- Institute of Interdisciplinary Medical Science, Shanghai University of Traditional Chinese Medicine, Shanghai, China
According to traditional Chinese medicine (TCM), chronic heart failure has the basic pathological characteristics of “heart-kidney yang deficiency.” Chronic heart failure with heart- and kidney-Yang deficiency has good overlap with New York Heart Association (NYHA) classes III and IV. Traditional Chinese medicine classical prescriptions for the treatment of chronic heart failure often take “warming and tonifying kidney-Yang” as the core, supplemented by herbal compositions with functions of “promoting blood circulation and dispersing blood stasis.” Nowadays, there are still many classical and folk prescriptions for chronic heart failure treatment, such as Zhenwu decoction, Bushen Huoxue decoction, Shenfu decoction, Sini decoction, as well as Qili Qiangxin capsule. This review focuses on classical formulations and their active constituents that play a key role in preventing chronic heart failure by suppressing inflammation and modulating immune and neurohumoral factors. In addition, given that mitochondrial metabolic reprogramming has intimate relation with inflammation, cardiac hypertrophy, and fibrosis, the regulatory role of classical prescriptions and their active components in metabolic reprogramming, including glycolysis and lipid β-oxidation, is also presented. Although the exact mechanism is unknown, the classical TCM prescriptions still have good clinical effects in treating chronic heart failure. This review will provide a modern pharmacological explanation for its mechanism and offer evidence for clinical medication by combining TCM syndrome differentiation with chronic heart failure clinical stages.
Introduction
The word “heart failure” has been recorded in the ancient Chinese medicine book “Huangdi Neijing” more than 2,300 years ago. According to the combination of different traditional Chinese medicine (TCM) syndrome elements, TCM can divide chronic heart failure into 4–6 TCM syndromes (1), which are mainly related to deficiency of heart and kidney yang and heart qi deficiency. The report of Shi et al. (2) compared the main TCM syndromes of chronic heart failure with the New York Heart Association (NYHA) classification, and found that there is a certain correlation between NYHA classification and the distribution of heart-kidney yang deficiency and heart-qi deficiency. Heart-qi deficiency is basically concentrated in categories I and II, and the frequency of kidney yang deficiency and body fluid retention in class III+IV is higher than that in class I and II. Obviously, in the NYHA classification, heart-kidney-yang deficiency is the stage where heart failure progresses into a more severe stage (2). Common classic and folk prescriptions for the treatment of chronic heart failure include Zhenwu decoction, Lingguizhugan decoction, Sini decoction, Shenfu decoction, Bushen Huoxue decoction, and Qili Qiangxin capsule, etc. (Table 1). Their effectiveness on chronic heart failure has been proved by increasing clinical investigations (3–5). They are superior to Western medicine alone in terms of treatment efficacy, whether judged by TCM symptom efficacy or by NYHA functional classification (Supplementary Table 1). The RCRI/Lee's score, 6MWD, LVEF, and CCE were also better to western medicine alone on the endpoints of chronic heart failure (Supplementary Table 1). In terms of blood parameters related to cardiac function and inflammation, such as NT-proBNP, cTnI, sST2, CK-MB, and hs-CRP, the combination of classical prescriptions also showed more favorable improvement in chronic heart failure (Supplementary Table 1).
The occurrence and development of chronic heart failure includes a variety of processes, which are related to inflammation of heart tissue, participation of immune cells (macrophages, lymphocytes, and neutrophils, etc.), regulation of neurohumoral factors, and programmed cell death (apoptosis, pyroptosis, etc.), myocardial hypertrophy, and cardiac fibrosis (6–8). Experimental investigations in animals have confirmed that a lot of classic prescriptions for tonifying kidney can improve inflammation (9, 10), oxidative stress and cellular injury (11), inhibit cardiomyocyte death (10, 11), and myocardial hypertrophy (12–14), regulate neurohumoral factors (15, 16), and alleviate cardiac fibrosis (17–20). Accumulating studies have been reported that (1) Lingguizhugan decoction and Zhenwu decoction prevent cardiac hypertrophy by inhibiting MAP kinase activity (12, 13); (2) Lingguizhugan decoction improves ventricular hypertrophy by restraining NLRP3/Caspase-1/GSDMD inflammation and pyroptosis pathways (10); (3) Lingguizhugan decoction also antagonizes oxidative stress injury through the Nrf2/Keap1/HO-1 pathway (11); (4) Shenfu recipe mitigates heart failure by activating AMPK-mediated metabolism of fatty acids and glucose in the heart (17); (5) Shenfu injection enhances eNOS activity through PI3K/Akt signaling pathway, promotes vasodilation and improves microcirculation of failing heart (21); (6) Sini Decoction significantly decreases the levels of hs-CRP, TNF-α, IL-6, and IL-1β in rat myocardial tissue, reduces the levels of renin, angiotensin II, aldosterone, ANP, and ET-1 in plasma, and limits the vascular inflammation of the heart (9, 15); (7) Sini decoction also reduces TLR-2/4 and TGF-β1 levels to improve early ventricular remodeling and cardiac function after myocardial infarction (18); (8) Qili Qiangxin capsule alleviates cardiac remodeling by inhibiting TGF-β1/Smad3 and NF-κB signaling pathways (19).
The levels of circulating pro-inflammatory biomarkers are closely related to disease severity and prognosis in patients with heart failure. Despite disappointing clinical trial results inflammation is still considered to be a major pathophysiological factor contributing to chronic heart failure, including heart failure with reduced ejection fraction and heart failure with preserved ejection fraction (6, 22). Moreover, inflammation and metabolic reprogramming are mutually regulated objects. For example, LPS can enhance the activity of HIF-1 and participate in the induction of glycolysis and pro-inflammatory genes, especially IL-1β. In contrast, the tricarboxylic acid cycle (TAC) intermediate, succinic acid, and itaconic acid, a derivative of citric acid, have pro- and anti-inflammatory effects, respectively (23–25). Therefore, metabolic reprogramming during heart failure has become a hotspot of research in recent years. Given that mitochondria are key subcellular organelles of cardiomyocytes, important discoveries have been made in mitochondrial energy, glucose, fatty acid, and amino acid metabolic reprogramming (also known as metabolic remodeling) in heart failure, which will be targets for next-generation treatments (26, 27). Heart failure is also a bioenergetic disease. In addition to cardiac hypertrophy (28, 29), metabolic reprogramming also occurs during cardiac fibrosis (30, 31). Last but not the least, neurohumoral factors, such as the renin-angiotensin-aldosterone system, are inducers of chronic heart failure and low-grade inflammation. Indeed, antagonism of neurohormonal systems is a key therapy for heart failure. In brief, this review focuses on the modern medical characteristics of classic kidney-tonifying prescriptions for the treatment of chronic heart failure, and discusses the classic and modern pharmacological mechanisms of active ingredients on inflammation, neurohumoral factors, and metabolic reprogramming.
Immunity and inflammation in the heart- and kidney-Yang deficiency
Insufficient tissue oxygen and nutrient supply, accumulated harmful metabolites and cell death are the main mechanisms of heart failure with reduced ejection fraction (HFrEF). HFrEF happens because of chronic low-grade inflammation and immune activation, tissue fibrosis, and remodeling, decreased ventricular compliance caused by chronic myocardial machinery overwork. The importance of inflammation in heart failure has been summarized, analyzed, and prospected by many excellent reviews (32–34), and we will not repeat them here. Now many kinds of immune cells, such as NK cells, T cells, B cells, monocytes, macrophages, are known to participate in the process of heart failure. CD4+ T lymphocytes are progressively augmented and broadly activated in circulation, failing heart and spleen, and are also necessary for pathological left ventricular remodeling in chronic heart failure (35). Treg, CD4+, CD8+ T cells, and the ratio of CD4+ to CD8+ T cells are expected to be biomarkers of heart failure (36). The plasticity of monocytes and the heterogeneity of monocyte-derived macrophages have important implications for cardiac remodeling. After myocardial injury, the medulla and extramedullary hematopoiesis enlarges, the recruitment of cardiac monocytes CCR2+ increases, and the enhanced cell wall tension stimulates the proliferation of local macrophages in the heart (37). Otherwise, patients with advanced heart failure have a high IL-6 level produced by peripheral blood monocytes, a low level of circulating NK cell number, and impaired cytolytic function of NK cells on IL-2 and IL-12. Furthermore, the impaired lytic function is closely related to the level of IL-6 produced by unstimulated peripheral blood mononuclear cells (38).
According to previous reports, inflammation and immune disorders are important factors for heart failure with kidney-yang deficiency. Many Chinese herbal compounds can alleviate these inflammation and immune system disorders. The following are some research reports. After Shenfu Injection was used to treat 56 patients with heart failure and Kidney-Yang deficiency, the increased levels of CD4+CD25+Foxp3+Treg and CD4+ T cells were suppressed, while the low level of IL-10 was elevated (39). In an early clinical study of 57 elderly people with kidney deficiency, the percentage of OKT4/CD4+ in the elderly was significantly lower than that of the control group, whereas OKT8/CD8+ was remarkably increased, and as a result the ratio of OKT4/OKT8 was also markedly decreased compared with the control group (40). Furthermore, their immunological changes have also been confirmed in rat and mouse models of kidney-Yang deficiency (Supplementary Table 2). Many cellular immune parameters are suppressed, such as thymus index, spleen index, T lymph and B lymph stimulation index, NK cell activity, macrophage phagocytosis, etc. (Supplementary Table 2). As the number of CD4+ cells decreases and the number of CD8+ cells increases, the ratio of CD4+/CD8+ is also reduced. The serum levels of IL-4 and the ratio of IL-4/IFN-γ are greatly elevated (Supplementary Table 2), yet the trend of the serum IL-2 and IFN-γ levels are contrary to the above. A variety of prescriptions for improving kidney-Yang deficiency, such as anti-aging tablet, Jinkui Shenqi pill and Yougui pill, reversed these cellular and humoral immune indicators (Supplementary Table 2). Kidney-Yang deficiency aggravates the inflammatory response of the heart, including the activated NF-κB pathway of myocardium, the elevated activities of ERK1/2, p38, and JNK in the tissues, the upregulated JAK2/STAT3 signal, and the unbalanced homeostasis of endoplasmic reticulum stress (19, 41–44). The application of prescriptions for resisting kidney-Yang deficiency can reverse the failure of heart function (Figure 1).
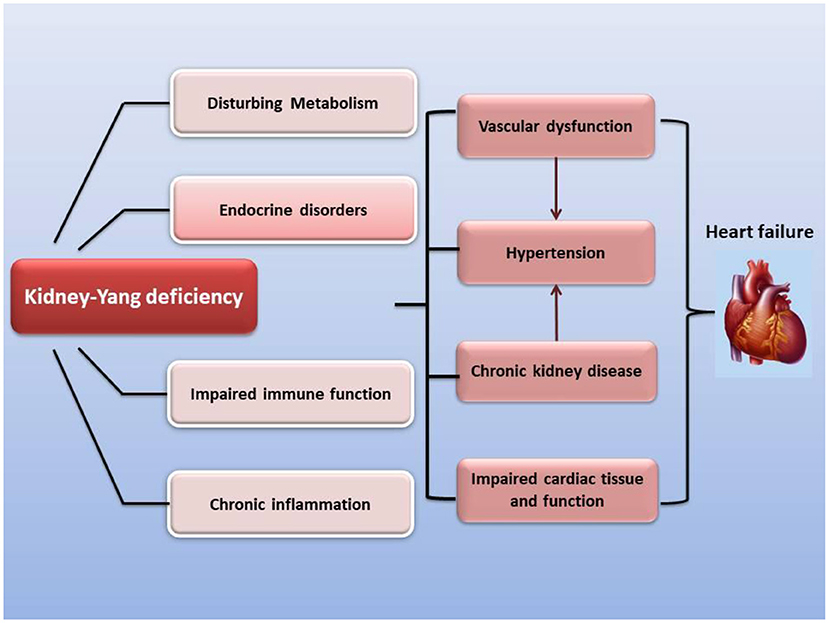
Figure 1. The modern medical understanding that kidney-Yang deficiency promotes the development of chronic heart failure. Kidney-Yang deficiency leads to metabolic disturbances, endocrine disorders, immune function imbalance, and low-grade chronic inflammation, which further contributes to vascular dysfunction, hypertension, chronic kidney disease, and cardiac tissue and functional imbalance, and eventually guides to chronic heart failure.
In the past 3 years, many studies have explored the mechanism of Zhenwu decoction on chronic heart failure by using network pharmacology methods, which revealed many signaling pathways, key target genes and key active ingredients of Zhenwu decoction. These studies have found that Zhenwu Decoction has multiple key signaling pathways for improving heart failure, including inflammation-related MAPK, NF-κB, IL-17, TNF-α, HIF-1α, TLR, and PPARG signaling pathways, as well as platelet activation and arachidonic acid metabolism. It also involves apoptosis, p53 signaling, adrenergic signaling in cardiomyocytes, renin-angiotensin system, and Insulin resistance related to myocardial damage, function, and fibrosis, in addition to calcium signaling and cGMP-PKG signaling related to cardiac contraction and relaxation (Supplementary Table 3). In the search for key target genes, the authors found that inflammation-related IL-6, TNF-α, PTGS2, PPARG, IKBKB, oxidative stress-related GSTP1, GSTM1, GSTM2, cell death-related CASP3, CASP8, CASP9, BCL2, and various receptors that affected myocardial function, such as adrenoceptor alpha subtype (ADRA2A, ADRA2B, and ADRA1A), androgen receptor (AR), estrogen receptor (ESR1), angiotensin II receptor 1 (AGTR1), epidermal growth factor receptor (EGFR), and cholinergic receptor muscarinic 1 (CHRM1), were targeted by Zhenwu decoction (Supplementary Table 3). Moreover, β-sitosterol, stigmasterol, kaempferol, norcoclaurine, 3β-acetoxyatractylone, paeoniflorin, (+)-catechin, hederagenin, and trametenolic acid were identified as key active ingredients of Zhenwu decoction (Supplementary Table 3). These findings indicated that the main active components in Zhenwu decoction can effectively act on key genes related to heart failure through molecular docking detection (Supplementary Table 3).
Chinese herbal medicinal ingredients for warming and tonifying kidney-Yang target involved in anti-inflammatory and cardiac function homeostasis
Traditional Chinese medicine herbs for warming and tonifying kidney-Yang account for a majority of all traditional and folk prescriptions for chronic heart failure treatment. Therefore, this section will discuss the effects of their active components on anti-inflammatory and immunomodulation. The main active ingredients are listed in Supplementary Table 4.
Higenamine, an active component of Aconitum carmichaeli, has shown a positive effect on improving chronic heart failure. Higenamine enhanced contractility of failing hearts and improved energy metabolism in cardiomyocytes, inhibited TGF-β1/Smad signaling transduction, thereby reducing cardiac fibrosis caused by cardiac fibroblast proliferation (45, 46). The activation of the NF-κB increases the production of inflammatory cytokines and chemokines. In previous studies, higenamine inhibited the activation of NF-κB, the expression of COX-2, iNOS, TNF-α, and IL-6, and the production of PGE2 and NO through LPS and IL-1β stimulation (47–49). Similarly, monkshood polysaccharide also had the effect of inhibiting inflammation by blocking NF-κB pathway, and prevented the expression of iNOS and inflammatory cytokines (TNF-α, IL-1β, and IL-6) (50). Macrophages and lymphocytes are the most common immune cells. In a murine model of spinal cord injury, Higenamine was found to promote the activation of M2 macrophages and reduce the production of HMGB1 through HO-1 induction (51). Other studies have also shown that monkshood polysaccharides inhibited the proliferation of splenic lymphocytes induced by LPS or ConA in vivo and in vitro, and the production of antibody in vivo in mice (52). Cinnamaldehyde, an important active ingredient of Cinnamomum cassia Presl., is also often used as a condiment in beverages, cakes, and perfumes. Previous studies have supported that Cinnamaldehyde attenuated cardiac hypertrophy and fibrosis caused by pressure overload (53) and acute myocardial ischemia induced by isoproterenol (54). Cardiac hypertrophy is a systemic chronic inflammation, while myocardial ischemia initiates an intense inflammatory response. The possible mechanisms by which cinnamaldehyde protects the heart are anti-inflammation. Numerous studies have demonstrated that cinnamaldehyde regulates the NF-κB, p38, JNK, and Jak/Stat pathways to inhibit expressions of IL-6, IL-8, and TNF-α induced by IL-1β (55) and MCP-1, MMP-2, and LOX-1 induced by ox-LDL (56). Cinnamaldehyde also ameliorated LPS-induced cardiac dysfunction by inhibiting ROS production and autophagy through TLR4-NOX4 pathway (57), suppressed fructose-induced cardiac inflammation and fibrosis by attenuating CD36-mediated TLR4/6 and IL-1R-associated kinase 4/1 signaling (58). In addition, the accumulation of succinate in cytoplasm stimulated HIF-1α activity, which activated the expression of NLRP3. Cinnamaldehyde significantly reduced synovial inflammation in rheumatoid arthritis rats by blocking the maturation of IL-1β derived from NLRP3 (59). Cornuside and morroniside are major active components of Cornus officinalis. Cornuside and morroniside have been reported to reduce heart damage caused by ligation of anterior descending coronary artery or ischemia-reperfusion (60, 61). The underlying mechanisms are that cornuside and morroniside significantly inhibit the expression of iNOS and COX-2 induced by LPS, and production of TNF-α, IL-1β, IL-6, NO, and PGE2 through the regulation of TLR4/NF-κB, ERK1/2, p38, JNK, STAT3, and Nrf2/HO-1 signaling pathways (62–64).
Psoraleae Fructus is a Traditional Chinese herb with the effect of warming kidney and enhancing yang. It contains terpenoids, coumarins, isopentenyl flavonoids, chalcones, and other active components, such as bakuchiol, psoralen, isopsoralen, corylifol A (also known as corylinin), neobavisoflavone, and isobavachalcone. It has been reported that bakuchiol in studies can attenuate myocardial ischemia-reperfusion injury, pathological myocardial hypertrophy, and heart failure (65, 66). Bakuchiol, psoralen, and isopsoralen demonstrated the effect of stimulating vasodilation through endothelium-dependent and endothelium-independent pathways (67). Other active constituents in Psoraleae Fructus such as Psoralen, corylifol A, neobavisoflavone, and bakuchiol inhibited the expression and secretion of TNF-α, IL-1β, and IL-6 (68). Bakuchiol also blocked NF-κB pathway activity (68).
Epimedium, Eucommia, Cistanche, and Cnidium are all important herbs for warming and tonifying kidney-Yang. Many studies have revealed that their active ingredients, such as icariin, icariside II, aucubin, echinacoside, and osthole improved myocardial hypertrophy, remodeling and fibrosis induced by pressure overload (69–73), or myocardial injury and remodeling caused by isoproterenol (74, 75). Moreover, icariin, syringaresinol, and osthole also prevented myocardial ischemia/reperfusion injury (76–78), and aucubin significantly reduced myocardial infarction-induced cardiac remodeling (79). Overall, accumulating reports indicate that icariin, icariside II, aucubin, echinacoside, and osthole all inhibited the activity of NF-κB (71, 73, 80–84). Other reports also pointed out that icariin blocked JNK (80), echinacoside prevented STAT3 (83), and echinacoside and aucubin reduced activity of inflammasome NLRP3 (75, 85). To sum up, the active ingredients of herbs for warming and tonifying Kidney-Yang basically demonstrated anti-inflammatory ability.
Effect of prescriptions on changes of sympathetic excitability and neurohumoral factors in chronic heart failure
Sympathetic hyperactivity is an identified hallmark of chronic heart failure. Cardiac sympathetic afferent reflex (CSAR), a sympathetic excitatory reflex with positive feedback characteristics, can be activated by endogenous substances during chronic heart failure, such as Ang II, AT1 receptor, NF-κB, and NADPH oxidase pathways (86–88). The pathological enhancement of CSAR is one of the major reasons for sympathetic excitation in chronic heart failure. The paraventricular nucleus (PVN) of the hypothalamus is an important component of the central pathway of the CSAR, and also plays a key center for integration of neuroendocrine and sympathetic nerve activities. The subfornical organ (SFO), as a periventricular structure, senses circulating IL-1β, TNF-α, and Ang II, elevates sympathetic excitability and transmits it to the downstream PVN (89–91). In addition, Ang II has been reported to promote the release and synthesis of PVN's excitatory neurotransmitter norepinephrine (92), enhancing the plasma norepinephrine level and eventually leading to impaired cardiac function in heart failure (90, 93). Furthermore, compared with normal rats, the norepinephrine gene expression and plasma concentration in the hypothalamus of rats with Yang deficiency reached a high level, suggesting its sympathetic hyperactivity (94). Two tonifying kidney-Yang prescriptions, Qili Qiangxin Capsule and Bushen Ningxin Granule, can downregulate the expression and secretion of norepinephrine in the hypothalamus due to heart failure or anxiety (95, 96) (Figure 2). For example, the 20(S)-protopanaxadiol of Ginseng in Qili Qiangxin Capsule, and the cedrol of Boziren in Bushen Ningxin Granules, reduce levels of norepinephrine in the hippocampus of rodents (97, 98) (Supplementary Table 5).
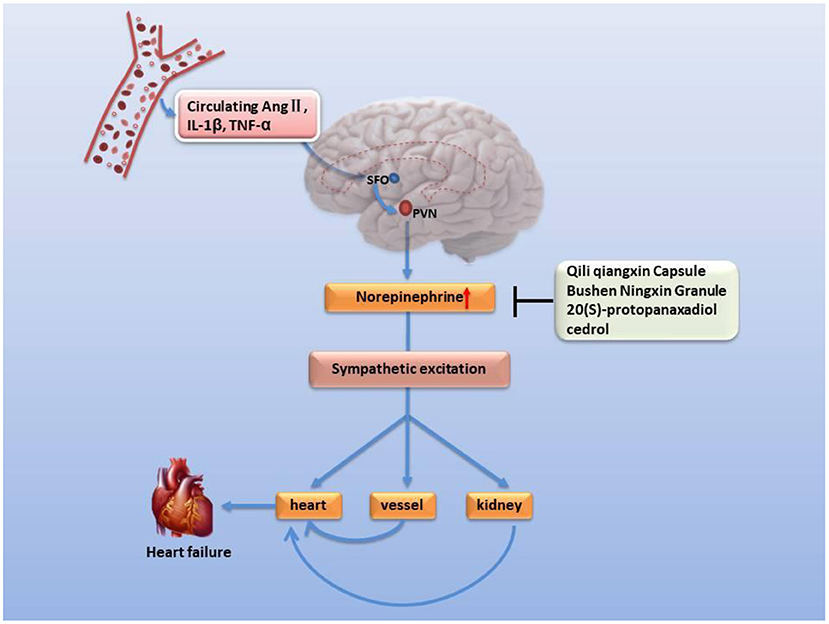
Figure 2. Inhibitory effect of classic formulations and their active ingredients on norepinephrine secretion and neural excitability in the hypothalamus. Circulating pro-inflammatory factors enhance sympathetic excitability and the risk of heart failure. SFO's perception of circulating proinflammatory factors leads to increased secretion of norepinephrine in PVN neurotransmitters and promotes sympathetic excitability, resulting in microvascular contraction, renal fluid retention and increased renin release, raising the level of angiotensin II and aldosterone in blood, elevating heart rate and arrhythmia, and causing left ventricular hypertrophy.
Corticotropin-releasing hormone (CRH) is one of a variety of neuroendocrine hormones secreted by PVN. The expression of CRH in PVN of rats with ischemia-induced heart failure is elevated with the increased expression of pro-inflammatory cytokines and pro-inflammatory genes, even up to two times (99, 100). Corticotropin-releasing hormone has been reported to enhance the excitability of hypothalamic sympathetic nerves in heart failure (101). However, the function of the hypothalamic-pituitary-adrenal axis decreases with age, especially in very elderly patients with heart failure, the gene expression and secretion of related neurohumoral declines, such as CRH (102, 103), 11β-HSD2 (104). Due to negative feedback, the expression and activity of mineralocorticoid receptor (MR) increased instead (105). Moreover, it is worth noting that chronic heart failure patients based on NYHA classes III and IV have a higher proportion of hypothermia (106, 107), anorexia (108, 109), depression (110, 111), and a poor prognosis, which is similar to the phenomenon of kidney-Yang deficiency of TCM (112) or a lower response of ACTH and cortisol (102, 113). Many classic prescriptions for warming and nourishing kidney-Yang, such as Sini decoction reverses hypothermia and balances hypothalamic-pituitary-adrenal axis in vivo (114–117) and improve chronic heart failure (38, 114, 118) (Figure 3). Urotensin II is a homolog of corticotropin-releasing factor, and its tissue UTR2 distribution is highly consistent with its receptor (119, 120). The activity of urotensin is mainly regulated by autocrine and paracrine mechanisms. Urotensin is a strong vasoconstrictor and proinflammatory mediator in patients with heart failure, which can lead to cardiac fibrosis, myocardial hypertrophy, and remodeling (121–124). These characteristics can explain the clinical findings related to heart failure. The plasma urotensin level of patients with heart failure is much higher than healthy individuals (125, 126). Studies have revealed that the traditional prescription Shenfu injection and Fuling Sini decoction effectively reduce the plasma urotensin levels in patients with chronic heart failure (127, 128). In addition, vasopressin (AVP) is another neurohumoral factor, which is secreted by magnocellular neurosecretory neurons in the hypothalamic supraoptic nucleus and PVN, and regulates blood pressure, hyponatremia, and edema associated with heart failure (129, 130). According to the dialectical classification of TCM, the expression levels of AVP and AQP-2 are much higher in patients with Yang deficiency and water retention syndrome (equivalent to NYHA class III+IV) than in those with both Qi and Yin deficiency syndrome (equivalent to NYHA class II) (131). The warming Yang prescriptions, such as Qili Qiangxin capsule, Shenfu injection, and Zhenwu decoction, can reduce plasma AVP levels, AVP V2 receptor and AQP-2 expression in renal tissue, and improve hemodynamics and cardiac function (16, 132, 133).
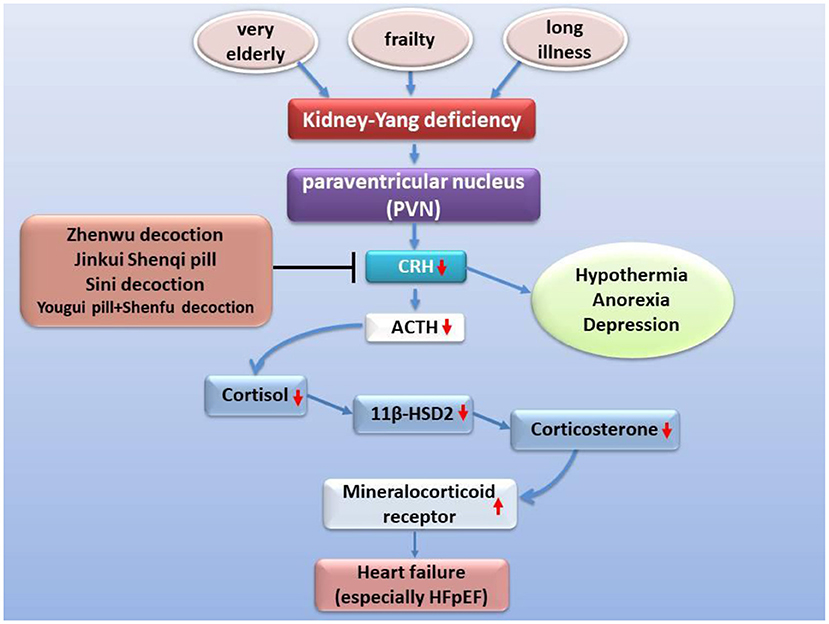
Figure 3. Improvement of heart failure by classic prescription via HPA axis. Kidney Yang deficiency is linked to heart failure via the hypothalamic-pituitary-adrenal axis. Under the condition of kidney-Yang deficiency driven by factors such as advanced age, the secretion of CRH secreted by PVN decreases, the activation of the hypothalamic-pituitary-adrenal axis is inhibited, the expression of 11β-HSD2 drops while the expression of mineralocorticoid receptor (MR) rises, cardiac hypertrophy and fibrosis, and functional events increased.
The renin-AngII-aldosterone system is an endocrine axis that maintains blood pressure and body fluid homeostasis. Homeostatic imbalance is directly involved in the various processes required for the progression of heart failure: oxidative stress, inflammation, cardiac hypertrophy, cell death, and tissue fibrosis. Multiple clinical investigations of hospitalized patients have found that elderly patients (average age about 73 years) with heart failure and kidney-Yang deficiency have higher plasma AngII level, slightly lower left ventricular ejection fraction (EF) and fractional shortening (FS), and much lower left ventricular diastolic function (E/A ratio) than heart failure patients with non-kidney-Yang deficiency (2, 5, 134). Moreover, patients with kidney-Yang deficiency are mostly distributed in NYHA class III and IV, and non-kidney-Yang deficiency exists in NYHA class II (2, 5, 134). It has been proved in animal experiments that a variety of herbal formulas, including Sini decoction, Qili Qiangxin capsules, and Shenfu Qiangxin Mixture, can effectively reduce the plasma Ang II and aldosterone level in rats in a dose-dependent manner (38, 135–137).
Abnormal energy metabolism in kidney-Yang deficiency
It was reported many years ago that pro-inflammatory stimuli inhibited Myc activity and cell proliferation, and participated in the enhancement of HIF-1α-dependent transcriptional programs and glycolysis (138). HIF-1α was induced in LPS-activated macrophages, which contribute to the induction of pro-inflammatory genes, especially IL-1β. The HIF-1α induction mechanism involved succinic acid, an intermediate product of the TAC with pro-inflammatory effects. HIF-1α also regulated pyruvate kinase M2 (PKM2) function (23). Metabolic reprogramming under inflammation is multifaceted. Sun et al. (25) summarized two main features of metabolic reprogramming of inflammation. First, it increased glycolysis and decreased lipid oxidative phosphorylation, which accelerated ATP production and biosynthesis; second, it showed epigenetic reprogramming to promote histone acetylation and inhibit DNA methylation, which is based on changes in the activity of acetyl and methyl donors and their metabolic enzymes. Fatty acid oxidation of myocardial mitochondria is the main source of ATP production. In the inflammatory state of heart failure, myocardial fatty acid uptake and mitochondrial oxidation are impaired, and energy metabolism is abnormal (Figure 1). The increased production of ATP by glycolysis and ketone oxidation was compensated to some extent (139). The changes in energy metabolism that lead to the reduction of the efficiency of the failing heart are due to the transcriptional changes of the key enzymes of the metabolic pathway and epigenetic changes (27). Na+/K+-ATPase and Ca2+-ATPase are important pumps to maintain the homeostasis of intracellular sodium and potassium levels and the excitability of cardiomyocytes, and they are also the main energy consumers of myocardium. A lot of evidence shows that glycolysis and Na+/K+-ATPase pumps are coupled in function, which provides ATP fuel for the pump (140, 141). As shown in Supplementary Table 6, the deficiency of kidney yang leads to disorders of energy metabolism. In animals with Kidney-Yang deficiency, the Na+/K+-ATPase and Ca2+-ATPase activities in the liver, skeletal muscle and myocardium were reduced (Supplementary Table 6), while the mitochondrial ATP production decreased and AMP increased in the liver and myocardium (Supplementary Table 6). The activities of SDH and respiratory chain complexes I, II, and III in the liver, skeletal muscle, and myocardium of kidney-Yang deficiency rat models also declined (Supplementary Table 6). The mitochondrial protein expressions and urine metabolite levels changed both in rat models and patients with kidney-Yang deficiency symptoms (Supplementary Table 6). A recent study showed that 27 metabolites involving amino acid, energy, ketone body, fatty acid, and methane metabolism changed significantly with the treatment of Yougui pill, a classic prescription for kidney-Yang deficiency (Supplementary Table 6).
The mechanism of active ingredients regulating metabolic reprogramming in heart failure
Inflammation induces reprogramming of cellular energy production and biosynthesis to ensure faster ATP production and biosynthesis for defense response and damage repair by increasing glycolysis and reducing fatty acid β-oxidation and promoting mitochondrial glutaminolysis (25). Now, many studies have revealed that glycolysis promoted inflammation, and that inflammation and glycolysis formed an interdependent and mutually promoting relationship (142–144), and on the contrary, controlling glycolysis reversely regulated inflammation (145). Metabolic remodeling precedes occurrence of cardiac hypertrophy, and more importantly, metabolic remodeling promotes cardiac hypertrophy (145, 146). Changes in cardiac energy metabolism promoted the development of heart failure and increased the severity of heart failure (147, 148). In patients with heart failure, the protein expression of the TAC enzymes and the pyruvate dehydrogenase complex subunits of the heart are up-regulated, and the expression of the proteins related to fatty acid oxidation and oxidative phosphorylation complex are down-regulated, which indicated that the substantial metabolic switch from free fatty acid oxidation to glycolysis in heart failure (149, 150). Numerous studies have determined that hexokinase 2 (HK2) (151, 152), PKM2 (153, 154), and lactate dehydrogenase A (LDHA) (155, 156) are key genes involved in glycolysis (Figure 4). Moreover, glycolysis is regulated by transcription factors such as HIF-1α (23, 157), mTOR (158, 159), STAT3 (160, 161), c-Myc (162, 163) (Figure 5), and others. Alteration of mitochondrial fatty acid oxidation is another side of metabolic reprogramming. The change of heart fatty acid oxidation varies with the type of heart failure. Fatty acid oxidation of myocardium increases in diabetes and obesity related heart failure, and decreases in hypertensive heart failure related to ischemia. Earlier reports pointed out that the activity of the long-chain fatty acid transporter carnitine palmitoyltransferase I (CPT1) in the hypertrophic heart was lower than that in the heart of control animals (164). As the rate-limiting step of mitochondrial β-oxidation, the CPT1 deficiency exacerbates cardiac hypertrophy caused by pressure overload (165) Moreover, inhibition of CPT1 induced myocardial hypertrophy and premature death in mice (166). Specific expression of acyl-CoA synthase-1 (ACSL1) in the mouse heart promoted the synthesis of long-chain fatty acids (LCFA), contributed to the oxidation of LCFA, reduced the lipotoxicity caused by transverse aortic constriction stress, and delayed progressive heart remodeling and failure (167). Long-chain 3-hydroxyacyl-CoA dehydrogenase (LCAD) is a β-oxidation rate-limiting enzyme. In the hearts of LCAD deleted mice, acetyl carnitine was extremely insufficient, which resulted in severe cardiac energy deficiency, and the mice developed cardiac hypertrophy and decreased left ventricular ejection fraction and diastolic filling rate (168–170) (Figure 6). Studies have reported that the HIF-1 transcription factor, which is sensitive to hypoxia, inhibited the expression of CPT1 and LCAD, and controlled fatty acid metabolism (171, 172). Cardiac fibrosis is the core pathology of heart failure. Inflammation and specific pro-fibrotic factors, such as transforming growth factor-β, contribute to the accumulation of extracellular matrix in the heart and the proliferation of cardiac fibroblasts (173, 174). Glutaminolysis is a part of metabolic reprogramming and is required for tissue fibrosis (175, 176). The differentiation and activation of fibroblasts induced by TGF-β1 includes glutaminolysis (177). Inhibition of glutaminase 1, a key enzyme of glutaminolysis in fibroblasts, attenuates tissue fibrosis (178). In addition, many reports point out that glutaminolysis is the driver of pulmonary hypertension and cardiac remodeling (179, 180). c-Myc is believed to be involved in the development of heart failure (181, 182) and the regulation of glutaminolysis (183, 184). The basic pathology of inflammation and metabolic reprogramming exist in heart failure. In recent years, accumulated evidence has gradually established the knowledge that heart failure includes the underlying pathology of inflammation and the phenomenon of metabolic reprogramming, which provides new pharmacological targets (Figures 4–7).
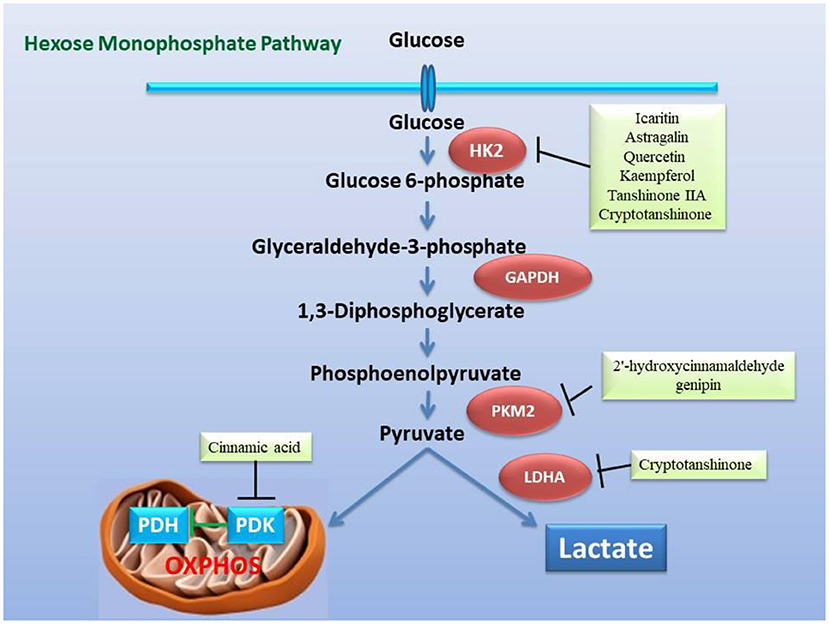
Figure 4. Inhibitory effect of the active ingredients of the classic formulas of invigorating kidney on the key enzymes related to glycolysis in the mitochondrial hexose pathway caused by heart failure. The active ingredients in the classic formulas have inhibitory effects on the enzymatic activity or expression of HK2, PKM2, LDHA, and PDK, respectively.
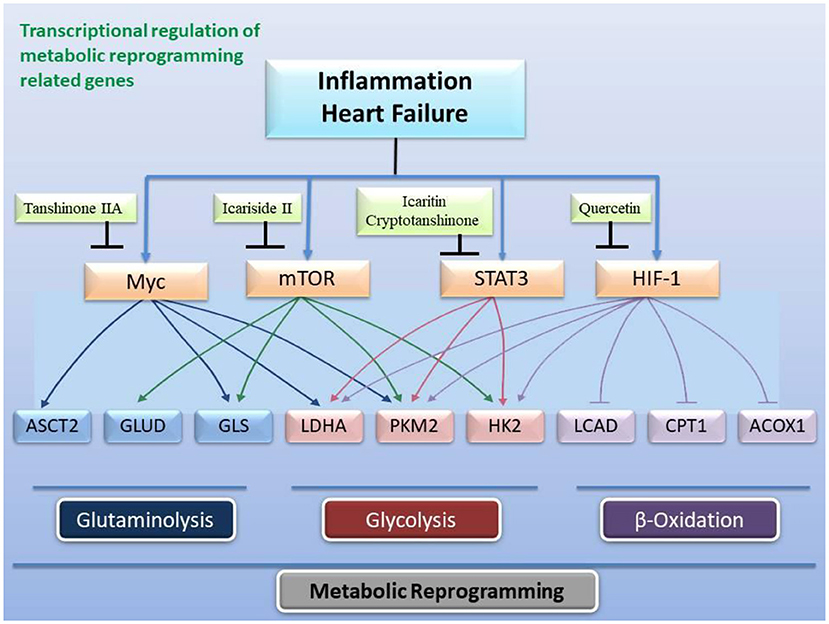
Figure 5. Transcriptional regulation of gene expression related to metabolic reprogramming by active ingredients in classical formulas. Heart failure and inflammation trigger the activity of transcription factors c-Myc, mTOR, STAT3, and HIF-1 to change the expression and activity of enzymes related to glycolysis, fatty acid β-oxidation, and glutaminolysis.
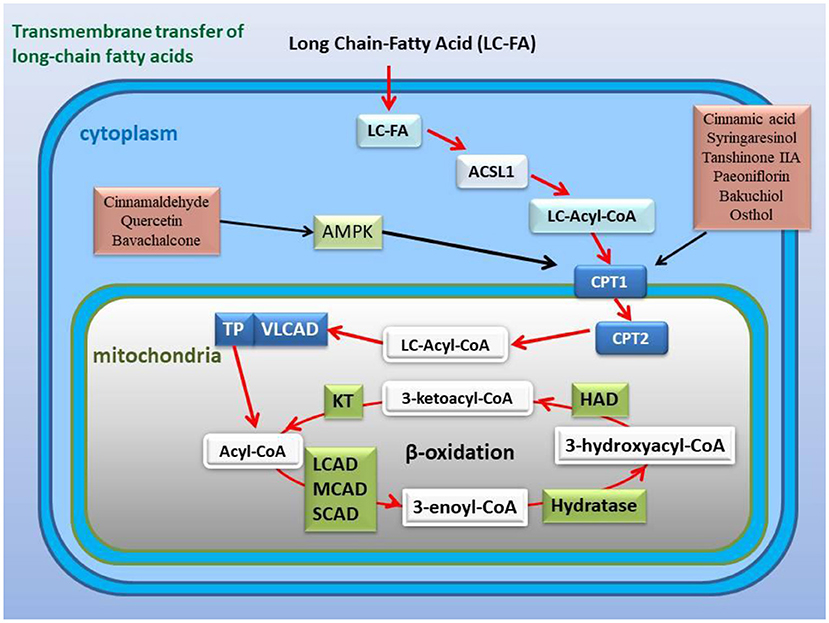
Figure 6. Active ingredients in the classic formulas activate the expression or activity of CPT1 and improve fatty acid β-oxidation with or without the AMPK pathway. CPT1 is a key channel for long-chain fatty acids across the mitochondrial outer membrane, and its activity or expression affects the β-oxidation of fatty acids.
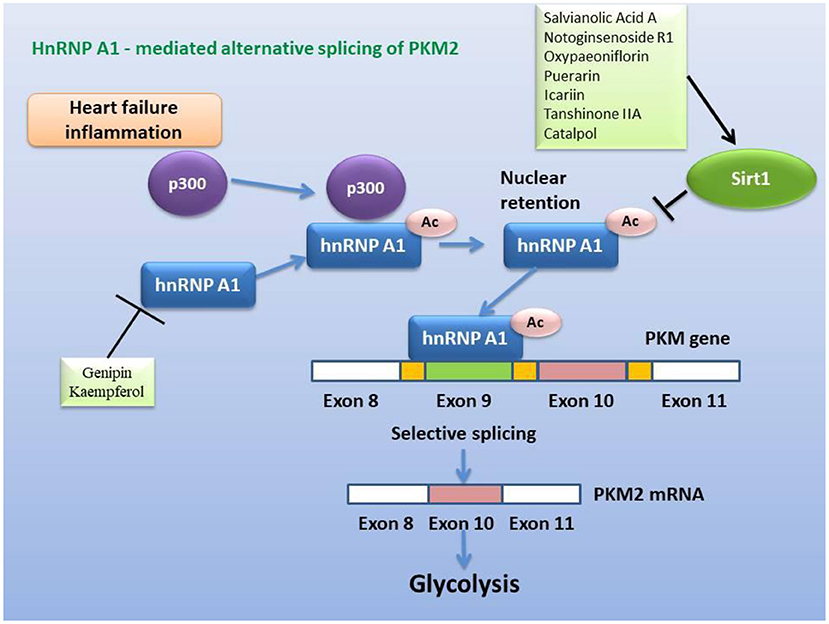
Figure 7. Inhibitory effect of active ingredients in classical recipes on hnRNP A1-mediated alternative splicing of PKM2, either directly or via activation of Sirt1. The heterogeneous nuclear ribonucleoprotein, which packs newly produced precursor mRNA, is acetylated and stabilized under conditions of heart failure and inflammation, and then selectively splices PKM2 mRNA to promote glycolysis.
In view of the fact that metabolic remodeling precedes and promotes the occurrence of myocardial hypertrophy, the inhibition of metabolic remodeling will contribute to the improvement of myocardial hypertrophy and heart failure. Animal and cell experiments have confirmed that the active ingredients of many herbs for “warming and tonifying Kidney-Yang” and “promoting blood circulation and dispersing blood stasis” from classic prescriptions have the effect of inhibiting metabolic reprogramming. Cinnamomum cassia is an important herbal medicine for warming and tonifying Kidney-Yang, whose active ingredients have the ability to inhibit glycolysis. Cinnamic acid blocks pyruvate dehydrogenase kinase activity (185), while 2'-hydroxycinnamaldehyde ameliorates PKM2-STAT3 signaling (186) (Figure 4). Epimedium brevicornum has been widely used in warming and nourishing kidney-yang for centuries. Its component icariside II acts as a natural inhibitor of mTOR to correct abnormal energy homeostasis (187), while icaritin inhibits IL-6-induced STAT3 pathway and HK2 expression (188) (Figure 5). Genipin, an active ingredient of Eucommia ulmoides, is also an inhibitor of UCP2. Studies have pointed out that genipin prevents the expression of glycolysis-related genes hnRNPA2/B1 and PKM2 (189, 190) (Figure 7). The active components of dodder seeds, such as astragalin, quercetin, and kaempferol, also play a role in reducing the expression of HK2 and improving glycolysis (191–193) (Figure 4). A recent study indicated that Kaempferol also reversed aerobic glycolysis by modulating hnRNPA1-PKM2 axis (194) (Figure 7). Besides, quercetin has also been found to stabilize HIF-1α, reduce the formation of heterodimers with β subunits (HIF-1β/ARNT), and inhibit aerobic glycolysis against glucose fluctuations (195, 196) (Figure 5). Salvia miltiorrhaza is an important herb for promoting blood circulation and removing blood stasis. Its main active components tanshinone IIA and cryptotanshinone have good anti-inflammatory effects (197–203). Tanshinone IIA inhibits HK2-mediated glycolysis, glucose consumption, and lactic acid production, blocks the effect of c-Myc conduction (200) (Figure 5). Cryptotanshinone down-regulates the expression of glycolysis-related genes, such as GLUT1, LDHA, and HK2, also cuts off STAT3-mediated glycolysis (201, 202) (Figure 5). Moreover, rosmarinic acid, is one of the components of Salvia miltiorrhiza, also has the effect of inhibiting glycolysis (203, 204). In addition, many other active ingredients of kidney-tonifying and blood-activating herbs, such as bergapten, baohuoside I, ligustilide, isoliquiritigenin, and curcumin, all reveal inhibitory effects on glycolysis (205–209) (Figure 4).
CPT-1 is a key restriction enzyme for LCFA β-oxidation. In inflammation and sepsis, the activity of myocardial CPT-1 is inhibited, which is a target of oxidative modification and a marker of myocardial dysfunction (210). Studies have shown that the active ingredients of Ramulus Cinnamomi, cinnamic acid, and syringaresinol, up-regulate the expression of CPT-1 through activating AMPK and PPARβ signaling (211, 212) (Figure 6). CPT-1 is a downstream gene regulated by AMPK pathway (213, 214). Activating blood herbal components, paeoniflorin from Paeonia suffruticosa and Paeonia veitchii, and tanshinone IIA from Salvia miltiorrhiza, restored the expression of CPT-1 through AMPK signaling pathway (215, 216) (Figure 6). Many components of kidney-tonifying herbs activate AMPK activity, such as cinnamaldehyde, quercetin, and bavachalcone (217–219), indicating that these active components may regulate fatty acids β-oxidation (Figure 6). The psoralen extract and the active ingredient bakuchiol, from kidney-tonifying herbal Psoraleae Fructus, reversed reverse the decline in the expression of PGC-1α and CPT-1 caused by cell senescence (220). Similar to the above examples, osthol, the active component of Cnidium monnieri, also has been reported to decrease SREBP-1c and increase CPT-1α expression (221) (Figure 6).
Metabolic reprogramming is a significant feature of failing hearts. As a biomarker of the Warburg effect, the activity of PKM2 is enhanced (222). While PKM2 maintains the aerobic glycolysis pathway, PKM2 also plays a key role in glutaminolysis (153, 223). Because the differentiation and activation of fibroblasts induced by TGF-β1 includes glutaminolysis (177), the prevention of glutaminolysis can contribute to antagonize cardiac fibrosis. However, there are few reports on glutaminolysis inhibitors, only tanshinone IIA and curcumin were found to down-regulate PKM2 expression (209, 224).
Conclusion and expectations
Deficiency of heart- and kidney-Yang is the key pathogenesis of chronic heart failure, which reflects the more serious pathological phenotype of heart failure. According to NYHA's classification of heart failure, patients with kidney-Yang deficiency are mainly elderly and distributed in classes III + IV. Warming and invigorating kidney-Yang has become an important TCM therapy for chronic heart failure. Numerous classic and folk prescriptions embody the therapeutic cogitation of warming and tonifying kidney-Yang. An important modern pharmacological mechanism of this therapy is anti-inflammatory, neurohumoral regulation, and maintaining the homeostasis of cardiac function. Given that metabolic reprogramming is closely linked to inflammation, cardiac hypertrophy, and fibrosis, abnormal energy metabolism is a reflection of the inflammatory phenotype in chronic heart failure on metabolic reprogramming. The key components of classic tonifying-kidney prescriptions can regulate the energy metabolism abnormality of heart- and kidney-yang deficiency, and modulate the expression and activity of enzyme genes in glycolysis and fatty acid β-oxidation pathways. These discussions provide a modern pharmacological interpretation of the mechanism of tonifying-kidney therapy in the treatment of chronic heart failure.
At present, the design of clinical studies on chronic heart failure with classic prescriptions for kidney-tonifying lacks a multi-center, randomized, double-blind, placebo-controlled study based on standard treatment and parallel groups, and the production of classic prescriptions used lacks standardization, which requires major improvements. Besides, network pharmacology and protein target discovery of animal experiments on chronic heart failure with classic kidney-invigorating prescriptions and active ingredients need further improvements to discover and screen core targets, and finally fully elucidate the molecular mechanism of classic kidney-invigorating prescriptions on chronic heart failure treatment. Metabolic reprogramming is an important mechanism of chronic heart failure, which is linked to inflammation, cardiac hypertrophy, and fibrosis. Considering the different pathological mechanisms between HFpEF and HFrEF, it is of great clinical significance to study the classical formulations and their active components on the metabolic phenotypes, underlying molecular mechanisms, and potential therapeutic targets of fatty acid oxidation, glucose oxidation, and ketone body oxidation in chronic failing hearts.
Author contributions
LC: conceptualization, data collection, and writing. DY: conceptualization and data collection. SL: study design, data collection, and writing. J-WX: study design, data collection, writing, and financial support. All authors read and approved the final manuscript.
Funding
This work was supported by grants from the Specialized Research Fund for the National Natural Science Foundation of China (81973511).
Conflict of interest
The authors declare that the research was conducted in the absence of any commercial or financial relationships that could be construed as a potential conflict of interest.
Publisher's note
All claims expressed in this article are solely those of the authors and do not necessarily represent those of their affiliated organizations, or those of the publisher, the editors and the reviewers. Any product that may be evaluated in this article, or claim that may be made by its manufacturer, is not guaranteed or endorsed by the publisher.
Supplementary material
The Supplementary Material for this article can be found online at: https://www.frontiersin.org/articles/10.3389/fcvm.2022.988360/full#supplementary-material
References
1. Zhu W-F editor. Application of National Standards: TCM Internal Disease Diagnosis and Treatment Routine (Book in Chinese). Changsha: Hunan Science and Technology Press (1999), p. 561.
2. Shi J, Luo L, Chen J, Wang J, Zhao H, Wang W. Study on the differences between traditional Chinese Medicine Syndromes in NYHA I-IV classification of chronic heart failure. Evid Based Complement Alternat Med. (2019) 2019:2543413. doi: 10.1155/2019/2543413
3. Sun J, Zhang K, Xiong WJ, Yang GY, Zhang YJ, Wang CC, et al. Clinical effects of a standardized Chinese herbal remedy, Qili Qiangxin, as an adjuvant treatment in heart failure: systematic review and meta-analysis. BMC Complement Altern Med. (2016) 16:201. doi: 10.1186/s12906-016-1174-1
4. Li X, Zhang J, Huang J, Ma A, Yang J, Li W, et al. A multicenter, randomized, double-blind, parallel-group, placebo-controlled study of the effects of Qili Qiangxin capsules in patients with chronic heart failure. J Am Coll Cardiol. (2013) 62:1065–72. doi: 10.1016/j.jacc.2013.05.035
5. Wang X, Zhao Z, Mao J, Du T, Chen Y, Xu H, et al. Randomized, double-blinded, multicenter, placebo-controlled trial of shenfu injection for treatment of patients with chronic heart failure during the acute phase of symptom aggravation (Yang and Qi deficiency syndrome). Evid Based Complement Alternat Med. (2019) 2019:9297163. doi: 10.1155/2019/9297163
6. Adamo L, Rocha-Resende C, Prabhu SD, Mann DL. Reappraising the role of inflammation in heart failure. Nat Rev Cardiol. (2020)17:269–25. doi: 10.1038/s41569-019-0315-x
7. Mishra S, Kass DA. Cellular and molecular pathobiology of heart failure with preserved ejection fraction. Nat Rev Cardiol. (2021) 18:400–23. doi: 10.1038/s41569-020-00480-6
8. Hartupee J, Mann DL. Neurohormonal activation in heart failure with reduced ejection fraction. Nat Rev Cardiol. (2017)14:30–8. doi: 10.1038/nrcardio.2016.163
9. Liu J, Peter K, Shi D, Zhang L, Dong G, Zhang D, et al. Anti-inflammatory effects of the Chinese herbal formula Sini Tang in myocardial infarction rats. Evid Based Complement Alternat Med. (2014) 2014:309378. doi: 10.1155/2014/309378
10. Zhou P, Zhang M, Zhao XN, Tang TJ, Wang X, Huang LL, et al. Exploring the mechanism of Ling-Gui-Zhu-Gan decoction in ventricular remodeling after acute myocardial infarction based on UPLC and in vivo experiments. Evid Based Complement Altern Med. (2022) 2022:8593176. doi: 10.1155/2022/8593176
11. Wang X, Tang T, Zhai M, Ge R, Wang L, Huang J, et al. Ling-Gui-Zhu-Gan decoction protects H9c2 cells against H2O2-induced oxidative injury via regulation of the Nrf2/Keap1/HO-1 signaling pathway. Evid Based Complement Altern Med. (2020) 2020:8860603. doi: 10.1155/2020/8860603
12. Chen Y, Li L, Hu C, Zhao X, Zhang P, Chang Y, et al. Lingguizhugan decoction dynamically regulates MAPKs and AKT signaling pathways to retrogress the pathological progression of cardiac hypertrophy to heart failure. Phytomedicine. (2022) 98:153951. doi: 10.1016/j.phymed.2022.153951
13. Lai J, Wu Y, Hang L, Gael A, Deng T, Yan Q, et al. Zhenwu decoction delays ventricular hypertrophy in rats with uremic cardiomyopathy. Nan Fang Yi Ke Da Xue Xue Bao. (2019) 39:113–9. doi: 10.12122/j.issn.1673-4254.2019.01.18
14. Xie ZX, Wang SY, Liang ZJ, Zeng LB. Effect of Zhenwu Tang granule on pressure-overloaded left ventricular myocardial hypertrophy in rats. World J Emerg Med. (2010) 1:149–53.
15. Zhu Y, Zhao J, Han Q, Wang Z, Wang Z, Dong X, et al. The effect and mechanism of Chinese herbal formula Sini Tang in heart failure after myocardial infarction in rats. Evid Based Complement Altern Med. (2018) 2018:5629342. doi: 10.1155/2018/5629342
16. Cui X, Zhang J, Li Y, Sun Y, Cao J, Zhao M, et al. Effects of Qili Qiangxin capsule on AQP2, V2R, and AT1R in rats with chronic heart failure. Evid Based Complement Altern Med. (2015) 2015:639450. doi: 10.1155/2015/639450
17. Huang Y, Zhang K, Jiang M, Ni J, Chen J, Li L, et al. Regulation of energy metabolism by combination therapy attenuates cardiac metabolic remodeling in heart failure. Int J Biol Sci. (2020) 16:3133–48. doi: 10.7150/ijbs.49520
18. Liu J, Peter K, Shi D, Zhang L, Dong G, Zhang D, et al. Traditional formula, modern application: Chinese medicine formula Sini Tang improves early ventricular remodeling and cardiac function after myocardial infarction in rats. Evid Based Complement Altern Med. (2014) 2014:141938. doi: 10.1155/2014/141938
19. Han A, Lu Y, Zheng Q, Zhang J, Zhao Y, Zhao M, et al. Qiliqiangxin attenuates cardiac remodeling via inhibition of TGF-β1/Smad3 and NF-κB signaling pathways in a rat model of myocardial infarction. Cell Physiol Biochem. (2018) 45:1797–806. doi: 10.1159/000487871
20. Wang J, Zhou J, Ding X, Zhu L, Jiang K, Fu M, et al. Qiliqiangxin improves cardiac function and attenuates cardiac remodeling in rats with experimental myocardial infarction. Int J Clin Exp Pathol. (2015) 8:6596–606.
21. Zhu J, Song W, Xu S, Ma Y, Wei B, Wang H, et al. Shenfu Injection promotes vasodilation by enhancing eNOS activity through the PI3K/Akt signaling pathway in vitro. Front Pharmacol. (2020) 11:121. doi: 10.3389/fphar.2020.00121
22. Murphy SP, Kakkar R, McCarthy CP, Januzzi JL Jr. Inflammation in heart failure: JACC state-of-the-art review. J Am Coll Cardiol. (2020) 75:1324–40. doi: 10.1016/j.jacc.2020.01.014
23. Corcoran SE, O'Neill LA. HIF1α and metabolic reprogramming in inflammation. J Clin Invest. (2016) 126:3699–707. doi: 10.1172/JCI84431
24. Williams NC, O'Neill LAJ. A role for the Krebs cycle intermediate citrate in metabolic reprogramming in innate immunity and inflammation. Front Immunol. (2018) 9:141. doi: 10.3389/fimmu.2018.00141
25. Sun L, Yang X, Yuan Z, Wang H. Metabolic reprogramming in immune response and tissue inflammation. Arterioscler Thromb Vasc Biol. (2020) 40:1990–2001. doi: 10.1161/ATVBAHA.120.314037
26. Brown DA, Perry JB, Allen ME, Sabbah HN, Stauffer BL, Shaikh SR, et al. Expert consensus document: mitochondrial function as a therapeutic target in heart failure. Nat Rev Cardiol. (2017) 14:238–50. doi: 10.1038/nrcardio.2016.203
27. Lopaschuk GD, Karwi QG, Tian R, Wende AR, Abel ED. Cardiac energy metabolism in heart failure. Circ Res. (2021) 128:1487–513. doi: 10.1161/CIRCRESAHA.121.318241
28. Lai L, Leone TC, Keller MP, Martin OJ, Broman AT, Nigro J, et al. Energy metabolic reprogramming in the hypertrophied and early stage failing heart: a multisystems approach. Circ Heart Fail. (2014) 7:1022–31. doi: 10.1161/CIRCHEARTFAILURE.114.001469
29. Kashihara T, Mukai R, Oka SI, Zhai P, Nakada Y, Yang Z, et al. YAP mediates compensatory cardiac hypertrophy through aerobic glycolysis in response to pressure overload. J Clin Invest. (2022) 132:e150595. doi: 10.1172/JCI150595
30. Rodriguez P, Sassi Y, Troncone L, Benard L, Ishikawa K, Gordon RE, et al. Deletion of delta-like 1 homologue accelerates fibroblast-myofibroblast differentiation and induces myocardial fibrosis. Eur Heart J. (2019) 40:967–78. doi: 10.1093/eurheartj/ehy188
31. Yang XQ, Zhao FF, Wang DX. Metabolism reprogramming: new insights of Dlk1 into cardiac fibrosis. Eur Heart J. (2019) 40:3574. doi: 10.1093/eurheartj/ehz603
32. Schiattarella GG, Sequeira V, Ameri P. Distinctive patterns of inflammation across the heart failure syndrome. Heart Fail Rev. (2021) 26:1333–44. doi: 10.1007/s10741-020-09949-5
33. Gordon JW, Shaw JA, Kirshenbaum LA. Multiple facets of NF-κB in the heart: to be or not to NF-κB. Circ Res. (2011) 108:1122–32. doi: 10.1161/CIRCRESAHA.110.226928
34. Choy KW, Murugan D, Mustafa MR. Natural products targeting ER stress pathway for the treatment of cardiovascular diseases. Pharmacol Res. (2018) 132:119–29. doi: 10.1016/j.phrs.2018.04.013
35. Bansal SS, Ismahil MA, Goel M, Patel B, Hamid T, Rokosh G, et al. Activated T lymphocytes are essential drivers of pathological remodeling in ischemic heart failure. Circ Heart Fail. (2017) 10:e003688. doi: 10.1161/CIRCHEARTFAILURE.116.003688
36. Rai A, Narisawa M, Li P, Piao L, Li Y, Yang G, et al. Adaptive immune disorders in hypertension and heart failure: focusing on T-cell subset activation and clinical implications. J Hypertens. (2020) 38:1878–89. doi: 10.1097/HJH.0000000000002456
37. Sager HB, Hulsmans M, Lavine KJ, Moreira MB, Heidt T, Courties G, et al. Proliferation and recruitment contribute to myocardial macrophage expansion in chronic heart failure. Circ Res. (2016) 119:853–64. doi: 10.1161/CIRCRESAHA.116.309001
38. Vredevoe DL, Widawski M, Fonarow GC, Hamilton M, Martínez-Maza O, Gage JR. Interleukin-6 (IL-6) expression and natural killer (NK) cell dysfunction and anergy in heart failure. Am J Cardiol. (2004) 93:1007–11. doi: 10.1016/j.amjcard.2003.12.054
39. Wang H, Hu Y, Song Q, Qiu Z, Bo R. The impact of Shenfu injection on the immune function in patients with chronic heart failure and heart kidney Yang deficiency syndrome. Chin J Integrat Med Cardio/Cerebrovasc Dis. (2016) 14:1441–4. doi: 10.3969/j.issn.1672-1349.2016.13.001
40. Fan GR. Abnormality of T lymphocyte subsets in aged persons with kidney deficiency syndrome and its influence on immunologic regulation. Zhongguo Zhong Xi Yi Jie He Za Zhi. (1992) 12:478–9; 453–4.
41. Zhao SY, Zhao HH, Hao TT, Li WW, Guo H. Effect of Bushen Huoxue prescription on cognitive dysfunction of KK-Ay type 2 diabetic mice. Evid Based Complement Alternat Med. (2021) 2021:6656362. doi: 10.1155/2021/6656362
42. Zhou J, Ma X, Shi M, Chen C, Sun Y, Li J, et al. Serum metabolomics analysis reveals that obvious cardioprotective effects of low dose Sini decoction against isoproterenol-induced myocardial injury in rats. Phytomedicine. (2017) 31:18–31. doi: 10.1016/j.phymed.2017.01.009
43. Cao H, Zhang A, Sun H, Zhou X, Guan Y, Liu Q, et al. Metabolomics-proteomics profiles delineate metabolic changes in kidney fibrosis disease. Proteomics. (2015) 15:3699–10. doi: 10.1002/pmic.201500062
44. Wang Y, Liu Z, Wei J, Di L, Wang S, Wu T, et al. Norlignans and phenolics from genus curculigo protect corticosterone-injured neuroblastoma cells SH-SY5Y by inhibiting endoplasmic reticulum stress-mitochondria pathway. J Ethnopharmacol. (2022) 296:115430. doi: 10.1016/j.jep.2022.115430
45. Wen J, Li M, Zhang W, Wang H, Bai Y, Hao J, et al. Role of higenamine in heart diseases: a mini-review. Front Pharmacol. (2022) 12:798495. doi: 10.3389/fphar.2021.798495
46. Zhu JX, Ling W, Xue C, Zhou Z, Zhang YS, Yan C, et al. Higenamine attenuates cardiac fibroblast abstract and fibrosis via inhibition of TGF-β1/Smad signaling. Eur J Pharmacol. (2021) 900:174013. doi: 10.1016/j.ejphar.2021.174013
47. Kang YJ, Lee YS, Lee GW, Lee DH, Ryu JC, Yun-Choi HS, et al. Inhibition of activation of nuclear factor kappaB is responsible for inhibition of inducible nitric oxide synthase expression by higenamine, an active component of aconite root. J Pharmacol Exp Ther. (1999) 291:314–20.
48. Lee HY, Lee JS, Kim EJ, Han JW, Lee HW, Kang YJ, et al. Inhibition of lipopolysaccharide-induced inducible nitric oxide (iNOS) mRNA expression and nitric oxide production by higenamine in murine peritoneal macrophages. Arch Pharm Res. (1999) 22:55–9. doi: 10.1007/BF02976436
49. Bai X, Ding W, Yang S, Guo X. Higenamine inhibits IL-1β-induced inflammation in human nucleus pulposus cells. Biosci Rep. (2019) 39:BSR20190857. doi: 10.1042/BSR20190857
50. Li X, Jiang J, Shi S, Bligh SW, Li Y, Jiang Y, et al. A RG-II type polysaccharide purified from Aconitum coreanum alleviates lipopolysaccharide-induced inflammation by inhibiting the NF-κB signal pathway. PLoS ONE. (2014) 9:e99697. doi: 10.1371/journal.pone.0099697
51. Zhang Z, Li M, Wang Y, Wu J, Li J. Higenamine promotes M2 macrophage activation and reduces Hmgb1 production through HO-1 induction in a murine model of spinal cord injury. Int Immunopharmacol. (2014) 23:681–7. doi: 10.1016/j.intimp.2014.10.022
52. Zhao C, Li M, Luo Y, Wu W. Isolation and structural characterization of an immunostimulating polysaccharide from fuzi, Aconitum carmichaeli. Carbohydr Res. (2006) 341:485–91. doi: 10.1016/j.carres.2005.11.032
53. Yang L, Wu QQ, Liu Y, Hu ZF, Bian ZY, Tang QZ. Cinnamaldehyde attenuates pressure overload-induced cardiac hypertrophy. Int J Clin Exp Pathol. (2015) 8:14345–54.
54. Song F, Li H, Sun J, Wang S. Protective effects of cinnamic acid and cinnamic aldehyde on isoproterenol-induced acute myocardial ischemia in rats. J Ethnopharmacol. (2013) 150:125–30. doi: 10.1016/j.jep.2013.08.019
55. Cheng WX, Zhong S, Meng XB, Zheng NY, Zhang P, Wang Y, et al. Cinnamaldehyde inhibits inflammation of human synoviocyte cells through regulation of Jak/Stat pathway and ameliorates collagen-induced arthritis in rats. J Pharmacol Exp Ther. (2020) 373:302–10. doi: 10.1124/jpet.119.262907
56. Li W, Zhi W, Zhao J, Yao Q, Liu F, Niu X. Cinnamaldehyde protects VSMCs against ox-LDL-induced proliferation and migration through S arrest and inhibition of p38, JNK/MAPKs and NF-κB. Vascul Pharmacol. (2018) 108:57–66. doi: 10.1016/j.vph.2018.05.005
57. Zhao H, Zhang M, Zhou F, Cao W, Bi L, Xie Y, et al. Cinnamaldehyde ameliorates LPS-induced cardiac dysfunction via TLR4-NOX4 pathway: the regulation of autophagy and ROS production. J Mol Cell Cardiol. (2016) 101:11–24. doi: 10.1016/j.yjmcc.2016.10.017
58. Kang LL, Zhang DM, Ma CH, Zhang JH, Jia KK, Liu JH, et al. Cinnamaldehyde and allopurinol reduce fructose-induced cardiac inflammation and fibrosis by attenuating CD36-mediated TLR4/6-IRAK4/1 signaling to suppress NLRP3 inflammasome activation. Sci Rep. (2016) 6:27460. doi: 10.1038/srep27460
59. Liu P, Wang J, Wen W, Pan T, Chen H, Fu Y, et al. Cinnamaldehyde suppresses NLRP3 derived IL-1β via activating succinate/HIF-1 in rheumatoid arthritis rats. Int Immunopharmacol. (2020) 84:106570. doi: 10.1016/j.intimp.2020.106570
60. Jiang WL, Zhang SM, Tang XX, Liu HZ. Protective roles of cornuside in acute myocardial ischemia and reperfusion injury in rats. Phytomedicine. (2011) 18:266–71. doi: 10.1016/j.phymed.2010.07.009
61. Yu B, Wang W. Cardioprotective effects of morroniside in rats following acute myocardial infarction. Inflammation. (2018) 41:432–6. doi: 10.1007/s10753-017-0699-x
62. Choi YH, Jin GY, Li GZ, Yan GH. Cornuside suppresses lipopolysaccharide-induced inflammatory mediators by inhibiting nuclear factor-kappa B activation in RAW 264.7 macrophages. Biol Pharm Bull. (2011) 34:959–66. doi: 10.1248/bpb.34.959
63. Park C, Cha HJ, Lee H, Kim GY, Choi YH. The regulation of the TLR4/NF-κB and Nrf2/HO-1 signaling pathways is involved in the inhibition of lipopolysaccharide-induced inflammation and oxidative reactions by morroniside in RAW 264.7 macrophages. Arch Biochem Biophys. (2021) 706:108926. doi: 10.1016/j.abb.2021.108926
64. Yuan J, Cheng W, Zhang G, Ma Q, Li X, Zhang B, et al. Protective effects of iridoid glycosides on acute colitis via inhibition of the inflammatory response mediated by the STAT3/NF-κB pathway. Int Immunopharmacol. (2020) 81:106240. doi: 10.1016/j.intimp.2020.106240
65. Feng J, Yang Y, Zhou Y, Wang B, Xiong H, Fan C, et al. Bakuchiol attenuates myocardial ischemia reperfusion injury by maintaining mitochondrial function: the role of silent information regulator 1. Apoptosis. (2016) 21:532–45. doi: 10.1007/s10495-016-1225-6
66. Wang Z, Gao L, Xiao L, Kong L, Shi H, Tian X, et al. Bakuchiol protects against pathological cardiac hypertrophy by blocking NF-κB signaling pathway. Biosci Rep. (2018) 38:BSR20181043. doi: 10.1042/BSR20181043
67. Kassahun Gebremeskel A, Wijerathne TD, Kim JH, Kim MJ, Seo CS, Shin HK, et al. Psoralea corylifolia extract induces vasodilation in rat arteries through both endothelium-dependent and -independent mechanisms involving inhibition of TRPC3 channel activity and elaboration of prostaglandin. Pharm Biol. (2017) 55:2136–44. doi: 10.1080/13880209.2017.1383484
68. Zhang X, Chang N, Zhang Y, Ye M, Han Z, Li J, et al. Bakuchiol protects against acute lung injury in septic mice. Inflammation. (2017) 40:351–9. doi: 10.1007/s10753-016-0481-5
69. Song YH, Cai H, Gu N, Qian CF, Cao SP, Zhao ZM. Icariin attenuates cardiac remodelling through down-regulating myocardial apoptosis and matrix metalloproteinase activity in rats with congestive heart failure. J Pharm Pharmacol. (2011) 63:541–9. doi: 10.1111/j.2042-7158.2010.01241.x
70. Liu XY, Liao HH, Feng H, Zhang N, Yang JJ, Li WJ, et al. Icariside II attenuates cardiac remodeling via AMPKα2/mTORC1 in vivo and in vitro. J Pharmacol Sci. (2018) 138:38–45. doi: 10.1016/j.jphs.2018.08.010
71. Fu S, Li YL, Wu YT, Yue Y, Qian ZQ, Yang DL. Icariside II attenuates myocardial fibrosis by inhibiting nuclear factor-κB and the TGF-β1/Smad2 signalling pathway in spontaneously hypertensive rats. Biomed Pharmacother. (2018) 100:64–71. doi: 10.1016/j.biopha.2018.01.138
72. Wu QQ, Xiao Y, Duan MX, Yuan Y, Jiang XH, Yang Z, et al. Aucubin protects against pressure overload-induced cardiac remodelling via the β3 -adrenoceptor-neuronal NOS cascades. Br J Pharmacol. (2018) 175:1548–66. doi: 10.1111/bph.14164
73. Zhou F, Zhong W, Xue J, Gu ZL, Xie ML. Reduction of rat cardiac hypertrophy by osthol is related to regulation of cardiac oxidative stress and lipid metabolism. Lipids. (2012) 47:987–94. doi: 10.1007/s11745-012-3710-1
74. Sharma S, Khan V, Dhyani N, Najmi AK, Haque SE. Icariin attenuates isoproterenol-induced cardiac toxicity in Wistar rats via modulating cGMP level and NF-κB signaling cascade. Hum Exp Toxicol. (2020) 39:117–26. doi: 10.1177/0960327119890826
75. Ni Y, Deng J, Liu X, Li Q, Zhang J, Bai H, et al. Echinacoside reverses myocardial remodeling and improves heart function via regulating SIRT1/FOXO3a/MnSOD axis in HF rats induced by isoproterenol. J Cell Mol Med. (2021) 25:203–16. doi: 10.1111/jcmm.15904
76. Zhai M, He L, Ju X, Shao L, Li G, Zhang Y, et al. Icariin acts as a potential agent for preventing cardiac ischemia/reperfusion injury. Cell Biochem Biophys. (2015) 72:589–97. doi: 10.1007/s12013-014-0506-3
77. Cho S, Cho M, Kim J, Kaeberlein M, Lee SJ, Suh Y. Syringaresinol protects against hypoxia/reoxygenation-induced cardiomyocytes injury and death by destabilization of HIF-1α in a FOXO3-dependent mechanism. Oncotarget. (2015) 6:43–55. doi: 10.18632/oncotarget.2723
78. Wu J, Yang Y, Xun N, Zeng L, Li Z, Yang W, et al. Osthole attenuates myocardial ischemia/reperfusion injury in rats by inhibiting apoptosis and inflammation. Am J Transl Res. (2018) 10:1109–116.
79. Yang Z, Wu QQ, Xiao Y, Duan MX, Liu C, Yuan Y, et al. Aucubin protects against myocardial infarction-induced cardiac remodeling via nNOS/NO-regulated oxidative stress. Oxid Med Cell Longev. (2018) 2018:4327901. doi: 10.1155/2018/4327901
80. Zhou H, Yuan Y, Liu Y, Ni J, Deng W, Bian ZY, et al. Icariin protects H9c2 cardiomyocytes from lipopolysaccharide-induced injury via inhibition of the reactive oxygen species-dependent c-Jun N-terminal kinases/nuclear factor-κB pathway. Mol Med Rep. (2015) 11:4327–32. doi: 10.3892/mmr.2015.3289
81. Zhou J, Deng Y, Li F, Yin C, Shi J, Gong Q. Icariside II attenuates lipopolysaccharide-induced neuroinflammation through inhibiting TLR4/MyD88/NF-κB pathway in rats. Biomed Pharmacother. (2019) 111:315–24. doi: 10.1016/j.biopha.2018.10.201
82. Duan M, Yuan Y, Liu C, Cai Z, Xie Q, Hu T, et al. Indigo fruits ingredient, aucubin, protects against LPS-induced cardiac dysfunction in mice. J Pharmacol Exp Ther. (2019) 371:348–59. doi: 10.1124/jpet.119.259069
83. Li L, Wan G, Han B, Zhang Z. Echinacoside alleviated LPS-induced cell apoptosis and inflammation in rat intestine epithelial cells by inhibiting the mTOR/STAT3 pathway. Biomed Pharmacother. (2018) 104:622–8. doi: 10.1016/j.biopha.2018.05.072
84. Fan H, Gao Z, Ji K, Li X, Wu J, Liu Y, et al. The in vitro and in vivo anti-inflammatory effect of osthole, the major natural coumarin from Cnidium monnieri (L.) Cuss, via the blocking of the activation of the NF-κB and MAPK/p38 pathways. Phytomedicine. (2019) 58:152864. doi: 10.1016/j.phymed.2019.152864
85. Gao S, Xu T, Guo H, Deng Q, Xun C, Liang W, et al. Ameliorative effects of echinacoside against spinal cord injury via inhibiting NLRP3 inflammasome signaling pathway. Life Sci. (2019) 237:116978. doi: 10.1016/j.lfs.2019.116978
86. Rigas A, Farmakis D, Papingiotis G, Bakosis G, Parissis J. Hypothalamic dysfunction in heart failure: pathogenetic mechanisms and therapeutic implications. Heart Fail Rev. (2018) 23:55–61. doi: 10.1007/s10741-017-9659-7
87. Chen WW, Xiong XQ, Chen Q, Li YH, Kang YM, Zhu GQ. Cardiac sympathetic afferent reflex and its implications for sympathetic activation in chronic heart failure and hypertension. Acta Physiol (Oxf). (2015) 213:778–94. doi: 10.1111/apha.12447
88. Kang YM, Yang Q, Yu XJ, Qi J, Zhang Y, Li HB, et al. Hypothalamic paraventricular nucleus activation contributes to neurohumoral excitation in rats with heart failure. Regen Med Res. (2014) 2:2. doi: 10.1186/2050-490X-2-2
89. Wei SG, Yu Y, Felder RB. Blood-borne interleukin-1β acts on the subfornical organ to upregulate the sympathoexcitatory milieu of the hypothalamic paraventricular nucleus. Am J Physiol Regul Integr Comp Physiol. (2018) 314:R447–58. doi: 10.1152/ajpregu.00211.2017
90. Yu Y, Wei SG, Weiss RM, Felder RB. TNF-α receptor 1 knockdown in the subfornical organ ameliorates sympathetic excitation and cardiac hemodynamics in heart failure rats. Am J Physiol Heart Circ Physiol. (2017) 313:H744–56. doi: 10.1152/ajpheart.00280.2017
91. Rossi NF, Zenner Z, Rishi AK, Levi E, Maliszewska-Scislo M. AT1 receptors in the subfornical organ modulate arterial pressure and the baroreflex in two-kidney, one-clip hypertensive rats. Am J Physiol Regul Integr Comp Physiol. (2019) 316:R172–85. doi: 10.1152/ajpregu.00289.2018
92. Singh RB, Hristova K, Fedacko J, El-Kilany G, Cornelissen G. Chronic heart failure: a disease of the brain. Heart Fail Rev. (2019) 24:301–7. doi: 10.1007/s10741-018-9747-3
93. Yu Y, Wei SG, Weiss RM, Felder RB. Angiotensin II type 1a receptors in the subfornical organ modulate neuroinflammation in the hypothalamic paraventricular nucleus in heart failure rats. Neuroscience. (2018) 381:46–58. doi: 10.1016/j.neuroscience.2018.04.012
94. Zheng L, Liu X, Wang L, Tao Z. The influence of kidney - yang deficiency on central neurotransmitter and cholinesterase. J New Chinese Med. (2000) 32:31–2. doi: 10.3969/j.issn.0256-7415.2000.05.019
95. Ma L, Yin Y, Zhang J, Liu H, Jia Z. Effects of Qiliqiangxin on hypothalamic paraventricular nucleus renin angiotensin system and sympathetic nervous system in rats with chronic heart failure (Article in Chinese). Chin Pharmacol Bull. (2016) 32: 575–80. doi: 10.3969/j.issn.1001-1978.2016.04.026
96. Jiang L, Yan Q, Wen Q. Experimental study of Bushen Ningxin Granules and impacts on norepinephrine and dopamine in brain tissue of experimental anxiety model rats (Article in Chinese). World J Integr Tradit Chin West Med. (2010) 5:1033–6. doi: 10.3969/j.issn.1673-6613.2010.12.009
97. Jiang N, Jingwei L, Wang H, Huang H, Wang Q, Zeng G, et al. Ginsenoside 20(S)-protopanaxadiol attenuates depressive-like behaviour and neuroinflammation in chronic unpredictable mild stress-induced depressive rats. Behav Brain Res. (2020) 393:112710. doi: 10.1016/j.bbr.2020.112710
98. Zhang K, Lu J, Yao L. Involvement of the dopamine D1 receptor system in the anxiolytic effect of cedrol in the elevated plus maze and light-dark box tests. J Pharmacol Sci. (2020) 142:26–33. doi: 10.1016/j.jphs.2019.11.004
99. Kang YM, Zhang ZH, Johnson RF, Yu Y, Beltz T, Johnson AK, et al. Novel effect of mineralocorticoid receptor antagonism to reduce proinflammatory cytokines and hypothalamic activation in rats with ischemia-induced heart failure. Circ Res. (2006) 99:758–66. doi: 10.1161/01.RES.0000244092.95152.86
100. Kang YM, Zhang ZH, Xue B, Weiss RM, Felder RB. Inhibition of brain proinflammatory cytokine synthesis reduces hypothalamic excitation in rats with ischemia-induced heart failure. Am J Physiol Heart Circ Physiol. (2008) 295:H227–36. doi: 10.1152/ajpheart.01157.2007
101. Kang YM, Zhang AQ, Zhao XF, Cardinale JP, Elks C, Cao XM, et al. Paraventricular nucleus corticotrophin releasing hormone contributes to sympathoexcitation via interaction with neurotransmitters in heart failure. Basic Res Cardiol. (2011) 106:473–83. doi: 10.1007/s00395-011-0155-2
102. Tenk J, Rostás I, Füredi N, Mikó A, Solymár M, Soós S, et al. Age-related changes in central effects of corticotropin-releasing factor (CRF) suggest a role for this mediator in aging anorexia and cachexia. Geroscience. (2017) 39:61–72. doi: 10.1007/s11357-017-9962-1
103. Kasckow JW, Regmi A, Mulchahey JJ, Plotsky PM, Hauger RL. Changes in brain corticotropin-releasing factor messenger RNA expression in aged Fischer 344 rats. Brain Res. (1999) 822: 228–30. doi: 10.1016/S0006-8993(98)01365-1
104. Henschkowski J, Stuck AE, Frey BM, Gillmann G, Dick B, Frey FJ, et al. Age-dependent decrease in 11beta-hydroxysteroid dehydrogenase type 2 (11beta-HSD2) activity in hypertensive patients. Am J Hypertens. (2008) 21:644–9. doi: 10.1038/ajh.2008.152
105. DuPont JJ, McCurley A, Davel AP, McCarthy J, Bender SB, Hong K, et al. Vascular mineralocorticoid receptor regulates microRNA-155 to promote vasoconstriction and rising blood pressure with aging. JCI Insight. (2016) 1:e88942. doi: 10.1172/jci.insight.88942
106. Nallamothu BK, Payvar S, Wang Y, Kosiborod M, Masoudi FA, Havranek EP, et al. Admission body temperature and mortality in elderly patients hospitalized for heart failure. J Am Coll Cardiol. (2006) 47:2563–4. doi: 10.1016/j.jacc.2006.03.017
107. Payvar S, Orlandi C, Stough WG, Elkayam U, Ouyang J, Casscells SW. Comparison of 60-day mortality in hospitalized heart failure patients with versus without hypothermia. Am J Cardiol. (2006) 98:1485–8. doi: 10.1016/j.amjcard.2006.06.051
108. Andreae C, Årestedt K, Evangelista L, Strömberg A. The relationship between physical activity and appetite in patients with heart failure: a prospective observational study. Eur J Cardiovasc Nurs. (2019) 18:410–7. doi: 10.1177/1474515119836567
109. Saitoh M, Dos Santos MR, Emami A, Ishida J, Ebner N, Valentova M, et al. Anorexia, functional capacity, and clinical outcome in patients with chronic heart failure: results from the Studies Investigating Co-morbidities Aggravating Heart Failure (SICA-HF). ESC Heart Fail. (2017) 4:448–57. doi: 10.1002/ehf2.12209
110. Rollman BL, Anderson AM, Rothenberger SD, Abebe KZ, Ramani R, Muldoon MF, et al. Efficacy of blended collaborative care for patients with heart failure and comorbid depression: a randomized clinical trial. JAMA Intern Med. (2021) 181:1369–80. doi: 10.1001/jamainternmed.2021.4978
111. Liguori I, Russo G, Curcio F, Sasso G, Della-Morte D, Gargiulo G, et al. Depression and chronic heart failure in the elderly: an intriguing relationship. J Geriatr Cardiol. (2018) 15:451–9. doi: 10.11909/j.issn.1671-5411.2018.06.014
112. Gibbs DM. Inhibition of corticotropin release during hypothermia: the role of corticotropin-releasing factor, vasopressin, and oxytocin. Endocrinology. (1985) 116:723–7. doi: 10.1210/endo-116-2-723
113. Cavagnini F, Invitti C, Passamonti M, Polli EE. Response of ACTH and cortisol to corticotropin-releasing hormone in anorexia nervosa. N Engl J Med. (1986) 314:184–5. doi: 10.1056/NEJM198601163140318
114. Tang N, Liu L, Qiu H, Shi W, Mao D. Analysis of gene expression and functional changes of adrenal gland in a rat model of kidney Yang deficiency syndrome treated with Sini decoction. Exp Ther Med. (2018) 16:3107–15. doi: 10.3892/etm.2018.6521
115. Ninomiya F. Clinical evaluation of perspiration reducing effects of a Kampo formula, Shigyaku-San, on Palmoplantar Hidrosis. Evid Based Complement Altern Med. (2008) 5:199–203. doi: 10.1093/ecam/nem008
116. Kanai S, Okano H, Abe H. Efficacy of toki-shigyakuka-gosyuyu-syokyo-to (danggui-sini-jia-wuzhuyu-shengjiang-tang) on peripheral circulation in autonomic disorders. Am J Chin Med. (1997) 25:69–78. doi: 10.1142/S0192415X9700010X
117. Cai D, Chen X, Liu Y. Effect of regulation of kidney-yin and kidney-yang on hypothalamus-pituitary-adrenal-thymus axis in monosodium L-glutamate rats. Zhongguo Zhong Xi Yi Jie He Za Zhi. (1999) 19:415–7.
118. Huang L, Cai H, Zhuang J, Chen Y, Jin Z, Zhang H, et al. Fuling Sini decoction for patients with chronic heart failure: a protocol for a systematic review and meta-analysis. Medicine (Baltimore). (2018) 97:e13692. doi: 10.1097/MD.0000000000013692
119. Douglas SA, Tayara L, Ohlstein EH, Halawa N, Giaid A. Congestive heart failure and expression of myocardial urotensin II. Lancet. (2002) 359:1990–7. doi: 10.1016/S0140-6736(02)08831-1
120. Dautzenberg FM, Higelin J, Wille S, Brauns O. Molecular cloning and functional expression of the mouse CRF2(a) receptor splice variant. Regul Pept. (2004) 121:89-97. doi: 10.1016/j.regpep.2004.04.009
121. Tzanidis A, Hannan RD, Thomas WG, Onan D, Autelitano DJ, See F, et al. Direct actions of urotensin II on the heart: implications for cardiac fibrosis and hypertrophy. Circ Res. (2003) 93:246–53. doi: 10.1161/01.RES.0000084382.64418.BC
122. Lim M, Honisett S, Sparkes CD, Komesaroff P, Kompa A, Krum H. Differential effect of urotensin II on vascular tone in normal subjects and patients with chronic heart failure. Circulation. (2004) 109:1212–4. doi: 10.1161/01.CIR.0000121326.69153.98
123. Liang Y, Wu X, Xu M, Ding L, Li H, Wu Y. Urotensin II induces activation of NLRP3 and pyroptosis through calcineurin in cardiomyocytes. Peptides. (2021) 144:170609. doi: 10.1016/j.peptides.2021.170609
124. Johns DG, Ao Z, Naselsky D, Herold CL, Maniscalco K, Sarov-Blat L, et al. Urotensin-II-mediated cardiomyocyte hypertrophy: effect of receptor antagonism and role of inflammatory mediators. Naunyn Schmiedebergs Arch Pharmacol. (2004) 370:238–50. doi: 10.1007/s00210-004-0980-z
125. Richards AM, Nicholls MG, Lainchbury JG, Fisher S, Yandle TG. Plasma urotensin II in heart failure. Lancet. (2002) 360:545–6. doi: 10.1016/S0140-6736(02)09709-X
126. Ng LL, Loke I, O'Brien RJ, Squire IB, Davies JE. Plasma urotensin in human systolic heart failure. Circulation. (2002) 106:2877–80. doi: 10.1161/01.CIR.0000044388.19119.02
127. Wang P, Xia Y, Zheng B. Treatment of chronic heart failure with Fuling Sini decoction and its effect on plasma urotensin II (articles in Chinese). Inform Tradit Chin Med. (2013) 30:58–60.
128. Liu X. The changes of plasma urotensin II and adrenomedullin in chronic heart failure and the intervention studies with Shenfu injection (Articles in Chinese). Med J West China. (2009) 21:1687–9. doi: 10.3969/j.issn.1672-3511.2009.10.018
129. Gheorghiade M, Niazi I, Ouyang J, Czerwiec F, Kambayashi J, Zampino M, et al. Vasopressin V2-receptor blockade with tolvaptan in patients with chronic heart failure: results from a double-blind, randomized trial. Circulation. (2003) 107:2690–6. doi: 10.1161/01.CIR.0000070422.41439.04
130. Martin PY, Abraham WT, Lieming X, Olson BR, Oren RM, Ohara M, et al. Selective V2-receptor vasopressin antagonism decreases urinary aquaporin-2 excretion in patients with chronic heart failure. J Am Soc Nephrol. (1999) 10:2165–70. doi: 10.1681/ASN.V10102165
131. Fang W, Zhu G, Chen T, Zhu K, He X. Correlation between distribution features of syndrome types and expression of AVP and AQP-2 in 60 patients with chronic systolic heart failure (article in Chinese). Chin Arch Tradit Chin Med. (2015) 33:345–7. doi: 10.13193/j.issn.1673-7717.2015.02.029
132. Xiong R, Li Y, Zheng K, Zhang T, Gao M, Li Y, et al. Er Shen Wan extract alleviates polyuria and regulates AQP 2 and AVPR 2 in a rat model of spleen-kidney Yang deficiency-induced diarrhea. Biomed Pharmacother. (2019) 110:302–11. doi: 10.1016/j.biopha.2018.11.147
133. Zhou X, Bao Y, Chen H, Xuan L, Chen X, Zhang J, et al. Effect of Zhenwu Tang on regulating of “AVP-V2R-AQP2” pathway in NRK-52E cells. Zhongguo Zhong Yao Za Zhi. (2018) 43:603–8. doi: 10.19540/j.cnki.cjcmm.20171113.007
134. Xian SX, Yang ZQ, Ren PH, Ye XH, Ye SL, Wang QH, et al. Effect of yangxinkang tablets on chronic heart failure: a multi-center randomized double-blind placebo-controlled trial. Chin J Integr Med. (2015) 21:733–42. doi: 10.1007/s11655-015-2170-x
135. Liu L, Mo Y, Wu B, Yu Z, Sun B. Effect of traditional Chinese medicine Poge heart-saving decoction on cardiac function in heart failure rat model. Evid Based Complement Alternat Med. (2020) 2020:8762509. doi: 10.1155/2020/8762509
136. Xu YW, Xu ZD, An R, Zhang H, Wang XH. Revealing the synergistic mechanism of Shenfu decoction for anti-heart failure through network pharmacology strategy. Chin J Nat Med. (2020) 18:536–49. doi: 10.1016/S1875-5364(20)30064-9
137. Xiang Q, Wang M, Ding Y, Fan M, Tong H, Chen J, et al. Qili qiangxin capsule combined with sacubitril/valsartan for HFrEF: a systematic review and meta-analysis. Front Pharmacol. (2022) 13:832782. doi: 10.3389/fphar.2022.832782
138. Liu L, Lu Y, Martinez J, Bi Y, Lian G, Wang T, et al. Proinflammatory signal suppresses proliferation and shifts macrophage metabolism from Myc-dependent to HIF1α-dependent. Proc Natl Acad Sci USA. (2016) 113:1564–9. doi: 10.1073/pnas.1518000113
139. Dolinsky VW, Cole LK, Sparagna GC, Hatch GM. Cardiac mitochondrial energy metabolism in heart failure: role of cardiolipin and sirtuins. Biochim Biophys Acta. (2016) 1861:1544. doi: 10.1016/j.bbalip.2016.03.008
140. Sepp M, Sokolova N, Jugai S, Mandel M, Peterson P, Vendelin M. Tight coupling of Na+/K+-ATPase with glycolysis demonstrated in permeabilized rat cardiomyocytes. PLoS ONE. (2014) 9:e99413. doi: 10.1371/journal.pone.0099413
141. Fernández-Moncada I, Barros LF. Non-preferential fuelling of the Na(+)/K(+)-ATPase pump. Biochem J. (2014) 460:353–61. doi: 10.1042/BJ20140003
142. Beckermann KE, Hongo R, Ye X, Young K, Carbonell K, Healey DCC, et al. CD28 costimulation drives tumor-infiltrating T cell glycolysis to promote inflammation. JCI Insight. (2020) 5:e138729. doi: 10.1172/jci.insight.138729
143. Wang Z, Zhao Q, Nie Y, Yu Y, Misra BB, Zabalawi M, et al. Solute Carrier Family 37 Member 2 (SLC37A2) negatively regulates murine macrophage inflammation by controlling glycolysis. iScience. (2020) 23:101125. doi: 10.1016/j.isci.2020.101125
144. Xiao W, Oldham WM, Priolo C, Pandey AK, Loscalzo J. Immunometabolic endothelial phenotypes: integrating inflammation and glucose metabolism. Circ Res. (2021) 129:9–29. doi: 10.1161/CIRCRESAHA.120.318805
145. Davogustto GE, Salazar RL, Vasquez HG, Karlstaedt A, Dillon WP, Guthrie PH, et al. Metabolic remodeling precedes mTORC1-mediated cardiac hypertrophy. J Mol Cell Cardiol. (2021) 158:115–27. doi: 10.1016/j.yjmcc.2021.05.016
146. Ritterhoff J, Young S, Villet O, Shao D, Neto FC, Bettcher LF, et al. Metabolic remodeling promotes cardiac hypertrophy by directing glucose to aspartate biosynthesis. Circ Res. (2020) 126:182–96. doi: 10.1161/CIRCRESAHA.119.315483
147. Chen Z, Liu M, Li L, Chen L. Involvement of the Warburg effect in non-tumor diseases processes. J Cell Physiol. (2018) 233:2839–49. doi: 10.1002/jcp.25998
148. Fillmore N, Levasseur JL, Fukushima A, Wagg CS, Wang W, Dyck JRB, et al. Uncoupling of glycolysis from glucose oxidation accompanies the development of heart failure with preserved ejection fraction. Mol Med. (2018) 24:3. doi: 10.1186/s10020-018-0005-x
149. Diakos NA, Navankasattusas S, Abel ED, Rutter J, McCreath L, Ferrin P, et al. Evidence of glycolysis up-regulation and pyruvate mitochondrial oxidation mismatch during mechanical unloading of the failing human heart: implications for cardiac reloading and conditioning. JACC Basic Transl Sci. (2016) 1:432–44. doi: 10.1016/j.jacbts.2016.06.009
150. Wang J, Bai L, Li J, Sun C, Zhao J, Cui C, et al. Proteomic analysis of mitochondria reveals a metabolic switch from fatty acid oxidation to glycolysis in the failing heart. Sci China C Life Sci. (2009) 52:1003–10. doi: 10.1007/s11427-009-0140-2
151. Wolf A, Agnihotri S, Micallef J, Mukherjee J, Sabha N, Cairns R, et al. Hexokinase 2 is a key mediator of aerobic glycolysis and promotes tumor growth in human glioblastoma multiforme. J Exp Med. (2011) 208:313–26. doi: 10.1084/jem.20101470
152. Wang L, Xiong H, Wu F, Zhang Y, Wang J, Zhao L, et al. Hexokinase 2-mediated Warburg effect is required for PTEN- and p53-deficiency-driven prostate cancer growth. Cell Rep. (2014) 8:1461–74. doi: 10.1016/j.celrep.2014.07.053
153. Li L, Peng G, Liu X, Zhang Y, Han H, Liu ZR. Pyruvate kinase M2 coordinates metabolism switch between glycolysis and glutaminolysis in cancer cells. iScience. (2020) 23:101684. doi: 10.1016/j.isci.2020.101684
154. Palsson-McDermott EM, Curtis AM, Goel G, Lauterbach MA, Sheedy FJ, Gleeson LE, et al. Pyruvate kinase M2 regulates Hif-1α activity and IL-1β induction and is a critical determinant of the warburg effect in LPS-activated macrophages. Cell Metab. (2015) 21:65–80. doi: 10.1016/j.cmet.2014.12.005
155. Zhao YH, Zhou M, Liu H, Ding Y, Khong HT, Yu D, et al. Upregulation of lactate dehydrogenase A by ErbB2 through heat shock factor 1 promotes breast cancer cell glycolysis and growth. Oncogene. (2009) 28:3689–701. doi: 10.1038/onc.2009.229
156. Chang CC, Zhang C, Zhang Q, Sahin O, Wang H, Xu J, et al. Upregulation of lactate dehydrogenase a by 14-3-3ζ leads to increased glycolysis critical for breast cancer initiation and progression. Oncotarget. (2016) 7:35270–83. doi: 10.18632/oncotarget.9136
157. Lum JJ, Bui T, Gruber M, Gordan JD, DeBerardinis RJ, Covello KL, et al. The transcription factor HIF-1alpha plays a critical role in the growth factor-dependent regulation of both aerobic and anaerobic glycolysis. Genes Dev. (2007) 21:1037–49. doi: 10.1101/gad.1529107
158. Hagiwara A, Cornu M, Cybulski N, Polak P, Betz C, Trapani F, et al. Hepatic mTORC2 activates glycolysis and lipogenesis through Akt, glucokinase, and SREBP1c. Cell Metab. (2012) 15:725–38. doi: 10.1016/j.cmet.2012.03.015
159. Cheng SC, Quintin J, Cramer RA, Shepardson KM, Saeed S, Kumar V, et al. mTOR- and HIF-1α-mediated aerobic glycolysis as metabolic basis for trained immunity. Science. (2014) 345:1250684. doi: 10.1126/science.1250684
160. Demaria M, Giorgi C, Lebiedzinska M, Esposito G, D'Angeli L, Bartoli A, et al. A STAT3-mediated metabolic switch is involved in tumour transformation and STAT3 addiction. Aging (Albany NY). (2010) 2:823–42. doi: 10.18632/aging.100232
161. Li M, Jin R, Wang W, Zhang T, Sang J, Li N, et al. STAT3 regulates glycolysis via targeting hexokinase 2 in hepatocellular carcinoma cells. Oncotarget. (2017) 8:24777–84. doi: 10.18632/oncotarget.15801
162. He TL, Zhang YJ, Jiang H, Li XH, Zhu H, Zheng KL. The c-Myc-LDHA axis positively regulates aerobic glycolysis and promotes tumor progression in pancreatic cancer. Med Oncol. (2015) 32:187. doi: 10.1007/s12032-015-0633-8
163. Zhang S, Li J, Xie P, Zang T, Shen H, Cao G, et al. STAT3/c-Myc axis-mediated metabolism alternations of inflammation-related glycolysis involve with colorectal carcinogenesis. Rejuvenation Res. (2019) 22:138–45. doi: 10.1089/rej.2018.2089
164. Sorokina N, O'Donnell JM, McKinney RD, Pound KM, Woldegiorgis G, LaNoue KF, et al. Recruitment of compensatory pathways to sustain oxidative flux with reduced carnitine palmitoyltransferase I activity characterizes inefficiency in energy metabolism in hypertrophied hearts. Circulation. (2007) 115:2033–41. doi: 10.1161/CIRCULATIONAHA.106.668665
165. He L, Kim T, Long Q, Liu J, Wang P, Zhou Y, et al. Carnitine palmitoyltransferase-1b deficiency aggravates pressure overload-induced cardiac hypertrophy caused by lipotoxicity. Circulation. (2012) 126:1705–16. doi: 10.1161/CIRCULATIONAHA.111.075978
166. Haynie KR, Vandanmagsar B, Wicks SE, Zhang J, Mynatt RL. Inhibition of carnitine palymitoyltransferase1b induces cardiac hypertrophy and mortality in mice. Diabetes Obes Metab. (2014) 16:757–60. doi: 10.1111/dom.12248
167. Goldenberg JR, Carley AN, Ji R, Zhang X, Fasano M, Schulze PC, et al. Preservation of acyl coenzyme A attenuates pathological and metabolic cardiac remodeling through selective lipid trafficking. Circulation. (2019) 139:2765–77. doi: 10.1161/CIRCULATIONAHA.119.039610
168. van Vlies N, Tian L, Overmars H, Bootsma AH, Kulik W, Wanders RJ, et al. Characterization of carnitine and fatty acid metabolism in the long-chain acyl-CoA dehydrogenase-deficient mouse. Biochem J. (2005) 387:185–93. doi: 10.1042/BJ20041489
169. Cox KB, Liu J, Tian L, Barnes S, Yang Q, Wood PA. Cardiac hypertrophy in mice with long-chain acyl-CoA dehydrogenase or very long-chain acyl-CoA dehydrogenase deficiency. Lab Invest. (2009) 89:1348–54. doi: 10.1038/labinvest.2009.86
170. Bakermans AJ, Geraedts TR, van Weeghel M, Denis S, João Ferraz M, Aerts JM, et al. Fasting-induced myocardial lipid accumulation in long-chain acyl-CoA dehydrogenase knockout mice is accompanied by impaired left ventricular function. Circ Cardiovasc Imaging. (2011) 4:558–65. doi: 10.1161/CIRCIMAGING.111.963751
171. Huang D, Li T, Li X, Zhang L, Sun L, He X, et al. HIF-1-mediated suppression of acyl-CoA dehydrogenases and fatty acid oxidation is critical for cancer progression. Cell Rep. (2014) 8:1930–42. doi: 10.1016/j.celrep.2014.08.028
172. Du W, Zhang L, Brett-Morris A, Aguila B, Kerner J, Hoppel CL, et al. HIF drives lipid deposition and cancer in ccRCC via repression of fatty acid metabolism. Nat Commun. (2017) 8:1769. doi: 10.1038/s41467-017-01965-8
173. Bacmeister L, Schwarzl M, Warnke S, Stoffers B, Blankenberg S, Westermann D, et al. Inflammation and fibrosis in murine models of heart failure. Basic Res Cardiol. (2019) 114:19. doi: 10.1007/s00395-019-0722-5
174. Shen JL, Xie XJ. Insight into the pro-inflammatory and profibrotic role of macrophage in heart failure with preserved ejection fraction. J Cardiovasc Pharmacol. (2020) 76:276–85. doi: 10.1097/FJC.0000000000000858
175. Hewitson TD, Smith ER. A metabolic reprogramming of glycolysis and glutamine metabolism is a requisite for renal fibrogenesis-why and how? Front Physiol. (2021) 12:645857. doi: 10.3389/fphys.2021.645857
176. Hamanaka RB, O'Leary EM, Witt LJ, Tian Y, Gökalp GA, Meliton AY, et al. Glutamine metabolism is required for collagen protein synthesis in lung fibroblasts. Am J Respir Cell Mol Biol. (2019) 61:597–606. doi: 10.1165/rcmb.2019-0008OC
177. Bernard K, Logsdon NJ, Benavides GA, Sanders Y, Zhang J, Darley-Usmar VM, et al. Glutaminolysis is required for transforming growth factor-β1-induced myofibroblast differentiation and activation. J Biol Chem. (2018) 293:1218–28. doi: 10.1074/jbc.RA117.000444
178. Cui H, Xie N, Jiang D, Banerjee S, Ge J, Sanders YY, et al. Inhibition of glutaminase 1 attenuates experimental pulmonary fibrosis. Am J Respir Cell Mol Biol. (2019) 61:492–500. doi: 10.1165/rcmb.2019-0051OC
179. Mprah R, Adzika GK, Gyasi YI, Ndzie Noah ML, Adu-Amankwaah J, Adekunle AO, et al. Glutaminolysis: a driver of vascular and cardiac remodeling in pulmonary arterial hypertension. Front Cardiovasc Med. (2021) 8:667446. doi: 10.3389/fcvm.2021.667446
180. Ryan JJ, Archer SL. The right ventricle in pulmonary arterial hypertension: disorders of metabolism, angiogenesis and adrenergic signaling in right ventricular failure. Circ Res. (2014) 115:176–88. doi: 10.1161/CIRCRESAHA.113.301129
181. Summer G, Kuhn AR, Munts C, Miranda-Silva D, Leite-Moreira AF, Lourenço AP, et al. A directed network analysis of the cardiome identifies molecular pathways contributing to the development of HFpEF. J Mol Cell Cardiol. (2020) 144:66–75. doi: 10.1016/j.yjmcc.2020.05.008
182. Lee HG, Chen Q, Wolfram JA, Richardson SL, Liner A, Siedlak SL, et al. Cell cycle re-entry and mitochondrial defects in myc-mediated hypertrophic cardiomyopathy and heart failure. PLoS ONE. (2009) 4:e7172. doi: 10.1371/journal.pone.0007172
183. Wise DR, DeBerardinis RJ, Mancuso A, Sayed N, Zhang XY, Pfeiffer HK, et al. Myc regulates a transcriptional program that stimulates mitochondrial glutaminolysis and leads to glutamine addiction. Proc Natl Acad Sci USA. (2008) 105:18782-7. doi: 10.1073/pnas.0810199105
184. Munksgaard Thorén M, Vaapil M, Staaf J, Planck M, Johansson ME, Mohlin S, et al. Myc-induced glutaminolysis bypasses HIF-driven glycolysis in hypoxic small cell lung carcinoma cells. Oncotarget. (2017) 8:48983–95. doi: 10.18632/oncotarget.16904
185. Lee EJ, Chung TW, Lee JH, Kim BS, Kim EY, Lee SO, et al. Water-extracted branch of Cinnamomum cassia promotes lung cancer cell apoptosis by inhibiting pyruvate dehydrogenase kinase activity. J Pharmacol Sci. (2018) 138:146–54. doi: 10.1016/j.jphs.2018.10.005
186. Hao L, Mao Y, Park J, Kwon BM, Bae EJ, Park BH. 2'-Hydroxycinnamaldehyde ameliorates imiquimod-induced psoriasiform inflammation by targeting PKM2-STAT3 signaling in mice. Exp Mol Med. (2021) 53:875–84. doi: 10.1038/s12276-021-00620-z
187. Zhang C, Yang L, Geng YD, An FL, Xia YZ, Guo C, et al. Icariside II, a natural mTOR inhibitor, disrupts aberrant energy homeostasis via suppressing mTORC1-4E-BP1 axis in sarcoma cells. Oncotarget. (2016) 7:27819–37. doi: 10.18632/oncotarget.8538
188. Li H, Liang Q, Wang L. Icaritin inhibits glioblastoma cell viability and glycolysis by blocking the IL-6/Stat3 pathway. J Cell Biochem. (2019) 120:7257–64. doi: 10.1002/jcb.28000
189. Brandi J, Cecconi D, Cordani M, Torrens-Mas M, Pacchiana R, Dalla Pozza E, et al. The antioxidant uncoupling protein 2 stimulates hnRNPA2/B1, GLUT1 and PKM2 expression and sensitizes pancreas cancer cells to glycolysis inhibition. Free Radic Biol Med. (2016) 101:305–16. doi: 10.1016/j.freeradbiomed.2016.10.499
190. Ayyasamy V, Owens KM, Desouki MM, Liang P, Bakin A, Thangaraj K, et al. Cellular model of Warburg effect identifies tumor promoting function of UCP2 in breast cancer and its suppression by genipin. PLoS ONE. (2011) 6:e24792. doi: 10.1371/journal.pone.0024792
191. Li W, Hao J, Zhang L, Cheng Z, Deng X, Shu G. Astragalin reduces hexokinase 2 through increasing mir-125b to inhibit the proliferation of hepatocellular carcinoma cells in vitro and in vivo. J Agric Food Chem. (2017) 65:5961–72. doi: 10.1021/acs.jafc.7b02120
192. Wu H, Pan L, Gao C, Xu H, Li Y, Zhang L, et al. Quercetin inhibits the proliferation of glycolysis-addicted HCC cells by reducing hexokinase 2 and Akt-mTOR pathway. Molecules. (2019) 24:1993. doi: 10.3390/molecules24101993
193. Yao S, Wang X, Li C, Zhao T, Jin H, Fang W. Kaempferol inhibits cell proliferation and glycolysis in esophagus squamous cell carcinoma via targeting EGFR signaling pathway. Tumour Biol. (2016) 37:10247–56. doi: 10.1007/s13277-016-4912-6
194. Wu H, Cui M, Li C, Li H, Dai Y, Cui K, et al. Kaempferol reverses aerobic glycolysis via miR-339-5p-Mediated PKM alternative splicing in colon cancer cells. J Agric Food Chem. (2021) 69:3060–8. doi: 10.1021/acs.jafc.0c07640
195. Xu WL, Liu S, Li N, Ye LF, Zha M, Li CY, et al. Quercetin antagonizes glucose fluctuation induced renal injury by inhibiting aerobic glycolysis via HIF-1α/miR-210/ISCU/FeS pathway. Front Med (Lausanne). (2021) 8:656086. doi: 10.3389/fmed.2021.656086
196. Wilson WJ, Poellinger L. The dietary flavonoid quercetin modulates HIF-1 alpha activity in endothelial cells. Biochem Biophys Res Commun. (2002) 293:446–50. doi: 10.1016/S0006-291X(02)00244-9
197. Guo R, Li L, Su J, Li S, Duncan SE, Liu Z, et al. Pharmacological activity and mechanism of tanshinone IIA in related diseases. Drug Des Devel Ther. (2020) 14:4735-48. doi: 10.2147/DDDT.S266911
198. Wang X, Yang Y, Liu X, Gao X. Pharmacological properties of tanshinones, the natural products from Salvia miltiorrhiza. Adv Pharmacol. (2020) 87:43-70. doi: 10.1016/bs.apha.2019.10.001
199. Liu QY, Zhuang Y, Song XR, Niu Q, Sun QS, Li XN, et al. Tanshinone IIA prevents LPS-induced inflammatory responses in mice via inactivation of succinate dehydrogenase in macrophages. Acta Pharmacol Sin. (2020) 42:987-97. doi: 10.1038/s41401-020-00535-x
200. Li M, Gao F, Zhao Q, Zuo H, Liu W, Li W. Tanshinone IIA inhibits oral squamous cell carcinoma via reducing Akt-c-Myc signaling-mediated aerobic glycolysis. Cell Death Dis. (2020) 11:381. doi: 10.1038/s41419-020-2579-9
201. Zhou J, Su CM, Chen HA, Du S, Li CW, Wu H, et al. Cryptanshinone inhibits the glycolysis and inhibits cell migration through PKM2/β-catenin axis in breast cancer. Onco Targets Ther. (2020) 13:8629–39. doi: 10.2147/OTT.S239134
202. Yang Y, Cao Y, Chen L, Liu F, Qi Z, Cheng X, et al. Cryptotanshinone suppresses cell proliferation and glucose metabolism via STAT3/SIRT3 signaling pathway in ovarian cancer cells. Cancer Med. (2018) 7:4610–8. doi: 10.1002/cam4.1691
203. Ma ZJ, Yan H, Wang YJ, Yang Y, Li XB, Shi AC, et al. Proteomics analysis demonstrating rosmarinic acid suppresses cell growth by blocking the glycolytic pathway in human HepG2 cells. Biomed Pharmacother. (2018) 105:334–49. doi: 10.1016/j.biopha.2018.05.129
204. Han S, Yang S, Cai Z, Pan D, Li Z, Huang Z, et al. Anti-Warburg effect of rosmarinic acid via miR-155 in gastric cancer cells. Drug Des Devel Ther. (2015) 9:2695–703. doi: 10.2147/DDDT.S82342
205. Santoro M, Guido C, De Amicis F, Sisci D, Cione E, Vincenza D, et al. Bergapten induces metabolic reprogramming in breast cancer cells. Oncol Rep. (2016) 35:568–76. doi: 10.3892/or.2015.4327
206. Ni F, Tang H, Wang C, Zhang H, Zheng C, Zhang N, et al. Baohuoside I inhibits the proliferation of pancreatic cancer cells via mTOR/S6K1-Caspases/Bcl2/Bax apoptotic signaling. Cancer Manag Res. (2019) 11:10609–21. doi: 10.2147/CMAR.S228926
207. Jiang X, Zhao W, Zhu F, Wu H, Ding X, Bai J, et al. Ligustilide inhibits the proliferation of non-small cell lung cancer via glycolytic metabolism. Toxicol Appl Pharmacol. (2021) 410:115336. doi: 10.1016/j.taap.2020.115336
208. Wang Y, Ma J, Yan X, Chen X, Si L, Liu Y, et al. Isoliquiritigenin Inhibits proliferation and induces apoptosis via alleviating hypoxia and reducing glycolysis in mouse melanoma B16F10 cells. Recent Pat Anticancer Drug Discov. (2016) 11:215–27. doi: 10.2174/1573406412666160307151904
209. Siddiqui FA, Prakasam G, Chattopadhyay S, Rehman AU, Padder RA, Ansari MA, et al. Curcumin decreases Warburg effect in cancer cells by down-regulating pyruvate kinase M2 via mTOR-HIF1α inhibition. Sci Rep. (2018) 8:8323. doi: 10.1038/s41598-018-25524-3
210. Eaton S, Fukumoto K, Stefanutti G, Spitz L, Zammit VA, Pierro A. Myocardial carnitine palmitoyltransferase I as a target for oxidative modification in inflammation and sepsis. Biochem Soc Trans. (2003) 31:1133–6. doi: 10.1042/bst0311133
211. Zhao L, Jiang SJ, Lu FE, Xu LJ, Zou X, Wang KF, et al. Effects of berberine and cinnamic acid on palmitic acid-induced intracellular triglyceride accumulation in NIT-1 pancreatic β cells. Chin J Integr Med. (2016) 22:496–502. doi: 10.1007/s11655-014-1986-0
212. Thach TT, Lee CK, Park HW, Lee SJ, Lee SJ. Syringaresinol induces mitochondrial biogenesis through activation of PPARβ pathway in skeletal muscle cells. Bioorg Med Chem Lett. (2016) 26:3978–83. doi: 10.1016/j.bmcl.2016.07.001
213. Velasco G, Gómez del Pulgar T, Carling D, Guzmán M. Evidence that the AMP-activated protein kinase stimulates rat liver carnitine palmitoyltransferase I by phosphorylating cytoskeletal components. FEBS Lett. (1998) 439:317–20. doi: 10.1016/S0014-5793(98)01400-8
214. Zhang J, Liang X, Li J, Yin H, Liu F, Hu C, et al. Apigenin attenuates acetaminophen-induced hepatotoxicity by activating AMP-activated protein kinase/carnitine palmitoyltransferase I pathway. Front Pharmacol. (2020) 11:549057. doi: 10.3389/fphar.2020.549057
215. Li YC, Qiao JY, Wang BY, Bai M, Shen JD, Cheng YX. Paeoniflorin ameliorates fructose-induced insulin resistance and hepatic steatosis by activating LKB1/AMPK and AKT pathways. Nutrients. (2018) 10:1024. doi: 10.3390/nu10081024
216. Wei B, You MG, Ling JJ, Wei LL, Wang K, Li WW, et al. Regulation of antioxidant system, lipids and fatty acid β-oxidation contributes to the cardioprotective effect of sodium tanshinone IIA sulphonate in isoproterenol-induced myocardial infarction in rats. Atherosclerosis. (2013) 230:148–56. doi: 10.1016/j.atherosclerosis.2013.07.005
217. Huang B, Yuan HD, Kim DY, Quan HY, Chung SH. Cinnamaldehyde prevents adipocyte differentiation and adipogenesis via regulation of peroxisome proliferator-activated receptor-γ (PPARγ) and AMP-activated protein kinase (AMPK) pathways. J Agric Food Chem. (2011) 59:3666–73. doi: 10.1021/jf104814t
218. Kim SG, Kim JR, Choi HC. Quercetin-induced AMP-activated protein kinase activation attenuates vasoconstriction through LKB1-ampk signaling pathway. J Med Food. (2018) 21:146–53. doi: 10.1089/jmf.2017.4052
219. Dang Y, Ling S, Duan J, Ma J, Ni R, Xu JW. Bavachalcone-induced manganese superoxide dismutase expression through the AMP-activated protein kinase pathway in human endothelial cells. Pharmacology. (2015) 95:105–10. doi: 10.1159/000375452
220. Seo E, Oh YS, Kim D, Lee MY, Chae S, Jun HS. Protective role of Psoralea corylifolia L. seed extract against hepatic mitochondrial dysfunction induced by oxidative stress or aging. Evid Based Complement Alternat Med. (2013) 2013:678028. doi: 10.1155/2013/678028
221. Qi ZG, Zhao X, Zhong W, Xie ML. Osthole improves glucose and lipid metabolism via modulation of PPARα/γ-mediated target gene expression in liver, adipose tissue, and skeletal muscle in fatty liver rats. Pharm Biol. (2016) 54:882–8. doi: 10.3109/13880209.2015.1089295
222. Rees ML, Subramaniam J, Li Y, Hamilton DJ, Frazier OH, Taegtmeyer H. A PKM2 signature in the failing heart. Biochem Biophys Res Commun. (2015) 459:430–6. doi: 10.1016/j.bbrc.2015.02.122
223. Wu H, Li Z, Yang P, Zhang L, Fan Y, Li Z. PKM2 depletion induces the compensation of glutaminolysis through β-catenin/c-Myc pathway in tumor cells. Cell Signal. (2014) 26:2397–405. doi: 10.1016/j.cellsig.2014.07.024
224. Zhang HS, Zhang FJ, Li H, Liu Y, Du GY, Huang YH. Tanshinone IIA inhibits human esophageal cancer cell growth through miR-122-mediated PKM2 down-regulation. Arch Biochem Biophys. (2016) 598:50–6. doi: 10.1016/j.abb.2016.03.031
Glossary
6MWD: 6-min walking distance;
11β-HSD2: 11 beta-hydroxysteroid dehydrogenase-2;
AAT: anti-aging tablet;
ABC transporters: ATP-binding cassette transporters;
ACE: angiotensin-converting enzyme;
ACHE: acetylcholinesterase;
ACSL1: acyl-CoA synthase-1;
ADRA: adrenoceptor alpha subtype;
ADRBK1: androgenic, beta, receptor kinase 1;
AGTR1/AT1 receptor: angiotensin II receptor type 1;
AHR: aryl hydrocarbon receptor;
AKR1C3: aldo-keto reductase family 1 member C3;
ALD: aldosterone;
ALOX5: arachidonate 5-lipoxygenase;
AMP: adenosine monophosphate;
AMPK: AMP-activated protein kinase;
Ang II: angiotensin II;
ANP: atrial natriuretic peptide;
AQP-2: aquaporin 2;
AR: androgen receptor;
ARNT: aryl hydrocarbon receptor nuclear translocator;
ATP: adenosine triphosphate;
ATP1A1/2: ATPase Na+/K+ transporting subunit alpha 1;
AVP: arginine vasopressin;
BCL2: BCL2 apoptosis regulator/B-cell CLL/lymphoma 2;
BNP: brain natriuretic peptide;
Ca2+-ATPase: calcium pump in sarcoplasmic reticulum or plasma membrane;
CACNA1G/L: low-voltage-activated Ca (v)3.1 T-type calcium channel subtypes G/L;
CASP: caspase;
CAT: catalase;
CCE: composite cardiac event;
cGMP: cyclic guanosine monophosphate;
CHRM1: cholinergic receptor muscarinic 1;
CK-MB: creatine kinase-MB;
CI: cardiac index;
CO: cardiac output;
COX-2/ PTGS2: cyclooxygenase 2 or prostaglandin-endoperoxide synthase 2;
CPS: carbamyl phosphate synthase;
CPT1: carnitine palmitoyltransferase 1;
CRH: corticotropin-releasing hormone;
CSAR: cardiac sympathetic afferent reflex;
CYP: cytochrome P450;
DLD: dihydrolipoamide dehydrogenase;
E/A ratio: the ratio of the early (E) to late (A) ventricular filling velocities;
EF: ejection fraction;
EGFR: epidermal growth factor receptor;
eNOS/NOS3: endothelial nitric oxide synthase;
EPO: erythropoietin;
ERK1/2: extracellular signal-regulated kinases 1/2;
ESR1: estrogen receptor alpha;
FGF23: fibroblast growth factor 23;
FS: fractional shortening;
GLUT1: glucose transporter type 1;
GR: glutathione reductase;
GSTM1/2: glutathione S-transferase mu 1;
GSTP1: glutathione S-transferase pi 1;
GUCY1B3: guanylate cyclase 1 soluble subunit beta 3;
HFpEF: heart failure with reduced ejection fraction;
HIF-1α: hypoxia inducible factor-1alpha;
HK2: hexokinase 2;
HMGB1: high mobility group box 1;
hnRNPA2/B1: heterogeneous nuclear ribonucleoprotein A2/B1;
HO-1: heme oxygenase-1;
HR: heart rate;
hs-CRP: high-sensitivity C-reactive protein;
ICAM1: intercellular adhesion molecule 1;
ICR: the Institute of Cancer Research in the USA;
IFN-γ: interferon-gamma;
IKBKB: inhibitor of nuclear factor kappa B kinase subunit beta;
IL-1β: interleukin-1beta;
IL-6: interleukin-6;
iNOS/NOS2: inducible nitric oxide synthase;
JNK: c-Jun N-terminal kinase;
JSP: Jinkui Shenqi pill;
α-KGDH: alpha ketoglutarate dehydrogenase;
KM: Kunming;
LCAD: long-chain 3-hydroxyacyl-CoA dehydrogenase;
LCFA: long-chain fatty acids;
LDH: lactate dehydrogenase;
LOX-1: lectin-like oxidized low-density lipoprotein receptor-1;
LPS: lipopolysaccharides;
LVDD: left ventricular diastolic dysfunction;
LVEDd: left ventricle end diastolic dimension;
LVEDV: left ventricular end-diastolic volume;
LVEF: left ventricular ejection fraction;
LVESd: left ventricular end-systolic diameter;
LVESV: left ventricular end systolic volume;
LVSD: left ventricular systolic dysfunction;
MAPK: mitogen-activated protein kinase;
MCAD: acyl CoA dehydrogenase;
MCP-1: monocyte chemoattractant protein-1;
MLHFQ: Minnesota living with heart failure questionnaire;
MMP-2/9: matrix metallopeptidase 2/9;
MR: mineralocorticoid receptor;
mTOR: mammalian target of rapamycin;
Mϕ: macrophages;
Na+/K+-ATPase: sodium-potassium pump in outer plasma membrane;
NCOA1: nuclear receptor coactivator 1;
NE: norepinephrine;
NF-κB: nuclear factor kappa light chain enhancer of activated B cells;
NK cells: natural killer cells;
NLR: nucleotide-binding domain, leucine rich repeat containing receptor;
NLRP3: NLR family pyrin domain containing 3;
NO: nitric oxide;
NOX: nicotinamide adenine dinucleotide phosphate oxidase;
Nrf2: nuclear factor erythroid 2-related factor 2;
NT-proBNP: N-terminal pro-B-type natriuretic peptide;
NYHA: New York Heart Association;
ox-LDL: oxidized low-density lipoprotein;
OXPHOS: oxidative phosphorylation;
PaO2: partial pressure of oxygen;
PaCO2: partial pressure of carbon dioxide;
PDH: pyruvate dehydrogenase;
PDK: pyruvate dehydrogenase kinase;
PGC-1α: peroxisome proliferator-activated receptor-gamma coactivator-1alpha;
PGE2: prostaglandin E2;
PGR: progesterone receptor;
PI3K: phosphatidylinositol-3 kinase;
PKG: cyclic GMP-dependent protein kinase;
PKM2: pyruvate kinase M2;
PLAU: plasminogen activator, urokinase;
PON1: paraoxonase 1;
PPARs: peroxisome proliferator-activated receptors;
PRKCA: protein kinase C alpha;
PVN: paraventricular nucleus;
RCRI/Lee's score: revised cardiac risk index score;
RELA: RELA proto-oncogene, NF-kB subunit;
RVID: right ventricular internal diameter;
RVOT: right ventricular outflow tract;
SaO2: oxygen saturation;
SARDH: sarcosine dehydrogenase;
SV: stroke volume;
SD: Sprague-Dawley;
SDH: succinate dehydrogenase complex;
SELE: selectin E;
SFO: subfornical organ;
SI: stimulation index;
SLP: splenic lymphocyte proliferation;
SOD: superoxide dismutase;
Stat3: signal transducer and activator of transcription 3;
TAC: tricarboxylic acid cycle;
TC: therapeutic compound;
TCM: traditional Chinese medicine;
TGF-β1: transforming growth factor-beta1;
TLR: Toll-like receptor;
TNF-α: tumor necrosis factor-alpha;
TRPC6: canonical transient receptor potential channel 6;
UTR: urotensin II receptor;
VCAM1: vascular cell adhesion molecule 1;
VEGFA: vascular endothelial growth factor A;
WBC: white blood cell;
Yang-Qi: Yang energy.
Keywords: chronic heart failure, classical prescription, inflammation, neurohumoral factors, energy metabolism
Citation: Chen L, Yu D, Ling S and Xu J-W (2022) Mechanism of tonifying-kidney Chinese herbal medicine in the treatment of chronic heart failure. Front. Cardiovasc. Med. 9:988360. doi: 10.3389/fcvm.2022.988360
Received: 07 July 2022; Accepted: 22 August 2022;
Published: 12 September 2022.
Edited by:
Jun Yu, Temple University, United StatesReviewed by:
Zhi Yang, General Hospital of Guangzhou Military Command, ChinaSihai Zhao, Xi'an Jiaotong University, China
Copyright © 2022 Chen, Yu, Ling and Xu. This is an open-access article distributed under the terms of the Creative Commons Attribution License (CC BY). The use, distribution or reproduction in other forums is permitted, provided the original author(s) and the copyright owner(s) are credited and that the original publication in this journal is cited, in accordance with accepted academic practice. No use, distribution or reproduction is permitted which does not comply with these terms.
*Correspondence: Shuang Ling, c2FyYWhfbGluZyYjeDAwMDQwOzEyNi5jb20=; Jin-Wen Xu, and4dTEwMDEmI3gwMDA0MDsxNjMuY29t
†ORCID: Shuang Ling orcid.org/0000-0002-2370-169X
Jin-Wen Xu orcid.org/0000-0001-6153-0003