not females. Brain Struct Funct. (2019) 224(5):1831–43. doi: 10.1007/s00429-019-01875-z
194. Guadagno A, Wong TP, Walker CD. Morphological and functional changes in the preweaning basolateral amygdala induced by early chronic stress associate with anxiety and fear behavior in adult male, but not female rats. Prog Neuropsychopharmacol Biol Psychiatry. (2018) 81:25–37. doi: 10.1016/j.pnpbp.2017.09.025
195. Guadagno A, Verlezza S, Long H, Wong TP, Walker CD. It is all in the right amygdala: increased synaptic plasticity and perineuronal nets in male, but not female, juvenile rat pups after exposure to early-life stress. J Neurosci. (2020) 40(43):8276–91. doi: 10.1523/JNEUROSCI.1029-20.2020
196. Loi M, Koricka S, Lucassen PJ, Joëls M. Age- and sex-dependent effects of early life stress on hippocampal neurogenesis. Front Endocrinol (Lausanne). (2014) 5:13. doi: 10.3389/fendo.2014.00013
197. Westenbroek C, Den Boer JA, Veenhuis M, Ter Horst GJ. Chronic stress and social housing differentially affect neurogenesis in male and female rats. Brain Res Bull. (2004) 64(4):303–8. doi: 10.1016/j.brainresbull.2004.08.006
198. Hillerer KM, Neumann ID, Couillard-Despres S, Aigner L, Slattery DA. Sex-dependent regulation of hippocampal neurogenesis under basal and chronic stress conditions in rats. Hippocampus. (2013) 23(6):476–87. doi: 10.1002/hipo.22107
199. Barha CK, Brummelte S, Lieblich SE, Galea LA. Chronic restraint stress in adolescence differentially influences hypothalamic-pituitary-adrenal axis function and adult hippocampal neurogenesis in male and female rats. Hippocampus. (2011) 21(11):1216–27. doi: 10.1002/hipo.20829
200. Weisbrod AS, Barry ES, Graham AM, Eklund M, Grunberg NE. Decreased BDNF in female but not male rats after exposure to stress: a sex-sensitive rat model of stress? Stress. (2019) 22(5):581–91. doi: 10.1080/10253890.2019.1617692
201. Niknazar S, Nahavandi A, Peyvandi AA, Peyvandi H, Akhtari AS, Karimi M. Comparison of the adulthood chronic stress effect on hippocampal BDNF signaling in male and female rats. Mol Neurobiol. (2016) 53(6):4026–33. doi: 10.1007/s12035-015-9345-5
202. Lin Y, Ter Horst GJ, Wichmann R, Bakker P, Liu A, Li X, et al. Sex differences in the effects of acute and chronic stress and recovery after long-term stress on stress-related brain regions of rats. Cereb Cortex. (2009) 19(9):1978–89. doi: 10.1093/cercor/bhn225
203. Liu LL, Li JM, Su WJ, Wang B, Jiang CL. Sex differences in depressive-like behaviour may relate to imbalance of microglia activation in the hippocampus. Brain Behav Immun. (2019) 81:188–97. doi: 10.1016/j.bbi.2019.06.012
204. Amadio P, Colombo GI, Tarantino E, Gianellini S, Ieraci A, Brioschi M, et al. BDNFVal66met polymorphism: a potential bridge between depression and thrombosis. Eur Heart J. (2017) 38(18):1426–35. doi: 10.1093/eurheartj/ehv655
205. Sandrini L, Amadio P, Ieraci A, Malara A, Werba JP, Soprano PM, et al. The α2-adrenergic receptor pathway modulating depression influences the risk of arterial thrombosis associated with BDNFVal66Met polymorphism. Biomed Pharmacother. (2022) 146:112557. doi: 10.1016/j.biopha.2021.112557
206. Braidy N, Guillemin G, Grant R. Promotion of cellular NAD(+) anabolism: therapeutic potential for oxidative stress in ageing and Alzheimer's disease. Neurotox Res. (2008) 13(3–4):173–84. doi: 10.1007/BF03033501
207. Braidy N, Guillemin GJ, Mansour H, Chan-Ling T, Poljak A, Grant R. Age related changes in NAD+ metabolism oxidative stress and Sirt1 activity in wistar rats. PLoS One. (2011) 6(4):e19194. doi: 10.1371/journal.pone.0019194
208. Braidy N, Poljak A, Grant R, Jayasena T, Mansour H, Chan-Ling T, et al. Mapping NAD(+) metabolism in the brain of ageing wistar rats: potential targets for influencing brain senescence. Biogerontology. (2014) 15(2):177–98. doi: 10.1007/s10522-013-9489-5
209. Clement J, Wong M, Poljak A, Sachdev P, Braidy N. The plasma NAD+ metabolome is dysregulated in “normal” aging. Rejuvenation Res. (2019) 22(2):121–30. doi: 10.1089/rej.2018.2077
210. Massudi H, Grant R, Braidy N, Guest J, Farnsworth B, Guillemin GJ. Age-associated changes in oxidative stress and NAD+ metabolism in human tissue. PLoS One. (2012) 7(7):e42357. doi: 10.1371/journal.pone.0042357
211. Seyedsadjadi N, Berg J, Bilgin AA, Braidy N, Salonikas C, Grant R. High protein intake is associated with low plasma NAD+ levels in a healthy human cohort. PLoS One. (2018) 13(8):e0201968. doi: 10.1371/journal.pone.0201968
212. Yoshino J, Baur JA, Imai SI. NAD+ intermediates: the biology and therapeutic potential of NMN and NR. Cell Metab. (2018) 27(3):513–28. doi: 10.1016/j.cmet.2017.11.002
213. Berthiaume JM, Kurdys JG, Muntean DM, Rosca MG. Mitochondrial NAD+/NADH redox state and diabetic cardiomyopathy. Antioxid Redox Signal. (2019) 30(3):375–98. doi: 10.1089/ars.2017.7415
214. Thomas M, Palombo P, Schuhmacher T, von Scheven G, Bazylianska V, Salzwedel J, et al. Impaired PARP activity in response to the β-adrenergic receptor agonist isoproterenol. Toxicol in Vitro. (2018) 50:29–39. doi: 10.1016/j.tiv.2018.02.001
215. Zhang F, Zhu X, Yu P, Sheng T, Wang Y, Ye Y. Crocin ameliorates depressive-like behaviors induced by chronic restraint stress via the NAMPT-NAD+-SIRT1 pathway in mice. Neurochem Int. (2022) 157:105343. doi: 10.1016/j.neuint.2022.105343
216. Tomassini A, Vitalone A, Marini F, Praticò G, Sciubba F, Bevilacqua M, et al. 1H NMR-based urinary metabolic profiling reveals changes in nicotinamide pathway intermediates due to postnatal stress model in rat. J Proteome Res. (2014) 13(12):5848–59. doi: 10.1021/pr500748r
217. Bignon Y, Rinaldi A, Nadour Z, Poindessous V, Nemazanyy I, Lenoir O, et al. Cell stress response impairs de novo NAD+ biosynthesis in the kidney. JCI Insight. (2022) 7(1):e153019. doi: 10.1172/jci.insight.153019
218. Joisten N, Ruas JL, Braidy N, Guillemin GJ, Zimmer P. The kynurenine pathway in chronic diseases: a compensatory mechanism or a driving force? Trends Mol Med. (2021) 27(10):946–54. doi: 10.1016/j.molmed.2021.07.006
219. Xie X, Yu C, Zhou J, Xiao Q, Shen Q, Xiong Z, et al. Nicotinamide mononucleotide ameliorates the depression-like behaviors and is associated with attenuating the disruption of mitochondrial bioenergetics in depressed mice. J Affect Disord. (2020) 263:166–74. doi: 10.1016/j.jad.2019.11.147
220. Schroeder R, Sridharan P, Nguyen L, Loren A, Williams NS, Kettimuthu KP, et al. Maternal P7C3-A20 treatment protects offspring from neuropsychiatric sequelae of prenatal stress. Antioxid Redox Signal. (2021) 35(7):511–30. doi: 10.1089/ars.2020.8227
221. Schroeder R, Nguyen L, Pieper AA, Stevens HE. Maternal treatment with P7C3-A20 protects from impaired maternal care after chronic gestational stress. Behav Brain Res. (2022) 416:113558. doi: 10.1016/j.bbr.2021.113558
222. Bosch-Presegué L, Vaquero A. Sirtuins in stress response: guardians of the genome. Oncogene. (2014) 33(29):3764–75. doi: 10.1038/onc.2013.344
223. Herskovits AZ, Guarente L. SIRT1 In neurodevelopment and brain senescence. Neuron. (2014) 81(3):471–83. doi: 10.1016/j.neuron.2014.01.028
224. Sims CA, Labiner HE, Shah SS, Baur JA. Longevity pathways in stress resistance: targeting NAD and sirtuins to treat the pathophysiology of hemorrhagic shock. Geroscience. (2021) 43(3):1217–28. doi: 10.1007/s11357-020-00311-z
225. Guarente L. Sirtuins as potential targets for metabolic syndrome. Nature. (2006) 444(7121):868–74. doi: 10.1038/nature05486
226. Caron AZ, He X, Mottawea W, Seifert EL, Jardine K, Dewar-Darch D, et al. The SIRT1 deacetylase protects mice against the symptoms of metabolic syndrome. FASEB J. (2014) 28(3):1306–16. doi: 10.1096/fj.13-243568
227. Schenk S, McCurdy CE, Philp A, Chen MZ, Holliday MJ, Bandyopadhyay GK, et al. Sirt1 enhances skeletal muscle insulin sensitivity in mice during caloric restriction. J Clin Invest. (2011) 121(11):4281–8. doi: 10.1172/JCI58554
228. Herskovits AZ, Guarente L. Sirtuin deacetylases in neurodegenerative diseases of aging. Cell Res. (2013) 23(6):746–58. doi: 10.1038/cr.2013.70
229. Converge consortium. Sparse whole-genome sequencing identifies two loci for major depressive disorder. Nature. (2015) 523(7562):588–91. doi: 10.1038/nature14659
230. Sánchez-Hidalgo AC, Muñoz MF, Herrera AJ, Espinosa-Oliva AM, Stowell R, Ayala A, et al. Chronic stress alters the expression levels of longevity-related genes in the rat hippocampus. Neurochem Int. (2016) 97:181–92. doi: 10.1016/j.neuint.2016.04.009
231. Abe-Higuchi N, Uchida S, Yamagata H, Higuchi F, Hobara T, Hara K, et al. Hippocampal sirtuin 1 signaling mediates depression-like behavior. Biol Psychiatry. (2016) 80(11):815–26. doi: 10.1016/j.biopsych.2016.01.009
232. Yu X, Hu Y, Huang W, Ye N, Yan Q, Ni W, et al. Role of AMPK/SIRT1-SIRT3 signaling pathway in affective disorders in unpredictable chronic mild stress mice. Neuropharmacology. (2020) 165:107925. doi: 10.1016/j.neuropharm.2019.107925
233. Duan CM, Zhang JR, Wan TF, Wang Y, Chen HS, Liu L. SRT2104 Attenuates chronic unpredictable mild stress-induced depressive-like behaviors and imbalance between microglial M1 and M2 phenotypes in the mice. Behav Brain Res. (2020) 378:112296. doi: 10.1016/j.bbr.2019.112296
234. Shlomi S, Toledano R, Nitzan K, Shahaf SD, Break EP, Frenkel D, et al. Imbalance in Sirt1 alternative splicing in response to chronic stress during the adolescence period in female mice. Int J Mol Sci. (2022) 23(9):4945. doi: 10.3390/ijms23094945
235. Dang R, Wang M, Li X, Wang H, Liu L, Wu Q, et al. Edaravone ameliorates depressive and anxiety-like behaviors via Sirt1/Nrf2/HO-1/Gpx4 pathway. J Neuroinflammation. (2022) 19(1):41. doi: 10.1186/s12974-022-02400-6
236. Shen J, Li Y, Qu C, Xu L, Sun H, Zhang J. The enriched environment ameliorates chronic unpredictable mild stress-induced depressive-like behaviors and cognitive impairment by activating the SIRT1/miR-134 signaling pathway in hippocampus. J Affect Disord. (2019) 248:81–90. doi: 10.1016/j.jad.2019.01.031
237. Liu SY, Li D, Zeng HY, Kan LY, Zou W, Zhang P, et al. Hydrogen sulfide inhibits chronic unpredictable mild stress-induced depressive-like behavior by upregulation of sirt-1: involvement in suppression of hippocampal endoplasmic Reticulum stress. Int J Neuropsychopharmacol. (2017) 20(11):867–76. doi: 10.1093/ijnp/pyx030
238. Shen J, Xu L, Qu C, Sun H, Zhang J. Resveratrol prevents cognitive deficits induced by chronic unpredictable mild stress: sirt1/miR-134 signalling pathway regulates CREB/BDNF expression in hippocampus in vivo and in vitro. Behav Brain Res. (2018) 349:1–7. doi: 10.1016/j.bbr.2018.04.050
239. Ferland CL, Hawley WR, Puckett RE, Wineberg K, Lubin FD, Dohanich GP, et al. Sirtuin activity in dentate gyrus contributes to chronic stress-induced behavior and extracellular signal-regulated protein kinases 1 and 2 cascade changes in the hippocampus. Biol Psychiatry. (2013) 74(12):927–35. doi: 10.1016/j.biopsych.2013.07.029
240. Kim HD, Hesterman J, Call T, Magazu S, Keeley E, Armenta K, et al. SIRT1 Mediates depression-like behaviors in the nucleus Accumbens. J Neurosci. (2016) 36(32):8441–52. doi: 10.1523/JNEUROSCI.0212-16.2016
241. Liu R, Dang W, Du Y, Zhou Q, Jiao K, Liu Z. SIRT2 Is involved in the modulation of depressive behaviors. Sci Rep. (2015) 5:8415. doi: 10.1038/srep08415
242. Zhang Z, Zhang P, Qi GJ, Jiao FJ, Wang QZ, Yan JG, et al. CDK5-mediated Phosphorylation of Sirt2 contributes to depressive-like behavior induced by social defeat stress. Biochim Biophys Acta Mol Basis Dis. (2018) 1864(2):533–41. doi: 10.1016/j.bbadis.2017.11.012
243. Xie X, Shen Q, Cao L, Chen Y, Ma L, Xiao Q, et al. Depression caused by long-term stress regulates premature aging and is possibly associated with disruption of circadian rhythms in mice. Physiol Behav. (2019) 199:100–10. doi: 10.1016/j.physbeh.2018.11.011
244. Schwarzmann L, Pliquett RU, Simm A, Bartling B. Sex-related differences in human plasma NAD+/NADH levels depend on age. Biosci Rep. (2021) 41(1):BSR20200340. doi: 10.1042/BSR20200340
245. Yang F, Deng X, Yu Y, Luo L, Chen X, Zheng J, et al. Association of human whole blood NAD+ contents with aging. Front Endocrinol (Lausanne). (2022) 13:829658. doi: 10.3389/fendo.2022.829658
246. Breton M, Costemale-Lacoste JF, Li Z, Lafuente-Lafuente C, Belmin J, Mericskay M. Blood NAD levels are reduced in very old patients hospitalized for heart failure. Exp Gerontol. (2020) 139:111051. doi: 10.1016/j.exger.2020.111051
247. Wang W, Li J, Cai L. Research progress of sirtuins in renal and cardiovascular diseases. Curr Opin Nephrol Hypertens. (2021) 30(1):108–14. doi: 10.1097/MNH.0000000000000660
248. Barcena de Arellano ML, Pozdniakova S, Kühl AA, Baczko I, Ladilov Y, Regitz-Zagrosek V. Sex differences in the aging human heart: decreased sirtuins, pro-inflammatory shift and reduced anti-oxidative defense. Aging (Albany NY). (2019) 11(7):1918–33. doi: 10.18632/aging.101881
249. Lei Y, Wang J, Wang D, Li C, Liu B, Fang X, et al. SIRT1 In forebrain excitatory neurons produces sexually dimorphic effects on depression-related behaviors and modulates neuronal excitability and synaptic transmission in the medial prefrontal cortex. Mol Psychiatry. (2020) 25(5):1094–111. doi: 10.1038/s41380-019-0352-1
250. Sasaki Y, Ikeda Y, Miyauchi T, Uchikado Y, Akasaki Y, Ohishi M. Estrogen-SIRT1 axis plays a pivotal role in protecting arteries against menopause-induced senescence and atherosclerosis. J Atheroscler Thromb. (2020) 27(1):47–59. doi: 10.5551/jat.47993
251. Quiñones M, Hernández-Bautista R, Beiroa D, Heras V, Torres-Leal FL, Lam BYH, et al. Sirt3 in POMC neurons controls energy balance in a sex- and diet-dependent manner. Redox Biol. (2021) 41:101945. doi: 10.1016/j.redox.2021.101945
252. Zeng H, He X, Chen JX. A sex-specific role of endothelial sirtuin 3 on blood pressure and diastolic dysfunction in female mice. Int J Mol Sci. (2020) 21(24):9744. doi: 10.3390/ijms21249744
253. Shen H, Holliday M, Sheikh-Hamad D, Li Q, Tong Q, Hamad CD, et al. Sirtuin-3 mediates sex differences in kidney ischemia-reperfusion injury. Transl Res. (2021) 235:15–31. doi: 10.1016/j.trsl.2021.03.015
254. Oliver DK, Intson K, Sargin D, Power SK, McNabb J, Ramsey Aje T. Chronic social isolation exerts opposing sex-specific consequences on serotonin neuronal excitability and behaviour. Neuropharmacology. (2020) 168:108015. doi: 10.1016/j.neuropharm.2020.108015
255. Sachs BD, Ni JR, Caron MG. Sex differences in response to chronic mild stress and congenital serotonin deficiency. Psychoneuroendocrinology. (2014) 40:123–9. doi: 10.1016/j.psyneuen.2013.11.008
256. Rincón-Cortés M, Grace AA. Sex-Dependent effects of stress on immobility behavior and VTA dopamine neuron activity: modulation by ketamine. Int J Neuropsychopharmacol. (2017) 20(10):823–32. doi: 10.1093/ijnp/pyx048
257. Dalla C, Antoniou K, Kokras N, Drossopoulou G, Papathanasiou G, Bekris S, et al. Sex differences in the effects of two stress paradigms on dopaminergic neurotransmission. Physiol Behav. (2008) 93(3):595–605. doi: 10.1016/j.physbeh.2007.10.020
258. Shepard R, Page CE, Coutellier L. Sensitivity of the prefrontal GABAergic system to chronic stress in male and female mice: relevance for sex differences in stress-related disorders. Neuroscience. (2016) 332:1–12. doi: 10.1016/j.neuroscience.2016.06.038
259. Seney ML, Chang LC, Oh H, Wang X, Tseng GC, Lewis DA, et al. The role of genetic sex in affect regulation and expression of GABA-related genes across Species. Front Psychiatry. (2013) 4:104. doi: 10.3389/fpsyt.2013.00104
260. Moench KM, Breach MR, Wellman CL. Prior stress followed by a novel stress challenge results in sex-specific deficits in behavioral flexibility and changes in gene expression in rat medial prefrontal cortex. Horm Behav. (2020) 117:104615. doi: 10.1016/j.yhbeh.2019.104615
261. Nahvi RJ, Sabban EL. Sex differences in the neuropeptide Y system and implications for stress related disorders. Biomolecules. (2020) 10(9):1248. doi: 10.3390/biom10091248
262. Ledoux VA, Smejkalova T, May RM, Cooke BM, Woolley CS. Estradiol facilitates the release of neuropeptide Y to suppress hippocampus-dependent seizures. J Neurosci. (2009) 29:1457–68. doi: 10.1523/jneurosci.4688-08.2009
263. Velíšková J, Iacobas D, Iacobas S, Sidyelyeva G, Chachua T, Velíšek L. Oestradiol regulates neuropeptide Y release and gene coupling with the GABAergic and glutamatergic synapses in the adult female rat dentate gyrus. J Neuroendocrinol. (2015) 27(12):911–20. doi: 10.1111/jne.12332
264. Hilke S, Holm L, Man K, Hökfelt T, Theodorsson E. Rapid change of neuropeptide Y levels and gene-expression in the brain of ovariectomized mice after administration of 17beta-estradiol. Neuropeptides. (2009) 43(4):327–32. doi: 10.1016/j.npep.2009.04.005
265. Karl T, Duffy L, Herzog H. Behavioural profile of a new mouse model for NPY deficiency. Eur J Neurosci. (2008) 28(1):173–80. doi: 10.1111/j.1460-9568.2008.06306.x
266. Painsipp E, Herzog H, Sperk G, Holzer P. Sex-dependent control of murine emotional-affective behaviour in health and colitis by peptide YY and neuropeptide Y. Br J Pharmacol. (2011) 163(6):1302–14. doi: 10.1111/j.1476-5381.2011.01326.x
267. Nahvi RJ, Nwokafor C, Serova LI, Sabban EL. Single prolonged stress as a prospective model for posttraumatic stress disorder in females. Front Behav Neurosci. (2019) 13:17. doi: 10.3389/fnbeh.2019.00017
268. Taniguchi S, Yanase T, Kurimoto F, Takayanagi R, Haji M, Kurose S, et al. Age-related increase in neuropeptide Y-like immunoreactivity in cerebrospinal fluid in women. Fukuoka Igaku Zasshi. (1994) 85:361–5.7883275
269. Escobar CM, Krajewski SJ, Sandoval-Guzmán T, Voytko ML, Rance NE. Neuropeptide Y gene expression is increased in the hypothalamus of older women. J Clin Endocrinol Metab. (2004) 89(5):2338–43. doi: 10.1210/jc.2003-031899
270. Gruenewald DA, Naai MA, Marck BT, Matsumoto AM. Age-related decrease in neuropeptide-Y gene expression in the arcuate nucleus of the male rat brain is independent of testicular feedback. Endocrinology. (1994) 134(6):2383–9. doi: 10.1210/endo.134.6.8194464
271. Sahu A, Kalra PS, Crowley WR, Kalra SP. Evidence that hypothalamic neuropeptide Y secretion decreases in aged male rats: implications for reproductive aging. Endocrinology. (1988) 122(5):2199–203. doi: 10.1210/endo-122-5-2199
272. Sahu A, Kalra SP, Crowley WR, Kalra PS. Aging in male rats modifies castration and testosterone-induced neuropeptide Y response in various microdissected brain nuclei. Brain Res. (1990) 515(1–2):287–91. doi: 10.1016/0006-8993(90)90608-e
273. Yam KY, Ruigrok SR, Ziko I, De Luca SN, Lucassen PJ, Spencer SJ, et al. Ghrelin and hypothalamic NPY/AgRP expression in mice are affected by chronic early-life stress exposure in a sex-specific manner. Psychoneuroendocrinology. (2017) 86:73–7. doi: 10.1016/j.psyneuen.2017.09.006
274. Toniazzo AP, Arcego DM, Lazzaretti C, Lampert C, Weis SN, Proto-Siqueira R, et al. Sex-specific effects of prepubertal stress and high-fat diet on leptin signaling in rats. Nutrition. (2018) 50:18–25. doi: 10.1016/j.nut.2017.10.018
275. Manni L, Di Fausto V, Chaldakov GN, Aloe L. Brain leptin and nerve growth factor are differently affected by stress in male and female mice: possible neuroendocrine and cardio-metabolic implications. Neurosci Lett. (2007) 426(1):39–44. doi: 10.1016/j.neulet.2007.08.015
276. Wallace AM, McMahon AD, Packard CJ, Kelly A, Shepherd J, Gaw A, et al. Plasma leptin and the risk of cardiovascular disease in the west of Scotland coronary prevention study (WOSCOPS). Circulation. (2001) 104:3052–6. doi: 10.1161/hc5001.101061
277. Wolk R, Berger P, Lennon RJ, Brilakis ES, Johnson BD, Somers VK. Plasma leptin and prognosis in patients with established coro- nary atherosclerosis. J Am Coll Cardiol. (2004) 44:1819–24. doi: 10.1016/j.jacc.2004.07.050
278. Charles LE, Burchfiel CM, Sarkisian K, Li S, Miller DB, Gu JK, et al. Leptin, adiponectin, and heart rate variability among police officers. Am J Hum Biol. (2015) 27(2):184–91. doi: 10.1002/ajhb.22636
279. Lew J, Sanghavi M, Ayers CR, McGuire DK, Omland T, Atzler D, et al. Sex-Based differences in cardiometabolic biomarkers. Circulation. (2017) 135(6):544–55. doi: 10.1161/CIRCULATIONAHA.116.023005
280. Hellström L, Wahrenberg H, Hruska K, Reynisdottir S, Arner P. Mechanisms behind gender differences in circulating leptin levels. J Intern Med. (2000) 247(4):457–62. doi: 10.1046/j.1365-2796.2000.00678.x
281. González M, del Mar Bibiloni M, Pons A, Llompart I, Tur JA. Inflammatory markers and metabolic syndrome among adolescents. Eur J Clin Nutr. (2012) 66(10):1141–5. doi: 10.1038/ejcn.2012.112
282. Song HJ, Oh S, Quan S, Ryu OH, Jeong JY, Hong KS, et al. Gender differences in adiponectin levels and body composition in older adults: hallym aging study. BMC Geriatr. (2014) 14:8. doi: 10.1186/1471-2318-14-8
283. Liu J, Guo M, Zhang D, Cheng SY, Liu M, Ding J, et al. Adiponectin is critical in determining susceptibility to depressive behaviors and has antidepressant-like activity. Proc Natl Acad Sci U S A. (2012) 109(30):12248–53. doi: 10.1073/pnas.1202835109
284. Zhou Z, Liu C, Xu S, Wang J, Guo F, Duan S, et al. Metabolism regulator adiponectin prevents cardiac remodeling and ventricular arrhythmias via sympathetic modulation in a myocardial infarction model. Basic Res Cardiol. (2022) 117(1):34. doi: 10.1007/s00395-022-00939-2
285. Vuong E, Mhlongo S, Chirwa E, Lombard C, Peer N, Hemmings SM, et al. Serum adiponectin-levels are predictive of probable posttraumatic stress disorder in women. Neurobiol Stress. (2022) 20:100477. doi: 10.1016/j.ynstr.2022.100477
286. Capllonch-Amer G, Sbert-Roig M, Galmés-Pascual BM, Proenza AM, Lladó I, Gianotti M, et al. Estradiol stimulates mitochondrial biogenesis and adiponectin expression in skeletal muscle. J Endocrinol. (2014) 221(3):391–403. doi: 10.1530/JOE-14-0008
287. Capllonch-Amer G, Lladó I, Proenza AM, García-Palmer FJ, Gianotti M. Opposite effects of 17-β estradiol and testosterone on mitochondrial biogenesis and adiponectin synthesis in white adipocytes. J Mol Endocrinol. (2014) 52(2):203–14. doi: 10.1530/JME-13-0201
288. Siegel C, McCullough LD. NAD+ depletion or PAR polymer formation: which plays the role of executioner in ischaemic cell death? Acta Physiol (Oxf). (2011) 203(1):225–34. doi: 10.1111/j.1748-1716.2010.02229.x
289. Liu F, Li Z, Li J, Siegel C, Yuan R, McCullough LD. Sex differences in caspase activation after stroke. Stroke. (2009) 40(5):1842–8. doi: 10.1161/STROKEAHA.108.538686
290. Du L, Bayir H, Lai Y, Zhang X, Kochanek PM, Watkins SC, et al. Innate gender-based proclivity in response to cytotoxicity and programmed cell death pathway. J Biol Chem. (2004) 279(37):38563–70. doi: 10.1074/jbc.M405461200
291. Renolleau S, Fau S, Goyenvalle C, Joly LM, Chauvier D, Jacotot E, et al. Specific caspase inhibitor Q-VD-OPh prevents neonatal stroke in P7 rat: a role for gender. J Neurochem. (2007) 100(4):1062–71. doi: 10.1111/j.1471-4159.2006.04269.x
292. Renolleau S, Fau S, Charriaut-Marlangue C. Gender-related differences in apoptotic pathways after neonatal cerebral ischemia. Neuroscientist. (2008) 14(1):46–52. doi: 10.1177/1073858407308889
293. Hagberg H, Wilson MA, Matsushita H, Zhu C, Lange M, Gustavsson M, et al. PARP-1 gene disruption in mice preferentially protects males from perinatal brain injury. J Neurochem. (2004) 90(5):1068–75. doi: 10.1111/j.1471-4159.2004.02547.x
294. Siegel CS, McCullough LD. NAD+ and nicotinamide: sex differences in cerebral ischemia. Neuroscience. (2013) 237:223–31. doi: 10.1016/j.neuroscience.2013.01.068
295. Li J, McCullough LD. Sex differences in minocycline-induced neuroprotection after experimental stroke. J Cereb Blood Flow Metab. (2009) 29(4):670–4. doi: 10.1038/jcbfm.2009.3
296. Lu Y, Zhang Y, Zhao X, Shang C, Xiang M, Li L, et al. Microbiota-derived short-chain fatty acids: implications for cardiovascular and metabolic disease. Front Cardiovasc Med. (2022) 9:900381. doi: 10.3389/fcvm.2022.900381
297. Dubinski P, Czarzasta K, Cudnoch-Jedrzejewska A. The influence of gut Microbiota on the cardiovascular system under conditions of obesity and chronic stress. Curr Hypertens Rep. (2021) 23(5):31. doi: 10.1007/s11906-021-01144-7
298. Sarkar A, Yoo JY, Valeria Ozorio Dutra S, Morgan KH, Groer M. The association between early-life gut Microbiota and long-term health and diseases. J Clin Med. (2021) 10(3):459. doi: 10.3390/jcm10030459
299. Murray E, Sharma R, Smith KB, Mar KD, Barve R, Lukasik M, et al. Probiotic consumption during puberty mitigates LPS-induced immune responses and protects against stress-induced depression- and anxiety-like behaviors in adulthood in a sex-specific manner. Brain Behav Immun. (2019) 81:198–212. doi: 10.1016/j.bbi.2019.06.016
300. Murray E, Smith KB, Stoby KS, Thomas BJ, Swenson MJ, Arber LA, et al. Pubertal probiotic blocks LPS-induced anxiety and the associated neurochemical and microbial outcomes, in a sex dependent manner. Psychoneuroendocrinology. (2020) 112:104481. doi: 10.1016/j.psyneuen.2019.104481
301. Rizzetto L, Fava F, Tuohy KM, Selmi C. Connecting the immune system, systemic chronic inflammation and the gut microbiome: the role of sex. J Autoimmun. (2018) 92:12–34. doi: 10.1016/j.jaut.2018.05.008
302. Herselman MF, Bailey S, Bobrovskaya L. The effects of stress and diet on the “brain-gut” and “gut-brain” pathways in animal models of stress and depression. Int J Mol Sci. (2022) 23(4):2013. doi: 10.3390/ijms23042013
303. Audet MC. Stress-induced disturbances along the gut microbiota-immune-brain axis and implications for mental health: does sex matter? Front Neuroendocrinol. (2019) 54:100772. doi: 10.1016/j.yfrne.2019.100772
304. Jaggar M, Rea K, Spichak S, Dinan TG, Cryan JF. You've got male: sex and the microbiota-gut-brain axis across the lifespan. Front Neuroendocrinol. (2020) 56:100815. doi: 10.1016/j.yfrne.2019.100815
305. Baker JM, Al-Nakkash L, Herbst-Kralovetz MM. Estrogen-gut microbiome axis: physiological and clinical implications. Maturitas. (2017) 103:45–53. doi: 10.1016/j.maturitas.2017.06.025
306. Bugiardini R, Manfrini O, Cenko E. Female sex as a biological variable: a review on younger patients with acute coronary syndrome. Trends Cardiovasc Med. (2019) 29(1):50–5. doi: 10.1016/j.tcm.2018.06.002
307. Gupta A, Wang Y, Spertus JA, Geda M, Lorenze N, Nkonde-Price C, et al. Trends in acute myocardial infarction in young patients and differences by sex and race, 2001 to 2010. J Am Coll Cardiol. (2014) 64(4):337–45. doi: 10.1016/j.jacc.2014.04.054
308. Pancholy SB, Shantha GP, Patel T, Cheskin LJ. Sex differences in short-term and long-term all-cause mortality among patients with ST-segment elevation myocardial infarction treated by primary percutaneous intervention: a meta-analysis. JAMA Intern Med. (2014) 174(11):1822–30. doi: 10.1001/jamainternmed.2014.4762
309. Peters SAE, Muntner P, Woodward M. Sex differences in the prevalence of, and trends in, cardiovascular risk factors, treatment, and control in the United States, 2001 to 2016. Circulation. (2019) 139(8):1025–35. doi: 10.1161/CIRCULATIONAHA.118.035550
310. Haider A, Bengs S, Luu J, Osto E, Siller-Matula JM, Muka T, et al. Sex and gender in cardiovascular medicine: presentation and outcomes of acute coronary syndrome. Eur Heart J. (2020) 41(13):1328–36. doi: 10.1093/eurheartj/ehz898
311. Stehli J, Duffy SJ, Burgess S, Kuhn L, Gulati M, Chow C, et al. Sex disparities in myocardial infarction: biology or bias? Heart Lung Circ. (2021) 30(1):18–26. doi: 10.1016/j.hlc.2020.06.025
312. Lawton JS. Sex and gender differences in coronary artery disease. Semin Thorac Cardiovasc Surg. (2011) 23(2):126–30. doi: 10.1053/j.semtcvs.2011.07.006
313. Agdamag AC, Patel H, Chandra S, Rao A, Suboc TM, Marinescu K, et al. Sex differences in takotsubo syndrome: a narrative review. J Womens Health (Larchmt). (2020) 29(8):1122–30. doi: 10.1089/jwh.2019.7741
314. Pattisapu VK, Hao H, Liu Y, Nguyen TT, Hoang A, Bairey Merz CN, et al. Sex- and age-based temporal trends in takotsubo syndrome incidence in the United States. J Am Heart Assoc. (2021) 10(20):e019583. doi: 10.1161/JAHA.120.019583
315. Aoki T, Fukumoto Y, Yasuda S, Sakata Y, Ito K, Takahashi J, et al. The great east Japan earthquake disaster and cardiovascular diseases. Eur Heart J. (2012) 33(22):2796–803. doi: 10.1093/eurheartj/ehs288
316. Esler M, Jennings G, Lambert G. Measurement of overall and cardiac norepinephrine release into plasma during cognitive challenge. Psychoneuroendocrinology. (1989) 14:477–81. doi: 10.1016/0306-4530(89)90047-4
317. Wallin BG, Esler M, Dorward P, Eisenhofer G, Ferrier C, Westerman R, et al. Simultaneous measurements of cardiac noradrenaline spillover and sympathetic outflow to skeletal muscle in humans. J Physiol. (1992) 453:45–58. doi: 10.1113/jphysiol.1992.sp019217
318. Fox BM, Becker BK, Loria AS, Hyndman KA, Jin C, Clark H, et al. Acute pressor response to psychosocial stress is dependent on endothelium-derived endothelin-1. J Am Heart Assoc. (2018) 7(4):e007863. doi: 10.1161/JAHA.117.007863
319. Mittleman MA, Maclure M, Sherwood JB, Mulry RP, Tofler GH, Jacobs SC, et al. Triggering of acute myocardial infarction onset by episodes of anger. Determinants of myocardial infarction onset study investigators. Circulation. (1995) 92(7):1720–5. doi: 10.1161/01.cir.92.7.1720
320. Von Känel R, Mills PJ, Fainman C, Dimsdale JE. Effects of psychological stress and psychiatric disorders on blood coagulation and fibrinolysis: a biobehavioral pathway to coronary artery disease? Psychosom Med. (2001) 63:531–44. doi: 10.1097/00006842-200107000-00003
321. Koudouovoh-Tripp P, Hüfner K, Egeter J, Kandler C, Giesinger JM, Sopper S, et al. Stress enhances proinflammatory platelet activity: the impact of acute and chronic mental stress. J Neuroimmune Pharmacol. (2021) 16(2):500–12. doi: 10.1007/s11481-020-09945-4
322. Von Kanel R, Dimsdale JE. Effects of sympathetic activation by adrenergic infusions on hemostasis in vivo. Eur J Haematol. (2000) 65:357–69. doi: 10.1034/j.1600-0609.2000.065006357.x
323. Steptoe A, Magid K, Edwards S, Brydon L, Hong Y, Erusalimsky J. The influence of psychological stress and socioeconomic status on platelet activation in men. Atherosclerosis. (2003) 168:57–63. doi: 10.1016/S0021-9150(02)00453-7
324. Wallén NH, Goodall AH, Li N, Hjemdahl P. Activation of haemostasis by exercise, mental stress and Adrenaline: effects on platelet sensitivity to thrombin and thrombin generation. Clin Sci (Lond). (1999) 97(1):27–35. doi: 10.1042/CS19990013
325. Wallén NH, Held C, Rehnqvist N, Hjemdahl P. Effects of mental and physical stress on platelet function in patients with stable angina pectoris and healthy controls. Eur Heart J. (1997) 18(5):807–15. doi: 10.1093/oxfordjournals.eurheartj.a015346
326. Grignani G, Pacchiarini L, Zucchella M, Tacconi F, Canevari A, Soffiantino F, et al. Effect of mental stress on platelet function in normal subjects and in patients with coronary artery disease. Haemostasis. (1992) 22(3):138–46. doi: 10.1159/000216310
327. Von Känel R, Dimsdale JE, Ziegler MG, Mills PJ, Patterson TL, Lee SK, et al. Effect of acute psychological stress on the hypercoagulable state in subjects (spousal caregivers of patients with Alzheimer’s disease) with coronary or cerebrovascular disease and/or systemic hypertension. Am J Cardiol. (2001) 87:1405–8. doi: 10.1016/S0002-9149(01)01564-8
328. Canevari A, Tacconi F, Zucchella M, Pacchiarini L, So antino F, Grignani G. Antithrombin III biological activity and emotional stress in patients with coronary artery disease. Haematologica. (1992) 77:180–2.1398305
329. Palermo A, Bertalero P, Pizza N, Amelotti R, Libretti A. Decreased fibrinolytic response to adrenergic stimulation in hypertensive patients. J Hypertens Suppl. (1989) 7(6):S162–3. doi: 10.1097/00004872-198900076-00077
330. Jern C, Eriksson E, Tengborn L, Risberg B, Wadenvik H, Jern S. Changes of plasma coagulation and fibrinolysis in response to mental stress. Thromb Haemost. (1989) 62(2):767–71. doi: 10.1055/s-0038-1646899
331. Jern C, Manhem K, Eriksson E, Tengborn L, Risberg B, Jern S. Hemostatic responses to mental stress during the menstrual cycle. Thromb Haemost. (1991) 66(5):614–8. doi: 10.1055/s-0038-1646469
332. Jilma B, Cvitko T, Winter-Fabry A, Petroczi K, Quehenberger P, Blann AD. High dose dexamethasone increases circulating P-selectin and von willebrand factor levels in healthy men. Thromb Haemost. (2005) 94(4):797–801. doi: 10.1160/TH04-10-0652
333. Rosenfeld BA, Faraday N, Campbell D, Dise K, Bell W, Goldschmidt P. Hemostatic effects of stress hormone infusion. Anesthesiology. (1994) 81(5):1116–26. doi: 10.1097/00000542-199411000-00005
334. Aschbacher K, von Känel R, Mills PJ, Roepke SK, Hong S, Dimsdale JE, et al. Longitudinal platelet reactivity to acute psychological stress among older men and women. Stress. (2009) 12(5):426–33. doi: 10.1080/10253890802574993
335. Ali-Saleh M, Sarig G, Ablin JN, Brenner B, Jacob G. Inhalation of a short-acting β2-adrenoreceptor agonist induces a hypercoagulable state in healthy subjects. PLoS One. (2016) 11(7):e0158652. doi: 10.1371/journal.pone.0158652
336. Hoppener MR, Kraaijenhagen RA, Hutten BA, Büller HR, Peters RJ, Levi M. Beta-receptor blockade decreases elevated plasma levels of factor VIII:c in patients with deep vein thrombosis. J Thromb Haemost. (2004) 2(8):1316–20. doi: 10.1111/j.1538-7836.2004.00851.x
337. Hao Z, Jiang X, Sharafeih R, Shen S, Hand AR, Cone RE, et al. Stimulated release of tissue plasminogen activator from artery wall sympathetic nerves: implications for stress-associated wall damage. Stress. (2005) 8(2):141–9. doi: 10.1080/10253890500168098
338. Hijmering ML, Stroes ES, Olijhoek J, Hutten BA, Blankestijn PJ, Rabelink TJ. Sympathetic activation markedly reduces endothelium-dependent, flow-mediated vasodilation. J Am Coll Cardiol. (2002) 39(4):683–8. doi: 10.1016/s0735-1097(01)01786-7
339. Eriksson M, Johansson K, Sarabi M, Lind L. Mental stress impairs endothelial vasodilatory function by a beta-adrenergic mechanism. Endothelium. (2007) 14(3):151–6. doi: 10.1080/10623320701421420
340. Slavish DC, Graham-Engeland JE, Smyth JM, Engeland CG. Salivary markers of inflammation in response to acute stress. Brain Behav Immun. (2015) 44:253–69. doi: 10.1016/j.bbi.2014.08.008
341. Kuebler U, Zuccarella-Hackl C, Arpagaus A, Wolf JM, Farahmand F, von Känel R, et al. Stress-induced modulation of NF-κB activation, inflammation-associated gene expression, and cytokine levels in blood of healthy men. Brain Behav Immun. (2015) 46:87–95. doi: 10.1016/j.bbi.2014.12.024
342. Yamauchi H, Yoshihisa A, Iwaya S, Owada T, Sato T, Suzuki S, et al. Clinical features of patients with decompensated heart failure after the great east Japan earthquake. Am J Cardiol. (2013) 112(1):94–9. doi: 10.1016/j.amjcard.2013.02.057
343. Wilbert-Lampen U, Nickel T, Leistner D, Güthlin D, Matis T, Völker C, et al. Modified serum profiles of inflammatory and vasoconstrictive factors in patients with emotional stress-induced acute coronary syndrome during world cup soccer 2006. J Am Coll Cardiol. (2010) 55(7):637–42. doi: 10.1016/j.jacc.2009.07.073
344. Cohen M, Granger S, Fuller-Thomson E. The association between bereavement and biomarkers of inflammation. Behav Med. (2015) 41(2):49–59. doi: 10.1080/08964289.2013.866539
345. Paine NJ, Bosch JA, Van Zanten JJ. Inflammation and vascular responses to acute mental stress: implications for the triggering of myocardial infarction. Curr Pharm Des. (2012) 18(11):1494–501. doi: 10.2174/138161212799504713
346. von Känel R, Kudielka BM, Hanebuth D, Preckel D, Fischer JE. Different contribution of interleukin-6 and cortisol activity to total plasma fibrin concentration and to acute mental stress-induced fibrin formation. Clin Sci (Lond). (2005) 109(1):61–7. doi: 10.1042/CS20040359
347. Kop WJ, Weissman NJ, Zhu J, Bonsall RW, Doyle M, Stretch MR, et al. Effects of acute mental stress and exercise on inflammatory markers in patients with coronary artery disease and healthy controls. Am J Cardiol. (2008) 101(6):767–73. doi: 10.1016/j.amjcard.2007.11.006
348. Poitras VJ, Pyke KE. The impact of acute mental stress on vascular endothelial function: evidence, mechanisms and importance. Int J Psychophysiol. (2013) 88(2):124–35. doi: 10.1016/j.ijpsycho.2013.03.019
349. Cardillo C, Kilcoyne CM, Quyyumi AA, Cannon RO 3rd, Panza JA. Role of nitric oxide in the vasodilator response to mental stress in normal subjects. Am J Cardiol. (1997) 80(8):1070–4. doi: 10.1016/s0002-9149(97)00605-x
350. Broadley AJ, Korszun A, Abdelaal E, Moskvina V, Jones CJ, Nash GB, et al. Inhibition of cortisol production with metyrapone prevents mental stress-induced endothelial dysfunction and baroreflex impairment. J Am Coll Cardiol. (2005) 46(2):344–50. doi: 10.1016/j.jacc.2005.03.068
351. Green DJ, Jones H, Thijssen D, Cable NT, Atkinson G. Flow-mediated dilation and cardiovascular event prediction: does nitric oxide matter? Hypertension. (2011) 57(3):363–9. doi: 10.1161/HYPERTENSIONAHA.110.167015
352. Sherwood A, Johnson K, Blumenthal JA, Hinderliter AL. Endothelial function and hemodynamic responses during mental stress. Psychosom Med. (1999) 61(3):365–70. doi: 10.1097/00006842-199905000-00017
353. Rogers KM, Bonar CA, Estrella JL, Yang S. Inhibitory effect of glucocorticoid on coronary artery endothelial function. Am J Physiol Heart Circ Physiol. (2002) 283(5):H1922–8. doi: 10.1152/ajpheart.00364.2002
354. Wallerath T, Witte K, Schäfer SC, Schwarz PM, Prellwitz W, Wohlfart P, et al. Down-regulation of the expression of endothelial NO synthase is likely to contribute to glucocorticoid-mediated hypertension. Proc Natl Acad Sci U S A. (1999) 96(23):13357–62. doi: 10.1073/pnas.96.23.13357
355. Liu Y, Mladinov D, Pietrusz JL, Usa K, Liang M. Glucocorticoid response elements and 11 -hydroxysteroid dehydrogenases in the regulation of endothelial nitric oxide synthase expression. Cardiovasc Res. (2008) 81:140–7. doi: 10.1093/cvr/cvn231
356. Kanse SM, Takahashi K, Warren JB, Ghatei M, Bloom SR. Glucocorticoids induce endothelin release from vascular smooth muscle cells but not endothelial cells. Eur J Pharm. (1991) 199:99–101. doi: 10.1016/0014-2999(91)90641-3
357. Harris KF, Matthews KA. Interactions between autonomic nervous system activity and endothelial function: a model for the development of cardiovascular disease. Psychosom Med. (2004) 66(2):153–64. doi: 10.1097/01.psy.0000116719.95524.e2
358. Mangiafico RA, Malatino LS, Attinà T, Messina R, Fiore CE. Exaggerated endothelin release in response to acute mental stress in patients with intermittent claudication. Angiology. (2002) 53(4):383–90. doi: 10.1177/000331970205300403
359. Treiber FA, Kapuku GK, Davis H, Pollock JS, Pollock DM. Plasma endothelin-1 release during acute stress: role of ethnicity and sex. Psychosom Med. (2002) 64:707–13.12271101
360. Spieker LE, Hürlimann D, Ruschitzka F, Corti R, Enseleit F, Shaw S, et al. Mental stress induces prolonged endothelial dysfunction via endothelin-A receptors. Circulation. (2002) 105(24):2817–20. doi: 10.1161/01.cir.0000021598.15895.34
361. Vaccarino V, Almuwaqqat Z, Kim JH, Hammadah M, Shah AJ, Ko YA, et al. Association of mental stress-induced myocardial ischemia with cardiovascular events in patients with coronary heart disease. JAMA. (2021) 326(18):1818–28. doi: 10.1001/jama.2021.17649
362. Lima BB, Hammadah M, Kim JH, Uphoff I, Shah A, Levantsevych O, et al. Association of transient endothelial dysfunction induced by mental stress with Major adverse cardiovascular events in men and women with coronary artery disease. JAMA Cardiol. (2019) 4(10):988–96. doi: 10.1001/jamacardio.2019.3252
363. Widmer RJ, Prasad M, Gomaa M, Sara JDS, Reriani MK, Lerman LO, et al. Vascular reactivity to mental stress is associated with poor cardiovascular disease outcomes in females following acute coronary syndrome. Coron Artery Dis. (2020) 31(3):300–5. doi: 10.1097/MCA.0000000000000831
364. Burger IA, Lohmann C, Messerli M, Bengs S, Becker A, Maredziak M, et al. Age- and sex-dependent changes in sympathetic activity of the left ventricular apex assessed by 18F-DOPA PET imaging. PLoS One. (2018) 13(8):e0202302. doi: 10.1371/journal.pone.0202302
365. Martin EA, Tan SL, MacBride LR, Lavi S, Lerman LO, Lerman A. Sex differences in vascular and endothelial responses to acute mental stress. Clin Auton Res. (2008) 18(6):339–45. doi: 10.1007/s10286-008-0497-5
366. Mehta PK, Hermel M, Nelson MD, Cook-Wiens G, Martin EA, Alkhoder AA, et al. Mental stress peripheral vascular reactivity is elevated in women with coronary vascular dysfunction: results from the NHLBI-sponsored cardiac autonomic nervous system (CANS) study. Int J Cardiol. (2018) 251:8–13. doi: 10.1016/j.ijcard.2017.10.061
367. Davies KJ. Adaptive homeostasis. Mol Aspects Med. (2016) 49:1–7. doi: 10.1016/j.mam.2016.04.007
368. Lomeli N, Bota DA, Davies KJA. Diminished stress resistance and defective adaptive homeostasis in age-related diseases. Clin Sci (Lond). (2017) 131(21):2573–99. doi: 10.1042/CS20160982
369. McEwen BS. Stress, adaptation, and disease. Allostasis and allostatic load. Ann N Y Acad Sci. (1998) 840:33–44. doi: 10.1111/j.1749-6632.1998.tb09546.x
370. Stoney CM, Davis MC, Matthews KA. Sex differences in physiological responses to stress and in coronary heart disease: a causal link? Psychophysiology. (1987) 24(2):127–31. doi: 10.1111/j.1469-8986.1987.tb00264.x
371. Pomatto LCD, Wong S, Tower J, Davies KJA. Sexual dimorphism in oxidant-induced adaptive homeostasis in multiple wild-type D. Melanogaster strains. Arch Biochem Biophys. (2017) 636:57–70. doi: 10.1016/j.abb.2017.10.021
372. Pomatto LCD, Tower J, Davies KJA. Sexual dimorphism and aging differentially regulate adaptive homeostasis. J Gerontol A Biol Sci Med Sci. (2018) 73(2):141–9. doi: 10.1093/gerona/glx083
373. Moshier SJ, Hearon BA, Calkins AW, Szuhany KL, Utschiq AC, Smits JAJ, et al. Clarifying the link between distress intolerance and exercise: elevated anxiety sensitivity predicts less vigorous exercise. Cognit Ther Res. (2013) 37(3):476–82. doi: 10.1007/s10608-012-9489-9
374. Prior A, Fenger-Grøn M, Larsen KK, Larsen FB, Robinson KM, Nielsen MG, et al. The association between perceived stress and mortality among people with multimorbidity: a prospective population-based cohort study. Am J Epidemiol. (2016) 184(3):199–210. doi: 10.1093/aje/kwv324
375. Altemus M, Sarvaiya N, Neill Epperson C. Sex differences in anxiety and depression clinical perspectives. Front Neuroendocrinol. (2014) 35(3):320–30. doi: 10.1016/j.yfrne.2014.05.004
376. Bale TL, Epperson CN. Sex differences and stress across the lifespan. Nat Neurosci. (2015) 18(10):1413–20. doi: 10.1038/nn.4112
377. Soares CN, Zitek B. Reproductive hormone sensitivity and risk for depression across the female life cycle: a continuum of vulnerability? J Psychiatry Neurosci. (2008) 33(4):331–43.18592034
378. Zuloaga DG, Heck AL, De Guzman RM, Handa RJ. Roles for androgens in mediating the sex differences of neuroendocrine and behavioral stress responses. Biol Sex Differ. (2020) 11(1):44. doi: 10.1186/s13293-020-00319-2
379. Lund TD, Rovis T, Chung WC, Handa RJ. Novel actions of estrogen receptor-beta on anxiety-related behaviors. Endocrinology. (2005) 146(2):797–807. doi: 10.1210/en.2004-1158
380. Lund TD, Hinds LR, Handa RJ. The androgen 5alpha-dihydrotestosterone and its metabolite 5alpha-androstan-3beta, 17beta-diol inhibit the hypothalamo-pituitary-adrenal response to stress by acting through estrogen receptor beta-expressing neurons in the hypothalamus. J Neurosci. (2006) 26:1448–56. doi: 10.1523/JNEUROSCI.3777-05.2006
381. Weiser MJ, Handa RJ. Estrogen impairs glucocorticoid dependent negative feedback on the hypothalamic-pituitary-adrenal axis via estrogen receptor alpha within the hypothalamus. Neuroscience. (2009) 159(2):883–95. doi: 10.1016/j.neuroscience.2008
382. Borrow AP, Handa RJ. Estrogen receptors modulation of anxiety-like behavior. Vitam Horm. (2017) 103:27–52. doi: 10.1016/bs.vh.2016.08.004
383. Byrnes EM, Casey K, Carini LM, Bridges RS. Reproductive experience alters neural and behavioural responses to acute oestrogen receptor α activation. J Neuroendocrinol. (2013) 25(12):1280–9. doi: 10.1111/jne.12113
384. Byrnes EM, Casey K, Bridges RS. Reproductive experience modifies the effects of estrogen receptor alpha activity on anxiety-like behavior and corticotropin releasing hormone mRNA expression. Horm Behav. (2012) 61(1):44–9. doi: 10.1016/j.yhbeh.2011.10.001
385. Imwalle DB, Scordalakes EM, Rissman EF. Estrogen receptor alpha influences socially motivated behaviors. Horm Behav. (2002) 42(4):484–91. doi: 10.1006/hbeh.2002.1837
386. Krezel W, Dupont S, Krust A, Chambon P, Chapman PF. Increased anxiety and synaptic plasticity in estrogen receptor beta -deficient mice. Proc Natl Acad Sci U S A. (2001) 98(21):12278–82. doi: 10.1073/pnas.221451898
387. Comings DE, Muhleman D, Johnson P, MacMurray JP. Potential role of the estrogen receptor gene (ESR1) in anxiety. Mol Psychiatry. (1999) 4(4):374–7. doi: 10.1038/sj.mp.4000503
388. Ryan J, Scali J, Carrière I, Peres K, Rouaud O, Scarabin P-Y, et al. Oestrogen receptor polymorphisms and late-life depression. Br J Psychiatry. (2011) 199:126–31. doi: 10.1192/bjp.bp.111.091751
389. Ryan J, Ancelin ML. Polymorphisms of estrogen receptors and risk of depression: therapeutic implications. Drugs. (2012) 72(13):1725–38. doi: 10.2165/11635960-000000000-00000
390. Imhof JT, Coelho ZM, Schmitt ML, Morato GS, Carobrez AP. Influence of gender and age on performance of rats in the elevated plus maze apparatus. Behav Brain Res. (1993) 56(2):177–80. doi: 10.1016/0166-4328(93)90036-p
391. Zimmerberg B, Farley MJ. Sex differences in anxiety behavior in rats: role of gonadal hormones. Physiol Behav. (1993) 54(6):1119–24. doi: 10.1016/0031-9384(93)90335-d
392. Steenbergen HL, Heinsbroek RP, Van Hest A, Van de Poll NE. Sex-dependent effects of inescapable shock administration on shuttlebox-escape performance and elevated plus-maze behavior. Physiol Behav. (1990) 48(4):571–6. doi: 10.1016/0031-9384(90)90302-k
393. Barros HM, Ferigolo M. Ethopharmacology of imipramine in the forced-swimming test: gender differences. Neurosci Biobehav Rev. (1998) 23(2):279–86. doi: 10.1016/s0149-7634(98)00029-3
394. Kokras N, Dalla C. Sex differences in animal models of psychiatric disorders. Br J Pharmacol. (2014) 171(20):4595–619. doi: 10.1111/bph.12710
395. Lu Y, Zhang J, Zhang L, Dang S, Su Q, Zhang H, et al. Hippocampal acetylation may improve prenatal-stress-induced depression-like behavior of male offspring rats through regulating AMPARs expression. Neurochem Res. (2017) 42(12):3456–64. doi: 10.1007/s11064-017-2393-7
396. Paré WP, Redei E. Sex differences and stress response of WKY rats. Physiol Behav. (1993) 54(6):1179–85. doi: 10.1016/0031-9384(93)90345-g
397. Võikar V, Kõks S, Vasar E, Rauvala H. Strain and gender differences in the behavior of mouse lines commonly used in transgenic studies. Physiol Behav. (2001) 72(1–2):271–81. doi: 10.1016/s0031-9384(00)00405-4
398. Yau SY, Lee TH, Formolo DA, Lee WL, Li LC, Siu PM, et al. Effects of maternal voluntary wheel running during pregnancy on adult hippocampal neurogenesis, temporal order memory, and depression-like behavior in adult female and male offspring. Front Neurosci. (2019) 13:470. doi: 10.3389/fnins.2019.00470
399. Hodes GE, Pfau ML, Purushothaman I, Ahn HF, Golden SA, Christoffel DJ, et al. Sex differences in nucleus Accumbens transcriptome profiles associated with susceptibility versus resilience to subchronic Variable stress. J Neurosci. (2015) 35(50):16362–76. doi: 10.1523/JNEUROSCI.1392-15.2015
400. Williams ES, Manning CE, Eagle AL, Swift-Gallant A, Duque-Wilckens N, Chinnusamy S, et al. Androgen-Dependent excitability of mouse ventral hippocampal afferents to nucleus Accumbens underlies sex-specific susceptibility to stress. Biol Psychiatry. (2020) 87(6):492–501. doi: 10.1016/j.biopsych.2019.08.006
401. Lasselin J. Back to the future of psychoneuroimmunology: studying inflammation-induced sickness behavior. Brain Behav Immun Health. (2021) 18:100379. doi: 10.1016/j.bbih.2021.1
402. Lasselin J, Schedlowski M, Karshikoff B, Engler H, Lekander M, Konsman JP. Comparison of bacterial lipopolysaccharide-induced sickness behavior in rodents and humans: relevance for symptoms of anxiety and depression. Neurosci Biobehav Rev. (2020) 115:15–24. doi: 10.1016/j.neubiorev.2020.05.001
403. Dowlati Y, Herrmann N, Swardfager W, Liu H, Sham L, Reim EK, et al. A meta-analysis of cytokines in major depression. Biol Psychiatry. (2010) 67(5):446–57. doi: 10.1016/j.biopsych.2009.09.033
404. Liu Y, Ho RC, Mak A. Interleukin (IL)-6, tumour necrosis factor alpha (TNF-α) and soluble interleukin-2 receptors (sIL-2R) are elevated in patients with major depressive disorder: a meta-analysis and meta-regression. J Affect Disord. (2012) 139(3):230–9. doi: 10.1016/j.jad.2011.08.003
405. Köhler-Forsberg O, Petersen L, Gasse C, Mortensen PB, Dalsgaard S, Yolken RH, et al. A nationwide study in Denmark of the association between treated infections and the subsequent risk of treated mental disorders in children and adolescents. JAMA Psychiatry. (2019) 76(3):271–9. doi: 10.1001/jamapsychiatry.2018.3428
406. Steptoe A, Hamer M, Chida Y. The effects of acute psychological stress on circulating inflammatory factors in humans: a review and meta-analysis. Brain Behav Immun. (2007) 21(7):901–12. doi: 10.1016/j.bbi.2007.03.011
407. Rosenblat JD, Cha DS, Mansur RB, McIntyre RS. Inflamed moods: a review of the interactions between inflammation and mood disorders. Prog Neuropsychopharmacol Biol Psychiatry. (2014) 53:23–34. doi: 10.1016/j.pnpbp.2014.01.013
408. Stapelberg NJC, Pratt R, Neumann DL, Shum DHK, Brandis S, Muthukkumarasamy V, et al. From feedback loop transitions to biomarkers in the psycho-immune-neuroendocrine network: detecting the critical transition from health to major depression. Neurosci Biobehav Rev. (2018) 90:1–15. doi: 10.1016/j.neubiorev.2018.03.005
409. Rivest S, Lacroix S, Vallières L, Nadeau S, Zhang J, Laflamme N. How the blood talks to the brain parenchyma and the paraventricular nucleus of the hypothalamus during systemic inflammatory and infectious stimuli. Proc Soc Exp Biol Med. (2000) 223(1):22–38. doi: 10.1046/j.1525-1373.2000.22304.x
410. Dantzer R, O'Connor JC, Freund GG, Johnson RW, Kelley KW. From inflammation to sickness and depression: when the immune system subjugates the brain. Nat Rev Neurosci. (2008) 9(1):46–56. doi: 10.1038/nrn2297
411. Cunningham C, Campion S, Teeling J, Felton L, Perry VH. The sickness behaviour and CNS inflammatory mediator profile induced by systemic challenge of mice with synthetic double-stranded RNA (poly I:c). Brain Behav Immun. (2007) 21(4):490–502. doi: 10.1016/j.bbi.2006.12.007
412. Kubera M, Curzytek K, Duda W, Leskiewicz M, Basta-Kaim A, Budziszewska B, et al. A new animal model of (chronic) depression induced by repeated and intermittent lipopolysaccharide administration for 4 months. Brain Behav Immun. (2013) 31:96–104. doi: 10.1016/j.bbi.2013.01.001
413. Borges BC, Rorato R, Antunes-Rodrigues J, Elias LL. Glial cell activity is maintained during prolonged inflammatory challenge in rats. Braz J Med Biol Res. (2012) 45(8):784–91. doi: 10.1590/s0100-879x2012007500069
414. Baghel MS, Singh B, Dhuriya YK, Shukla RK, Patro N, Khanna VK, et al. Postnatal exposure to poly (I:c) impairs learning and memory through changes in synaptic plasticity gene expression in developing rat brain. Neurobiol Learn Mem. (2018) 155:379–89. doi: 10.1016/j.nlm.2018.09.005
415. Karshikoff B, Lekander M, Soop A, Lindstedt F, Ingvar M, Kosek E, et al. Modality and sex differences in pain sensitivity during human endotoxemia. Brain Behav Immun. (2015) 46:35–43. doi: 10.1016/j.bbi.2014.11.014
416. Lasselin J, Elsenbruch S, Lekander M, Axelsson J, Karshikoff B, Grigoleit JS, et al. Mood disturbance during experimental endotoxemia: predictors of state anxiety as a psychological component of sickness behavior. Brain Behav Immun. (2016) 57:30–7. doi: 10.1016/j.bbi.2016.01.003
417. Engler H, Brendt P, Wischermann J, Wegner A, Röhling R, Schoemberg T, et al. Selective increase of cerebrospinal fluid IL-6 during experimental systemic inflammation in humans: association with depressive symptoms. Mol Psychiatry. (2017) 22(10):1448–54. doi: 10.1038/mp.2016.264
418. Eisenberger NI, Inagaki TK, Rameson LT, Mashal NM, Irwin MR. An fMRI study of cytokine-induced depressed mood and social pain: the role of sex differences. Neuroimage. (2009) 47(3):881–90. doi: 10.1016/j.neuroimage.2009.04.040
419. Coyle SM, Calvano SE, Lowry SF. Gender influences in vivo human responses to endotoxin. Shock. (2006) 26(6):538–43. doi: 10.1097/01.shk.0000232589.39001.4d
420. Murtaj V, Belloli S, Di Grigoli G, Pannese M, Ballarini E, Rodriguez-Menendez V, et al. Age and sex influence the neuro-inflammatory response to a peripheral acute LPS challenge. Front Aging Neurosci. (2019) 11:299. doi: 10.3389/fnagi.2019.00299
421. Wegner A, Benson S, Rebernik L, Spreitzer I, Jäger M, Schedlowski M, et al. Sex differences in the pro-inflammatory cytokine response to endotoxin unfold in vivo but not ex vivo in healthy humans. Innate Immun. (2017) 23(5):432–9. doi: 10.1177/1753425917707026
422. Abbasi A, de Paula Vieira R, Bischof F, Walter M, Movassaghi M, Berchtold NC, et al. Sex-specific variation in signaling pathways and gene expression patterns in human leukocytes in response to endotoxin and exercise. J Neuroinflammation. (2016) 13(1):289. doi: 10.1186/s12974-016-0758-5
423. Lefèvre N, Corazza F, Duchateau J, Desir J, Casimir G. Sex differences in inflammatory cytokines and CD99 expression following in vitro lipopolysaccharide stimulation. Shock. (2012) 38(1):37–42. doi: 10.1097/SHK.0b013e3182571e46
424. Harper JA, South C, Trivedi MH, Toups MS. Pilot investigation into the sickness response to influenza vaccination in adults: effect of depression and anxiety. Gen Hosp Psychiatry. (2017) 48:56–61. doi: 10.1016/j.genhosppsych.2017.07.005
425. Muscatell KA, Moieni M, Inagaki TK, Dutcher JM, Jevtic I, Breen EC, et al. Exposure to an inflammatory challenge enhances neural sensitivity to negative and positive social feedback. Brain Behav Immun. (2016) 57:21–9. doi: 10.1016/j.bbi.2016.03.022
426. Moieni M, Irwin MR, Jevtic I, Breen EC, Eisenberger NI. Inflammation impairs social cognitive processing: a randomized controlled trial of endotoxin. Brain Behav Immun. (2015) 48:132–8. doi: 10.1016/j.bbi.2015.03.002
427. Benson S, Engler H, Wegner A, Rebernik L, Spreitzer I, Schedlowski M, et al. What makes you feel sick after inflammation? Predictors of acute and persisting physical sickness symptoms induced by experimental endotoxemia. Clin Pharmacol Ther. (2017) 102(1):141–51. doi: 10.1002/cpt.618
428. Eisenberger NI, Inagaki TK, Mashal NM, Irwin MR. Inflammation and social experience: an inflammatory challenge induces feelings of social disconnection in addition to depressed mood. Brain Behav Immun. (2010) 24(4):558–63. doi: 10.1016/j.bbi.2009.12.009
429. Morera LP, Marchiori GN, Medrano LA, Defagó MD. Stress, dietary patterns and cardiovascular disease: a Mini-review. Front Neurosci. (2019) 13:1226. doi: 10.3389/fnins.2019.01226
430. Michels N, Sioen I, Braet C, Eiben G, Hebestreit A, Huybrechts I, et al. Stress, emotional eating behaviour and dietary patterns in children. Appetite. (2012) 59(3):762–9. doi: 10.1016/j.appet.2012.08.010
431. Razzoli M, Pearson C, Crow S, Bartolomucci A. Stress, overeating, and obesity: insights from human studies and preclinical models. Neurosci Biobehav Rev. (2017) 76(Pt A):154–62. doi: 10.1016/j.neubiorev.2017.01.026
432. Ip CK, Zhang L, Farzi A, Qi Y, Clarke I, Reed F, et al. Amygdala NPY circuits promote the development of accelerated obesity under chronic stress conditions. Cell Metab. (2019) 30(1):111–128.e6. doi: 10.1016/j.cmet.2019.04.001
433. Ulrich-Lai YM, Figueiredo HF, Ostrander MM, Choi DC, Engeland WC, Herman JP. Chronic stress induces adrenal hyperplasia and hypertrophy in a subregion-specific manner. Am J Physiol Endocrinol Metab. (2006) 291(5):E965–73. doi: 10.1152/ajpendo.00070.2006
434. Yau YH, Potenza MN. Stress and eating behaviors. Minerva Endocrinol. (2013) 38(3):255–67.24126546
435. Kuo WC, Bratzke LC, Oakley LD, Kuo F, Wang H, Brown RL. The association between psychological stress and metabolic syndrome: a systematic review and meta-analysis. Obes Rev. (2019) 20(11):1651–64. doi: 10.1111/obr.12915
436. Thompson S, Thompson SH, Romeo S. Gender and racial differences in emotional eating, food addiction symptoms, and body weight satisfac- tion among undergraduates. J Diabetes Obes. (2015) 2(2):93–8. doi: 10.15436/2376-0494.15.035
437. Anversa RG, Campbell EJ, Ch'ng SS, Gogos A, Lawrence AJ, Brown RM. A model of emotional stress-induced binge eating in female mice with no history of food restriction. Genes Brain Behav. (2020) 19(3):e12613. doi: 10.1111/gbb.12613
438. Furman O, Tsoory M, Chen A. Differential chronic social stress models in male and female mice. Eur J Neurosci. (2022) 55(9–10):2777–93. doi: 10.1111/ejn.15481
439. Paré WP, Blair GR, Kluczynski J, Tejani-Butt S. Gender differences in acute and chronic stress in wistar Kyoto (WKY) rats. Integr Physiol Behav Sci. (1999) 34(4):227–41. doi: 10.1007/BF02688691
440. Booth FW, Roberts CK, Laye MJ. Lack of exercise is a major cause of chronic diseases. Compr Physiol. (2012) 2(2):1143–211. doi: 10.1002/cphy.c110025
441. Hamer M. Psychosocial stress and cardiovascular disease risk: the role of physical activity. Psychosom Med. (2012) 74(9):896–903. doi: 10.1097/PSY.0b013e31827457f4
442. DeVallance E, Riggs D, Jackson B, Parkulo T, Zaslau S, Chantler PD, et al. Effect of chronic stress on running wheel activity in mice. PLoS One. (2017) 12(9):e0184829. doi: 10.1371/journal.pone.0184829
443. Khakpoor S, Saed O, Shahsavar A. The concept of “anxiety sensitivity” in social anxiety disorder presentations, symptomatology, and treatment: a theoretical perspective. Cogent Psychol. (2019) 6(1):1617658. doi: 10.1080/23311908.2019.1617658
444. Mantar A, Yemez B, Alkın T. Anxiety sensitivity and its importance in psychiatric disorders. Turk Psikiyatri Derg. (2011) 22(3):187–93.21870308
445. Olatunji BO, Wolitzky-Taylor KB. Anxiety sensitivity and the anxiety disorders: a meta-analytic review and synthesis. Psychol Bull. (2009) 135(6):974–99. doi: 10.1037/a0017428
446. Goodin BR, McGuire LM, Stapleton LM, Quinn NB, Fabian LA, Haythornthwaite JA, et al. Pain catastrophizing mediates the relationship between self-reported strenuous exercise involvement and pain ratings: moderating role of anxiety sensitivity. Psychosom Med. (2009) 71(9):1018–25. doi: 10.1097/PSY.0b013e3181bc62ba
447. McWilliams LA, Asmundson GJG. Is there a negative association between anxiety sensitivity and arousal-increasing substances and activities? J Anxiety Disord. (2001) 15:161–70. doi: 10.1016/S0887-6185(01)00056-1
448. Smits JA, Zvolensky MJ. Emotional vulnerability as a function of physical activity among individuals with panic disorder. Depress Anxiety. (2006) 23(2):102–6. doi: 10.1002/da.20146
449. Pagotto U, Fallo F, Fava GA, Boscaro M, Sonino N. Anxiety sensitivity in essential-hypertension. Stress Med. (1992) 8(2):113–5. doi: 10.1002/smi.2460080210
450. Chiaie RD, Iannucci G, Paroli M, Salviati M, Caredda M, Pasquini M, et al. Symptomatic subsyndromal depression in hospitalized hypertensive patients. J Affect Disord. (2011) 135(1–3):168–76. doi: 10.1016/j.jad.2011.07.008
451. Norman SB, Lang AJ. The functional impact of anxiety sensitivity in the chronically physically ill. Depress Anxiety. (2005) 21(4):154–60. doi: 10.1002/da.20076
452. Seldenrijk A, van Hout HP, van Marwijk HW, de Groot E, Gort J, Rustemeijer C, et al. Sensitivity to depression or anxiety and subclinical cardiovascular disease. J Affect Disord. (2013) 146(1):126–31. doi: 10.1016/j.jad.2012.06.026
453. DeWolfe CEJ, Watt MC, Romero-Sanchiz P, Stewart SH. Gender differences in physical activity are partially explained by anxiety sensitivity in post-secondary students. J Am Coll Health. (2020) 68(3):219–22. doi: 10.1080/07448481.2018.1549048
454. Osuji E, Prior PL, Suskin N, Frisbee JC, Frisbee SJ. The relationship between anxiety sensitivity and clinical outcomes in cardiac rehabilitation: a scoping review. Am J Prev Cardiol. (2022) 12:100376. doi: 10.1016/j.ajpc.2022
455. Kashani M, Eliasson A, Vernalis M. Perceived stress correlates with disturbed sleep: a link connecting stress and cardiovascular disease. Stress. (2012) 15(1):45–51. doi: 10.3109/10253890.2011.578266
456. Vgontzas AN, Bixler EO, Lin HM, Prolo P, Mastorakos G, Vela-Bueno A, et al. Chronic insomnia is associated with nyctohemeral activation of the hypothalamic-pituitary-adrenal axis: clinical implications. J Clin Endocrinol Metab. (2001) 86(8):3787–94. doi: 10.1210/jcem.86.8.7778
457. Han KS, Kim L, Shim I. Stress and sleep disorder. Exp Neurobiol. (2012) 21(4):141–50. doi: 10.5607/en.2012.21.4.141
458. Ihara T, Nakamura Y, Mitsui T, Tsuchiya S, Kanda M, Kira S, et al. Intermittent restraint stress induces circadian misalignment in the mouse bladder, leading to nocturia. Sci Rep. (2019) 9(1):10069. doi: 10.1038/s41598-019-46517-w
459. Hagan JJ, Leslie RA, Patel S, Evans ML, Wattam TA, Holmes S, et al. Orexin A activates locus coeruleus cell firing and increases arousal in the rat. Proc Natl Acad Sci U S A. (1999) 96(19):10911–6. doi: 10.1073/pnas.96.19.10911
460. Ida T, Nakahara K, Murakami T, Hanada R, Nakazato M, Murakami N. Possible involvement of orexin in the stress reaction in rats. Biochem Biophys Res Commun. (2000) 270(1):318–23. doi: 10.1006/bbrc.2000.2412
461. Al-Barazanji KA, Wilson S, Baker J, Jessop DS, Harbuz MS. Central orexin-A activates hypothalamic-pituitary-adrenal axis and stimulates hypothalamic corticotropin releasing factor and arginine vasopressin neurones in conscious rats. Neuroendocrinol. (2001) 13(5):421–4. doi: 10.1046/j.1365-2826.2001.00655.x
462. Jászberényi M, Bujdosó E, Pataki I, Telegdy G. Effects of orexins on the hypothalamic-pituitary-adrenal system. J Neuroendocrinol. (2000) 12(12):1174–8. doi: 10.1046/j.1365-2826.2000.00572.x
463. Shirasaka T, Nakazato M, Matsukura S, Takasaki M, Kannan H. Sympathetic and cardiovascular actions of orexins in conscious rats. Am J Physiol. (1999) 277(6):R1780–5. doi: 10.1152/ajpregu.1999.277.6.R1780
464. Smith PM, Connolly BC, Ferguson AV. Microinjection of orexin into the rat nucleus tractus solitarius causes increases in blood pressure. Brain Res. (2002) 950(1–2):261–7. doi: 10.1016/s0006-8993(02)03048-2
465. Lutter M, Krishnan V, Russo SJ, Jung S, McClung CA, Nestler EJ. Orexin signaling mediates the antidepressant-like effect of calorie restriction. J Neurosci. (2008) 28(12):3071–5. doi: 10.1523/JNEUROSCI.5584-07.2008
466. Kim TK, Kim JE, Park JY, Lee JE, Choi J, Kim H, et al. Antidepressant effects of exercise are produced via suppression of hypocretin/orexin and melanin-concentrating hormone in the basolateral amygdala. Neurobiol Dis. (2015) 79:59–69. doi: 10.1016/j.nbd.2015.04.004
467. Nocjar C, Zhang J, Feng P, Panksepp J. The social defeat animal model of depression shows diminished levels of orexin in mesocortical regions of the dopamine system, and of dynorphin and orexin in the hypothalamus. Neuroscience. (2012) 218:138–53. doi: 10.1016/j.neuroscience.2012.05.033
468. Nollet M, Gaillard P, Minier F, Tanti A, Belzung C, Leman S. Activation of orexin neurons in dorsomedial/perifornical hypothalamus and antidepressant reversal in a rodent model of depression. Neuropharmacology. (2011) 61(1–2):336–46. doi: 10.1016/j.neuropharm.2011.04.022
469. Chung HS, Kim JG, Kim JW, Kim HW, Yoon BJ. Orexin administration to mice that underwent chronic stress produces bimodal effects on emotion-related behaviors. Regul Pept. (2014) 194–195:16–22. doi: 10.1016/j.regpep.2014.11.003
470. Grafe LA, Bhatnagar S. Orexins and stress. Front Neuroendocrinol. (2018) 51:132–45. doi: 10.1016/j.yfrne.2018.06.003
471. Grafe LA, Cornfeld A, Luz S, Valentino R, Bhatnagar S. Orexins mediate sex differences in the stress response and in cognitive flexibility. Biol Psychiatry. (2017) 81(8):683–92. doi: 10.1016/j.biopsych.2016.10.013
472. van Jaarsveld CH, Fidler JA, Steptoe A, Boniface D, Wardle J. Perceived stress and weight gain in adolescence: a longitudinal analysis. Obesity (Silver Spring). (2009) 17(12):2155–61. doi: 10.1038/oby.2009.183
473. Lissau I, Sørensen TI. Parental neglect during childhood and increased risk of obesity in young adulthood. Lancet. (1994) 343(8893):324–7. doi: 10.1016/s0140-6736(94)91163-0
474. Wardle J, Chida Y, Gibson EL, Whitaker KL, Steptoe A. Stress and adiposity: a meta-analysis of longitudinal studies. Obesity (Silver Spring). (2011) 19(4):771–8. doi: 10.1038/oby.2010.241
475. Räikkönen K, Hautanen A, Keltikangas-Järvinen L. Association of stress and depression with regional fat distribution in healthy middle-aged men. J Behav Med. (1994) 17(6):605–16. doi: 10.1007/BF01857600
476. Pasquali R, Cantobelli S, Casimirri F, Capelli M, Bortoluzzi L, Flamia R, et al. The hypothalamic-pituitary-adrenal axis in obese women with different patterns of body fat distribution. J Clin Endocrinol Metab. (1993) 77(2):341–6. doi: 10.1210/jcem.77.2.8393881
477. Branth S, Ronquist G, Stridsberg M, Hambraeus L, Kindgren E, Olsson R, et al. Development of abdominal fat and incipient metabolic syndrome in young healthy men exposed to long-term stress. Nutr Metab Cardiovasc Dis. (2007) 17(6):427–35. doi: 10.1016/j.numecd.2006.03.001
478. Bergmann N, Ballegaard S, Krogh J, Bech P, Hjalmarson Å, Gyntelberg F, et al. Chronic psychological stress seems associated with elements of the metabolic syndrome in patients with ischaemic heart disease. Scand J Clin Lab Invest. (2017) 77(7):513–9. doi: 10.1080/00365513.2017.1354254
479. Kopf D, Westphal S, Luley CW, Ritter S, Gilles M, Weber-Hamann B, et al. Lipid metabolism and insulin resistance in depressed patients: significance of weight, hypercortisolism, and antidepressant treatment. J Clin Psychopharmacol. (2004) 24(5):527–31. doi: 10.1097/01.jcp.0000138762.23482.63
480. Peters A, McEwen BS. Stress habituation, body shape and cardiovascular mortality. Neurosci Biobehav Rev. (2015) 56:139–50. doi: 10.1016/j.neubiorev.2015.07.001
481. Stewart PM, Petersenn S. Rationale for treatment and therapeutic options in cushing's disease. Best Pract Res Clin Endocrinol Metab. (2009) 23(Suppl 1):S15–22. doi: 10.1016/S1521-690X(09)70004-1
482. McDonough AK, Curtis JR, Saag KG. The epidemiology of glucocorticoid-associated adverse events. Curr Opin Rheumatol. (2008) 20(2):131–7. doi: 10.1097/BOR.0b013e3282f51031
483. Weber-Hamann B, Hentschel F, Kniest A, Deuschle M, Colla M, Lederbogen F, et al. Hypercortisolemic depression is associated with increased intra-abdominal fat. Psychosom Med. (2002) 64(2):274–7. doi: 10.1097/00006842-200203000-00010
484. Peckett AJ, Wright DC, Riddell MC. The effects of glucocorticoids on adipose tissue lipid metabolism. Metab Clin Exp. (2011) 60(11):1500–10. doi: 10.1016/j.metabol.2011.06.012
485. Tsigos C, Chrousos GP. Differential diagnosis and management of cushing's syndrome. Annu Rev Med. (1996) 47:443–61. doi: 10.1146/annurev.med.47.1.443
486. Tsigos C, Kyrou J, Chrousos GP. In: DeGroot L, editors. Stress, endocrine physiology and pathophysiology. Section Adrenal Glands and Function (2004). Available at: http://www.endotext.org
487. Fransson L, Franzén S, Rosengren V, Wolbert P, Sjöholm Å, Ortsäter H. β-Cell adaptation in a mouse model of glucocorticoid-induced metabolic syndrome. J Endocrinol. (2013) 219(3):231–41. doi: 10.1530/JOE-13-0189
488. Abad V, Chrousos GP, Reynolds JC, Nieman LK, Hill SC, Weinstein RS, et al. Glucocorticoid excess during adolescence leads to a major persistent deficit in bone mass and an increase in central body fat. J Bone Miner Res. (2001) 16(10):1879–85. doi: 10.1359/jbmr.2001.16.10.1879
489. Campbell JE, Peckett AJ, Dsouza A, Hawk TJ, Riddell MC. Adipogenic and lipolytic effects of chronic glucocorticoid exposure. Am J Physiol Cell Physiol. (2011) 300(1):C198–209. doi: 10.1152/ajpcell.00045.2010
490. Jahng JW, Kim NY, Ryu V, Yoo SB, Kim BT, Kang DW, et al. Dexamethasone reduces food intake, weight gain and the hypothalamic 5-HT concentration and increases plasma leptin in rats. Eur J Pharmacol. (2008) 581(1–2):64–70. doi: 10.1016/j.ejphar.2007.11.029
491. Rebuffé-Scrive M, Walsh UA, McEwen B, Rodin J. Effect of chronic stress and exogenous glucocorticoids on regional fat distribution and metabolism. Physiol Behav. (1992) 52(3):583–90. doi: 10.1016/0031-9384(92)90351-2
492. Grassi G, Quarti-Trevano F, Seravalle G, Dell'Oro R. Cardiovascular risk and adrenergic overdrive in the metabolic syndrome. Nutr Metab Cardiovasc Dis. (2007) 17(6):473–81. doi: 10.1016/j.numecd.2007.01.004
493. Flaa A, Aksnes TA, Kjeldsen SE, Eide I, Rostrup M. Increased sympathetic reactivity may predict insulin resistance: an 18-year follow-up study. Metab Clin Exp. (2008) 57(10):1422–7. doi: 10.1016/j.metabol.2008.05.012
494. Matsuura N, Nagasawa K, Minagawa Y, Ito S, Sano Y, Yamada Y, et al. Restraint stress exacerbates cardiac and adipose tissue pathology via β-adrenergic signaling in rats with metabolic syndrome. Am J Physiol Heart Circ Physiol. (2015) 308(10):H1275–86. doi: 10.1152/ajpheart.00906.2014
495. Wirtz PH, Ehlert U, Bärtschi C, Redwine LS, von Känel R. Changes in plasma lipids with psychosocial stress are related to hypertension status and the norepinephrine stress response. Metab Clin Exp. (2009) 58(1):30–7. doi: 10.1016/j.metabol.2008.08.003
496. Lambert EA, Lambert GW. Stress and its role in sympathetic nervous system activation in hypertension and the metabolic syndrome. Curr Hypertens Rep. (2011) 13(3):244–8. doi: 10.1007/s11906-011-0186-y
497. Kuo LE, Kitlinska JB, Tilan JU, Li L, Baker SB, Johnson MD, et al. Neuropeptide Y acts directly in the periphery on fat tissue and mediates stress-induced obesity and metabolic syndrome. Nat Med. (2007) 13(7):803–11. doi: 10.1038/nm1611
498. Ailanen L, Ruohonen ST, Vähätalo LH, Tuomainen K, Eerola K, Salomäki-Myftari H, et al. The metabolic syndrome in mice overexpressing neuropeptide Y in noradrenergic neurons. J Endocrinol. (2017) 234(1):57–72. doi: 10.1530/JOE-16-0223
499. Rohleder N. Stimulation of systemic low-grade inflammation by psychosocial stress. Psychosom Med. (2014) 76(3):181–9. doi: 10.1097/PSY.0000000000000049
500. Liu YZ, Wang YX, Jiang CL. Inflammation: the common pathway of stress-related diseases. Front Hum Neurosci. (2017) 11:316. doi: 10.3389/fnhum.2017.00316
501. Ippoliti F, Canitano N, Businaro R. Stress and obesity as risk factors in cardiovascular diseases: a neuroimmune perspective. J Neuroimmune Pharmacol. (2013) 8(1):212–26. doi: 10.1007/s11481-012-9432-6
502. Baker RG, Hayden MS, Ghosh S. NF-κB, inflammation, and metabolic disease. Cell Metab. (2011) 13(1):11–22. doi: 10.1016/j.cmet.2010.12.008
503. Jeon BT, Jeong EA, Shin HJ, Lee Y, Lee DH, Kim HJ, et al. Resveratrol attenuates obesity-associated peripheral and central inflammation and improves memory deficit in mice fed a high-fat diet. Diabetes. (2012) 61(6):1444–54. doi: 10.2337/db11-1498
504. Thaler JP, Yi CX, Schur EA, Guyenet SJ, Hwang BH, Dietrich MO, et al. Obesity is associated with hypothalamic injury in rodents and humans. J Clin Invest. (2012) 122(2):778. doi: 10.1172/JCI62813
505. Abbott NJ. Inflammatory mediators and modulation of blood–brain barrier permeability. Cell Mol Neurobiol. (2000) 20:131–47. doi: 10.1023/A:1007074420772
506. Kamari Y, Shaish A, Vax E, Shemesh S, Kandel-Kfir M, Arbel Y, et al. Lack of interleukin-1α or interleukin-1β inhibits transformation of steatosis to steatohepatitis and liver fibrosis in hypercholesterolemic mice. J Hepatol. (2011) 55(5):1086–94. doi: 10.1016/j.jhep.2011.01.048
507. Tilg H, Moschen AR. IL-1 cytokine family members and NAFLD: neglected in metabolic liver inflammation. J Hepatol. (2011) 55(5):960–2. doi: 10.1016/j.jhep.2011.04.007
508. Tack CJ, Stienstra R, Joosten LA, Netea MG. Inflammation links excess fat to insulin resistance: the role of the interleukin-1 family. Immunol Rev. (2012) 249(1):239–52. doi: 10.1111/j.1600-065X.2012.01145.x
509. Zhu CB, Blakely RD, Hewlett WA. The proinflammatory cytokines interleukin-1beta and tumor necrosis factor-alpha activate serotonin transporters. Neuropsychopharmacology. (2006) 31(10):2121–31. doi: 10.1038/sj.npp.1301029
510. Wellen KE, Hotamisligil GS. Inflammation, stress, and diabetes. J Clin Invest. (2005) 115(5):1111–9. doi: 10.1172/JCI25102
511. Day CP. Non-alcoholic fatty liver disease: current concepts and management strategies. Clin Med (Lond). (2006) 6(1):19–25. doi: 10.7861/clinmedicine.6-1-19
512. Nicolaides NC, Kyratzi E, Lamprokostopoulou A, Chrousos GP, Charmandari E. Stress, the stress system and the role of glucocorticoids. Neuroimmunomodulation. (2015) 22(1–2):6–19. doi: 10.1159/000362736
513. Richards JC, Hof A, Alvarenga M. Serum lipids and their relationships with hostility and angry affect and behaviors in men. Health Psychol. (2000) 19(4):393–8. doi: 10.1037/0278-6133.19.4.393
514. Lata H, Ahuja GK, Narang AP, Walia L. Effect of immobilisation stress on lipid peroxidation and lipid profile in rabbits. Indian J Clin Biochem. (2004) 19(2):1–4. doi: 10.1007/BF02894248
515. Sato T, Yamamoto H, Sawada N, Nashiki K, Tsuji M, Muto K, et al. Restraint stress alters the duodenal expression of genes important for lipid metabolism in rat. Toxicology. (2006) 227(3):248–61. doi: 10.1016/j.tox.2006.08.009
516. Nayanatara AK, Nagaraja HS, Ramaswamy C, Bhagyalakshmi K, Ramesh BM, Damodara GKM, et al. Effect of chronic unpredictable stressors on some selected lipid parameters and biochemical parameters in wistar rats. J Chin Clin Med. (2009) 4(2):92–7.
517. Devaki M, Nirupama R, Yajurvedi HN. Chronic stress-induced oxidative damage and hyperlipidemia are accompanied by atherosclerotic development in rats. Stress. (2013) 16(2):233–43. doi: 10.3109/10253890.2012.719052
518. Neves VJ, Moura MJ, Tamascia ML, Ferreira R, Silva NS, Costa R, et al. Proatherosclerotic effects of chronic stress in male rats: altered phenylephrine sensitivity and nitric oxide synthase activity of aorta and circulating lipids. Stress. (2009) 12(4):320–7. doi: 10.1080/10253890802437779
519. Geerling JJ, Boon MR, Kooijman S, Parlevliet ET, Havekes LM, Romijn JA, et al. Sympathetic nervous system control of triglyceride metabolism: novel concepts derived from recent studies. J Lipid Res. (2014) 55(2):180–9. doi: 10.1194/jlr.R045013
520. Frohlich ED. State of the art lecture. Risk mechanisms in hypertensive heart disease. Hypertension. (1999) 34(4 Pt 2):782–9. doi: 10.1161/01.HYP.34.4.782
521. Schmidt KL, Macdougall-Shackleton EA, Macdougall-Shackleton SA. Developmental stress has sex-specific effects on nestling growth and adult metabolic rates but no effect on adult body size or body composition in song sparrows. J Exp Biol. (2012) 215(Pt 18):3207–17. doi: 10.1242/jeb.068965
522. Verma R, Balhara YP, Gupta CS. Gender differences in stress response: role of developmental and biological determinants. Ind Psychiatry J. (2011) 20(1):4–10. doi: 10.4103/0972-6748.98407
523. Stopa LRS, de Souza CF, Santos GF, Martins AB, Ferreira RN, de Andrade FG, et al. Sex differences in glucocorticoids-induced anabolic effects in rats. Physiol Behav. (2019) 209:112587. doi: 10.1016/j.physbeh.2019.112587
524. Fachin A, Silva RK, Noschang CG, Pettenuzzo L, Bertinetti L, Billodre MN, et al. Stress effects on rats chronically receiving a highly palatable diet are sex-specific. Appetite. (2008) 51(3):592–8. doi: 10.1016/j.appet.2008.04.016
525. Link JC, Reue K. Genetic basis for sex differences in obesity and lipid metabolism. Annu Rev Nutr. (2017) 37:225–45. doi: 10.1146/annurev-nutr-071816-064827
526. Hazzard WR. Atherogenesis: why women live longer than men. Geriatrics. (1985) 40(1):42–51; 54.3965355
527. Djekic K, Ipp E. Loss of sex difference in high-density lipoprotein cholesterol in diabetic women during acute stress. J Clin Endocrinol Metab. (2014) 99(11):E2357–61. doi: 10.1210/jc.2014-2466
528. Ancelin ML, Carrière I, Boulenger JP, Malafosse A, Stewart R, Cristol JP, et al. Gender and genotype modulation of the association between lipid levels and depressive symptomatology in community-dwelling elderly (the ESPRIT study). Biol Psychiatry. (2010) 68(2):125–32. doi: 10.1016/j.biopsych.2010.04.011
529. LaRosa JC. Lipids and cardiovascular disease: do the findings and therapy apply equally to men and women? Womens Health Issues. (1992) 2(2):102–11; discussion 111–3. doi: 10.1016/s1049-3867(05)80278-6
530. Balog M, Mlinarević D, Šerić V, Miljanović M, Blažeković R, Degmečić IV, et al. Plasma content of glucose, C-reactive protein, uric acid and cholesterol in male, female and ovariectomized rats upon acute and chronic stress–a path for development of cardiovascular diseases. Coll Antropol. (2015) 39(2):385–92.26753455
531. Luft C, Levices IP, Pedrazza L, de Oliveira JR, Donadio MVF. Sex-dependent metabolic effects of pregestational exercise on prenatally stressed mice. J Dev Orig Health Dis. (2021) 12(2):271–9. doi: 10.1017/S2040174420000343
532. Juárez YR, Quiroga S, Prochnik A, Wald M, Tellechea ML, Genaro AM, et al. Influence of prenatal stress on metabolic abnormalities induced by postnatal intake of a high-fat diet in BALB/c mice. J Dev Orig Health Dis. (2021) 12(5):721–30. doi: 10.1017/S2040174420000987
533. Sher LD, Geddie H, Olivier L, Cairns M, Truter N, Beselaar L, et al. Chronic stress and endothelial dysfunction: mechanisms, experimental challenges, and the way ahead. Am J Physiol Heart Circ Physiol. (2020) 319(2):H488–506. doi: 10.1152/ajpheart.00244.2020
534. Toda N, Nakanishi-Toda M. How mental stress affects endothelial function. Pflugers Arch. (2011) 462(6):779–94. doi: 10.1007/s00424-011-1022-6
535. Gottdiener JS, Kop WJ, Hausner E, McCeney MK, Herrington D, Krantz DS. Effects of mental stress on flow-mediated brachial arterial dilation and influence of behavioral factors and hypercholesterolemia in subjects without cardiovascular disease. Am J Cardiol. (2003) 92(6):687–91. doi: 10.1016/s0002-9149(03)00823-3
536. Takase B, Akima T, Uehata A, Ohsuzu F, Kurita A. Effect of chronic stress and sleep deprivation on both flow-mediated dilation in the brachial artery and the intracellular magnesium level in humans. Clin Cardiol. (2004) 27(4):223–7. doi: 10.1002/clc.4960270411
537. Mausbach BT, Roepke SK, Ziegler MG, Milic M, von Känel R, Dimsdale JE, et al. Association between chronic caregiving stress and impaired endothelial function in the elderly. J Am Coll Cardiol. (2010) 55(23):2599–606. doi: 10.1016/j.jacc.2009.11.093
538. Hammadah M, Kim JH, Al Mheid I, Samman Tahhan A, Wilmot K, Ramadan R, et al. Coronary and peripheral vasomotor responses to mental stress. J Am Heart Assoc. (2018) 7(10):e008532. doi: 10.1161/JAHA.118.008532
539. Fuchs LC, Landas SK, Johnson AK. Behavioral stress alters coronary vascular reactivity in borderline hypertensive rats. J Hypertens. (1997) 15(3):301–7. doi: 10.1097/00004872-199715030-00012
540. d'Audiffret AC, Frisbee SJ, Stapleton PA, Goodwill AG, Isingrini E, Frisbee JC. Depressive behavior and vascular dysfunction: a link between clinical depression and vascular disease? J Appl Physiol. (2010) 108(5):1041–51. doi: 10.1152/japplphysiol.01440.2009
541. Chung IM, Kim YM, Yoo MH, Shin MK, Kim CK, Suh SH. Immobilization stress induces endothelial dysfunction by oxidative stress via the activation of the angiotensin II/its type I receptor pathway. Atherosclerosis. (2010) 213(1):109–14. doi: 10.1016/j.atherosclerosis.2010.08.052
542. Bouzinova EV, Norregaard R, Boedtkjer DM, Razgovorova IA, Moeller AM, Kudryavtseva O, et al. Association between endothelial dysfunction and depression-like symptoms in chronic mild stress model of depression. Psychosom Med. (2014) 76(4):268–76. doi: 10.1097/PSY.0000000000000062
543. Schaeuble D, Packard AEB, McKlveen JM, Morano R, Fourman S, Smith BL, et al. Prefrontal Cortex regulates chronic stress-induced cardiovascular susceptibility. J Am Heart Assoc. (2019) 8(24):e014451. doi: 10.1161/JAHA.119.014451
544. Groeschel M, Braam B. Connecting chronic and recurrent stress to vascular dysfunction: no relaxed role for the renin-angiotensin system. Am J Physiol Renal Physiol. (2011) 300(1):F1–10. doi: 10.1152/ajprenal.00208.2010
545. Côco H, Pernomian L, Pereira PC, Gomes MS, Marchi KC, Lopes AH, et al. Chronic restraint stress increases angiotensin II potency in the rat carotid: role of cyclooxygenases and reactive oxygen species. J Pharm Pharmacol. (2017) 69(1):52–65. doi: 10.1111/jphp.12659
546. Kröller-Schön S, Jansen T, Tran TLP, Kvandová M, Kalinovic S, Oelze M, et al. Endothelial α1AMPK modulates angiotensin II-mediated vascular inflammation and dysfunction. Basic Res Cardiol. (2019) 114(2):8. doi: 10.1007/s00395-019-0717-2
547. Li R, Mi X, Yang S, Yang Y, Zhang S, Hui R, et al. Long-term stimulation of angiotensin II induced endothelial senescence and dysfunction. Exp Gerontol. (2019) 119:212–20. doi: 10.1016/j.exger.2019.02.012
548. Pavel J, Benicky J, Murakami Y, Sanchez-Lemus E, Saavedra JM. Peripherally administered angiotensin II AT1 receptor antagonists are anti-stress compounds in vivo. Ann N Y Acad Sci. (2008) 1148:360–6. doi: 10.1196/annals.1410.006
549. Clamage DM, Sanford CS, Vander AJ, Mouw DR. Effects of psychosocial stimuli on plasma renin activity in rats. Am J Physiol. (1976) 231(4):1290–4. doi: 10.1152/ajplegacy.1976.231.4.1290
550. Whitworth JA, Mangos GJ, Kelly JJ. Cushing, cortisol, and cardiovascular disease. Hypertension. (2000) 36(5):912–6. doi: 10.1161/01.hyp.36.5.912
551. Mangos GJ, Walker BR, Kelly JJ, Lawson JA, Webb DJ, Whitworth JA. Cortisol inhibits cholinergic vasodilation in the human forearm. Am J Hypertens. (2000) 13(11):1155–60. doi: 10.1016/s0895-7061(00)01201-2
552. Hunter RW, Bailey MA. Glucocorticoids and 11β-hydroxysteroid dehydrogenases: mechanisms for hypertension. Curr Opin Pharmacol. (2015) 21:105–14. doi: 10.1016/j.coph.2015.01.005
553. Bhagat K, Vallance P. Inflammatory cytokines impair endothelium-dependent dilatation in human veins in vivo. Circulation. (1997) 96(9):3042–7. doi: 10.1161/01.cir.96.9.3042
554. Hingorani AD, Cross J, Kharbanda RK, Mullen MJ, Bhagat K, Taylor M, et al. Acute systemic inflammation impairs endothelium-dependent dilatation in humans. Circulation. (2000) 102(9):994–9. doi: 10.1161/01.cir.102.9.994
555. Seidel M, Billert H, Kurpisz M. Regulation of eNOS expression in HCAEC cell line treated with opioids and proinflammatory cytokines. Kardiol Pol. (2006) 64(2):153–8; discussion 159–60.16502366
556. Zhang J, Patel JM, Li YD, Block ER. Proinflammatory cytokines downregulate gene expression and activity of constitutive nitric oxide synthase in porcine pulmonary artery endothelial cells. Res Commun Mol Pathol Pharmacol. (1997) 96(1):71–87.9178369
557. Esler M. Mental stress and human cardiovascular disease. Neurosci Biobehav Rev. (2017) 74(Pt B):269–76. doi: 10.1016/j.neubiorev.2016.10.011
558. Liu MY, Li N, Li WA, Khan H. Association between psychosocial stress and hypertension: a systematic review and meta-analysis. Neurol Res. (2017) 39(6):573–80. doi: 10.1080/01616412.2017.1317904
559. Esler M, Eikelis N, Schlaich M, Lambert G, Alvarenga M, Dawood T, et al. Chronic mental stress is a cause of essential hypertension: presence of biological markers of stress. Clin Exp Pharmacol Physiol. (2008) 35(4):498–502. doi: 10.1111/j.1440-1681.2008.04904.x
560. Mozaffari MS, Schaffer SW. Effect of hypertension and hypertension-glucose intolerance on myocardial ischemic injury. Hypertension. (2003) 42(5):1042–9. doi: 10.1161/01.HYP.0000095614.91961.40
561. Roohafza H, Sattari N, Nouri F, Talaei M, Masoumi G, Sarrafzadegan N, et al. Do any kinds of perceived stressors lead to hypertension? A longitudinal cohort study. Hypertens Res. (2022) 45(6):1058–66. doi: 10.1038/s41440-022-00895-3
562. Oparil S, Zaman MA, Calhoun DA. Pathogenesis of hypertension. Ann Intern Med. (2003) 139(9):761–76. doi: 10.7326/0003-4819-139-9-200311040-00011
563. Lucini D, Di Fede G, Parati G, Pagani M. Impact of chronic psychosocial stress on autonomic cardiovascular regulation in otherwise healthy subjects. Hypertension. (2005) 46(5):1201–6. doi: 10.1161/01.HYP.0000185147.32385.4b
564. Zhang DY, Anderson AS. The sympathetic nervous system and heart failure. Cardiol Clin. (2014) 32(1):33–vii. doi: 10.1016/j.ccl.2013.09.010
565. Kelly JJ, Mangos G, Williamson PM, Whitworth JA. Cortisol and hypertension. Clin Exp Pharmacol Physiol Suppl. (1998) 25:S51–6. doi: 10.1111/j.1440-1681.1998.tb02301.x
566. Hamer M, Steptoe A. Cortisol responses to mental stress and incident hypertension in healthy men and women. J Clin Endocrinol Metab. (2012) 97(1):E29–34. doi: 10.1210/jc.2011-2132
567. Wang M, Pan W, Xu Y, Zhang J, Wan J, Jiang H. Microglia-Mediated neuroinflammation: a potential target for the treatment of cardiovascular diseases. J Inflamm Res. (2022) 15:3083–94. doi: 10.2147/JIR.S350109
568. Stapelberg NJC, Neumann DL, Shum DHK, McConnell H, Hamilton-Craig I. From physiome to pathome: a systems biology model of major depressive disorder and the psycho-immune-neuroendocrine network. Curr Psychiatr Rev. (2015) 11(1):32–62. doi: 10.2174/1573400510666140619211733
569. Segiet A, Smykiewicz P, Kwiatkowski P, Żera T. Tumour necrosis factor and interleukin 10 in blood pressure regulation in spontaneously hypertensive and normotensive rats. Cytokine. (2019) 113:185–94. doi: 10.1016/j.cyto.2018.07.003
570. Moreira JD, Chaudhary P, Frame AA, Puleo F, Nist KM, Abkin EA, et al. Inhibition of microglial activation in rats attenuates paraventricular nucleus inflammation in Gαi2 protein-dependent, salt-sensitive hypertension. Exp Physiol. (2019) 104(12):1892–910. doi: 10.1113/EP087924
571. Yu XJ, Liu XJ, Guo J, Su YK, Zhang N, Qi J, et al. Blockade of microglial activation in hypothalamic paraventricular nucleus improves high salt-induced hypertension. Am J Hypertens. (2022) 35(9):820–7. doi: 10.1093/ajh/hpac052
572. Shi P, Diez-Freire C, Jun JY, Qi Y, Katovich MJ, Li Q, et al. Brain microglial cytokines in neurogenic hypertension. Hypertension. (2010) 56(2):297–303. doi: 10.1161/HYPERTENSIONAHA.110.150409
573. Sriramula S, Cardinale JP, Francis J. Inhibition of TNF in the brain reverses alterations in RAS components and attenuates angiotensin II-induced hypertension. PLoS One. (2013) 8(5):e63847. doi: 10.1371/journal.pone.0063847
574. Lucà F, Abrignani MG, Parrini I, Di Fusco SA, Giubilato S, Rao CM, et al. Update on management of cardiovascular diseases in women. J Clin Med. (2022) 11(5):1176. doi: 10.3390/jcm11051176
575. Shufelt CL, Pacheco C, Tweet MS, Miller VM. Sex-Specific physiology and cardiovascular disease. Adv Exp Med Biol. (2018) 1065:433–54. doi: 10.1007/978-3-319-77932-4_27
576. Appelman Y, van Rijn BB, Ten Haaf ME, Boersma E, Peters SA. Sex differences in cardiovascular risk factors and disease prevention. Atherosclerosis. (2015) 241(1):211–8. doi: 10.1016/j.atherosclerosis.2015.01.027
577. Nickander J, Themudo R, Sigfridsson A, Xue H, Kellman P, Ugander M. Females have higher myocardial perfusion, blood volume and extracellular volume compared to males—an adenosine stress cardiovascular magnetic resonance study. Sci Rep. (2020) 10(1):10380. doi: 10.1038/s41598-020-67196-y
578. Gyllenhammar T, Carlsson M, Jögi J, Arheden H, Engblom H. Myocardial perfusion by CMR coronary sinus flow shows sex differences and lowered perfusion at stress in patients with suspected microvascular angina. Clin Physiol Funct Imaging. (2022) 42(3):208–19. doi: 10.1111/cpf.12750
579. Pepine CJ, Ferdinand KC, Shaw LJ, Light-McGroary KA, Shah RU, Gulati M, et al. ACC CVD in women committee. Emergence of nonobstructive coronary artery disease: a woman's problem and need for change in definition on angiography. J Am Coll Cardiol. (2015) 66(17):1918–33. doi: 10.1016/j.jacc.2015.08.876
580. Ouellette ML, Löffler AI, Beller GA, Workman VK, Holland E, Bourque JM. Clinical characteristics, sex differences, and outcomes in patients with normal or near-normal coronary arteries, non-obstructive or obstructive coronary artery disease. J Am Heart Assoc. (2018) 7(10):e007965. doi: 10.1161/JAHA.117.007965
581. Bairey Merz CN, Pepine CJ, Walsh MN, Fleg JL. Ischemia and No obstructive coronary artery disease (INOCA): developing evidence-based therapies and research agenda for the next decade. Circulation. (2017) 135(11):1075–92. doi: 10.1161/CIRCULATIONAHA
582. Shah SJ, Lam CSP, Svedlund S, Saraste A, Hage C, Tan RS, et al. Prevalence and correlates of coronary microvascular dysfunction in heart failure with preserved ejection fraction: pROMIS-HFpEF. Eur Heart J. (2018) 39(37):3439–50. doi: 10.1093/eurheartj/ehy531
583. Haider A, Bengs S, Maredziak M, Messerli M, Fiechter M, Giannopoulos AA, et al. Heart rate reserve during pharmacological stress is a significant negative predictor of impaired coronary flow reserve in women. Eur J Nucl Med Mol Imaging. (2019) 46(6):1257–67. doi: 10.1007/s00259-019-4265-7
584. Albert CM, McGovern BA, Newell JB, Ruskin JN. Sex differences in cardiac arrest survivors. Circulation. (1996) 93(6):1170–6. doi: 10.1161/01.cir.93.6.1170
585. Du XJ, Riemersma RA, Dart AM. Cardiovascular protection by oestrogen is partly mediated through modulation of autonomic nervous function. Cardiovasc Res. (1995) 30(2):161–5. doi: 10.1016/S0008-6363(95)00030-5
586. Ottenson B, Sørensen MB. Women at cardiac risk: is HRT the route to maintaining cardiovascular health? Int J Gynecol Obstet. (1997) 59:S19–27. doi: 10.1016/S0020-7292(97)90195-8
587. Liccardo D, Arosio B, Corbi G, Cannavo A. Sex/gender- and age-related differences in β-adrenergic receptor signaling in cardiovascular diseases. J Clin Med. (2022) 11(15):4280. doi: 10.3390/jcm11154280
588. Matsukawa T, Sugiyama Y, Watanabe T, Kobayashi F, Mano T. Gender difference in age-related changes in muscle sympathetic nerve activity in healthy subjects. Am J Physiol. (1998) 275(5):R1600–4. doi: 10.1152/ajpregu.1998.275.5.R1600
589. Parashar R, Amir M, Pakhare A, Rathi P, Chaudhary L. Age related changes in autonomic functions. J Clin Diagn Res. (2016) 10(3):CC11–5. doi: 10.7860/JCDR/2016/16889.7497
590. Oneglia A, Nelson MD, Merz CNB. Sex differences in cardiovascular aging and heart failure. Curr Heart Fail Rep. (2020) 17(6):409–23. doi: 10.1007/s11897-020-00487-7
591. Yim SF, Lau TK, Sahota DS, Chung TK, Chang AM, Haines CJ. Prospective randomized study of the effect of “add-back” hormone replacement on vascular function during treatment with gonadotropin-releasing hormone agonists. Circulation. (1998) 98(16):1631–5. doi: 10.1161/01.cir.98.16.1631
592. Lew R, Komesaroff P, Williams M, Dawood T, Sudhir K. Endogenous estrogens influence endothelial function in young men. Circ Res. (2003) 93(11):1127–33. doi: 10.1161/01.RES.0000103633.57225.BC
593. Morimoto K, Kurahashi Y, Shintani-Ishida K, Kawamura N, Miyashita M, Uji M, et al. Estrogen replacement suppresses stress-induced cardiovascular responses in ovariectomized rats. Am J Physiol Heart Circ Physiol. (2004) 287(5):H1950–6. doi: 10.1152/ajpheart.00341.2004
594. Laitinen T, Hartikainen J, Vanninen E, Niskanen L, Geelen G, Länsimies E. Age and gender dependency of baroreflex sensitivity in healthy subjects. J Appl Physiol. (1998) 84(2):576–83. doi: 10.1152/jappl.1998.84.2.576
595. Fu Q, Ogoh S. Sex differences in baroreflex function in health and disease. J Physiol Sci. (2019) 69(6):851–9. doi: 10.1007/s12576-019-00727-z
596. Corrigan FE 3rd, Al Mheid I, Eapen DJ, Hayek SS, Sher S, Martin GS, et al. Low testosterone in men predicts impaired arterial elasticity and microvascular function. Int J Cardiol. (2015) 194:94–9. doi: 10.1016/j.ijcard.2015.05.065
597. Empen K, Lorbeer R, Dörr M, Haring R, Nauck M, Gläser S, et al. Association of testosterone levels with endothelial function in men: results from a population-based study. Arterioscler Thromb Vasc Biol. (2012) 32(2):481–6. doi: 10.1161/ATVBAHA.111.232876
598. Mäkinen JI, Perheentupa A, Irjala K, Pöllänen P, Mäkinen J, Huhtaniemi I, et al. Endogenous testosterone and brachial artery endothelial function in middle-aged men with symptoms of late-onset hypogonadism. Aging Male. (2011) 14(4):237–42. doi: 10.3109/13685538.2011.593655
599. Sansone A, Rastrelli G, Cignarelli A, de Rocco Ponce M, Condorelli RA, Giannetta E, et al. Effect of treatment with testosterone on endothelial function in hypogonadal men: a systematic review and meta-analysis. Int J Impot Res. (2020) 32(4):379–86. doi: 10.1038/s41443-019-0163-6
600. Rech CM, Clapauch R, de Souza M, Bouskela E. Low testosterone levels are associated with endothelial dysfunction in oophorectomized early postmenopausal women. Eur J Endocrinol. (2016) 174(3):297–306. doi: 10.1530/EJE-15-0878
601. Worboys S, Kotsopoulos D, Teede H, McGrath B, Davis SR. Evidence that parenteral testosterone therapy may improve endothelium-dependent and -independent vasodilation in postmenopausal women already receiving estrogen. J Clin Endocrinol Metab. (2001) 86(1):158–61. doi: 10.1210/jcem.86.1.7103
602. Chinnathambi V, Balakrishnan M, Ramadoss J, Yallampalli C, Sathishkumar K. Testosterone alters maternal vascular adaptations: role of the endothelial NO system. Hypertension. (2013) 61(3):647–54. doi: 10.1161/HYPERTENSIONAHA.111.00486
603. Costa TJ, Ceravolo GS, dos Santos RA, de Oliveira MA, Araújo PX, Giaquinto LR, et al. Association of testosterone with estrogen abolishes the beneficial effects of estrogen treatment by increasing ROS generation in aorta endothelial cells. Am J Physiol Heart Circ Physiol. (2015) 308(7):H723–32. doi: 10.1152/ajpheart.00681.2014
604. Sullivan JC, Sasser JM, Pollock DM, Pollock JS. Sexual dimorphism in renal production of prostanoids in spontaneously hypertensive rats. Hypertension. (2005) 45(3):406–11. doi: 10.1161/01.HYP.0000156879.83448.93
605. Alhashim A, Abdelbary M, Sullivan JC, Naeini SE, Elmarakby AA. Sexual dimorphism in renal heme oxygenase-1 and arachidonic acid metabolizing enzymes in spontaneously hypertensive rats versus normotensive wistar Kyoto rats. Prostaglandins Other Lipid Mediat. (2022) 161:106650. doi: 10.1016/j.prostaglandins.2022.106650
606. Bernatova I, Puzserova A, Dubovicky M. Sex differences in social stress-induced pressor and behavioral responses in normotensive and prehypertensive rats. Gen Physiol Biophys. (2010) 29(4):346–54. doi: 10.4149/gpb_2010_04_346
607. Hinterdobler J, Schunkert H, Kessler T, Sager HB. Impact of acute and chronic psychosocial stress on vascular inflammation. Antioxid Redox Signal. (2021) 35(18):1531–50. doi: 10.1089/ars.2021.0153
608. Zhang T, Zhai Y, Chen Y, Zhou Z, Yang J, Liu H. Effects of emotional and physiological stress on plaque instability in apolipoprotein E knockout mice. J Physiol Biochem. (2011) 67(3):401–13. doi: 10.1007/s13105-011-0090-6
609. Heidt T, Sager HB, Courties G, Dutta P, Iwamoto Y, Zaltsman A, et al. Chronic variable stress activates hematopoietic stem cells. Nat Med. (2014) 20(7):754–8. doi: 10.1038/nm.3589
610. Bot I, Shi GP, Kovanen PT. Mast cells as effectors in atherosclerosis. Arterioscler Thromb Vasc Biol. (2015) 35(2):265–71. doi: 10.1161/ATVBAHA.114.303570
611. Krystel-Whittemore M, Dileepan KN, Wood JG. Mast cell: a multi-functional master. Cell Front Immunol. (2016) 6:620. doi: 10.3389/fimmu.2015.00620
612. Theoharides TC, Alysandratos KD, Angelidou A, Delivanis DA, Sismanopoulos N, Zhang B, et al. Mast cells and inflammation. Biochim Biophys Acta. (2012) 1822(1):21–33. doi: 10.1016/j.bbadis.2010.12.014
613. Alevizos M, Karagkouni A, Panagiotidou S, Vasiadi M, Theoharides TC. Stress triggers coronary mast cells leading to cardiac events. Ann Allergy Asthma Immunol. (2014) 112(4):309–16. doi: 10.1016/j.anai.2013.09.017
614. Lagraauw HM, Wezel A, van der Velden D, Kuiper J, Bot I. Stress-induced mast cell activation contributes to atherosclerotic plaque destabilization. Sci Rep. (2019) 9(1):2134. doi: 10.1038/s41598-019-38679-4
615. Chikahisa S, Harada S, Shimizu N, Shiuchi T, Otsuka A, Nishino S, et al. Mast cell involvement in glucose tolerance impairment caused by chronic mild stress with sleep disturbance. Sci Rep. (2017) 7(1):13640. doi: 10.1038/s41598-017-14162-w
616. Pohl CS, Medland JE, Mackey E, Edwards LL, Bagley KD, DeWilde MP, et al. Early weaning stress induces chronic functional diarrhea, intestinal barrier defects, and increased mast cell activity in a porcine model of early life adversity. Neurogastroenterol Motil. (2017) 29(11). doi: 10.1111/nmo.13118
617. Joshi A, Page CE, Damante M, Dye CN, Haim A, Leuner B, et al. Sex differences in the effects of early life stress exposure on mast cells in the developing rat brain. Horm Behav. (2019) 113:76–84. doi: 10.1016/j.yhbeh.2019.04.012
618. Bauch HJ, Grünwald J, Vischer P, Gerlach U, Hauss WH. A possible role of catecholamines in atherogenesis and subsequent complications of atherosclerosis. Exp Pathol. (1987) 31(4):193–204. doi: 10.1016/S0232-1513(87)80001-4
619. Pauletto P, Scannapieco G, Pessina AC. Sympathetic drive and vascular damage in hypertension and atherosclerosis. Hypertension. (1991) 17(4):III75–81.2013498
620. Helin P, Lorenzen I, Garbarsch C, Matthiessen ME. Arteriosclerosis in rabbit aorta induced by noradrenaline. The importance of the duration of the noradrenaline action. Atherosclerosis. (1970) 12(1):125–32. doi: 10.1016/0021-9150(70)90089-4
621. Cavallero C, Di Tondo U, Mingazzini PL, Nicosia R, Pericoli MN, Sarti P, et al. Cell proliferation in the atherosclerotic plaques of cholesterol-fed rabbits. Part 3. Histological and radioautographic observations on glucocorticoids-treated rabbits. Atherosclerosis. (1976) 25(2–3):145–52. doi: 10.1016/0021-9150(76)90020-4
622. Barnett PA, Spence JD, Manuck SB, Jennings JR. Psychological stress and the progression of carotid artery disease. J Hypertens. (1997) 15(1):49–55. doi: 10.1097/00004872-199715010-00004
623. Vasamsetti SB, Florentin J, Coppin E, Stiekema LCA, Zheng KH, Nisar MU, et al. Sympathetic neuronal activation triggers myeloid progenitor proliferation and differentiation. Immunity. (2018) 49(1):93–106.e7. doi: 10.1016/j.immuni.2018.05.004
624. Powell ND, Sloan EK, Bailey MT, Arevalo JM, Miller GE, Chen E, et al. Social stress up-regulates inflammatory gene expression in the leukocyte transcriptome via β-adrenergic induction of myelopoiesis. Proc Natl Acad Sci U S A. (2013) 110(41):16574–9. doi: 10.1073/pnas.1310655110
625. Yao BC, Meng LB, Hao ML, Zhang YM, Gong T, Guo ZG. Chronic stress: a critical risk factor for atherosclerosis. J Int Med Res. (2019) 47(4):1429–40. doi: 10.1177/0300060519826820
626. Sager HB, Dutta P, Dahlman JE, Hulsmans M, Courties G, Sun Y, et al. RNAi targeting multiple cell adhesion molecules reduces immune cell recruitment and vascular inflammation after myocardial infarction. Sci Transl Med. (2016) 8:342ra80. doi: 10.1126/scitranslmed.aaf143
627. Fantidis P. The role of the stress-related anti-inflammatory hormones ACTH and cortisol in atherosclerosis. Curr Vasc Pharmacol. (2010) 8(4):517–25. doi: 10.2174/157016110791330889
628. de Prada TP, Pozzi AO, Coronado MT, Pounchard MA, Gonzalez P, Boscá L, et al. Atherogenesis takes place in cholesterol-fed rabbits when circulating concentrations of endogenous cortisol are increased and inflammation suppressed. Atherosclerosis. (2007) 191(2):333–9. doi: 10.1016/j.atherosclerosis.2006.05.049
629. Okutsu M, Lira VA, Higashida K, Peake J, Higuchi M, Suzuki K. Corticosterone accelerates atherosclerosis in the apolipoprotein E-deficient mouse. Atherosclerosis. (2014) 232(2):414–9. doi: 10.1016/j.atherosclerosis.2013.11.076
630. Smyth GP, Stapleton PP, Freeman TA, Concannon EM, Mestre JR, Duff M, et al. Glucocorticoid pretreatment induces cytokine overexpression and nuclear factor-kappaB activation in macrophages. J Surg Res. (2004) 116(2):253–61. doi: 10.1016/S0022-4804(03)00300-7
631. Mateo T, Nabah YNA, Taha MA, Mata M, Cerda ´-Nicola ´s M, Proudfoot AEI, et al. Angiotensin II-induced mononuclear leukocyte interactions with arteriolar and venular endothelium are mediated by the release of different CC chemokines. J Immunol. (2006) 176:5577–86. doi: 10.4049/jimmunol.176.9.5577
632. Zhou H, Mehta S, Srivastava SP, Grabinska K, Zhang X, Wong C, et al. Endothelial cell-glucocorticoid receptor interactions and regulation of wnt signaling. JCI Insight. (2020) 5(3):e131384. doi: 10.1172/jci.insight.131384
633. Mateo T, Naim Abu Nabah Y, Losada M, Estellés R, Company C, Bedrina B, et al. A critical role for TNFalpha in the selective attachment of mononuclear leukocytes to angiotensin-II-stimulated arterioles. Blood. (2007) 110(6):1895–902. doi: 10.1182/blood-2007-01-070607
634. Tang YL, Jiang JH, Wang S, Liu Z, Tang XQ, Peng J, et al. TLR4/NF-κB Signaling contributes to chronic unpredictable mild stress-induced atherosclerosis in ApoE-/- mice. PLoS One. (2015) 10(4):e0123685. doi: 10.1371/journal.pone.0123685
635. Wang S, Xiaoling G, Pingting L, Shuqiang L, Yuaner Z. Chronic unpredictable mild stress combined with a high-fat diets aggravates atherosclerosis in rats. Lipids Health Dis. (2014) 13:77. doi: 10.1186/1476-511X-13-77
636. Chumaeva N, Hintsanen M, Juonala M, Raitakari OT, Keltikangas-Järvinen L. Sex differences in the combined effect of chronic stress with impaired vascular endothelium functioning and the development of early atherosclerosis: the cardiovascular risk in young Finns study. BMC Cardiovasc Disord. (2010) 10:34. doi: 10.1186/1471-2261-10-34
637. Shivpuri S, Gallo LC, Crouse JR, Allison MA. The association between chronic stress type and C-reactive protein in the multi-ethnic study of atherosclerosis: does gender make a difference? J Behav Med. (2012) 35(1):74–85. doi: 10.1007/s10865-011-9345-5
638. von Känel R, Mills PJ, Fainman C, Dimsdale JE. Effects of psychological stress and psychiatric disorders on blood coagulation and fibrinolysis: a biobehavioral pathway to coronary artery disease? Psychosom Med. (2001) 63(4):531–44. doi: 10.1097/00006842-200107000-00003
639. Brydon L, Magid K, Steptoe A. Platelets, coronary heart disease, and stress. Brain Behav Immun. (2006) 20(2):113–9. doi: 10.1016/j.bbi.2005.08.002
640. Samad Z, Boyle S, Ersboll M, Vora AN, Zhang Y, Becker RC, et al. REMIT Investigators. Sex differences in platelet reactivity and cardiovascular and psychological response to mental stress in patients with stable ischemic heart disease: insights from the REMIT study. J Am Coll Cardiol. (2014) 64(16):1669–78. doi: 10.1016/j.jacc.2014.04.087
641. Dong T, Cheng YW, Yang F, Sun PW, Zhu CJ, Zhu L, et al. Chronic stress facilitates the development of deep venous thrombosis. Oxid Med Cell Longev. (2015) 2015:384535. doi: 10.1155/2015/384535
642. Jin X, Jin C, Nakamura K, Jin T, Xin M, Wan Y, et al. Increased dipeptidyl peptidase-4 accelerates chronic stress-related thrombosis in a mouse carotid artery model. J Hypertens. (2020) 38(8):1504–13. doi: 10.1097/HJH.0000000000002418
643. Schins A, Honig A, Crijns H, Baur L, Hamulyák K. Increased coronary events in depressed cardiovascular patients: 5-HT2A receptor as missing link? Psychosom Med. (2003) 65(5):729–37. doi: 10.1097/01.psy.0000088596.42029.10
644. Serra-Millàs M. Are the changes in the peripheral brain-derived neurotrophic factor levels due to platelet activation? World J Psychiatry. (2016) 6(1):84–101. doi: 10.5498/wjp.v6.i1.84
645. Austin AW, Wissmann T, von Kanel R. Stress and hemostasis: an update. Semin Thromb Hemost. (2013) 39(8):902–12. doi: 10.1055/s-0033-1357487
646. Chen S, Du C, Shen M, Zhao G, Xu Y, Yang K, et al. Sympathetic stimulation facilitates thrombopoiesis by promoting megakaryocyte adhesion, migration, and proplatelet formation. Blood. (2016) 127(8):1024–35. doi: 10.1182/blood-2015-07-660746
647. von Känel R. Acute mental stress and hemostasis: when physiology becomes vascular harm. Thromb Res. (2015) 135(Suppl 1):S52–5. doi: 10.1016/S0049-3848(15)50444-1
648. Sandrini L, Ieraci A, Amadio P, Zarà M, Barbieri SS. Impact of acute and chronic stress on thrombosis in healthy individuals and cardiovascular disease patients. Int J Mol Sci. (2020) 21(21):7818. doi: 10.3390/ijms21217818
649. Karamouzis I, Berardelli R, D'Angelo V, Fussotto B, Zichi C, Giordano R, et al. Enhanced oxidative stress and platelet activation in patients with cushing's syndrome. Clin Endocrinol (Oxf). (2015) 82(4):517–24. doi: 10.1111/cen.12524
650. Frimerman A, Miller HI, Laniado S, Keren G. Changes in hemostatic function at times of cyclic variation in occupational stress. Am J Cardiol. (1997) 79(1):72–5. doi: 10.1016/s0002-9149(96)00680-7
651. Pozzi AO, Bernardo E, Coronado MT, Punchard MA, González P, Fantidis P. Acute arterial thrombosis in the absence of inflammation: the stress-related anti-inflammatory hormone ACTH participates in platelet-mediated thrombosis. Atherosclerosis. (2009) 204(1):79–84. doi: 10.1016/j.atherosclerosis.2008.08.023
652. Stämpfli SF, Camici GG, Keller S, Rozenberg I, Arras M, Schuler B, et al. Restraint stress enhances arterial thrombosis in vivo–role of the sympathetic nervous system. Stress. (2014) 17(1):126–32. doi: 10.3109/10253890.2013.862616
653. Lande K, Kjeldsen SE, Os I, Westheim A, Hjermann I, Eide I, et al. Increased platelet and vascular smooth muscle reactivity to low-dose Adrenaline infusion in mild essential hypertension. J Hypertens. (1988) 6:219–25. doi: 10.1097/00004872-198803000-00006
654. Golaszewska A, Misztal T, Marcinczyk N, Chabielska E, Rusak T. Adrenaline May contribute to prothrombotic condition via augmentation of platelet procoagulant response, enhancement of fibrin formation, and attenuation of fibrinolysis. Front Physiol. (2021) 12:657881. doi: 10.3389/fphys.2021.657881
655. Shimbo D, Child J, Davidson K, Geer E, Osende JI, Reddy S, et al. Exaggerated serotonin-mediated platelet reactivity as a possible link in depression and acute coronary syndromes. Am J Cardiol. (2002) 89(3):331–3. doi: 10.1016/s0002-9149(01)02236-6
656. Hüfner K, Koudouovoh-Tripp P, Kandler C, Hochstrasser T, Malik P, Giesinger J, et al. Differential changes in platelet reactivity induced by acute physical compared to persistent mental stress. Physiol Behav. (2015) 151:284–91. doi: 10.1016/j.physbeh.2015.07.021
657. Williams MS, Ziegelstein RC, McCann UD, Gould NF, Ashvetiya T, Vaidya D. Platelet serotonin signaling in patients with cardiovascular disease and comorbid depression. Psychosom Med. (2019) 81(4):352–62. doi: 10.1097/PSY.0000000000000689
658. Taliaz D, Loya A, Gersner R, Haramati S, Chen A, Zangen A. Resilience to chronic stress is mediated by hippocampal brain-derived neurotrophic factor. J Neurosci. (2011) 31(12):4475–83. doi: 10.1523/JNEUROSCI.5725-10.2011
659. Sandrini L, Ieraci A, Amadio P, Veglia F, Popoli M, Lee FS, et al. Sub-Chronic stress exacerbates the pro-thrombotic phenotype in BDNFVal/met mice: gene-environment interaction in the modulation of arterial thrombosis. Int J Mol Sci. (2018) 19(10):3235. doi: 10.3390/ijms19103235
660. Sandrini L, Ieraci A, Amadio P, Popoli M, Tremoli E, Barbieri SS. Apocynin prevents abnormal megakaryopoiesis and platelet activation induced by chronic stress. Oxid Med Cell Longev. (2017) 2017:9258937. doi: 10.1155/2017/9258937
661. Duan L, Rao X, Xia C, Rajagopalan S, Zhong J. The regulatory role of DPP4 in atherosclerotic disease. Cardiovasc Diabetol. (2017) 16(1):76. doi: 10.1186/s12933-017-0558-y
662. Izzi B, Tirozzi A, Cerletti C, Donati MB, de Gaetano G, Hoylaerts MF, et al. Beyond haemostasis and thrombosis: platelets in depression and its co-morbidities. Int J Mol Sci. (2020) 21(22):8817. doi: 10.3390/ijms21228817
663. Morais-Silva G, Costa-Ferreira W, Gomes-de-Souza L, Pavan JC, Crestani CC, Marin MT. Cardiovascular outcomes related to social defeat stress: new insights from resilient and susceptible rats. Neurobiol Stress. (2019) 11:100181. doi: 10.1016/j.ynstr.2019.100181
664. Costa-Ferreira W, Vieira JO, Almeida J, Gomes-de-Souza L, Crestani CC. Involvement of type 1 angiontensin II receptor (AT1) in cardiovascular changes induced by chronic emotional stress: comparison between homotypic and heterotypic stressors. Front Pharmacol. (2016) 7:262. doi: 10.3389/fphar.2016.00262
665. Schroeder EB, Liao D, Chambless LE, Prineas RJ, Evans GW, Heiss G. Hypertension, blood pressure, and heart rate variability: the atherosclerosis risk in communities (ARIC) study. Hypertension. (2003) 42(6):1106–11. doi: 10.1161/01.HYP.0000100444.71069.73
666. Kilit C, Pasali Kilit T, Onrat E. Autonomic modulation in hypertension without hypertrophy. Acta Cardiol. (2015) 70(6):721–7. doi: 10.2143/AC.70.6.3120186
667. Alter P, Grimm W, Vollrath A, Czerny F, Maisch B. Heart rate variability in patients with cardiac hypertrophy–relation to left ventricular mass and etiology. Am Heart J. (2006) 151(4):829–36. doi: 10.1016/j.ahj.2005.06.016
668. Mandawat MK, Wallbridge DR, Pringle SD, Riyami AA, Latif S, Macfarlane PW, et al. Heart rate variability in left ventricular hypertrophy. Br Heart J. (1995) 73(2):139–44. doi: 10.1136/hrt.73.2.139
669. Hill LK, Watkins LL, Hinderliter AL, Blumenthal JA, Sherwood A. Racial differences in the association between heart rate variability and left ventricular mass. Exp Physiol. (2017) 102(7):764–72. doi: 10.1113/EP086228
670. Tsuji H, Larson MG, Venditti FJ Jr, Manders ES, Evans JC, Feldman CL, et al. Impact of reduced heart rate variability on risk for cardiac events. The framingham heart study. Circulation. (1996) 94(11):2850–5. doi: 10.1161/01.cir.94.11.2850
671. Lakusic N, Mahovic D, Sonicki Z, Slivnjak V, Baborski F. Outcome of patients with normal and decreased heart rate variability after coronary artery bypass grafting surgery. Int J Cardiol. (2013) 166(2):516–8. doi: 10.1016/j.ijcard.2012.04.040
672. Buccelletti E, Gilardi E, Scaini E, Galiuto L, Persiani R, Biondi A, et al. Heart rate variability and myocardial infarction: systematic literature review and metanalysis. Eur Rev Med Pharmacol Sci. (2009) 13(4):299–307.19694345
673. Liu W, Wang X, Mei Z, Gong J, Gao X, Zhao Y, et al. Chronic stress promotes the progression of pressure overload-induced cardiac dysfunction through inducing more apoptosis and fibrosis. Physiol Res. (2015) 64(3):325–34. doi: 10.33549/physiolres.932778
674. Shanks J, Abukar Y, Lever NA, Pachen M, LeGrice IJ, Crossmand DJ. Reverse re-modelling chronic heart failure by reinstating heart rate variability. Basic Res Cardiol. (2002) 117:4. doi: 10.1007/s00395-022-00911-0
675. Ghantous CM, Azrak Z, Hanache S, Abou-Kheir W, Zeidan A. Differential role of leptin and adiponectin in cardiovascular system. Int J Endocrinol. (2015) 2015:534320. doi: 10.1155/2015/534320
676. Zhao S, Kusminski CM, Scherer PE. Adiponectin, leptin and cardiovascular disorders. Circ Res. (2021) 128(1):136–49. doi: 10.1161/CIRCRESAHA.120.314458
677. Na KS, Kim EK, Park JT. Decreased plasma adiponectin among male firefighters with symptoms of post-traumatic stress disorder. J Affect Disord. (2017) 221:254–8. doi: 10.1016/j.jad.2017.06.015
678. Llorente R, Miguel-Blanco C, Aisa B, Lachize S, Borcel E, Meijer OC, et al. Long term sex-dependent psychoneuroendocrine effects of maternal deprivation and juvenile unpredictable stress in rats. J Neuroendocrinol. (2011) 23(4):329–44. doi: 10.1111/j.1365-2826.2011.02109.x
679. Milaneschi Y, Lamers F, Bot M, Drent ML, Penninx BW. Leptin dysregulation is specifically associated with Major depression with atypical features: evidence for a mechanism connecting obesity and depression. Biol Psychiatry. (2017) 81(9):807–14. doi: 10.1016/j.biopsych.2015.10.023
680. Komesaroff PA, Esler MD, Sudhir K. Estrogen supplementation attenuates glucocorticoid and catecholamine responses to mental stress in perimenopausal women. J Clin Endocrinol Metab. (1999) 84(2):606–10. doi: 10.1210/jcem.84.2.5447
681. Ndzie Noah ML, Adzika GK, Mprah R, Adekunle AO, Adu-Amankwaah J, Sun H. Sex-Gender disparities in cardiovascular diseases: the effects of estrogen on eNOS, lipid profile, and NFATs during catecholamine stress. Front Cardiovasc Med. (2021) 8:639946. doi: 10.3389/fcvm.2021.639946
682. Ueyama T, Hano T, Kasamatsu K, Yamamoto K, Tsuruo Y, Nishio I. Estrogen attenuates the emotional stress-induced cardiac responses in the animal model of tako-tsubo (ampulla) cardiomyopathy. J Cardiovasc Pharmacol. (2003) 42(Suppl 1):S117–9. doi: 10.1097/00005344-200312001-00024
683. Ueyama T, Ishikura F, Matsuda A, Asanuma T, Ueda K, Ichinose M, et al. Chronic estrogen supplementation following ovariectomy improves the emotional stress-induced cardiovascular responses by indirect action on the nervous system and by direct action on the heart. Circ J. (2007) 71(4):565–73. doi: 10.1253/circj.71.565
684. Cao X, Zhou C, Chong J, Fu L, Zhang L, Sun D, et al. Estrogen resisted stress-induced cardiomyopathy through increasing the activity of β₂AR-gαs signal pathway in female rats. Int J Cardiol. (2015) 187:377–86. doi: 10.1016/j.ijcard.2015.02.113
685. Singh T, Khan H, Gamble DT, Scally C, Newby DE, Dawson D. Takotsubo syndrome: pathophysiology, emerging concepts, and clinical implications. Circulation. (2022) 145(13):1002–19. doi: 10.1161/CIRCULATIONAHA.121.055854
686. Murakami T, Yoshikawa T, Maekawa Y, Ueda T, Isogai T, Sakata K, et al. Gender differences in patients with takotsubo cardiomyopathy: multi-center registry from Tokyo CCU network. PLoS One. (2015) 10(8):e0136655. doi: 10.1371/journal.pone.0136655
687. Mori H, Ishikawa S, Kojima S, Hayashi J, Watanabe Y, Hoffman JI, et al. Increased responsiveness of left ventricular apical myocardium to adrenergic stimuli. Cardiovasc Res. (1993) 27(2):192–8. doi: 10.1093/cvr/27.2.192
688. Möller C, Stiermaier T, Meusel M, Jung C, Graf T, Eitel I. Microcirculation in patients with takotsubo syndrome-the prospective CIRCUS-TTS study. J Clin Med. (2021) 10(10):2127. doi: 10.3390/jcm10102127
689. Wittstein IS. Why age matters in takotsubo syndrome. J Am Coll Cardiol. (2020) 75(16):1878–81. doi: 10.1016/j.jacc.2020.03.030
690. Scally C, Abbas H, Ahearn T, Srinivasan J, Mezincescu A, Rudd A, et al. Myocardial and systemic inflammation in acute stress-induced (takotsubo) cardiomyopathy. Circulation. (2019) 139(13):1581–92. doi: 10.1161/CIRCULATIONAHA.118.037975
691. Godsman N, Kohlhaas M, Nickel A, Cheyne L, Mingarelli M, Schweiger L, et al. Metabolic alterations in a rat model of takotsubo syndrome. Cardiovasc Res. (2022) 118(8):1932–46. doi: 10.1093/cvr/cvab081
692. Dawson DK, Neil CJ, Henning A, Cameron D, Jagpal B, Bruce M, et al. Tako-Tsubo cardiomyopathy: a heart stressed out of energy? JACC Cardiovasc Imaging. (2015) 8(8):985–7. doi: 10.1016/j.jcmg.2014.10.004
693. Wilson HM, Cheyne L, Brown PAJ, Kerr K, Hannah A, Srinivasan J, et al. Characterization of the myocardial inflammatory response in acute stress-induced (takotsubo) cardiomyopathy. JACC Basic Transl Sci. (2018) 3(6):766–78. doi: 10.1016/j.jacbts.2018.08.006
694. Neil C, Nguyen TH, Kucia A, Crouch B, Sverdlov A, Chirkov Y, et al. Slowly resolving global myocardial inflammation/oedema in tako-tsubo cardiomyopathy: evidence from T2-weighted cardiac MRI. Heart. (2012) 98(17):1278–84. doi: 10.1136/heartjnl-2011-301481
695. Murakami T, Komiyama T, Matsumoto S, Kajiwara H, Kobayashi H, Ikari Y. Examination of gender differences in patients with takotsubo syndrome according to left ventricular biopsy: two case reports. J Med Case Rep. (2021) 15(1):281. doi: 10.1186/s13256-021-02856-9
696. Xinxing W, Wei L, Lei W, Rui Z, Baoying J, Lingjia Q. A neuroendocrine mechanism of co-morbidity of depression-like behavior and myocardial injury in rats. PLoS One. (2014) 9(2):e88427. doi: 10.1371/journal.pone.0088427
697. Mercanoglu G, Safran N, Uzun H, Eroglu L. Chronic emotional stress exposure increases infarct size in rats: the role of oxidative and nitrosative damage in response to sympathetic hyperactivity. Methods Find Exp Clin Pharmacol. (2008) 30(10):745–52. doi: 10.1358/mf.2008.30.10.1316822
698. Wang Y, Zhang H, Chai F, Liu X, Berk M. The effects of escitalopram on myocardial apoptosis and the expression of bax and bcl-2 during myocardial ischemia/reperfusion in a model of rats with depression. BMC Psychiatry. (2014) 14:349. doi: 10.1186/s12888-014-0349-x
699. Wang Y, Liu X, Zhang D, Chen J, Liu S, Berk M. The effects of apoptosis vulnerability markers on the myocardium in depression after myocardial infarction. BMC Med. (2013) 11:32. doi: 10.1186/1741-7015-11-32
700. Grippo AJ, Santos CM, Johnson RF, Beltz TG, Martins JB, Felder RB, et al. Increased susceptibility to ventricular arrhythmias in a rodent model of experimental depression. Am J Physiol Heart Circ Physiol. (2004) 286(2):H619–26. doi: 10.1152/ajpheart.00450.2003
701. Du Toit EF, Tai WS, Cox A, O'Connor D, Griffith TA, Helman T, et al. Synergistic effects of low-level stress and a western diet on metabolic homeostasis, mood, and myocardial ischemic tolerance. Am J Physiol Regul Integr Comp Physiol. (2020) 319(3):R347–357. doi: 10.1152/ajpregu.00322.2019
702. Helman TJ, Headrick JP, Peart JN, Stapelberg NJC. Central and cardiac stress resilience consistently linked to integrated immuno-neuroendocrine responses across stress models in male mice. Eur J Neurosci. (2022) 56(4):4333–62. doi: 10.1111/ejn.15747
703. Headrick JP, Peart JN, Budiono BP, Shum DHK, Neumann DL, Stapelberg NJC. The heartbreak of depression: ‘psycho-cardiac’ coupling in myocardial infarction. J Mol Cell Cardiol. (2017) 106:14–28. doi: 10.1016/j.yjmcc.2017.03.007
704. Zhuo C, Wang Y, Wang X, Wang Y, Chen Y. Cardioprotection by ischemic postconditioning is abolished in depressed rats: role of akt and signal transducer and activator of transcription-3. Mol Cell Biochem. (2011) 346(1–2):39–47. doi: 10.1007/s11010-010-0589-0
705. Scott SR, Singh K, Yu Q, Sen CK, Wang M. Sex as biological Variable in cardiac mitochondrial bioenergetic responses to acute stress. Int J Mol Sci. (2022) 23(16):9312. doi: 10.3390/ijms23169312
706. Pope BS, Wood SK. Advances in understanding mechanisms and therapeutic targets to treat comorbid depression and cardiovascular disease. Neurosci Biobehav Rev. (2020) 116:337–49. doi: 10.1016/j.neubiorev.2020.06.031
707. Hollis F, Pope BS, Gorman-Sandler E, Wood SK. Neuroinflammation and mitochondrial dysfunction link social stress to depression. Curr Top Behav Neurosci. (2022) 54:59–93. doi: 10.1007/7854_2021_300
708. Vásquez-Trincado C, García-Carvajal I, Pennanen C, Parra V, Hill JA, Rothermel BA, et al. Mitochondrial dynamics, mitophagy and cardiovascular disease. J Physiol. (2016) 594(3):509–25. doi: 10.1113/JP271301
709. Salnikova D, Orekhova V, Grechko A, Starodubova A, Bezsonov E, Popkova T, et al. Mitochondrial dysfunction in vascular wall cells and its role in atherosclerosis. Int J Mol Sci. (2021) 22(16):8990. doi: 10.3390/ijms22168990
710. Poznyak AV, Ivanova EA, Sobenin IA, Yet SF, Orekhov AN. The role of mitochondria in cardiovascular diseases. Biology (Basel). (2020) 9(6):137. doi: 10.3390/biology9060137
711. Uchikado Y, Ikeda Y, Ohishi M. Current understanding of the pivotal role of mitochondrial dynamics in cardiovascular diseases and senescence. Front Cardiovasc Med. (2022) 9:905072. doi: 10.3389/fcvm.2022.905072
712. Panatto JP, Jeremias IC, Ferreira GK, Ramos AC, Rochi N, Gonçalves CL, et al. Inhibition of mitochondrial respiratory chain in the brain of rats after hepatic failure induced by Acetaminophen. Mol Cell Biochem. (2011) 350(1–2):149–54. doi: 10.1007/s11010-010-0689-x
713. Madrigal JL, Olivenza R, Moro MA, Lizasoain I, Lorenzo P, Rodrigo J, et al. Glutathione depletion, lipid peroxidation and mitochondrial dysfunction are induced by chronic stress in rat brain. Neuropsychopharmacology. (2001) 24(4):420–9. doi: 10.1016/S0893-133X(00)00208-6
714. Psarra AM, Sekeris CE. Glucocorticoid receptors and other nuclear transcription factors in mitochondria and possible functions. Biochim Biophys Acta. (2009) 1787(5):431–6. doi: 10.1016/j.bbabio.2008.11.011
715. Shaw GA. Mitochondria as the target for disease related hormonal dysregulation. Brain Behav Immun Health. (2021) 18:100350. doi: 10.1016/j.bbih.2021.100350
716. Shimamoto A, Rappeneau V. Sex-dependent mental illnesses and mitochondria. Schizophr Res. (2017) 187:38–46. doi: 10.1016/j.schres.2017.02.025
717. Dykens JA, Simpkins JW, Wang J, Gordon K. Polycyclic phenols, estrogens and neuroprotection: a proposed mitochondrial mechanism. Exp Gerontol. (2003) 38(1–2):101–7. doi: 10.1016/s0531-5565(02)00162-6
718. Nilsen J, Diaz Brinton R. Mechanism of estrogen-mediated neuroprotection: regulation of mitochondrial calcium and bcl-2 expression. Proc Natl Acad Sci U S A. (2003) 100(5):2842–7. doi: 10.1073/pnas.0438041100
719. Simpkins JW, Yang SH, Sarkar SN, Pearce V. Estrogen actions on mitochondria–physiological and pathological implications. Mol Cell Endocrinol. (2008) 290(1–2):51–9. doi: 10.1016/j.mce.2008.04.013
720. Irwin RW, Yao J, Hamilton RT, Cadenas E, Brinton RD, Nilsen J. Progesterone and estrogen regulate oxidative metabolism in brain mitochondria. Endocrinology. (2008) 149(6):3167–75. doi: 10.1210/en.2007-1227
721. Zheng J, Ramirez VD. Rapid inhibition of rat brain mitochondrial proton F0F1-ATPase activity by estrogens: comparison with na+, K+ -ATPase of porcine cortex. Eur J Pharmacol. (1999) 368(1):95–102. doi: 10.1016/s0014-2999(99)00012-6
722. Wang M, Smith K, Yu Q, Miller C, Singh K, Sen CK. Mitochondrial connexin 43 in sex-dependent myocardial responses and estrogen-mediated cardiac protection following acute ischemia/reperfusion injury. Basic Res Cardiol. (2019) 115(1):1. doi: 10.1007/s00395-019-0759-5
723. Sripetchwandee J, Pintana H, Sa-Nguanmoo P, Boonnag C, Pratchayasakul W, Chattipakorn N, et al. Comparative effects of sex hormone deprivation on the brain of insulin-resistant rats. J Endocrinol. (2019):JOE-18-0552.R2. doi: 10.1530/JOE-18-0552
724. Apaiajai N, Chunchai T, Jaiwongkam T, Kerdphoo S, Chattipakorn SC, Chattipakorn N. Testosterone deprivation aggravates left-ventricular dysfunction in male obese insulin-resistant rats via impairing cardiac mitochondrial function and dynamics proteins. Gerontology. (2018) 64(4):333–43. doi: 10.1159/000487188
725. Minta W, Palee S, Mantor D, Sutham W, Jaiwongkam T, Kerdphoo S, et al. Estrogen deprivation aggravates cardiometabolic dysfunction in obese-insulin resistant rats through the impairment of cardiac mitochondrial dynamics. Exp Gerontol. (2018) 103:107–14. doi: 10.1016/j.exger.2018.01.006
726. Shinlapawittayatorn K, Pongkan W, Sivasinprasasn S, Chattipakorn SC, Chattipakorn N. Sexual dimorphism in cardiometabolic and cardiac mitochondrial function in obese rats following sex hormone deprivation. Nutr Diabetes. (2022) 12(1):11. doi: 10.1038/s41387-022-00189-0
727. Pereira SP, Tavares LC, Duarte AI, Baldeiras I, Cunha-Oliveira T, Martins JD, et al. Sex-dependent vulnerability of fetal nonhuman primate cardiac mitochondria to moderate maternal nutrient reduction. Clin Sci (Lond). (2021) 135(9):1103–26. doi: 10.1042/CS20201339
Keywords: sympathetic nervous system, takotsubo cardiomyopathy, chronic stress, sexual dimophism, glucocortcoids, coronary artery disease
Citation: Helman TJ, Headrick JP, Stapelberg NJC and Braidy N (2023) The sex-dependent response to psychosocial stress and ischaemic heart disease. Front. Cardiovasc. Med. 10:1072042. doi: 10.3389/fcvm.2023.1072042
Received: 17 October 2022; Accepted: 3 April 2023;
Published: 21 April 2023.
Edited by:
Hamidreza Goodarzynejad, University Health Network, CanadaReviewed by:
Bruno B. Lima, Harvard Medical School, United States© 2023 Helman, Headrick, Stapelberg and Braidy. This is an open-access article distributed under the terms of the Creative Commons Attribution License (CC BY). The use, distribution or reproduction in other forums is permitted, provided the original author(s) and the copyright owner(s) are credited and that the original publication in this journal is cited, in accordance with accepted academic practice. No use, distribution or reproduction is permitted which does not comply with these terms.
*Correspondence: Tessa J. Helman dC5oZWxtYW5AdW5zdy5lZHUuYXU=
Specialty Section: This article was submitted to Sex and Gender in Cardiovascular Medicine, a section of the journal Frontiers in Cardiovascular Medicine
Disclaimer: All claims expressed in this article are solely those of the authors and do not necessarily represent those of their affiliated organizations, or those of the publisher, the editors and the reviewers. Any product that may be evaluated in this article or claim that may be made by its manufacturer is not guaranteed or endorsed by the publisher.
Research integrity at Frontiers
Learn more about the work of our research integrity team to safeguard the quality of each article we publish.