- 1Department of Medical Genetics, Life Sciences Institute, The University of British Columbia, Vancouver, BC, Canada
- 2Department of Internal Medicine III (Cardiology and Angiology), Innsbruck Medical University, Innsbruck, Austria
- 3Department of Cardiology, University Heart Center, University Hospital Zurich, Zurich, Switzerland
- 4Institute of Molecular Biotechnology of the Austrian Academy of Sciences, VBC – Vienna BioCenter, Vienna, Austria
Cardiac regeneration is one of the grand challenges in repairing injured human hearts. Numerous studies of signaling pathways and metabolism on cardiac development and disease pave the way for endogenous cardiomyocyte regeneration. New drug delivery approaches, high-throughput screening, as well as novel therapeutic compounds combined with gene editing will facilitate the development of potential cell-free therapeutics. In parallel, progress has been made in the field of cell-based therapies. Transplantation of human pluripotent stem cell (hPSC)-derived cardiomyocytes (hPSC-CMs) can partially rescue the myocardial defects caused by cardiomyocyte loss in large animals. In this review, we summarize current cell-based and cell-free regenerative therapies, discuss the importance of cardiomyocyte maturation in cardiac regenerative medicine, and envision new ways of regeneration for the injured heart.
Introduction
Cardiovascular disease (CVD) remains a leading cause of morbidity and mortality globally. As cardiac regeneration is limited in adults, damaged cardiac regions form compensatory scars with very few functional cardiomyocytes, ultimately resulting in cardiac dysfunction and chronic heart failure. Current clinical therapies have been shown to enhance cardiac function, but none of them is designed to directly address the restoration of cardiomyocyte loss (1). Heart transplantation represents a standard treatment for patients with end-stage heart failure, however, the availability of organ donors is far from adequate to meet demand (2). It is therefore paramount to develop cardiac regenerative medicines.
Over the past two decades, fundamental advances have been made to uncover the cellular and molecular mechanisms of heart development (3, 4). The discovery of multiple signaling pathways and metabolic regulation of cardiac growth and homeostasis has shed light on potential endogenous mechanisms of cardiomyocyte regeneration. Novel drug delivery systems such as the adeno-associated virus 9 (AAV9) system or heart-targeted nanoparticles and the development of novel small molecules might allow for myocardial regeneration approaches in clinical settings (5–7). Moreover, human pluripotent stem cells (hPSCs)-derived cardiomyocytes (hPSC-CMs) have been extensively used for disease modeling and drug screening in CVD (8, 9). With the advancement of hPSC-CM research and cardiac organoid engineering, it has become possible to graft stem cell-derived-CMs into the injured heart, providing directions for optimizing these approaches. In this review, we list some candidates for cell-free regenerative therapy, discuss the transplantation of adult stem cells and hPSC-CMs in cell-based therapy, and envision new regenerative approaches to repair damaged hearts.
Mechanisms underlying cardiac regeneration
Although the adult heart has been shown to lack regenerative capacity in mammals (10, 11), the heart can effectively regenerate within the first week after birth. Studies of apical resection (12, 13) and left anterior descending (LAD) coronary artery ligation (14–16) in neonatal rodents have shown that murine, as well as rat cardiomyocytes, have an intrinsic regenerative capacity within the first 7 days after birth. Similarly, the neonatal porcine heart is capable of regeneration after acute myocardial infarction (MI) during the first 2 days after birth (17). Furthermore, we recently reported the complete functional recovery after a massive MI in a human newborn (18). Compared to the neonatal mammalian heart, adult mammalian cardiomyocytes are highly differentiated and often contain more than one nucleus and well-aligned sarcomeres to maintain cardiac function (19); however, this in turn hinders myocardial regeneration in the adult heart once the heart is damaged (Figure 1). Therefore, inducing mature cardiomyocytes to re-enter the cell cycle from a quiescent state is one of the strategies to repair damaged hearts.
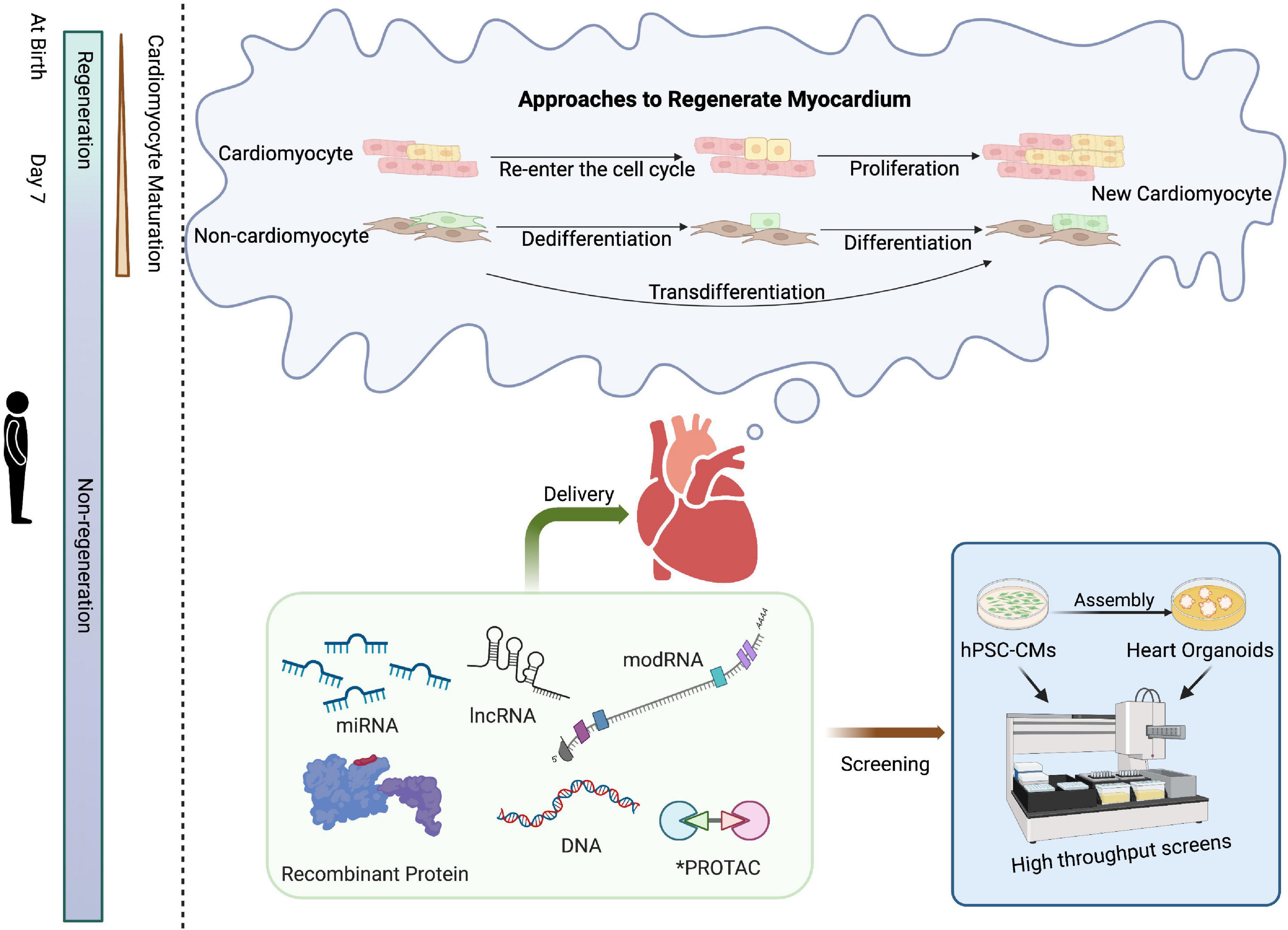
Figure 1. Schematic of approaches for cardiac regenerative medicine using cell-free therapies. Mammals have the intrinsic capability to structurally and functionally regenerate their hearts shortly after birth, a capacity that is subsequently lost. Approaches to cardiac regeneration involve the re-entry of cardiomyocytes into the cell cycle and/or transdifferentiation of other resident cell types into cardiomyocytes. Recombinant proteins, RNA-based drugs, PROTAC, or small molecules could serve as viable strategies for cardiac repair. High-throughput screening of drug candidates can be performed in hPSC-CMs or, at lower throughput, cardiac organoids prior to clinical application. Created with BioRender.com.
To date, extensive studies of neonatal heart regeneration and adult heart repair following injury in mammals have identified fundamental mechanisms underlying cardiac regeneration, providing directions for repair after myocardial injury. The transcription factor GATA4 (20), for example, is known to play an essential role in cardiomyocyte replication in neonatal mice. Myocardial Erbb2 (21) and BMP (22, 23) signaling were found to control cardiomyocyte proliferation. Inhibition of adrenergic receptor (AR) and thyroid hormone (TH) pathways promoted cardiomyocyte regeneration in mice after postnatal day 7 (24). Activation of Neuregulin1/ErbB4 signaling (25) or overexpression of a single transcription factor, namely Tbx20 (22), promoted the repair of damaged adult cardiomyocytes after myocardial infarction in mice and enhanced cardiomyocyte cell-cycle entry. Deletion of Salvador, a component in the Hippo pathway, improved heart function after myocardial infarction (26). Moreover, deletion of the hypoxia response element Meis1 increased the number of cardiomyocytes, especially mononucleated cardiomyocytes in adult mice (27).
In addition to directly inducing cardiomyocyte proliferation, several studies have demonstrated that other cardiac cell types, such as fibroblasts, can transdifferentiate into functional cardiomyocytes, which may be a potential and viable approach to heart regeneration in vivo. A classic combination of transcription factors Gata4, Mef2c, and Tbx5 (GMT) enabled direct reprogramming of postnatal cardiac or dermal fibroblasts into spontaneously contracting cardiomyocyte-like cells with cardiac-specific markers and contracted spontaneously (28). One study showed that blocking TGF-β and WNT signaling increased the efficiency of reprogramming in GMT-overexpressing cardiac fibroblasts. In vivo, mice treated with GMT, TGF-β inhibitor SB431542, and WNT inhibitor XAV939 for 2 weeks after myocardial infarction significantly improved reprogramming and cardiac function compared to mice treated with GMT only (29). In addition, the transcription factor Tead1 (Td) could efficiently replace Tbx5 in the GMT cocktail, enhancing reprogramming efficacy (30). Such reprogramming can also be achieved by chemical induction alone. A combination of nine compounds induced the transdifferentiation of fibroblasts into contracting cardiomyocyte-like cells (31). Importantly, fibroblasts can be directly reprogrammed to spontaneously contracting patches of differentiated cardiomyocytes without a pluripotent intermediate through transgenic expression of Oct4, Sox2, Klf4, and c-Myc (32). Recent studies have shown that in addition to fibroblasts, endocardial cells have the potential to generate cardiomyocytes (33). For example, the deletion of the stem cell leukemia (SCL) gene induces the expression of cardiac-specific proteins in endothelial cells (34).
Numerous studies have uncovered mechanisms that promote cardiac regeneration, and artificially increasing or decreasing these critical molecules in vivo may alleviate or even rescue the pathogenesis heart disease process. Thus, the discovery of druggable regenerative targets is vital to cell-free therapies.
Cell-free therapies
For cardiac repair, recombinant DNA, RNA-based, or recombinant protein therapeutics have been used in regenerative medicine. Here, we discuss some potential drug/molecule candidates for cell-free therapies based on preclinical reports of cardiac regeneration (Table 1).
In murine MI models, for example, injection of Neuregulin1 induced a sustained improvement in myocardial function and attenuated compensatory hypertrophy following MI (25). Adenoviral-based delivery of cyclin A2 increased myofilament density at the border zone of the MI and improved cardiac function (35). Moreover, cardiac-specific overexpression of FGF16 via AAV subtype 9 (AAV9) led to an upregulation of genes associated with cell proliferation in Gata4-ablated mouse hearts (20). Combined intramyocardial injection of CDK1/CCNB/CDK4/CCND significantly improved ejection fraction (EF), stroke volume, cardiac output, and markedly reduced the scar size (36). Down-regulation of Lrp6, a Wnt co-receptor, promoted adult post-MI cardiac repair by increasing cardiomyocyte proliferation (37). Delivery of IGF2BP3 through AAV9-Igf2bp3 into neonatal mouse hearts 3 days prior to LAD ligation significantly improved heart function as determined at 3-weeks post-injury (38). Some RNAs are potential targets for cardiac regeneration. For example, silencing miR-99/100 and Let-7 can induce cardiomyocyte dedifferentiation and improve heart function in adult LAD-treated mice (39). Knockdown of LncDACH1 using LncDACH1 shRNA (Adv-shLncDACH1) reactivated cardiomyocyte proliferation in adult mice and enhanced cardiac function in the injured heart (40). Delivery of Pkm2 modified RNA (modRNA) in mice hearts can increase cardiomyocyte cell proliferation and improve cardiac function after myocardial infarction (41). Moreover, one study showed chronic hypoxia-induced cardiac regeneration in adult mice. Long-term low oxygen treatment induced cardiomyocyte proliferation and angiogenesis in vivo, thereby reducing myocardial fibrosis and improving left ventricular systolic function in mice with myocardial infarction (42). In addition, induction of non-cardiomyocyte transdifferentiation into cardiomyocytes in vivo can also be achieved. Direct intramyocardial injection of GMT transdifferentiated non-cardiomyocytes into new cardiomyocyte-like cells, decreased infarct size, and attenuated cardiac dysfunction after myocardial infarction in mice hearts (43).
In swine MI models, cardiomyocyte hypertrophy and fibrosis following chronic MI were reduced when IGF-1/HGF was intramyocardially delivered into the injured area (44). Subcutaneous injection of a daily dose of growth hormone-releasing hormone agonist (GHRH-A) into pigs with a LAD ligation showed left ventricular structural and functional improvements, whereas cardiomyocyte proliferation was not significantly altered (45). In addition, the cardiomyogenic factor Follistatin Like 1 (FSTL1), produced by the epicardium, can stimulate recovery of contractile function within 2 weeks and limit fibrosis 4 weeks after MI injury, suggesting that FSTL1 has therapeutic efficacy in a large animal MI I/R swine model (46).
Although there many targets for cardiac regeneration have been identified and validated in animal models, the drugs currently available for clinical application are limited. The development of human cardiomyocytes from pluripotent stem cells will undoubtedly help test delivery systems and screen novel drugs for cardiac regeneration in a human “background” since hPSC-CMs from patients can also be used for preclinical tests for drug toxicity, thus enabling more precise and personalized treatments (47, 48). For example, one study designed an engineered bivalent neuregulin-1β that attenuates doxorubicin-induced double-strand DNA breaks in hPSC-CMs, with the vision to utilize such treatment to protect the heart from doxorubicin cardiotoxicity (49). hPSC-CMs from Arg663His-mutated patients can be treated with the L-type Ca2+ channel blocker verapamil to avoid the development of the hypertrophic cardiomyopathy phenotype in vitro. Therefore, verapamil might be a potential drug for patients with Arg663His-mutated hypertrophic cardiomyopathy (50). Compared with 2D hPSC-CMs, human cardiac organoids generated from human pluripotent stem cells through cell self-assembly (51) and 3D printing (52) are more similar in the structure and function of the human heart. Combined with gene editing, these 3D tissues can now be used to model various cardiovascular diseases such as myocardial infarction (51) and thus can ultimately be used as models for screening a collection of drug candidates (Figure 1).
Cell-based therapies for cardiac regeneration
Heart transplantation is currently the only restorative therapy for end-stage heart failure patients. Although the development of new drugs and surgical as well as improved storage techniques have led to an increase in successful heart transplantations, heart transplantation is still a high-risk medical procedure, and there remains an insufficient amount of donor hearts. In addition, immunosuppression is required after heart transplantation, which is a risk factor for complications. In recent years, cell-based therapies have been proposed as a promising approach for treating advanced heart failure and repairing damaged myocardial tissue.
Adult stem cells transplantations
Early evidence suggested that adult stem cells such as bone marrow cells (BMCs), bone marrow-purified haematopoietic stem cells (HSCs), and bone marrow-purified mesenchymal stem cells (MSCs) can differentiate into cardiomyocytes. A 2001 study showed that 9 days after transplantation of c-kit+ BMCs in a LAD mouse model, newly formed myocytes occupied 68% of the infarcted region in the ventricle leading to an overall improvement in cardiac function (53). Then, one report claimed that the grafts of c-Kit+, stem cell antigen-1 positive (Sca-1+) BMCs migrated to ischemic areas where they differentiated into cardiomyocytes and endothelial cells (54). C-kit+ cells (55) and Sca-1+ cells (56, 57)were hence considered as adult cardiac stem/progenitor cells (CPCs). However, multiple follow-up studies showed negative results (58, 59). One study found that transplantation of HSCs into adult mouse hearts did not result in any detectable transdifferentiation into cardiomyocytes, nor was there a significant increase in cardiomyocytes in the HSCs-treated hearts (58). Likewise, multiple laboratories have demonstrated that the transplantation of c-kit+ cells into infarcted adult mouse hearts did not result in the differentiation of cardiomyocytes (60, 61). Additional studies further showed that Sca-1+ cells do not generate new cardiomyocytes (62–64), but are rather precursors of endothelial cells (62). Moreover, lineage-tracing techniques have confirmed that both c-kit+ and Sca1+ adult stem cells in transplanted mice cannot differentiate into cardiomyocytes in vivo (62–67). Thus, the concept of adult cardiac stem cells, as well as the idea that adult stem/progenitor cells can promote cardiac remuscularization, have been rejected.
Nonetheless, numerous clinical trials of bone marrow-derived adult stem cell transplantation have been conducted [reviewed in (68–70)]. As expected from foundational research, the overall clinical benefit was not significant. To date, there is growing evidence that the minute benefits of adult stem cell therapy could be attributed to the effects of secreted factors acting on neighboring cells through a paracrine mechanism (69, 70). Several key secreted growth factors have been identified, such as VEGF, HGF, IGF-1, and TGF-β, mediators that stimulate angiogenesis, inhibit apoptosis or modulate inflammatory pathways (71, 72). In addition, exosomes might be one of the reasons for the improvement of cardiac function after such adult stem cell transplantation. Treating the infarcted area with exosomes secreted by cardiac mesenchymal stem cells can enhance cardiac angiogenesis, promote cardiomyocyte proliferation, and maintain cardiac function in mouse hearts (73). In addition, one study found that both live and dead adult stem cells induced macrophage accumulation in the infarcted area of hearts, improving the heart function after I/R injury, which also occurred after the direct induction of innate immune response. Thus, the recovery of the infarcted area of the heart following adult stem cell therapy may attribute to an acute inflammatory wound-healing response through the accumulation of regional macrophages (74).
Pluripotent stem cell-based therapies for cardiac regeneration
Human embryonic stem cells (ESCs) have the ability to differentiate into multiple cell types and thus have great therapeutic potential in regenerative medicine. However, because human ESCs are extracted from blastocysts, both scientific research and clinical applications of human ESCs face ethical issues (75). In 2006, Takahashi and Yamanaka successfully induced pluripotent stem cells (iPSCs) from fibroblasts by the introduction of four factors, Oct3/4, Sox2, c-Myc and Klf4. The self-renewal and differentiation capacity of pluripotent stem cells is largely comparable to that of embryonic stem cells but avoids ethical issues (76). In recent years, many laboratories have reported the development of cardiomyocytes from ESCs (77, 78) and iPSCs (79–84). ESC-derived cardiomyocytes (ESC-CMs) and iPSC-derived cardiomyocytes (iPSC-CMs), here collectively referred to as hPSC-CMs, express molecular markers and exhibit subcellular structures and electrophysiology resembling primary, albeit immature cardiomyocytes.
Several groups have transplanted hPSC-CM in experimental cardiovascular disease models in vivo (85–93), providing experimental feasibility studies for future clinical applications (Table 2). Studies have confirmed that hPSC-CMs can engraft, survive, and electrically couple with host myocardial tissue in vivo and improve contractile function after infarction. For example, in both acute myocardial infarction and chronic post-infarction heart disease in rats, transplanted hPSC-CMs can survive and form viable tissue containing striated cardiomyocytes. These hPSC-CM injections attenuated ventricular dilatation and preserved systolic function after acute myocardial infarction but are insufficient to alter adverse remodeling of chronic myocardial infarction rats (90, 91). In addition, transplanted hPSC-CMs could remuscularize cryoinjured guinea-pig hearts, thereby preserving cardiac function (92). Intramyocardial delivery of one billion hPSC-CMs into Macaques suffering an ischemia/reperfusion injury also resulted in the remuscularization of substantial areas of the infarcted monkey heart (93). The hPSC-CM engraftment is indeed promising as a cell-based therapy. However, there are key issues that remain to be solved.
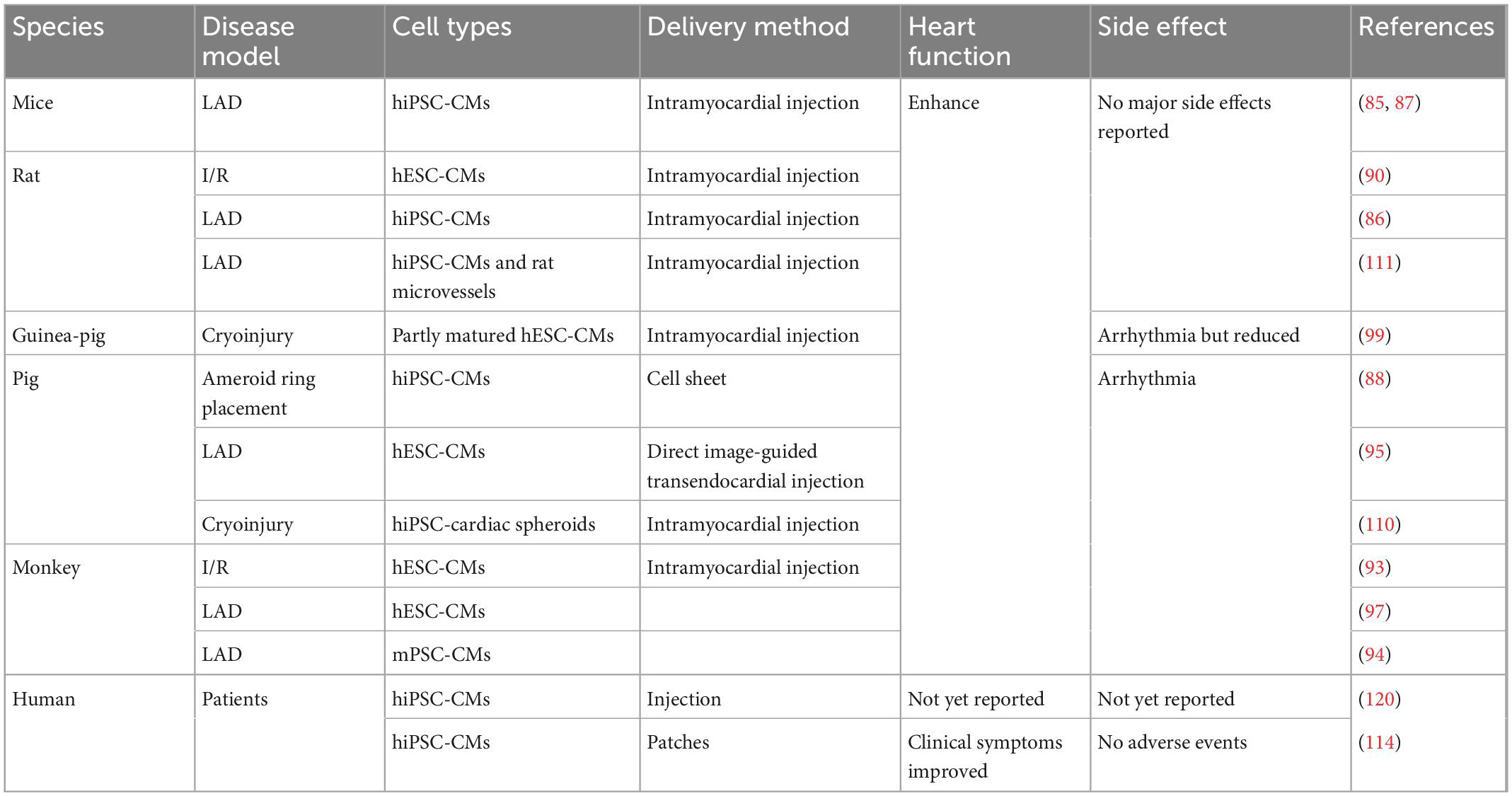
Table 2. Preclinical and clinical studies of hPSC-CMs transplantations for treatment of cardiac disease.
Arrhythmias are considered the most critical side effect of engraftment, as they can be lethal, especially in pigs and primates. In ischemia/reperfusion-injured macaques, ventricular arrhythmias were observed despite remuscularization (93). Similar results were found in myocardial-infarcted cynomolgus monkeys. Ventricular tachycardias happened following the transplantation of monkey iPSC-derived cardiomyocytes (mPSC-CMs) (94). Studies in infarcted hearts of rats and pigs also showed the development of arrhythmias and tachyarrhythmias following injection of immature hPSC-CMs (95, 96). In infarcted hPSC-CM recipient pigs, frequent and fatal ventricular tachyarrhythmias were observed during the first few days of post-transplantation, and normal sinus rhythm was observed 28 days after transplantation (95). Such graft-related ventricular arrhythmias most likely originate from an ectopic pacemaker formed by the transplanted hPSC-CMs (97). To eliminate such arrhythmic events, several strategies have been considered. Pharmacologic treatment is one of the solutions to engraftment arrhythmia. One study showed that a combination of amiodarone and ivabradine could effectively suppress arrhythmia in infarcted hPSC-CM recipient pigs (98). In addition, the engraftment of more mature cardiomyocytes was beneficial in reducing arrhythmia events (99). This study showed that hPSC-CMs cultured on polydimethylsiloxane (PDMS) substrates exhibited increased expression of cardiac maturation markers and improved structural and functional properties of more mature cardiomyocytes in vitro. They then found that transplantation of this PDMS-treated hPSC-CMs in an infarcted guinea pig enhanced post-transplant structure and alignment, host-graft electromechanical integration, and importantly, reduced proarrhythmic behavior (99). To engraft matured hPSC-CMs, several studies have attempted to induce cardiomyocyte maturation in vitro. For example, using 3–6 months long-term cultures, hPSC-CMs exhibited an adult-like phenotype, including increased cell size or greater myofibril density and alignment (100, 101). In addition, electric pacing and mechanical stimulation were shown to promote hPSC-CMs maturation in vitro (102, 103). hPSCs-CMs treated with a maturation medium including a peroxisome proliferator-activated nuclear receptors alpha (PPARa) agonist, palmitate, dexamethasone, and Tri-iodo-l-thyronine (T3) (104) in the presence of low glucose resulted in hPSC-CMs with increased the expression of genes associated with fatty acid oxidation (FAO), mitochondrial respiration, and muscle function (105). In addition, insulin-like growth factor-1 (IGF-1) or low glucose in culture media was shown to promote cardiomyocyte maturation (106, 107). In contrast to monolayer cardiomyocyte cultures, hPSCs-CMs grown in 3D in vitro appear to be more mature and thereby better mimic bona fide cardiomyocytes (108). In particular, self-organizing cardiac organoids, as compared to 2D-grown hPSC-CMs, exhibit increased expression of cardiac ion channels (KCNH2), structural proteins (TNNI1, TTN, and MYH6), cardiac transcriptional factors (TBX5 and MEF2C), or sarcoplasmic reticulum proteins (RYR2 and ATP2A2), indicating improved maturity (108).
During the transplantation of exogenous hPSC-CMs, the nutrient-deprived and hypoxic environment in the infarcted area is a major challenge (109). Although studies demonstrated that hPSC-CMs could be engrafted in monkey hearts and survive up to 3 months (93, 94, 97), another report found that the engrafted hPSC-CMs were massively reduced in numbers after 8 weeks post-transplantation in pig hearts (110). Therefore, the addition of support cells may be beneficial for hPSC-CMs integration and survival. Indeed, co-transplantation of microvessels and hPSC-CMs into the ischemic area of the LAD-treated rats promoted the survival of hPSC-CMs in vivo and improved cardiac function compared with the transplantation of hPSC-CMs alone (111). Although the mechanisms involved in the functional integration and survival of hPSC-CMs in host tissues are not fully understood, studies have found vascularization occurs after hPSC-CMs transplantation and may be related to cytokines such as VEGF secreted by the grafted cells (109, 112). Therefore, the addition of VEGF (113) or other pro-angiogenic factors before transplantation may also contribute to the improvement of hPSC-CMs survival and subsequent enhanced cardiac function.
Besides cardiomyocyte maturation and vascularization, the mode of delivery might be critical. Intracardiac injection is the current delivery method, but grafts may be eluted with the circulatory system. To enhance hPSC-CMs survival, a multicomponent pro-survival cocktail was developed, and its co-injection with hPSC-CMs improved graft residency in vivo (90, 91). Bioengineering methods such as cell patches (114, 115) and cell sheets (116, 117) have also been devised to improve cell engraftment rates, however, integrating cells in biomaterials with host myocardium is a big challenge. For example, transplantation of hPSC-CMs sheets improved cardiac systolic function not attributable to graft integration into the host myocardium but most likely due to neovascularization (118). Recently microneedle patches were developed to be inserted into the myocardium, improving the connection between the graft and the host myocardium (119).
So far, there have been two clinical trials engrafting hPSC-CMs for heart disease. Two patients in China underwent an experimental treatment for heart disease based on hPSC-CMs, though the clinical outcomes have not yet been published (120). In Japan, one male patient who suffered from severe heart failure due to ischemic cardiomyopathy was treated with clinical-grade hPSC-CMs patches. The clinical symptoms apparently improved 6 months after surgery, without any major adverse events or changes in the cardiac wall motion at the site of the transplant. However, more details need to be disclosed (114). Regardless, these first human clinical trials hold promises for the use of hPSC-CMs to repair cardiac damage (Figure 2).
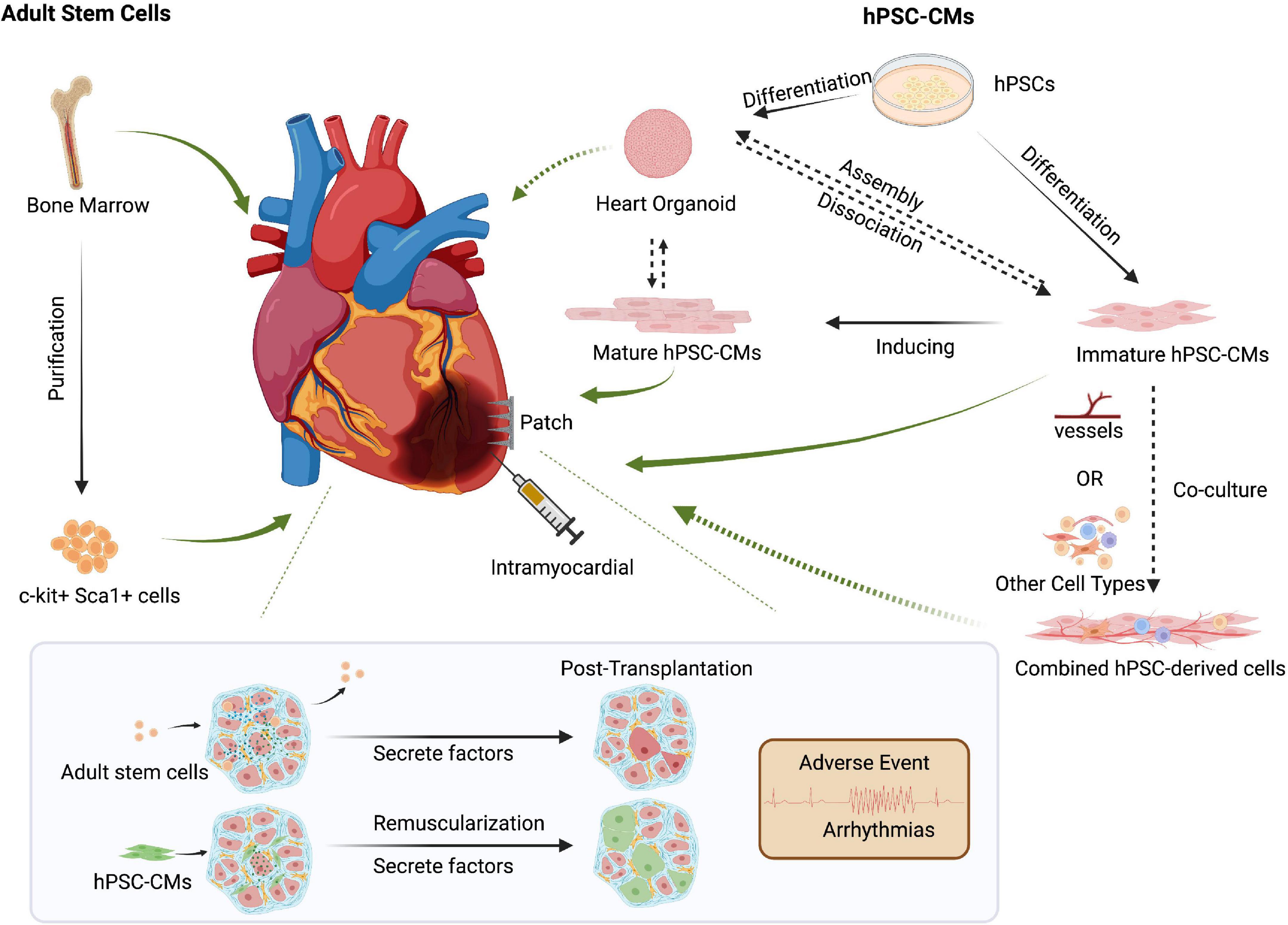
Figure 2. Cell-based approaches to cardiac regenerative medicine. Delivery methods such as intracardiac injection and cell patches can be used for cell-based therapies. Though controversial, transplanting bone marrow-derived adult stem cells could promote cardiac function via secreted factors. Human pluripotent stem cell-derived cardiomyocytes (hPSC-CMs) can repair damaged hearts through tissue replacement of lost cardiomyocytes and help promote cardiac function by secreting growth factors such as VEGF. However, preclinical models and clinical trials must carefully address post-transplant arrhythmias and other side effects. The increased maturity of hPSC-CMs might reduce unwanted and potentially lethal arrhythmic events. Co-delivery of multiple cell types, including endothelial cells or other cardiac cell types, might improve hPSC-CMs retention and thereby promote the repair of injured hearts. Created with BioRender.com.
Future directions and conclusion
The field of cardiac regeneration has made remarkable progress in recent years. Both cell-free and cell-based methods are vigorously researched and developed to promote and improve cardiac regeneration for clinical applications. Along the way, numerous molecular mechanisms and key factors involving cardiomyocyte’s re-entry into the cell cycle or trans-differentiation of non-cardiomyocytes into cardiomyocytes were discovered and are now being translated to drug development. Although some molecules, such as recombinant proteins, small molecule inhibitors, or RNA-based therapies, are being developed, more effective drugs need to be discovered. Moreover, Proteolysis Targeting chimera (PROTAC) technologies might provide viable modes of drug delivery for targeted and time-resolved degradation of candidate drug targets (121, 122).
For cardiac repair using cell-based systems, hPSC-CMs have the potential to form functional tissue containing striated cardiomyocytes in vivo. To achieve clinical use, hPSC-CMs will be required to be mass-produced with strict quality standards. Therefore, allogeneic, off-the-shelf hPSCs-CMs must be developed. In addition to pharmacological immunosuppression, including new-generation drugs with fewer side effects, gene-edited hypoimmune hPSC-CM have been generated to overcome the rejection from the host (123). Another obstacle is the maturity of transplanted hPSC-CMs, in particular, addressing and reducing arrhythmic events triggered by the transplanted cardiomyocytes that have to be functionally integrated into the electrically coupled cardiac tissue. Compared to monolayer cultures, 3D hPSCs-CMs appeared to express more maturation markers and functionally mimic more mature cardiomyocytes, including the formation of tight junctions between cardiomyocytes. Thus, transplantation of hPSC-CM aggregates rather than loose single cardiomyocytes may contribute to graft survival, improve functionality and reduce arrhythmias. However, several studies suggest that the optimal timing of transplantation depends on the developmental stage of hPSC-CMs (124, 125). Moreover, the mode of delivery of such cell-based therapies will be critical. Balancing hPSC-CMs maturity, effective delivery, and transplantation timing must be the focus of future research.
Besides cardiomyocytes, the heart contains multiple other cell types, such as endothelial cells, fibroblasts, smooth muscle cells, or different types of immune cells, that might affect graft survival and improve the function of damaged hearts (126). As more hPSC-derived cell types can be faithfully generated, co-transplantation of multiple cell types might therefore greatly improve cell-based therapies for cardiac diseases. For instance, our group developed stem cell-derived self-organizing 3D blood vessel organoids (BVOs) that form bona fide and functionally perfused vascular trees containing arterioles, capillaries, and venules when transplanted into immunodeficient mice (127). Such BVOs and other approaches to generate human endothelial cells and blood vessels, such as 3D printing, could be utilized to enhance and maintain the engraftment of stem cell-derived cardiomyocytes.
Author contributions
JW wrote and revised the draft. JP designed and supervised and revised the study. MA designed the tables. BH revised the draft. All authors read and approved the submitted version.
Funding
JP was supported by a Canada 150 Chair in Functional Genomics, a Paul G. Allen Distinguished Investigator Award, and the T. von Zastrow Foundation.
Conflict of interest
JP was the founder of Angios Biotech which develops blood vessel organoids for drug screening and clinical use in humans.
The remaining authors declare that the research was conducted in the absence of any commercial or financial relationships that could be construed as a potential conflict of interest.
Publisher’s note
All claims expressed in this article are solely those of the authors and do not necessarily represent those of their affiliated organizations, or those of the publisher, the editors and the reviewers. Any product that may be evaluated in this article, or claim that may be made by its manufacturer, is not guaranteed or endorsed by the publisher.
References
1. Heidenreich P, Bozkurt B, Aguilar D, Allen L, Byun J, Colvin M, et al. 2022 Aha/Acc/Hfsa guideline for the management of heart failure: a report of the American college of cardiology/American heart association joint committee on clinical practice guidelines. Circulation. (2022) 2022:1063. doi: 10.1161/CIR.0000000000001063
2. Piperata A, Caraffa R, Bifulco O, Avesani M, Apostolo A, Gerosa G, et al. Marginal donors and organ shortness: concomitant surgical procedures during heart transplantation: a literature review. J Cardiovasc Med. (2022) 23:167–75. doi: 10.2459/jcm.0000000000001233
3. Cui Y, Zheng Y, Liu X, Yan L, Fan X, Yong J, et al. Single-cell transcriptome analysis maps the developmental track of the human heart. Cell Rep. (2019) 26:1934–50e5. doi: 10.1016/j.celrep.2019.01.079
4. Lescroart F, Zaffran S. Single cell approaches to understand the earliest steps in heart development. Curr Cardiol Rep. (2022) 2022:1682. doi: 10.1007/s11886-022-01681-w
5. Wang Q, Song Y, Chen J, Li Q, Gao J, Tan H, et al. Direct in vivo reprogramming with non-viral sequential targeting nanoparticles promotes cardiac regeneration. Biomaterials. (2021) 276:121028. doi: 10.1016/j.biomaterials.2021.121028
6. Fan C, Joshi J, Li F, Xu B, Khan M, Yang J, et al. Nanoparticle-mediated drug delivery for treatment of ischemic heart disease. Front Bioengin Biotechnol. (2020) 8:687. doi: 10.3389/fbioe.2020.00687
7. Naso M, Tomkowicz B, Perry W, Strohl W. Adeno-associated virus (Aav) as a vector for gene therapy. BioDrugs. (2017) 31:317–34. doi: 10.1007/s40259-017-0234-5
8. Matsa E, Burridge P, Wu J. Human stem cells for modeling heart disease and for drug discovery. Sci Trans Med. (2014) 6:s6–6. doi: 10.1126/scitranslmed.3008921
9. Smith A, Macadangdang J, Leung W, Laflamme M, Kim D. Human ipsc-derived cardiomyocytes and tissue engineering strategies for disease modeling and drug screening. Biotechnol Adv. (2017) 35:77–94. doi: 10.1016/j.biotechadv.2016.12.002
10. Jopling C, Sleep E, Raya M, Martí M, Raya A, Belmonte J. Zebrafish heart regeneration occurs by cardiomyocyte dedifferentiation and proliferation. Nature. (2010) 464:606–9. doi: 10.1038/nature08899
11. Laube F, Heister M, Scholz C, Borchardt T, Braun T. Re-programming of newt cardiomyocytes is induced by tissue regeneration. J Cell Sci. (2006) 119:4719–29. doi: 10.1242/jcs.03252
12. Porrello E, Mahmoud A, Simpson E, Hill J, Richardson J, Olson E, et al. Transient regenerative potential of the neonatal mouse heart. Science. (2011) 331:1078–80. doi: 10.1126/science.1200708
13. Mahmoud A, Porrello E, Kimura W, Olson E, Sadek H. Surgical models for cardiac regeneration in neonatal mice. Nat Prot. (2014) 9:305–11. doi: 10.1038/nprot.2014.021
14. Haubner B, Adamowicz-Brice M, Khadayate S, Tiefenthaler V, Metzler B, Aitman T, et al. Complete cardiac regeneration in a mouse model of myocardial infarction. Aging. (2012) 4:966–77. doi: 10.18632/aging.100526
15. Haubner B, Schuetz T, Penninger JMA. Reproducible protocol for neonatal ischemic injury and cardiac regeneration in neonatal mice. Basic Res Cardiol. (2016) 111:64. doi: 10.1007/s00395-016-0580-3
16. Wang Z, Cui M, Shah A, Tan W, Liu N, Bassel-Duby R, et al. Cell-type-specific gene regulatory networks underlying murine neonatal heart regeneration at single-cell resolution. Cell Rep. (2020) 33:108472. doi: 10.1016/j.celrep.2020.108472
17. Zhu W, Zhang E, Zhao M, Chong Z, Fan C, Tang Y, et al. Regenerative potential of neonatal porcine hearts. Circulation. (2018) 138:2809–16. doi: 10.1161/CIRCULATIONAHA.118.034886
18. Haubner B, Schneider J, Schweigmann U, Schuetz T, Dichtl W, Velik-Salchner C, et al. Functional recovery of a human neonatal heart after severe myocardial infarction. Circ Res. (2016) 118:216–21. doi: 10.1161/CIRCRESAHA.115.307017
19. Derks W, Bergmann O. Polyploidy in cardiomyocytes. Circ Res. (2020) 126:552–65. doi: 10.1161/CIRCRESAHA.119.315408
20. Yu W, Huang X, Tian X, Zhang H, He L, Wang Y, et al. Gata4 regulates fgf16 to promote heart repair after injury. Development. (2016) 143:936–49. doi: 10.1242/dev.130971
21. D’Uva G, Aharonov A, Lauriola M, Kain D, Yahalom-Ronen Y, Carvalho S, et al. Erbb2 triggers mammalian heart regeneration by promoting cardiomyocyte dedifferentiation and proliferation. Nat Cell Biol. (2015) 17:627–38. doi: 10.1038/ncb3149
22. Chakraborty S, Sengupta A, Yutzey K. Tbx20 promotes cardiomyocyte proliferation and persistence of fetal characteristics in adult mouse hearts. J Mol Cell Cardiol. (2013) 62:203–13. doi: 10.1016/j.yjmcc.2013.05.018
23. Wu C, Kruse F, Vasudevarao M, Junker J, Zebrowski D, Fischer K, et al. Spatially resolved genome-wide transcriptional profiling identifies bmp signaling as essential regulator of zebrafish cardiomyocyte regeneration. Dev Cell. (2016) 36:36–49. doi: 10.1016/j.devcel.2015.12.010
24. Payumo A, Chen X, Hirose K, Chen X, Hoang A, Khyeam S, et al. Adrenergic-thyroid hormone interactions drive postnatal thermogenesis and loss of mammalian heart regenerative capacity. Circulation. (2021) 144:1000–3. doi: 10.1161/CIRCULATIONAHA.121.054846
25. Bersell K, Arab S, Haring B, Kühn B. Neuregulin1/Erbb4 signaling induces cardiomyocyte proliferation and repair of heart injury. Cell. (2009) 138:257–70. doi: 10.1016/j.cell.2009.04.060
26. Leach J, Heallen T, Zhang M, Rahmani M, Morikawa Y, Hill M, et al. Hippo pathway deficiency reverses systolic heart failure after infarction. Nature. (2017) 550:260–4. doi: 10.1038/nature24045
27. Mahmoud A, Kocabas F, Muralidhar S, Kimura W, Koura A, Thet S, et al. Meis1 regulates postnatal cardiomyocyte cell cycle arrest. Nature. (2013) 497:249–53. doi: 10.1038/nature12054
28. Ieda M, Fu J, Delgado-Olguin P, Vedantham V, Hayashi Y, Bruneau B, et al. Direct reprogramming of fibroblasts into functional cardiomyocytes by defined factors. Cell. (2010) 142:375–86. doi: 10.1016/j.cell.2010.07.002
29. Mohamed T, Stone N, Berry E, Radzinsky E, Huang Y, Pratt K, et al. Chemical enhancement of in vitro and in vivo direct cardiac reprogramming. Circulation. (2017) 135:978–95. doi: 10.1161/CIRCULATIONAHA.116.024692
30. Singh V, Pinnamaneni J, Pugazenthi A, Sanagasetti D, Mathison M, Martin J, et al. Hippo pathway effector tead1 induces cardiac fibroblast to cardiomyocyte reprogramming. J Am Heart Assoc. (2021) 10:e022659. doi: 10.1161/JAHA.121.022659
31. Cao N, Huang Y, Zheng J, Spencer C, Zhang Y, Fu J, et al. Conversion of human fibroblasts into functional cardiomyocytes by small molecules. Science. (2016) 352:1216–20. doi: 10.1126/science.aaf1502
32. Efe J, Hilcove S, Kim J, Zhou H, Ouyang K, Wang G, et al. Conversion of mouse fibroblasts into cardiomyocytes using a direct reprogramming strategy. Nat Cell Biol. (2011) 13:215–22. doi: 10.1038/ncb2164
33. Zhang H, Lui K, Zhou B. Endocardial cell plasticity in cardiac development, diseases and regeneration. Circ Res. (2018) 122:774–89. doi: 10.1161/CIRCRESAHA.117.312136
34. Fioret Bryan A, Heimfeld Jeremy D, Paik David T, Hatzopoulos Antonis K. Endothelial cells contribute to generation of adult ventricular myocytes during cardiac homeostasis. Cell Rep. (2014) 8:229–41. doi: 10.1016/j.celrep.2014.06.004
35. Woo Y, Panlilio C, Cheng R, Liao G, Atluri P, Hsu V, et al. Therapeutic delivery of cyclin A2 induces myocardial regeneration and enhances cardiac function in ischemic heart failure. Circulation. (2006) 114:455. doi: 10.1161/CIRCULATIONAHA.105.000455
36. Mohamed T, Ang Y, Radzinsky E, Zhou P, Huang Y, Elfenbein A, et al. Regulation of cell cycle to stimulate adult cardiomyocyte proliferation and cardiac regeneration. Cell. (2018) 173:104–16e12. doi: 10.1016/j.cell.2018.02.014
37. Wu Y, Zhou L, Liu H, Duan R, Zhou H, Zhang F, et al. Lrp6 downregulation promotes cardiomyocyte proliferation and heart regeneration. Cell Res. (2020) 2020:411. doi: 10.1038/s41422-020-00411-7
38. Wang Z, Cui M, Shah A, Ye W, Tan W, Min Y, et al. Mechanistic basis of neonatal heart regeneration revealed by transcriptome and histone modification profiling. Proc Natl Acad Sci USA. (2019) 116:18455–65. doi: 10.1073/pnas.1905824116
39. Aguirre A, Montserrat N, Zacchigna S, Nivet E, Hishida T, Krause Marie N, et al. In vivo activation of a conserved microrna program induces mammalian heart regeneration. Cell Stem Cell. (2014) 15:589–604. doi: 10.1016/j.stem.2014.10.003
40. Cai B, Ma W, Wang X, Sukhareva N, Hua B, Zhang L, et al. Targeting lncdach1 promotes cardiac repair and regeneration after myocardium infarction. Cell Death Different. (2020) 27:2158–75. doi: 10.1038/s41418-020-0492-5
41. Magadum A, Singh N, Kurian A, Munir I, Mehmood T, Brown K, et al. Pkm2 regulates cardiomyocyte cell cycle and promotes cardiac regeneration. Circulation. (2020) 141:1249–65. doi: 10.1161/CIRCULATIONAHA.119.043067
42. Nakada Y, Canseco D, Thet S, Abdisalaam S, Asaithamby A, Santos C, et al. Hypoxia induces heart regeneration in adult mice. Nature. (2017) 541:222–7. doi: 10.1038/nature20173
43. Qian L, Huang Y, Spencer C, Foley A, Vedantham V, Liu L, et al. In vivo reprogramming of murine cardiac fibroblasts into induced cardiomyocytes. Nature. (2012) 485:593–8. doi: 10.1038/nature11044
44. Koudstaal S, Bastings M, Feyen D, Waring C, van Slochteren F, Dankers P, et al. Sustained delivery of insulin-like growth factor-1/hepatocyte growth factor stimulates endogenous cardiac repair in the chronic infarcted pig heart. J Cardiovasc Trans Res. (2014) 7:232–41. doi: 10.1007/s12265-013-9518-4
45. Bagno L, Kanashiro-Takeuchi R, Suncion V, Golpanian S, Karantalis V, Wolf A, et al. Growth hormone–releasing hormone agonists reduce myocardial infarct scar in swine with subacute ischemic cardiomyopathy. J Am Heart Assoc. (2015) 4:e001464. doi: 10.1161/JAHA.114.001464
46. Wei K, Serpooshan V, Hurtado C, Diez-Cuñado M, Zhao M, Maruyama S, et al. Epicardial Fstl1 reconstitution regenerates the adult mammalian heart. Nature. (2015) 525:479–85. doi: 10.1038/nature15372
47. Cao X, Jahng J, Lee C, Zha Y, Wheeler M, Sallam K, et al. Generation of three induced pluripotent stem cell lines from hypertrophic cardiomyopathy patients carrying myh7 mutations. Stem Cell Res. (2021) 55:102455. doi: 10.1016/j.scr.2021.102455
48. Thomas D, Cunningham N, Shenoy S, Wu J. Human Ipscs in cardiovascular research: current approaches in cardiac differentiation, maturation strategies, and scalable production. Cardiovasc Res. (2021) 118:20–36. doi: 10.1093/cvr/cvab115
49. Jay S, Murthy A, Hawkins J, Wortzel J, Steinhauser M, Alvarez L, et al. An engineered bivalent neuregulin protects against doxorubicin-induced cardiotoxicity with reduced proneoplastic potential. Circulation. (2013) 128:152–61. doi: 10.1161/CIRCULATIONAHA.113.002203
50. Lan F, Lee Andrew S, Liang P, Sanchez-Freire V, Nguyen Patricia K, Wang L, et al. Abnormal calcium handling properties underlie familial hypertrophic cardiomyopathy pathology in patient-specific induced pluripotent stem cells. Cell Stem Cell. (2013) 12:101–13. doi: 10.1016/j.stem.2012.10.010
51. Kim H, Kamm R, Vunjak-Novakovic G, Wu J. Progress in multicellular human cardiac organoids for clinical applications. Cell Stem Cell. (2022) 29:503–14. doi: 10.1016/j.stem.2022.03.012
52. Kupfer M, Lin W, Ravikumar V, Qiu K, Wang L, Gao L, et al. In situ expansion, differentiation, and electromechanical coupling of human cardiac muscle in a 3d bioprinted, chambered organoid. Circ Res. (2020) 127:207–24. doi: 10.1161/CIRCRESAHA.119.316155
53. Orlic D, Kajstura J, Chimenti S, Jakoniuk I, Anderson S, Li B, et al. Bone marrow cells regenerate infarcted myocardium. Nature. (2001) 410:701–5. doi: 10.1038/35070587
54. Jackson K, Majka S, Wang H, Pocius J, Hartley C, Majesky M, et al. Regeneration of ischemic cardiac muscle and vascular endothelium by adult stem cells. J Clin Investigat. (2001) 107:1395–402. doi: 10.1172/JCI12150
55. Beltrami A, Barlucchi L, Torella D, Baker M, Limana F, Chimenti S, et al. Adult cardiac stem cells are multipotent and support myocardial regeneration. Cell. (2003) 114:763–76. doi: 10.1016/S0092-867400687-1
56. Oh H, Bradfute S, Gallardo T, Nakamura T, Gaussin V, Mishina Y, et al. Cardiac progenitor cells from adult myocardium: homing, differentiation, and fusion after infarction. Proc Natl Acad Sci USA. (2003) 100:12313–8. doi: 10.1073/pnas.2132126100
57. Matsuura K, Nagai T, Nishigaki N, Oyama T, Nishi J, Wada H, et al. Adult cardiac sca-1-positive cells differentiate into beating cardiomyocytes *. J Biol Chem. (2004) 279:11384–91. doi: 10.1074/jbc.M310822200
58. Murry C, Soonpaa M, Reinecke H, Nakajima H, Nakajima H, Rubart M, et al. Haematopoietic stem cells do not transdifferentiate into cardiac myocytes in myocardial infarcts. Nature. (2004) 428:664–8. doi: 10.1038/nature02446
59. Balsam L, Wagers A, Christensen J, Kofidis T, Weissman I, Robbins R. Haematopoietic stem cells adopt mature haematopoietic fates in ischaemic myocardium. Nature. (2004) 428:668–73. doi: 10.1038/nature02460
60. van Berlo J, Kanisicak O, Maillet M, Vagnozzi R, Karch J, Lin S, et al. C-kit+ cells minimally contribute cardiomyocytes to the heart. Nature. (2014) 509:337–41. doi: 10.1038/nature13309
61. Sultana N, Zhang L, Yan J, Chen J, Cai W, Razzaque S, et al. Resident C-kit+ cells in the heart are not cardiac stem cells. Nat Commun. (2015) 6:8701. doi: 10.1038/ncomms9701
62. Vagnozzi R, Sargent M, Lin S, Palpant N, Murry C, Molkentin J. Genetic lineage tracing of sca-1+ cells reveals endothelial but not myogenic contribution to the murine heart. Circulation. (2018) 138:2931–9. doi: 10.1161/CIRCULATIONAHA.118.035210
63. Zhang L, Sultana N, Yan J, Yang F, Chen F, Chepurko E, et al. Cardiac sca-1+ cells are not intrinsic stem cells for myocardial development, renewal, and repair. Circulation. (2018) 138:2919–30. doi: 10.1161/CIRCULATIONAHA.118.035200
64. Neidig L, Weinberger F, Palpant N, Mignone J, Martinson A, Sorensen D, et al. Evidence for minimal cardiogenic potential of stem cell antigen 1–positive cells in the adult mouse heart. Circulation. (2018) 138:2960–2. doi: 10.1161/CIRCULATIONAHA.118.035273
65. Tang J, Li Y, Huang X, He L, Zhang L, Wang H, et al. Fate mapping of sca1+ cardiac progenitor cells in the adult mouse heart. Circulation. (2018) 138:2967–9. doi: 10.1161/CIRCULATIONAHA.118.036210
66. Soonpaa M, Lafontant P, Reuter S, Scherschel J, Srour E, Zaruba M, et al. Absence of cardiomyocyte differentiation following transplantation of adult cardiac-resident sca-1+ cells into infarcted mouse hearts. Circulation. (2018) 138:2963–6. doi: 10.1161/CIRCULATIONAHA.118.035391
67. He L, Han M, Zhang Z, Li Y, Huang X, Liu X, et al. Reassessment of C-kit+ cells for cardiomyocyte contribution in adult heart. Circulation. (2019) 140:164–6. doi: 10.1161/CIRCULATIONAHA.119.039909
68. Hansson E, Lendahl U. Regenerative medicine for the treatment of heart disease. J Int Med. (2013) 273:235–45. doi: 10.1111/joim.12033
69. Gerbin K, Murry C. The winding road to regenerating the human heart. Cardiovasc Pathol. (2015) 24:133–40. doi: 10.1016/j.carpath.2015.02.004
70. Michler R. The current status of stem cell therapy in ischemic heart disease. J Cardiac Surg. (2018) 33:520–31. doi: 10.1111/jocs.13789
71. Mirotsou M, Jayawardena T, Schmeckpeper J, Gnecchi M, Dzau V. Paracrine mechanisms of stem cell reparative and regenerative actions in the heart. J Mol Cell Cardiol. (2011) 50:280–9. doi: 10.1016/j.yjmcc.2010.08.005
72. Sid-Otmane C, Perrault L, Ly H. Mesenchymal stem cell mediates cardiac repair through autocrine, paracrine and endocrine axes. J Trans Med. (2020) 18:336. doi: 10.1186/s12967-020-02504-8
73. Ju C, Shen Y, Ma G, Liu Y, Cai J, Kim I, et al. Transplantation of cardiac mesenchymal stem cell-derived exosomes promotes repair in ischemic myocardium. J Cardiovasc Trans Res. (2018) 11:420–8. doi: 10.1007/s12265-018-9822-0
74. Vagnozzi R, Maillet M, Sargent M, Khalil H, Johansen A, Schwanekamp J, et al. An acute immune response underlies the benefit of cardiac stem cell therapy. Nature. (2020) 577:405. doi: 10.1038/s41586-019-1802-2
75. Volarevic V, Markovic B, Gazdic M, Volarevic A, Jovicic N, Arsenijevic N, et al. Ethical and safety issues of stem cell-based therapy. Int J Med Sci. (2018) 15:36–45. doi: 10.7150/ijms.21666
76. Takahashi K, Yamanaka S. Induction of pluripotent stem cells from mouse embryonic and adult fibroblast cultures by defined factors. Cell. (2006) 126:663–76. doi: 10.1016/j.cell.2006.07.024
77. Kehat I, Kenyagin-Karsenti D, Snir M, Segev H, Amit M, Gepstein A, et al. Human embryonic stem cells can differentiate into myocytes with structural and functional properties of cardiomyocytes. J Clin Invest. (2001) 108:407–14. doi: 10.1172/JCI12131
78. Murry C, Keller G. Differentiation of embryonic stem cells to clinically relevant populations: lessons from embryonic development. Cell. (2008) 132:661–80. doi: 10.1016/j.cell.2008.02.008
79. Mendjan S, Mascetti Victoria L, Ortmann D, Ortiz M, Karjosukarso Dyah W, Ng Y, et al. Nanog and Cdx2 pattern distinct subtypes of human mesoderm during exit from pluripotency. Cell Stem Cell. (2014) 15:310–25. doi: 10.1016/j.stem.2014.06.006
80. Mauritz C, Schwanke K, Reppel M, Neef S, Katsirntaki K, Maier L, et al. Generation of functional murine cardiac myocytes from induced pluripotent stem cells. Circulation. (2008) 118:507–17. doi: 10.1161/CIRCULATIONAHA.108.778795
81. Karakikes I, Ameen M, Termglinchan V, Wu J. Human induced pluripotent stem cell–derived cardiomyocytes. Circ Res. (2015) 117:80–8. doi: 10.1161/CIRCRESAHA.117.305365
82. Sharma A, Marceau C, Hamaguchi R, Burridge P, Rajarajan K, Churko J, et al. Human induced pluripotent stem cell–derived cardiomyocytes as an in vitro model for coxsackievirus b3–induced myocarditis and antiviral drug screening platform. Circ Res. (2014) 115:556–66. doi: 10.1161/CIRCRESAHA.115.303810
83. Lian X, Zhang J, Azarin S, Zhu K, Hazeltine L, Bao X, et al. Directed cardiomyocyte differentiation from human pluripotent stem cells by modulating wnt/β-catenin signaling under fully defined conditions. Nat Prot. (2013) 8:162–75. doi: 10.1038/nprot.2012.150
84. Burridge P, Matsa E, Shukla P, Lin Z, Churko J, Ebert A, et al. Chemically defined generation of human cardiomyocytes. Nat Methods. (2014) 11:855–60. doi: 10.1038/nmeth.2999
85. Rojas S, Kensah G, Rotaermel A, Baraki H, Kutschka I, Zweigerdt R, et al. Transplantation of purified ipsc-derived cardiomyocytes in myocardial infarction. PLoS One. (2017) 12:e0173222. doi: 10.1371/journal.pone.0173222
86. Guan X, Xu W, Zhang H, Wang Q, Yu J, Zhang R, et al. Transplantation of human induced pluripotent stem cell-derived cardiomyocytes improves myocardial function and reverses ventricular remodeling in infarcted rat hearts. Stem Cell Res Ther. (2020) 11:73. doi: 10.1186/s13287-020-01602-0
87. Jiang X, Yang Z, Dong M. Cardiac repair in a murine model of myocardial infarction with human induced pluripotent stem cell-derived cardiomyocytes. Stem Cell Res Ther. (2020) 11:297. doi: 10.1186/s13287-020-01811-7
88. Ishida M, Miyagawa S, Saito A, Fukushima S, Harada A, Ito E, et al. Transplantation of human-induced pluripotent stem cell-derived cardiomyocytes is superior to somatic stem cell therapy for restoring cardiac function and oxygen consumption in a porcine model of myocardial infarction. Transplantation. (2019) 103:291–8. doi: 10.1097/tp.0000000000002384
89. Robey T, Saiget M, Reinecke H, Murry C. Systems approaches to preventing transplanted cell death in cardiac repair. J Mol Cell Cardiol. (2008) 45:567–81. doi: 10.1016/j.yjmcc.2008.03.009
90. Laflamme M, Chen K, Naumova A, Muskheli V, Fugate J, Dupras S, et al. Cardiomyocytes derived from human embryonic stem cells in pro-survival factors enhance function of infarcted rat hearts. Nat Biotechnol. (2007) 25:1015–24. doi: 10.1038/nbt1327
91. Fernandes S, Naumova A, Zhu W, Laflamme M, Gold J, Murry C. Human embryonic stem cell-derived cardiomyocytes engraft but do not alter cardiac remodeling after chronic infarction in rats. J Mol Cell Cardiol. (2010) 49:941–9. doi: 10.1016/j.yjmcc.2010.09.008
92. Shiba Y, Fernandes S, Zhu W, Filice D, Muskheli V, Kim J, et al. Human Es-cell-derived cardiomyocytes electrically couple and suppress arrhythmias in injured hearts. Nature. (2012) 489:322–5. doi: 10.1038/nature11317
93. Chong J, Yang X, Don C, Minami E, Liu Y, Weyers J, et al. Human embryonic-stem-cell-derived cardiomyocytes regenerate non-human primate hearts. Nature. (2014) 510:273–7. doi: 10.1038/nature13233
94. Shiba Y, Gomibuchi T, Seto T, Wada Y, Ichimura H, Tanaka Y, et al. Allogeneic transplantation of ips cell-derived cardiomyocytes regenerates primate hearts. Nature. (2016) 538:388–91.
95. Romagnuolo R, Masoudpour H, Porta-Sánchez A, Qiang B, Barry J, Laskary A, et al. Human embryonic stem cell-derived cardiomyocytes regenerate the infarcted pig heart but induce ventricular tachyarrhythmias. Stem Cell Rep. (2019) 12:967–81. doi: 10.1016/j.stemcr.2019.04.005
96. Kadota S, Pabon L, Reinecke H, Murry C. In vivo maturation of human induced pluripotent stem cell-derived cardiomyocytes in neonatal and adult rat hearts. Stem Cell Rep. (2017) 8:278–89. doi: 10.1016/j.stemcr.2016.10.009
97. Liu Y, Chen B, Yang X, Fugate J, Kalucki F, Futakuchi-Tsuchida A, et al. Human embryonic stem cell–derived cardiomyocytes restore function in infarcted hearts of non-human primates. Nat Biotechnol. (2018) 36:597–605. doi: 10.1038/nbt.4162
98. Nakamura K, Neidig L, Yang X, Weber G, El-Nachef D, Tsuchida H, et al. Pharmacologic therapy for engraftment arrhythmia induced by transplantation of human cardiomyocytes. Stem Cell Rep. (2021) 16:2473–87. doi: 10.1016/j.stemcr.2021.08.005
99. Dhahri W, Valdman T, Wilkinson D, Pereira E, Ceylan E, Andharia N, et al. In vitro matured human pluripotent stem cell-derived cardiomyocytes form grafts with enhanced structure and function in injured hearts. Circulation. (2022) 145:1412–26. doi: 10.1161/CIRCULATIONAHA.121.053563
100. Lundy S, Zhu W, Regnier M, Laflamme M. Structural and functional maturation of cardiomyocytes derived from human pluripotent stem cells. Stem Cells Dev. (2013) 22:1991–2002. doi: 10.1089/scd.2012.0490
101. Ebert A, Joshi A, Andorf S, Dai Y, Sampathkumar S, Chen H, et al. Proteasome-dependent regulation of distinct metabolic states during long-term culture of human ipsc-derived cardiomyocytes. Circ Res. (2019) 125:90–103. doi: 10.1161/CIRCRESAHA.118.313973
102. Ruan J, Tulloch N, Razumova M, Saiget M, Muskheli V, Pabon L, et al. Mechanical stress conditioning and electrical stimulation promote contractility and force maturation of induced pluripotent stem cell-derived human cardiac tissue. Circulation. (2016) 134:1557–67. doi: 10.1161/CIRCULATIONAHA.114.014998
103. Ronaldson-Bouchard K, Ma S, Yeager K, Chen T, Song L, Sirabella D, et al. Advanced maturation of human cardiac tissue grown from pluripotent stem cells. Nature. (2018) 556:239–43. doi: 10.1038/s41586-018-0016-3
104. Yang X, Rodriguez M, Pabon L, Fischer K, Reinecke H, Regnier M, et al. Tri-iodo-L-thyronine promotes the maturation of human cardiomyocytes-derived from induced pluripotent stem cells. J Mol Cell Cardiol. (2014) 72:296–304. doi: 10.1016/j.yjmcc.2014.04.005
105. Funakoshi S, Fernandes I, Mastikhina O, Wilkinson D, Tran T, Dhahri W, et al. Generation of mature compact ventricular cardiomyocytes from human pluripotent stem cells. Nat Commun. (2021) 12:3155. doi: 10.1038/s41467-021-23329-z
106. Huang C, Peres Moreno Maia-Joca R, Ong C, Wilson I, DiSilvestre D, Tomaselli G, et al. Enhancement of human ipsc-derived cardiomyocyte maturation by chemical conditioning in a 3d environment. J Mol Cell Cardiol. (2020) 138:1–11. doi: 10.1016/j.yjmcc.2019.10.001
107. Feyen D, McKeithan W, Bruyneel A, Spiering S, Hörmann L, Ulmer B, et al. Metabolic maturation media improve physiological function of human ipsc-derived cardiomyocytes. Cell Rep. (2020) 32:107925. doi: 10.1016/j.celrep.2020.107925
108. Hofbauer P, Jahnel S, Papai N, Giesshammer M, Deyett A, Schmidt C, et al. Cardioids reveal self-organizing principles of human cardiogenesis. Cell. (2021) 2021:34. doi: 10.1016/j.cell.2021.04.034
109. Zhao X, Chen H, Xiao D, Yang H, Itzhaki I, Qin X, et al. Comparison of non-human primate versus human induced pluripotent stem cell-derived cardiomyocytes for treatment of myocardial infarction. Stem Cell Rep. (2018) 10:422–35. doi: 10.1016/j.stemcr.2018.01.002
110. Kawaguchi S, Soma Y, Nakajima K, Kanazawa H, Tohyama S, Tabei R, et al. Intramyocardial transplantation of human ips cell–derived cardiac spheroids improves cardiac function in heart failure animals. JACC Basic Trans Sci. (2021) 6:239–54. doi: 10.1016/j.jacbts.2020.11.017
111. Sun X, Wu J, Qiang B, Romagnuolo R, Gagliardi M, Keller G, et al. Transplanted microvessels improve pluripotent stem cell–derived cardiomyocyte engraftment and cardiac function after infarction in rats. Sci Trans Med. (2020) 12:eaax2992. doi: 10.1126/scitranslmed.aax2992
112. Nagase K, Nagumo Y, Kim M, Kim H, Kyung H, Chung H, et al. Local release of vegf using fiber mats enables effective transplantation of layered cardiomyocyte sheets. Macromol Biosci. (2017) 17:1700073. doi: 10.1002/mabi.201700073
113. Ai X, Yan B, Witman N, Gong Y, Yang L, Tan Y, et al. Transient secretion of Vegf protein from transplanted hipsc-cms enhances engraftment and improves rat heart function post mi. Mol Ther. (2022) 2022:12. doi: 10.1016/j.ymthe.2022.08.012
114. Miyagawa S, Kainuma S, Kawamura T, Suzuki K, Ito Y, Iseoka H, et al. Transplantation of ipsc-derived cardiomyocyte patches for ischemic cardiomyopathy. MedRxiv [preprint]. (2022). doi: 10.1101/2021.12.27.21268295
115. Miyagawa S, Kawamura T, Ito E, Takeda M, Iseoka H, Yokoyama J, et al. Evaluation of the Efficacy and safety of a clinical grade human induced pluripotent stem cell-derived cardiomyocyte patch: a pre-clinical study. bioRxiv [preprint]. (2021). doi: 10.1101/2021.04.07.438744
116. Kawamura M, Miyagawa S, Fukushima S, Saito A, Miki K, Funakoshi S, et al. Enhanced therapeutic effects of human ips cell derived-cardiomyocyte by combined cell-sheets with omental flap technique in porcine ischemic cardiomyopathy model. Sci Rep. (2017) 7:8824. doi: 10.1038/s41598-017-08869-z
117. Kawamura M, Miyagawa S, Miki K, Saito A, Fukushima S, Higuchi T, et al. Feasibility, safety, and therapeutic efficacy of human induced pluripotent stem cell-derived cardiomyocyte sheets in a porcine ischemic cardiomyopathy model. Circulation. (2012) 126:S29–37. doi: 10.1161/CIRCULATIONAHA.111.084343
118. Masumoto H, Matsuo T, Yamamizu K, Uosaki H, Narazaki G, Katayama S, et al. Pluripotent stem cell-engineered cell sheets reassembled with defined cardiovascular populations ameliorate reduction in infarct heart function through cardiomyocyte-mediated neovascularization. Stem Cells. (2012) 30:1196–205. doi: 10.1002/stem.1089
119. Tang J, Wang J, Huang K, Ye Y, Su T, Qiao L, et al. Cardiac cell–integrated microneedle patch for treating myocardial infarction. Sci Adv. (2018) 4:eaat9365. doi: 10.1126/sciadv.aat9365
120. Mallapaty S. Revealed: two men in china were first to receive pioneering stem-cell treatment for heart disease. Nature. (2020) 581:249–51. doi: 10.1038/d41586-020-01285-w
121. Donovan K, Ferguson F, Bushman J, Eleuteri N, Bhunia D, Ryu S, et al. Mapping the degradable kinome provides a resource for expedited degrader development. Cell. (2020) 10:38. doi: 10.1016/j.cell.2020.10.038
122. Sun X, Wang J, Yao X, Zheng W, Mao Y, Lan T, et al. A chemical approach for global protein knockdown from mice to non-human primates. Cell Dis. (2019) 5:10. doi: 10.1038/s41421-018-0079-1
123. Deuse T, Tediashvili G, Hu X, Gravina A, Tamenang A, Wang D, et al. Hypoimmune induced pluripotent stem cell–derived cell therapeutics treat cardiovascular and pulmonary diseases in immunocompetent allogeneic mice. Proc Natl Acad Sci USA. (2021) 118:e2022091118. doi: 10.1073/pnas.2022091118
124. Peinkofer G, Maass M, Pfannkuche K, Sachinidis A, Baldus S, Hescheler J, et al. Persistence of intramyocardially transplanted murine induced pluripotent stem cell-derived cardiomyocytes from different developmental stages. Stem Cell Res Ther. (2021) 12:46. doi: 10.1186/s13287-020-02089-5
125. Funakoshi S, Miki K, Takaki T, Okubo C, Hatani T, Chonabayashi K, et al. Enhanced engraftment, proliferation and therapeutic potential in heart using optimized human ipsc-derived cardiomyocytes. Sci Rep. (2016) 6:19111. doi: 10.1038/srep19111
126. Varzideh F, Pahlavan S, Ansari H, Halvaei M, Kostin S, Feiz M, et al. Human cardiomyocytes undergo enhanced maturation in embryonic stem cell-derived organoid transplants. Biomaterials. (2019) 192:537–50. doi: 10.1016/j.biomaterials.2018.11.033
127. Wimmer R, Leopoldi A, Aichinger M, Wick N, Hantusch B, Novatchkova M, et al. Human blood vessel organoids as a model of diabetic vasculopathy. Nature. (2019) 565:505–10. doi: 10.1038/s41586-018-0858-8
128. Tian Y, Liu Y, Wang T, Zhou N, Kong J, Chen L, et al. A microrna-hippo pathway that promotes cardiomyocyte proliferation and cardiac regeneration in mice. Sci Trans Med. (2015) 7:ra38–38. doi: 10.1126/scitranslmed.3010841
129. Liu H, Shi J, Wang H, Xuan Q, Bei Y, Sun W, et al. Abstract 309: mir-31a-5p controls cardiomyocyte proliferation in postnatal hearts. Circ Res. (2015) 117:A309. doi: 10.1161/res.117.suppl_1.309
130. Collesi C, Zentilin L, Sinagra G, Giacca M. Notch1 signaling stimulates proliferation of immature cardiomyocytes. J Cell Biol. (2008) 183:117–28. doi: 10.1083/jcb.200806091
131. Rojas A, Kong S, Agarwal P, Gilliss B, Pu W, Black B. Gata4 is a direct transcriptional activator of Cyclin D2 and Cdk4 and is required for cardiomyocyte proliferation in anterior heart field-derived myocardium. Mol Cell Biol. (2008) 28:5420–31. doi: 10.1128/MCB.00717-08
132. Bassat E, Mutlak Y, Genzelinakh A, Shadrin I, Baruch Umansky K, Yifa O, et al. The extracellular matrix protein agrin promotes heart regeneration in mice. Nature. (2017) 547:179–84. doi: 10.1038/nature22978
133. Magadum A, Ding Y, He L, Kim T, Vasudevarao M, Long Q, et al. Live cell screening platform identifies pparδ as a regulator of cardiomyocyte proliferation and cardiac repair. Cell Res. (2017) 27:1002–19. doi: 10.1038/cr.2017.84
134. Eulalio A, Mano M, Ferro M, Zentilin L, Sinagra G, Zacchigna S, et al. Functional screening identifies mirnas inducing cardiac regeneration. Nature. (2012) 492:376–81. doi: 10.1038/nature11739
135. Gise A, Lin Z, Schlegelmilch K, Honor L, Pan G, Buck J, et al. Yap1, the nuclear target of hippo signaling, stimulates heart growth through cardiomyocyte proliferation but not hypertrophy. Proc Natl Acad Sci USA. (2012) 109:2394–9. doi: 10.1073/pnas.1116136109
136. Engel F, Hsieh P, Lee R, Keating M. Fgf1/P38 map kinase inhibitor therapy induces cardiomyocyte mitosis, reduces scarring, and rescues function after myocardial infarction. Proc Natl Acad Sci USA. (2006) 103:15546–51. doi: 10.1073/pnas.0607382103
137. Morikawa Y, Heallen T, Leach J, Xiao Y, Martin J. Dystrophin–glycoprotein complex sequesters yap to inhibit cardiomyocyte proliferation. Nature. (2017) 547:227–31. doi: 10.1038/nature22979
138. Li J, Gao E, Vite A, Yi R, Gomez L, Goossens S, et al. Alpha-catenins control cardiomyocyte proliferation by regulating yap activity. Circ Res. (2015) 116:70–9. doi: 10.1161/CIRCRESAHA.116.304472
Keywords: cardiac regeneration, cell-free therapies, cell-based therapies, hPSC-CMs, transplantation
Citation: Wang J, An M, Haubner BJ and Penninger JM (2023) Cardiac regeneration: Options for repairing the injured heart. Front. Cardiovasc. Med. 9:981982. doi: 10.3389/fcvm.2022.981982
Received: 30 June 2022; Accepted: 29 December 2022;
Published: 12 January 2023.
Edited by:
Ajit Magadum, Temple University, United StatesReviewed by:
Alessandro Bertero, University of Turin, ItalyVerena Schwach, University of Twente, Netherlands
Jie Na, Tsinghua University, China
Copyright © 2023 Wang, An, Haubner and Penninger. This is an open-access article distributed under the terms of the Creative Commons Attribution License (CC BY). The use, distribution or reproduction in other forums is permitted, provided the original author(s) and the copyright owner(s) are credited and that the original publication in this journal is cited, in accordance with accepted academic practice. No use, distribution or reproduction is permitted which does not comply with these terms.
*Correspondence: Josef M. Penninger, am9zZWYucGVubmluZ2VyQHViYy5jYQ==