- 1General Practice Ward/International Medical Center Ward, General Practice Medical Center, West China Hospital, Sichuan University, Chengdu, China
- 2Department of Emergency Medicine and National Clinical Research Center for Geriatrics, Disaster Medicine Center, West China Hospital, Sichuan University West China School of Medicine, Chengdu, China
- 3Institute of Biomedical Engineering, West China School of Basic Medical Sciences and Forensic Medicine, Sichuan University, Chengdu, China
Pyroptosis is primarily considered a pro-inflammatory class of caspase-1- and gasdermin D (GSDMD)-dependent programmed cell death. Inflammasome activation promotes the maturation and release of interleukin (IL)-1β and IL-18, cleavage of GSDMD, and development of pyroptosis. Recent studies have reported that NLRP3 inflammasome activation-mediated pyroptosis aggravates the formation and development of diabetes cardiomyopathy (DCM). These studies provide theoretical mechanisms for exploring a novel approach to treat DCM-associated cardiac dysfunction. Accordingly, this review aims to summarize studies that investigated possible DCM therapies targeting pyroptosis and elucidate the molecular mechanisms underlying NLRP3 inflammasome-mediated pyroptosis, and its potential association with the pathogenesis of DCM. This review may serve as a basis for the development of potential pharmacological agents as novel and effective treatments for managing and treating DCM.
Introduction
Diabetes and heart failure have a bidirectional link. The prevalence of diabetes in patients with heart failure caused by cardiomyopathy ranges from 10 to 40% (1). Meanwhile, heart failure is a common and serious cardiovascular complication in patients with diabetes. The Framingham Heart Study showed that the incidence of heart failure is two- to fivefold higher in patients with diabetes compared with that in healthy individuals (2). In addition, pre-diabetes is also related to an elevated risk of heart failure, and the relative risks is 1.09–1.40 according to different diagnosis criteria (3). Notably, both pre-diabetes and diabetes are associated with an increasing risk of cardiac events and mortality in patients with heart failure (4, 5). Therefore, glycometabolism disorder is an important hazard factor for heart failure, and the two potential mechanisms are as follows: promoting the development of coronary atherosclerotic stenosis, which leads to ischemic heart disease characterized by systolic dysfunction; and more importantly, the classic presentation of diabetes, namely, diabetes cardiomyopathy (DCM) (1).
Diabetes cardiomyopathy is characterized by cardiac changes in function, metabolism, and structure without typical chronic cardiovascular complications, such as valvular heart disease, hypertension, and ischemic heart disease (6). DCM is the most frequent complication of diabetes and causes myocardial fibrosis, ventricular enlargement, and cardiac dysfunction, ultimately leading to clinical heart failure (7–9). Owing to its substantial impact on individuals cardiovascular health and lack of relevant targeted therapy, the pathogenesis of DCM has been a trending theme of research.
The abnormal metabolism of DCM is primarily due to myocardial tissue insulin resistance, compensatory hyperinsulinemia, and hyperglycemia, resulting in several conditions, including glycolipid metabolic disorders, oxidative stress, and advanced glycation end product deposition (1, 10). Previous review had well summarized the mechanisms of DCM, such as mitochondrial dysfunction, endoplasmic reticulum stress, and inflammation (11–13). Among the multiple mechanisms of DCM, cardiomyocyte death is a terminal pathway during the development of DCM, following by systolic dysfunction, myocardial compensatory hypertrophy, cardiac fibrosis, and electrocardiographic conduction disorder (14). Previous studies have analyzed that development of DCM caused by cardiomyocyte death, involving apoptosis, autophagy, necrosis, and entosis, and recent evidence obtained using electron microscopy has shown that pyroptosis-regulated cell death (pyroptosis) is a key pathogenetic factor in diabetes and DCM (15–17). Subsequently, an increasing number of pre-clinical studies have investigated the association between pyroptosis and DCM. Several molecular mechanisms have been elucidated, however, further related research is warranted.
Mechanisms of pyroptosis
Pyroptosis presented as programmed and inflammatory cell death and characterized by caspase-1- and gasdermin D (GSDMD)-mediated formation of plasma membrane pores, following by cell lysis and the secretion of proinflammatory cytokines, such as IL-1β and IL-18, and cellular component (18). Pathogen associated molecular patterns (PAMPs) and damage associated molecular patterns (DAMPs) are identified by pattern recognition receptors (PRRs) to activate the intrinsic immune reaction (19–22). PRRs is divided into cell membrane PRR and cytoplasmic PRR according to receptors site. The former is expressed on the membrane of immunocyte, commonly known as Toll-like receptors (TLRs), which can identify the exogenous infection signals of the intracellular environment (20). The latter expressed in cytoplasm, it can identify invasive pathogens; The most common are retinoic acid-inducible gene I-like receptors, absent in melanoma 2 (AIM2)-like receptors (ALRs) and nucleotide-binding oligomerization domain (NOD)-like receptors (NLRs) (22–25).
When the ALRs and NLRs recognize DAMPs and PAMPs, the caspase-1 activated complex initiates assembly, this process is called formation of inflammasome (26). Further, it was regard as a processor of pro-caspases-1 to active caspase-1, which subsequently promoting maturation and release of IL-1β and IL-18 from precursor (26). Thus, inflammasome mainly contain three components: caspase-1, apoptosis-associated speck-like protein containing a caspase recruitment domain (ASC), and receptors. According to different receptors, inflammasomes mainly classified as AIM2, NOD-like receptor protein 1 (NLRP1), NLRP3, NLR family CARD-domain containing protein 4 (NLRC4), and NLRP6 inflammasomes. Although multiple kinds of inflammasomes are being intensively studied, NLRP3 inflammasome is currently the most studied, with the most abundant relevant evidence, and the most widely involved in inflammatory and immune diseases. Importantly, accumulating studies have revealed that NLRP3 inflammasome-mediated pyroptosis plays a significant role in inducing the formation and development of DCM. Thus, our current review aims to elucidate the molecular mechanisms of NLRP3 inflammasome-mediated pyroptosis and its potential association with the pathogenesis of DCM (Figure 1). Finally, we summarized the progress of clinical drug research for DCM targeting pyroptosis (Table 1).
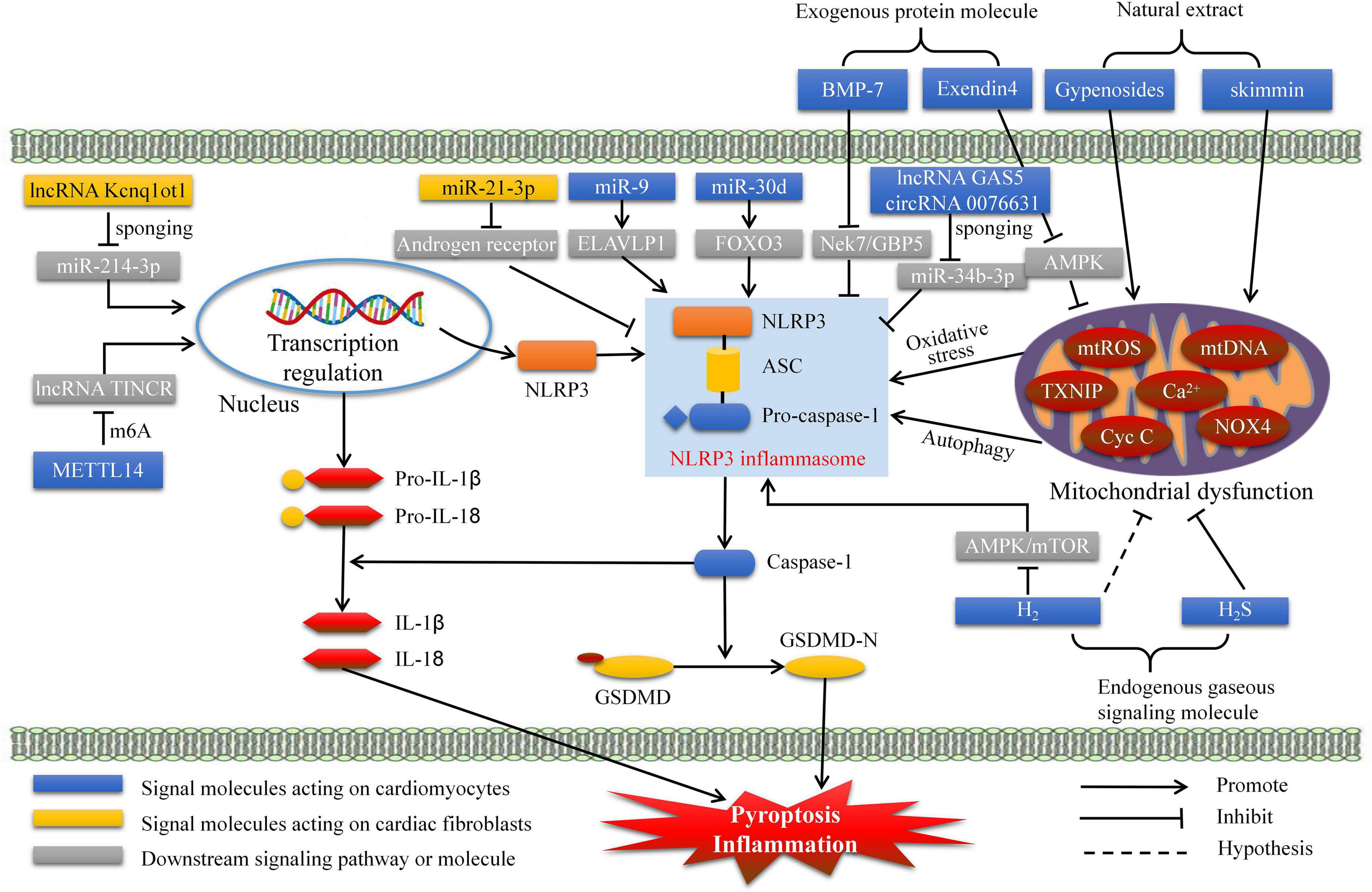
Figure 1. Pre-clinical treatment for DCM targeting NLRP3 inflammasome mediated pyroptosis. DCM, diabetes cardiomyopathy; GSDMD, gasdermin D; H2, hydrogen; H2S, hydrogen sulfide; METTL14, methyltransferase-like 14; BMP-7, bone morphogenetic protein-7; ELAVLP1, ELAV like protein 1; mtROS, mitochondrial reactive oxygen species.
Pre-clinical diabetes cardiomyopathy treatments targeting nucleotide-binding oligomerization domain-like receptor protein 3 inflammasome-mediated pyroptosis
Natural extracts
Cardiac pumping dysfunction in DCM is mainly due to cardiomyocyte injury and death. Thus, suppressing pyroptosis in cardiomyocytes is important. Gypenosides, the principal component of Gynostemma, exert various cardiovascular protective effects, such as reducing blood pressure, improving lipid and glucose metabolism, and inhibiting inflammation (27, 28). Zhang et al. reported that gypenosides can ameliorate high glucose-induced DCM by inhibiting reactive oxygen species (ROS)- and cytochrome c-mediated NLRP3 inflammasome activation and pyroptosis (29). Skimmin is a coumarin and glycoside with several biological activities, including antifibrosis, antioxidation, and anti-inflammation (30, 31). A recent study has shown that skimmin protects against streptozotocin (STZ)-induced DCM by improving autophagy and inhibiting NLRP3 inflammasome-mediated pyroptosis in rat cardiac tissues. Therefore, ginsenosides and skimmin are promising therapeutic drugs for DCM treatment.
Non-coding RNAs
Studies in recent years have recognized the important physiological roles of non-coding RNAs (ncRNAs), including circular RNAs (circRNAs), long ncRNAs (lncRNAs), and microRNAs (miRNAs) (32). These ncRNAs are related to DCM through transcriptional and post-transcriptional regulation but not directly involved in protein translation. For example, miRNA-9 expression is downregulated in the cardiac tissue of patients with diabetes and in cardiomyocytes treated with high glucose (33). Furthermore, miRNA-9 mimics inhibit pyroptosis (determined from the caspase-1 and IL-1β levels) in cardiomyocytes by targeting ELAV-like protein 1 to ameliorate hyperglycemia-induced DCM (33). Moreover, Li et al. observed that miR-30d level was increased and in STZ-treated diabetic rat hearts and high glucose-induced cardiac cell. Next, miR-30d was proved to inhibit Forkhead box O3 activities (apoptosis inhibitor) and exacerbates pyroptosis in DCM (34).
Xu et al. confirmed that GAS5 sponges miR-34b-3p to promote aryl hydrocarbon receptor expression and subsequently suppresses NLRP3 inflammasome-mediated pyroptosis in cardiomyocytes to alleviate DCM (35). Interestingly, Yang et al. reported that caspase-1-associated circRNA (hsa_circ_0076631) also sponges miR-214-3p (endogenous) to enhance high glucose-treated NLRP3 inflammasome activation and pyroptosis in cardiomyocytes (36). In addition, epigenetic regulation of lncRNAs can be modified by N6-methyladenosine (m6A), whose level and activity impact cell pathophysiology (37). Recent research has reported that the overexpression of the lncRNA TINCR enhances cardiomyocyte NLRP3 inflammasome activities, pyroptosis, and DCM, and the epigenetic regulation of TINCR is controlled by methyltransferase-like 14-mediated m6A methylation.
In addition to cardiomyocytes, cardiac fibroblasts are also vulnerable to high glucose levels and exacerbate fibrosis and DCM. Yang et al. reported that lncRNA Kcnq1ot1 activates the caspase-1 and TGF-β1 pathways to aggravate fibrosis and DCM by sponging miR-214-3p in cardiac fibroblasts. Moreover, miR-21-3p expression in cardiac fibroblasts is upregulated under STZ treatment, whereas functional inhibition of miR-21-3p improves pyroptosis and collagen deposition by elevating the androgen receptor. These studies demonstrate that ncRNAs play crucial roles in DCM pathogenesis.
Endogenous gaseous signaling molecules
Endogenous gas signaling molecules serve important physiological and pharmacological functions and are associated with diabetes and related complications. Kar et al. found that hydrogen sulfide can be regulated by physical exercise and serves as a cardioprotective antioxidant that suppresses the activation of NLRP3, IL-1β, IL-18, and caspase-1 (38). Another recent study has demonstrated that hydrogen inhibits cardiomyocyte pyroptosis in cardiac fibroblasts by blocking the AMPK/mTOR/NLRP3 signaling pathway and improves fibrosis by inhibiting the TGF-β1/Smad signaling pathway (39). Moreover, hydrogen, as a therapeutic antioxidant, can reduce intracellular oxygen free radicals and inhibit ROS production (40). Thus, hydrogen inhibits the pathogenesis of DCM through multiple pathways. Hydrogen sulfide and hydrogen have been validated as gaseous signaling molecules that prevent DCM by alleviating pyroptosis.
Exogenous protein molecules
Bone morphogenetic protein-7 (BMP-7), also known as osteogenic protein-1, is used in clinical medicine to treat osteoporosis and fracture (41). BMP-7 inhibits inflammation and improves neovascularization (42, 43). Furthermore, BMP-7 inhibits NLRP3 inflammasome-mediated pyroptosis by blocking Nek7/GBP5 signaling to improve deleterious cardiac function and remodeling (44). Exendin-4, a glucagon-like peptide-1 analog, has an extended half-life because it avoids the clearance of dipeptidyl peptidase IV (45). Numerous studies have emphasized its protective effects on glucose metabolism and cardiac function (46). Additionally, exendin-4 inhibits pyroptosis via the ROS/AMPK/TXNIP/NLPR3 pathway, indicating that exendin-4 is a potential therapeutic drug for DCM (47). Secreted frizzled-related proteins (SFRPs) are a family of secreted proteins, and they were characterized by negative regulation of pyroptosis through Wnt/β-catenin and Notch signaling pathways in cardiovascular disease and inflammatory disease (48, 49). Recent study demonstrated that SFRP5 is a powerful prognostic assessment factor of heart failure for patients with type 2 diabetes (T2D). Thus, SFRPs may be a novel and potential exogenous inhibitory molecules of DCM by targeting pyroptosis (50). It is innovative and significant to carry out related research.
Clinical diabetes cardiomyopathy therapies targeting pyroptosis
Sodium-glucose cotransporter-2 inhibitors
Sodium-glucose cotransporter-2 (SGLT2) inhibitors are a relatively new type of hypoglycemic drug that increases urinary glucose excretion for the treatment of T2D (51, 52). Several clinical trials have revealed that SGLT2 inhibitors exert powerful cardiovascular protective effects, such as empagliflozin, canagliflozin, and dapagliflozin, on patients with T2D (53–55). Furthermore, the EMPRISE trial verified that empagliflozin decreases the risk of hospitalization for heart failure in patients with T2D (56). However, these cardiovascular protective effects of SGLT2 inhibitors cannot be attributed merely to their hypoglycemic and natriuretic effects. Pre-clinical studies revealed that SGLT2 inhibitors attenuate myocardial oxidative stress, fibrosis, and DCM by inhibiting NLRP3 inflammasome-mediated pyroptosis in diabetic mouse heart (57–59). To date, clinical studies confirming that SGLT2 inhibitors can improve DCM are lacking, although two related clinical trials are in progress. The DAPA-MEMRI trial (Identifier: NCT04591639) enrolled heart failure patients with T2D from October 2020 to explore the protective effects of SGLT2 inhibitors on cardiac function and remodeling by using cardiac magnetic resonance imaging (MRI) and echocardiography (Table 1). The results of this study provide direct evidence that SGLT2 inhibitors can improve DCM in humans. However, the other study (Identifier: NCT04200586) has not recruited participants yet (Table 1). These results suggest that SGLT2 is a promising drug for DCM treatment.
Phosphodiesterase type 5 inhibitors
Cyclic guanosine monophosphate-phosphodiesterase type 5 (PDE5) inhibitors have gained attention because they can alleviate cardiac stress responses and improve hypertrophy and cardiac damage from multiple adverse stimuli in clinical and pre-clinical studies (60, 61). Early studies in men with heart failure and preserved ejection fraction showed that PDE5 inhibitors improved pulmonary pressure, cardiac geometry, and pump function (62). A recent RECOGITO study (Identifier: NCT01803828) has enrolled 122 men and women with well-controlled T2D and revealed that treatment with 20 mg tadalafil for 20 weeks can significantly mitigate DCM in men but not in women (63) (Table 1).
Mechanistically, PDE5 inhibitors exert protective effects on the cardiovascular system by activating protein kinase G (PKG), PKG-dependent hydrogen sulfide generation, nitric oxide expression, and glycogen synthase kinase-3β phosphorylation (64–66). In addition to hydrogen sulfide-mediated NLRP3 activation (38, 39), the PDE-5 inhibitor TPN171H, an icariin derivative, displays significant anti-inflammatory activities via suppressing NLRP3 inflammasome-mediated pyroptosis via cathepsin B (67–69). These results indicate that PDE5 inhibitors provide cardioprotection against DCM by inhibiting NLRP3 inflammasome-mediated pyroptosis; however, direct evidence is still lacking.
Aldose reductase inhibitors
Aldose reductase, as a polyol pathway enzyme, is significant upregulated in the conditions of oxidative stress and is the important inducer of the ROS related inflammatory response in diabetes (70). Pal et al. demonstrated that aldose reductase inhibitors prevent NLRP3 inflammasome-mediated pyroptosis and cytokine release in monocytes and STZ-induced diabetic mouse heart (71). Thus, aldose reductase inhibitors targeting NLRP3 inflammasome-mediated pyroptosis may be potential agents for DCM treatment. To the best of our knowledge, only one phase III trial (Identifier: NCT04083339) has been conducted to test the safety and efficacy of AT-001 (aldose reductase inhibitor) in patients with DCM. Although this study was started on 10 September 2019, the anticipated results have not been published yet (Table 1).
Fenofibrate
Fenofibrate is a peroxisome proliferator-activated receptor α agonist that has been widely used in the clinic for several decades because of its remarkable effect of reducing triglycerides (72). It can ameliorate diabetic retinopathy and stimulate angiogenesis by deregulating the activity of the NLRP3 inflammasome in STZ-induced diabetic mice (73, 74). Fenofibrate exerts a considerable protective effect on the heart, but whether it can ameliorate DCM remains unclear. A randomized controlled study (Identifier: NCT01752842) tested whether 160 mg fenofibrate per day for 12 weeks can improve heart muscle function in patients with T2D (Table 1). However, results of this study revealed no significant difference in cardiac diastolic function as measured by E′ (cm/s) and fractional shortening percentage between the placebo and fenofibrate groups.
Alpha-lipoic acid
Alpha-lipoic acid (ALA), also known as thioctic acid, is a vitamin-like sulfur-containing organic compound abundant in human organs and tissues (75). Early studies demonstrated that ALA is involved in improving hyperglycemia and deregulating inflammation (76–78). Recent studies have reported that ALA alleviates dyslipidemia and inflammation by modulating NLRP3 inflammasome activation in rats with high-fat diet- and STZ-induced T2D (79, 80). A randomized controlled study (Identifier: NCT04141475) involving patients diagnosed with diabetes from October 2019 evaluated the effect of ALA (Physiomance Acide Lipoïque Gold) in DCM by measuring the left ventricular ejection fraction (Table 1). The results of this study are worth investigating further.
Conclusion and perspectives
Pyroptosis is primarily considered a pro-inflammatory class of caspase-1- and GSDMD-dependent programmed cell death via the NLRP3 inflammasome. An increasing number of preclinical studies have emphasized that pyroptosis, which is different from apoptosis and necrosis, is involved in the pathogenesis of DCM. For example, natural extracts (derivatives), ncRNAs, endogenous gaseous molecules, and exogenous proteins have been explored and recognized for their key roles in pyroptosis and DCM. These studies offer theoretical mechanisms for developing new drugs to treat DCM-related cardiac dysfunction in the future. In addition, some clinical studies are actively exploring marketed drugs that may treat DCM, such as SGLT2 inhibitors, PDE5 inhibitors, aldose reductase inhibitors, fenofibrate, and ALA. The pharmacology of these drugs involves the inhibition of NLRP3 inflammasome-mediated pyroptosis. Thus, they may be the earliest evidence-based medicine for clinical use. However, basic and clinical investigations are still warranted to establish novel and effective treatments targeting pyroptosis for managing and treating DCM.
Author contributions
YJ, DL, and QZ designed the research. YJ and DL wrote the first draft of the manuscript. JY, QZ, WJ, and XL reviewed the manuscript and provided critical scientific input. QZ had main responsibility for the final content of the manuscript. All authors approved the final draft of the manuscript.
Funding
This work was financially supported by grants from National Key Research and Development Program of China (Nos. 2020AAA0105000 and 2020AAA0105005), Sichuan Science and Technology Program (Nos. 2022YFS0279, 2021YFQ0062, and 2022JDRC0148), Health Commission of Sichuan Province (Nos. ZH2022-101 and ZH2020-104), Sichuan University West China Nursing Discipline Development Special Fund Project (Nos. HXHL20017, HXHL20046, and HXHL21016).
Conflict of interest
The authors declare that the research was conducted in the absence of any commercial or financial relationships that could be construed as a potential conflict of interest.
Publisher’s note
All claims expressed in this article are solely those of the authors and do not necessarily represent those of their affiliated organizations, or those of the publisher, the editors and the reviewers. Any product that may be evaluated in this article, or claim that may be made by its manufacturer, is not guaranteed or endorsed by the publisher.
References
1. Paolillo S, Marsico F, Prastaro M, Renga F, Esposito L, De Martino F, et al. Diabetic cardiomyopathy: definition, diagnosis, and therapeutic implications. Heart Fail Clin. (2019) 15:341–7. doi: 10.1016/j.hfc.2019.02.003
2. Kannel WB, McGee DL. Diabetes and cardiovascular disease. The Framingham study. JAMA. (1979) 241:2035–8. doi: 10.1001/jama.241.19.2035
3. Cai X, Liu X, Sun L, He Y, Zheng S, Zhang Y, et al. Prediabetes and the risk of heart failure: a meta-analysis. Diabetes Obes Metab. (2021) 23:1746–53. doi: 10.1111/dom.14388
4. Mai L, Wen W, Qiu M, Liu X, Sun L, Zheng H, et al. Association between prediabetes and adverse outcomes in heart failure. Diabetes Obes Metab. (2021) 23:2476–83. doi: 10.1111/dom.14490
5. Cavender MA, Steg PG, Smith SC Jr, Eagle K, Ohman EM, Goto S, et al. Impact of diabetes mellitus on hospitalization for heart failure, cardiovascular events, and death: outcomes at 4 years from the reduction of atherothrombosis for continued health (REACH) registry. Circulation. (2015) 132:923–31. doi: 10.1161/CIRCULATIONAHA.114.014796
6. Prandi FR, Evangelista I, Sergi D, Palazzuoli A, Romeo F. Mechanisms of cardiac dysfunction in diabetic cardiomyopathy: molecular abnormalities and phenotypical variants. Heart Fail Rev. (2022). doi: 10.1007/s10741-021-10200-y
7. Jia G, Hill MA, Sowers JR. Diabetic cardiomyopathy: an update of mechanisms contributing to this clinical entity. Circ Res. (2018) 122:624–38. doi: 10.1161/CIRCRESAHA.117.311586
8. Braunwald E. Cardiomyopathies: an overview. Circ Res. (2017) 121:711–21. doi: 10.1161/CIRCRESAHA.117.311812
9. Timmis AD. Diabetic heart disease: clinical considerations. Heart. (2001) 85:463–9. doi: 10.1136/heart.85.4.463
10. Jia G, DeMarco VG, Sowers JR. Insulin resistance and hyperinsulinaemia in diabetic cardiomyopathy. Nat Rev Endocrinol. (2016) 12:144–53. doi: 10.1038/nrendo.2015.216
11. Zheng H, Zhu H, Liu X, Huang X, Huang A, Huang Y. Mitophagy in diabetic cardiomyopathy: roles and mechanisms. Front Cell Dev Biol. (2021) 9:750382. doi: 10.3389/fcell.2021.750382
12. Lin J, Duan J, Wang Q, Xu S, Zhou S, Yao K. Mitochondrial dynamics and mitophagy in cardiometabolic disease. Front Cardiovasc Med. (2022) 9:917135. doi: 10.3389/fcvm.2022.917135
13. Tan Y, Zhang Z, Zheng C, Wintergerst KA, Keller BB, Cai L. Mechanisms of diabetic cardiomyopathy and potential therapeutic strategies: preclinical and clinical evidence. Nat Rev Cardiol. (2020) 17:585–607. doi: 10.1038/s41569-020-0339-2
14. Frustaci A, Kajstura J, Chimenti C, Jakoniuk I, Leri A, Maseri A, et al. Myocardial cell death in human diabetes. Circ Res. (2000) 87:1123–32. doi: 10.1161/01.res.87.12.1123
15. Shen X, Zheng S, Metreveli NS, Epstein PN. Protection of cardiac mitochondria by overexpression of MnSOD reduces diabetic cardiomyopathy. Diabetes. (2006) 55:798–805. doi: 10.2337/diabetes.55.03.06.db05-1039
16. Van Linthout S, Spillmann F, Riad A, Trimpert C, Lievens J, Meloni M, et al. Human apolipoprotein A-I gene transfer reduces the development of experimental diabetic cardiomyopath. Circulation. (2008) 117:1563–73. doi: 10.1161/CIRCULATIONAHA.107.710830
17. Yu W, Wu J, Cai F, Xiang J, Zha W, Fan D, et al. Curcumin alleviates diabetic cardiomyopathy in experimental diabetic rats. PLoS One. (2012) 7:e52013. doi: 10.1371/journal.pone.0052013
18. Cookson BT, Brennan MA. Pro-inflammatory programmed cell death. Trends Microbiol. (2001) 9:113–4. doi: 10.1016/s0966-842x(00)01936-3
19. Kimbrell DA, Beutler B. The evolution and genetics of innate immunity. Nat Rev Genet. (2001) 2:256–67. doi: 10.1038/35066006
20. Imler JL, Hoffmann JA. Toll signaling: the TIReless quest for specificity. Nat Immunol. (2003) 4:105–6. doi: 10.1038/ni0203-105
21. Pedra JH, Cassel SL, Sutterwala FS. Sensing pathogens and danger signals by the inflammasome. Curr Opin Immunol. (2009) 21:10–6. doi: 10.1016/j.coi.2009.01.006
22. Liu X, Lieberman J. A mechanistic understanding of pyroptosis: the fiery death triggered by invasive infection. Adv Immunol. (2017) 135:81–117. doi: 10.1016/bs.ai.2017.02.002
23. Burdette DL, Monroe KM, Sotelo-Troha K, Iwig JS, Eckert B, Hyodo M, et al. STING is a direct innate immune sensor of cyclic di-GMP. Nature. (2011) 478:515–8. doi: 10.1038/nature10429
24. Takeuchi O, Akira S. Pattern recognition receptors and inflammation. Cell. (2010) 140:805–20. doi: 10.1016/j.cell.2010.01.022
25. Sun L, Wu J, Du F, Chen X, Chen ZJ. Cyclic GMP-AMP synthase is a cytosolic DNA sensor that activates the type I interferon pathway. Science. (2013) 339:786–91. doi: 10.1126/science.1232458
26. Martinon F, Burns K, Tschopp J. The inflammasome: a molecular platform triggering activation of inflammatory caspases and processing of proIL-beta. Mol Cell. (2002) 10:417–26. doi: 10.1016/s1097-2765(02)00599-3
27. Zhang HK, Ye Y, Zhao ZN, Li KJ, Du Y, Hu QM, et al. Neuroprotective effects of gypenosides in experimental autoimmune optic neuritis. Int J Ophthalmol. (2017) 10:541–9. doi: 10.18240/ijo.2017.04.07
28. Zhao TT, Kim KS, Shin KS, Park HJ, Kim HJ, Lee KE, et al. Gypenosides ameliorate memory deficits in MPTP-lesioned mouse model of Parkinson’s disease treated with L-DOPA. BMC Complement Altern Med. (2017) 17:449. doi: 10.1186/s12906-017-1959-x
29. Zhang H, Chen X, Zong B, Yuan H, Wang Z, Wei Y, et al. Gypenosides improve diabetic cardiomyopathy by inhibiting ROS-mediated NLRP3 inflammasome activation. J Cell Mol Med. (2018) 22:4437–48. doi: 10.1111/jcmm.13743
30. Zhang S, Ma J, Sheng L, Zhang D, Chen X, Yang J, et al. Total coumarins from hydrangea paniculata show renal protective effects in lipopolysaccharide-induced acute kidney injury via anti-inflammatory and antioxidant activities. Front Pharmacol. (2017) 8:872. doi: 10.3389/fphar.2017.00872
31. Sen Z, Jie M, Jingzhi Y, Dongjie W, Dongming Z, Xiaoguang C. Total coumarins from hydrangea paniculata protect against cisplatin-induced acute kidney damage in mice by suppressing renal inflammation and apoptosis. Evid Based Complement Alternat Med. (2017) 2017:5350161. doi: 10.1155/2017/5350161
32. Ghafouri-Fard S, Shoorei H, Mohaqiq M, Majidpoor J, Moosavi MA, Taheri M. Exploring the role of non-coding RNAs in autophagy. Autophagy. (2022) 18:949–70. doi: 10.1080/15548627.2021.1883881
33. Jeyabal P, Thandavarayan RA, Joladarashi D, Suresh Babu S, Krishnamurthy S, Bhimaraj A, et al. MicroRNA-9 inhibits hyperglycemia-induced pyroptosis in human ventricular cardiomyocytes by targeting ELAVL1. Biochem Biophys Res Commun. (2016) 471:423–9. doi: 10.1016/j.bbrc.2016.02.065
34. Li X, Du N, Zhang Q, Li J, Chen X, Liu X, et al. MicroRNA-30d regulates cardiomyocyte pyroptosis by directly targeting foxo3a in diabetic cardiomyopathy. Cell Death Dis. (2014) 5:e1479. doi: 10.1038/cddis.2014.430
35. Xu Y, Fang H, Xu Q, Xu C, Yang L, Huang C. LncRNA GAS5 inhibits NLRP3 inflammasome activation-mediated pyroptosis in diabetic cardiomyopathy by targeting miR-34b-3p/AHR. Cell Cycle. (2020) 19:3054–65. doi: 10.1080/15384101.2020.1831245
36. Yang F, Li A, Qin Y, Che H, Wang Y, Lv J, et al. A novel circular RNA mediates pyroptosis of diabetic cardiomyopathy by functioning as a competing endogenous RNA. Mol Ther Nucleic Acids. (2019) 17:636–43. doi: 10.1016/j.omtn.2019.06.026
37. Zhang C, Fu J, Zhou Y. A review in research progress concerning m6A methylation and immunoregulation. Front Immunol. (2019) 10:922. doi: 10.3389/fimmu.2019.00922
38. Kar S, Shahshahan HR, Hackfort BT, Yadav SK, Yadav R, Kambis TN, et al. Exercise training promotes cardiac hydrogen sulfide biosynthesis and mitigates pyroptosis to prevent high-fat diet-induced diabetic cardiomyopathy. Antioxidants. (2019) 8:638. doi: 10.3390/antiox8120638
39. Zou R, Nie C, Pan S, Wang B, Hong X, Xi S, et al. Co-administration of hydrogen and metformin exerts cardioprotective effects by inhibiting pyroptosis and fibrosis in diabetic cardiomyopathy. Free Radic Biol Med. (2022) 183:35–50. doi: 10.1016/j.freeradbiomed.2022.03.010
40. Hirano SI, Ichikawa Y, Sato B, Yamamoto H, Takefuji Y, Satoh F. Potential therapeutic applications of hydrogen in chronic inflammatory diseases: possible inhibiting role on mitochondrial stress. Int J Mol Sci. (2021) 22:2549. doi: 10.3390/ijms22052549
41. Cecchi S, Bennet SJ, Arora M. Bone morphogenetic protein-7: review of signalling and efficacy in fracture healing. J Orthop Translat. (2016) 4:28–34. doi: 10.1016/j.jot.2015.08.001
42. Singla DK, Singla R, Wang J. BMP-7 treatment increases M2 macrophage differentiation and reduces inflammation and plaque formation in Apo E-/- Mice. PLoS One. (2016) 11:e0147897. doi: 10.1371/journal.pone.0147897
43. Higgins DF, Ewart LM, Masterson E, Tennant S, Grebnev G, Prunotto M, et al. BMP7-induced-Pten inhibits Akt and prevents renal fibrosis. Biochim Biophys Acta Mol Basis Dis. (2017) 1863:3095–104. doi: 10.1016/j.bbadis.2017.09.011
44. Elmadbouh I, Singla DK. BMP-7 attenuates inflammation-induced pyroptosis and improves cardiac repair in diabetic cardiomyopathy. Cells. (2021) 10:2640. doi: 10.3390/cells10102640
45. Sennik D, Ahmed F, Russell-Jones D. Exenatide, a GLP-1 agonist in the treatment of Type 2 diabetes. Expert Rev Endocrinol Metab. (2012) 7:15–26. doi: 10.1586/eem.11.79
46. Rajabi H, Ahmadi M, Aslani S, Saberianpour S, Rahbarghazi R. Exendin-4 as a versatile therapeutic agent for the amelioration of diabetic changes. Adv Pharm Bull. (2022) 12:237–47. doi: 10.34172/apb.2022.025
47. Wei H, Bu R, Yang Q, Jia J, Li T, Wang Q, et al. Exendin-4 protects against hyperglycemia-induced cardiomyocyte pyroptosis via the AMPK-TXNIP pathway. J Diabetes Res. (2019) 2019:8905917. doi: 10.1155/2019/8905917
48. Huang A, Huang Y. Role of SFRPS in cardiovascular disease. Ther Adv Chronic Dis. (2020) 11:2040622320901990. doi: 10.1177/2040622320901990
49. Jiang P, Wei K, Chang C, Zhao J, Zhang R, Xu L, et al. SFRP1 negatively modulates pyroptosis of fibroblast-like synoviocytes in rheumatoid arthritis: a review. Front Immunol. (2022) 13:903475. doi: 10.3389/fimmu.2022.903475
50. Wu J, Zheng H, Liu X, Chen P, Zhang Y, Luo J, et al. Prognostic value of secreted frizzled-related protein 5 in heart failure patients with and without type 2 diabetes mellitus. Circ Heart Fail. (2020) 13:e007054. doi: 10.1161/CIRCHEARTFAILURE.120.007054
51. Hsia DS, Grove O, Cefalu WT. An update on sodium-glucose co-transporter-2 inhibitors for the treatment of diabetes mellitus. Curr Opin Endocrinol Diabetes Obes. (2017) 24:73–9. doi: 10.1097/MED.0000000000000311
52. Wilding J, Fernando K, Milne N, Evans M, Ali A, Bain S, et al. SGLT2 inhibitors in type 2 diabetes management: key evidence and implications for clinical practice. Diabetes Ther. (2018) 9:1757–73. doi: 10.1007/s13300-018-0471-8
53. Zinman B, Wanner C, Lachin JM, Fitchett D, Bluhmki E, Hantel S, et al. Empagliflozin, cardiovascular outcomes, and mortality in type 2 diabetes. N Engl J Med. (2015) 373:2117–28. doi: 10.1056/NEJMoa1504720
54. Neal B, Perkovic V, Mahaffey KW, de Zeeuw D, Fulcher G, Erondu N, et al. Canagliflozin and cardiovascular and renal events in type 2 diabetes. N Engl J Med. (2017) 377:644–57. doi: 10.1056/NEJMoa1611925
55. Wiviott SD, Raz I, Bonaca MP, Mosenzon O, Kato ET, Cahn A, et al. Dapagliflozin and cardiovascular outcomes in type 2 diabetes. N Engl J Med. (2019) 380:347–57. doi: 10.1056/NEJMoa1812389
56. Patorno E, Pawar A, Franklin JM, Najafzadeh M, Deruaz-Luyet A, Brodovicz KG, et al. Empagliflozin and the risk of heart failure hospitalization in routine clinical care. Circulation. (2019) 139:2822–30. doi: 10.1161/CIRCULATIONAHA.118.039177
57. Li C, Zhang J, Xue M, Li X, Han F, Liu X, et al. SGLT2 inhibition with empagliflozin attenuates myocardial oxidative stress and fibrosis in diabetic mice heart. Cardiovasc Diabetol. (2019) 18:15. doi: 10.1186/s12933-019-0816-2
58. Ye Y, Bajaj M, Yang HC, Perez-Polo JR, Birnbaum Y. SGLT-2 inhibition with dapagliflozin reduces the activation of the Nlrp3/ASC inflammasome and attenuates the development of diabetic cardiomyopathy in mice with type 2 diabetes. further augmentation of the effects with saxagliptin, a DPP4 inhibitor. Cardiovasc Drugs Ther. (2017) 31:119–32. doi: 10.1007/s10557-017-6725-2
59. Kim SR, Lee SG, Kim SH, Kim JH, Choi E, Cho W, et al. SGLT2 inhibition modulates NLRP3 inflammasome activity via ketones and insulin in diabetes with cardiovascular disease. Nat Commun. (2020) 11:2127. doi: 10.1038/s41467-020-15983-6
60. Takimoto E, Champion HC, Li M, Belardi D, Ren S, Rodriguez ER, et al. Chronic inhibition of cyclic GMP phosphodiesterase 5A prevents and reverses cardiac hypertrophy. Nat Med. (2005) 11:214–22. doi: 10.1038/nm1175
61. Roy S, Kloner RA, Salloum FN, Jovin IS. Cardiac effects of phosphodiesterase-5 inhibitors: efficacy and safety. Cardiovasc Drugs Ther. (2021). doi: 10.1007/s10557-021-07275-y
62. Waxman AB. Pulmonary hypertension in heart failure with preserved ejection fraction: a target for therapy? Circulation. (2011) 124:133–5. doi: 10.1161/CIRCULATIONAHA.111.038885
63. Pofi R, Giannetta E, Feola T, Galea N, Barbagallo F, Campolo F, et al. Sex-specific effects of daily tadalafil on diabetic heart kinetics in RECOGITO, a randomized, double-blind, placebo-controlled trial. Sci Transl Med. (2022) 14:eabl8503. doi: 10.1126/scitranslmed.abl8503
64. Das A, Durrant D, Salloum FN, Xi L, Kukreja RC. PDE5 inhibitors as therapeutics for heart disease, diabetes and cancer. Pharmacol Ther. (2015) 147:12–21. doi: 10.1016/j.pharmthera.2014.10.003
65. La Fuente JM, Fernandez A, Pepe-Cardoso AJ, Martinez-Salamanca JI, Louro N, Angulo J. L-cysteine/hydrogen sulfide pathway induces cGMP-dependent relaxation of corpus cavernosum and penile arteries from patients with erectile dysfunction and improves arterial vasodilation induced by PDE5 inhibition. Eur J Pharmacol. (2019) 863:172675. doi: 10.1016/j.ejphar.2019.172675
66. Lee HJ, Feliers D, Mariappan MM, Sataranatarajan K, Choudhury GG, Gorin Y, et al. Tadalafil integrates nitric oxide-hydrogen sulfide signaling to inhibit high glucose-induced matrix protein synthesis in podocytes. J Biol Chem. (2015) 290:12014–26. doi: 10.1074/jbc.M114.615377
67. Zhao C, Hu L, He X, Li L, Yin M, Tettey AT, et al. TPN171H alleviates pulmonary hypertension via inhibiting inflammation in hypoxia and monocrotaline-induced rats. Vascul Pharmacol. (2022) 145:107017. doi: 10.1016/j.vph.2022.107017
68. Wu ZM, Luo J, Shi XD, Zhang SX, Zhu XB, Guo J. Icariin alleviates rheumatoid arthritis via regulating miR-223-3p/NLRP3 signalling axis. Autoimmunity. (2020) 53:450–8. doi: 10.1080/08916934.2020.1836488
69. Su B, Ye H, You X, Ni H, Chen X, Li L. Icariin alleviates murine lupus nephritis via inhibiting NF-kappaB activation pathway and NLRP3 inflammasome. Life Sci. (2018) 208:26–32. doi: 10.1016/j.lfs.2018.07.009
70. Srivastava SK, Ramana KV, Bhatnagar A. Role of aldose reductase and oxidative damage in diabetes and the consequent potential for therapeutic options. Endocr Rev. (2005) 26:380–92. doi: 10.1210/er.2004-0028
71. Pal PB, Sonowal H, Shukla K, Srivastava SK, Ramana KV. Aldose reductase mediates NLRP3 inflammasome-initiated innate immune response in hyperglycemia-induced Thp1 monocytes and male mice. Endocrinology. (2017) 158:3661–75. doi: 10.1210/en.2017-00294
72. McKeage K, Keating GM. Fenofibrate: a review of its use in dyslipidaemia. Drugs. (2011) 71:1917–46. doi: 10.2165/11208090-000000000-00000
73. Deng Y, Han X, Yao Z, Sun Y, Yu J, Cai J, et al. PPARalpha agonist stimulated angiogenesis by improving endothelial precursor cell function via a NLRP3 inflammasome pathway. Cell Physiol Biochem. (2017) 42:2255–66. doi: 10.1159/000479999
74. Liu Q, Zhang F, Zhang X, Cheng R, Ma JX, Yi J, et al. Fenofibrate ameliorates diabetic retinopathy by modulating Nrf2 signaling and NLRP3 inflammasome activation. Mol Cell Biochem. (2018) 445:105–15. doi: 10.1007/s11010-017-3256-x
75. Singh U, Jialal I. Alpha-lipoic acid supplementation and diabetes. Nutr Rev. (2008) 66:646–57. doi: 10.1111/j.1753-4887.2008.00118.x
76. Abdelhalim MAK, Moussa SAA, Qaid HA, Al-Ayed MS. Potential effects of different natural antioxidants on inflammatory damage and oxidative-mediated hepatotoxicity induced by gold nanoparticles. Int J Nanomed. (2018) 13:7931–8. doi: 10.2147/IJN.S171931
77. Castro MC, Villagarcia HG, Massa ML, Francini F. Alpha-lipoic acid and its protective role in fructose induced endocrine-metabolic disturbances. Food Funct. (2019) 10:16–25. doi: 10.1039/c8fo01856a
78. Rochette L, Ghibu S, Muresan A, Vergely C. Alpha-lipoic acid: molecular mechanisms and therapeutic potential in diabetes. Can J Physiol Pharmacol. (2015) 93:1021–7. doi: 10.1139/cjpp-2014-0353
79. Ko CY, Lo YM, Xu JH, Chang WC, Huang DW, Wu JS, et al. Alpha-lipoic acid alleviates NAFLD and triglyceride accumulation in liver via modulating hepatic NLRP3 inflammasome activation pathway in type 2 diabetic rats. Food Sci Nutr. (2021) 9:2733–42. doi: 10.1002/fsn3.2235
Keywords: diabetic cardiomyopathy, pyroptosis, NLRP3, caspase-1, pharmacology
Citation: Jia Y, Li D, Yu J, Jiang W, Liao X and Zhao Q (2022) Potential diabetic cardiomyopathy therapies targeting pyroptosis: A mini review. Front. Cardiovasc. Med. 9:985020. doi: 10.3389/fcvm.2022.985020
Received: 03 July 2022; Accepted: 01 August 2022;
Published: 18 August 2022.
Edited by:
Yuli Huang, Southern Medical University, ChinaReviewed by:
Jiandi Wu, The Second People’s Hospital of Foshan, ChinaCopyright © 2022 Jia, Li, Yu, Jiang, Liao and Zhao. This is an open-access article distributed under the terms of the Creative Commons Attribution License (CC BY). The use, distribution or reproduction in other forums is permitted, provided the original author(s) and the copyright owner(s) are credited and that the original publication in this journal is cited, in accordance with accepted academic practice. No use, distribution or reproduction is permitted which does not comply with these terms.
*Correspondence: Qian Zhao, MjczNTUxNTFAcXEuY29t