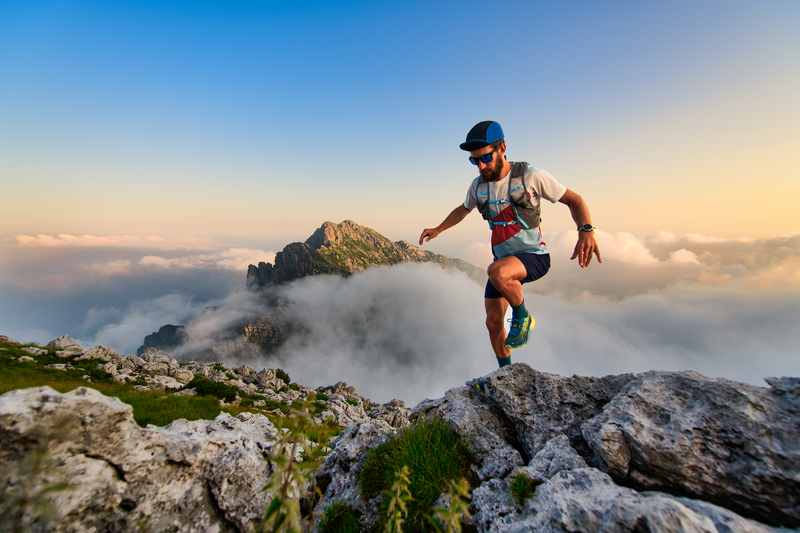
94% of researchers rate our articles as excellent or good
Learn more about the work of our research integrity team to safeguard the quality of each article we publish.
Find out more
REVIEW article
Front. Cardiovasc. Med. , 15 November 2022
Sec. Hypertension
Volume 9 - 2022 | https://doi.org/10.3389/fcvm.2022.970036
Hypertension has developed into an escalating serious global public health problem with multiple and unclear pathophysiological mechanisms. Recent studies have identified intestinal microbiota as a key perpetrator of hypertension through a variety of mechanisms. In this review, we highlight the potential roles of the intestinal microbiota and its metabolites in the development of hypertension, as well as the therapeutic potential for targeting intestinal microbiomes. We also shed light on the main limitations and challenges of the current research and suggest directions for future investigations. Finally, we discuss the development of accurate and personalized preventive and therapeutic strategies for hypotension by the modulation of intestinal microbes and metabolites.
Hypertension is recognized as the most prominent risk factor for cardiovascular disease (CVD) and stroke worldwide (1, 2) and leads to ~9.4 million deaths globally every year (3). In the United States, approximately half of the adult population has hypertension (4); in China, the number of adult patients with hypertension between 2012 and 2015 reached 244.5 million (5). Approximately, 50% of adults between 35 and 75 years of age have hypertension; however, less than one-third are receiving treatment, and fewer than one in 12 are in control of their blood pressure (6). In addition, there is a continuous increase in the prevalence of hypertension, which has led to the search for more effective strategies to prevent and modify the development of hypertension.
The pathogenesis and pathophysiology of hypertension are complex and unclear. Previous studies have shown that hypertension is thought to be driven by a combination of genetic and lifestyle factors, but genome-wide association studies show that only ~5% of the incidence of hypertension can be explained by genetics (7). In addition to genetic factors, the environment, diet, nervous system, and immune response have been reported as independent risk factors for hypertension (8, 9). Moreover, recent studies have shown that intestinal dysbiosis is regarded as an essential risk factor for hypertension (10, 11), which has provided a promising new therapeutic approach for hypertension (12, 13). Following the development and maturity of genome sequencing, bioinformatics, and metagenomics technologies, great progress has been made in studying intestinal microbiota. The intestinal microbiota contains more than 100 trillion microorganisms, but Bacteroidetes, Firmicutes, and Actinobacteria account for the vast majority (14, 15). The intestinal microbiota remains homeostatic, but the microbial composition varies between individuals (10). Meanwhile, the intestinal microbiota is intimately connected with many crucial organs or systems of the host, such as the brain, autonomic nervous system, bone marrow, kidney, vasculature, and immune system (16). Various factors lead to changes in the composition and positioning of microbiota, which are known as dysbiosis, and predispose patients to multiple diseases, such as gastrointestinal disease, obesity, type 2 diabetes mellitus (T2DM), non-alcoholic fatty liver disease, non-alcoholic steatohepatitis (NASH), chronic kidney disease (CKD), CVD, and hypertension (10, 17, 18). Studies have identified the association of intestinal dysbiosis with hypertension and associations of the brain–gut, kidney–gut, and microbial metabolite–host interactions in BP homeostasis mediated by the metabolic potential of the intestinal microbiota (19). In this review, the evidence supporting the role of intestinal microbiota in hypertension is summarized. Furthermore, the complex reciprocity between the intestinal microbiota and the development of hypertension and the underlying mechanisms is emphasized. Finally, the potential benefits of targeting the intestinal microbiota to regulate BP and prevent or treat hypertension are also described.
Studies have demonstrated a direct correlation between the gut microbiota and hypertension in both patients and animal models, including Dahl salt-sensitive rats, spontaneously hypertensive rats (SHR), angiotensin II (Ang II)-induced hypertensive rats, and deoxycorticosterone acetate (DOCA)-salt mice (20–23) (Figure 1). Bier et al. used 16S rRNA amplicon sequencing to detect the composition of the intestinal microbiota in fecal samples of Dahl salt-sensitive rats induced by a high-salt diet. They found a positive correlation between abundance and BP in six taxa, including the Pseudomonadales order, the Christensenellaceae, Barnesiellaceae, Eubacteriaceae families, and the Erwinia and Anaerofustis genera, whereas the abundance of the Anaerostipes genus showed a significant negative correlation with BP (24). Wilck et al. revealed that the distribution of Lactobacillus spp. was suppressed in high-salt diet-induced hypertensive mice, as the supplementation of Lactobacillus spp. in a mice model was shown to attenuate salt-sensitive hypertension, presumably by modulating the response of Th17-cells (25). In high-salt diet-induced hypertensive rats, Bier et al. found that the abundance of the Erwinia genus, Christensenellaceae, and Corynebacteriaceae was increased, whereas that of Anaerostipes was significantly reduced (24). Another study in rats with high-salt-induced hypertension showed that Spirochaete, Actinobacteria, Firmicutes, and Proteobacteria were elevated and Verrucomicrobia and Bacteroidetes were decreased (26). Yang et al. isolated the fecal DNA from SHR; mean arterial pressure, MAP: 148 ± 10 mmHg) and Wistar rats (MAP: 108 ± 2 mmHg), and found that the abundance of Firmicutes and Verrucomicrobia was significantly increased in SHR, whereas that of Bacteroidetes and Actinobacteria was reduced (27). Similarly, in SHR, Adnan et al. reported the proportion of Firmicutes and Lactobacillus was increased, but that of Bacteroidetes, Adlercreutzia, and Bifidobacterium was suppressed (28). In addition, in Wistar rats, Yan et al. reported that the high-salt diet-induced gut dysbiosis, including the reduction of beneficial Bacteroides, which could inhibit the production of intestinal-derived corticosterone induced by a high-salt diet through its metabolite arachidonic acid (26). Chima et al. found that Ang II-treated mice were accompanied by significant alterations in the microbiota (29). For example, consistent with other results, Anaeroplasmataceae increased in the Ang II-treated groups, whereas Lachnospiraceae decreased (29, 30). Moreover, shifts in the gut microbiome-associated metabolites, which are completely dependent on the intestinal microbiota, were observed in an Ang II-induced hypertension mouse model of hypertension (29). For example, microbiome associated- metabolites, such as 4-ethylphenylsulfate, p-cresol sulfate, p-cresol glucuronide, taurodeoxycholate, and taurodeoxycholic acid, were upregulated by Ang II (29, 31). However, metabolites such as N,N,N-trimethyl-5-aminovalerate, trans-4-hydroxyproline, indoleacetate, and xylose were significantly downregulated by Ang II treatment (29).
Figure 1. The intestinal microbiota composition in hypertension animal models and human individuals of hypertension. (A) The intestinal microbiota changes in different hypertension animal models. (B) The intestinal microbiota changes in hypertension individuals.
In hypertensive DOCA-salt rats, Firmicutes and Lactobacillales were found to be increased while Sutterella, Actinobacteria, and Oscillospira were reduced (32). Hsu et al. revealed that the proportion of Akkermansia and Odoribacter was increased and that of Lactobacillus was reduced in a model of maternal high-fructose diet-induced hypertension (33). Cold exposure has been recognized as an important risk factor for hypertension. Wang et al. analyzed the gut microbiota of rats using the 16S rDNA sequence in cold-induced hypertension and found that the abundance of Quinella, Rothia, and Senegalimassilia genera was significantly increased, but that of Lactobacillus, Lachnospiraceae, and Ruminococcaceae was decreased (34). Individuals with obstructive sleep apnea (OSA) are at increased risk for systemic hypertension; Durgan et al. established an OSA-related model of hypertensive rats by intermittent hypoxia and a high-fat diet. In this OSA-related model of hypertension, the abundance of Lactococcus and Coprobacillus was elevated and that of Ruminococcaceae was decreased (35). However, there are differences in the dysbiosis characteristics, including at the phylum, family, and genus levels, of different animal models of hypertension (36) (Figure 1; Table 1).
Human investigations have illustrated the relationship between the composition of the intestinal microbiome and hypertension (37–40). Multiple clinical studies have demonstrated changes in the composition of intestinal microbiota in patients with hypertension (Table 2). Hypertension is usually accompanied by decreased intestinal biodiversity and dysbiosis, such as increased Firmicutes/Bacteroidetes ratio (48). In patients with hypertension, the bacteria which are beneficial to health conditions were diminished, including Faecalibacterium, Bacteroides, Roseburia, Bifidobacterium, Coprococcus, and Butyrivibrio, whereas Veillonella, Prevotella, and Klebsiella were increased (3, 11, 41, 49). Sun et al. examined cross-sectional associations between measures of gut microbial diversity and taxonomic composition and BP in 529 participants. The data showed that 18 genera were associated with hypertension, including Anaerovorax, Clostridium IV, Oscillibacter, and Sporobacter, and the distribution of Veillonella aligned with hypertensive individuals (42). Moreover, Anaerovorax, Catabacter, and Robinsoneilla were demonstrated to be positively correlated with hypertension (42). Dan et al. performed 16S amplicon sequencing of 129 fecal samples, including 62 cases with normal BP and 67 cases with high BP, and found that there were 54 differentially expressed genera between the hypertensive and normal BP groups; 18 genera were significantly more abundant in the hypertensive group, including Acetobacteroides, Alistipes, Bacteroides, Barnesiella, Butyricimonas, Christensenella, Cosenzaea, Desulfovibrio, Dialister, Eisenbergiella, Faecalitalea, Megasphaera, Microvirgula, Mitsuokella, Parabacteroides, Proteiniborus, Clostridium sensu tricto, and Terrisporobacter (43). Kim et al. analyzed 40 fecal samples from 22 hypertensive individuals and 18 normal controls and found that Parabacteroides johnsonii, Eubacterium siraeum, and Alistipes finegoldii were present at a higher abundance in patients with hypertension, and Bacteroides thetaiotaomicron, a butyrate-producing bacterium, was present at a lower abundance in the hypertensive group (44). Yan et al. characterized the gut microbiome in 60 patients with hypertension (BP ≥ 140/90 mmHg) and 60 sex-, age-, and body weight-matched healthy controls (BP ≤ 120/80 mmHg) by comparing fecal samples based on whole-metagenome shotgun sequencing. Yan et al. found that Klebsiella, Clostridium, Streptococcus, Parabacteroides, Eggerthella, and Salmonella were frequently distributed in the hypertensive gut compared with normal controls, whereas Faecalibacterium, Roseburia, and Synergistetes were higher in the control group than in patients with hypertension (45). Calderón-Pérez et al. reported a higher distribution of Bacteroides coprocola, Bacteroides plebeius, and Lachnospiraceae genera in individuals with hypertension, but a lower abundance of Ruminococcaceae, Ruminococcaceae, Christensenellaceae, Faecalibacterium prausnitzii, and Roseburia hominis in the hypertensive gut (46). Recently, Palmu et al. reported that 45 microbial genera were observed to be positively associated with BP indices, of which 27 belong to the phylum Firmicutes, whereas there were negative associations between 19 different Lactobacillus species and BP indexes (47). Importantly, the richness of opportunistic pathogenic taxa, gram-negative bacteria, and lactate-producing genera was increased, as well as the Firmicutes/Bacteroidetes ratio, which is deemed as an indicator of intestinal microbiota health (10, 27, 50, 51). However, as shown in Table 2, the studies have yielded inconsistent results, which may be largely due to the heterogeneity of intestinal microbiota. Existing evidence has demonstrated that fecal microbiota transplantation (FMT) from patients and animals with hypertension to normotensive individuals can elevate BP levels (41, 52), and impair endothelial function (53). Human and animal studies further indicated that the intestinal microbiota could reasonably be regarded as a fundamental mediator of hypertension. However, the optimal profile of the intestinal microbiota in animal models of hypertension or human subjects remains contentious or even inverse. Thus, clarifying the specific microbial alternations in the state of hypertension is the next urgent and challenging task.
In addition, patients with hypertension also had lower α-diversity and abundance of short-chain fatty acids (SCFAs)-producing microbiota (10, 39, 50). SCFAs are saturated fatty acids that contain carbon chains of one to six carbons in length, the main SCFAs in the human body are acetate, propionate, and butyrate (54). SCFAs serve as energy substrates for intestinal epithelial cells as well as key regulators of anti-inflammatory responses, lipid metabolic pathways, and gluconeogenesis via a series of G-protein-coupled receptors (GPCRs) (55). Verhaar et al. studied the feces of 4,672 individuals (49.8 ± 11.7 years, 48% men) from six different ethnic groups, finding that Roseburia spp., Clostridium spp., Romboutsia spp., and Ruminococcaceae spp. were the best microbial predictors for SBP. Fecal SCFA levels, such as acetate and propionate, were lower in young Dutch participants with low SBP (39). Bier et al. observed a negative correlation between the taxa of the Actinobacteria phylum and the butyric acid level, independent of dietary changes in Dahl salt-sensitive rats (24). Lactate-producing bacteria are positively associated with SBP, whereas butyrate-producing bacteria and acetate-producing microbiota are negatively associated with SBP (56, 57).
As previous studies have shown that the sympathetic nervous system (SNS) modulates BP levels by promoting peripheral vasoconstriction, and heart rate, and by regulating water and sodium balance by innervating the nephron, the renal vasculature, and the juxtaglomerular cells (58–60). Excessive sympathetic activation was considered to be one of the major pathogenetic mechanisms of hypertension. Importantly, recent studies have demonstrated that sympathetic activation could be regulated by intestinal microbiota (Figure 2A). Studies have revealed that the microbiota modulates sympathetic activation via a gut–brain circuit mediated by the metabolites, including gamma-aminobutyric acid, dopamine, noradrenaline, and serotonin (5-hydroxytryptamine, 5HT), which are synthesized by intestinal microbiota, such as Candida, Escherichia, Streptococcus, Bacillus, and Enterococcus (56, 61, 62). Specifically, the dysbiosis-related bacterial metabolite imbalance increases the production of serotonin 5HT by the enterochromaffin cells in the gut (63); then, 5HT can modulate the activity of gut vagal afferents via 5HT3 receptors (5HT3Rs) potentially dampening the vagal gut–brain neural axis, whereas 5HT released into circulation can affect the vasculature and cause vasoconstriction (64). Nonetheless, the increased sympathetic activation can also contribute to epithelial dysfunction, increased intestinal permeability, and dysbiosis, increasing the translocation of microbiota metabolites into circulation (64). As a result, it further adversely affects the cardio-renal tissues.
Figure 2. The key mechanisms of intestinal microbiota in regulating the hypertension process. Intestinal dysbiosis leads to disruption of the nervous system, renal function, intestinal SCFAs, immune system, and increases LPS production and intestinal permeability, thereby aggravating the progression of hypertension. (A) Intestinal microbiota and its metabolites (mainly including GABA, dopamine, noradrenaline and 5HT) regulate hypertension by modifying sympathetic and vagal nerve activity and neuroinflammatory response through the gut-brain axis. (B) Intestinal microbiota and its metabolites regulate hypertension via renal sympathetic regulation, humoral regulation, vasoactive substances (RAAS, nitric oxide, and endothelin), and even lead to hypertension by triggering chronic kidney disease (CKD). (C) The indigestible dietary fiber was fermented into SCFAs, primarily involving acetate, propionate, and butyrate, by intestinal microbiota. Subsequently, the SCFAs regulate hypertension via binding to the specific GPCRs which mainly include GPR43, GPR41, GPR109A, and Olfr78. (D) Intestinal microbiota and endotoxins (LPS) regulate hypertension via the immune and inflammation systems. Abbreviations: BP, blood pressure; PNS, peripheral nervous system; SNS, sympathetic nervous system; ENS, enteric nervous system; RAAS, renin-angiotensin-aldosterone system; GABA, gamma-aminobutyric acid; NE, noradrenaline; 5HT, 5-hydroxytryptamine; ROS, reactive oxygen species; TMA, trimethylamine; TMAO, trimethylamine-N-oxide; FMO, flavin monooxygenases; CKD, chronic kidney disease; SCFAs, short-chain fatty acids; GPR, G protein coupled receptor; HDAC, histone deacetylase; TLR4, Toll-like receptor 4.
Intestinal dysbiosis contributes to the increase of intestinal barrier permeability and activation of immune cells, which leads to impaired blood–brain barrier (BBB) function and directly results in central nervous system neuroinflammation, which plays a pivotal role in the progression of hypertension (16, 65). Furthermore, intestinal microbiota alteration affects the cerebral immune cells, such as microglia, the most abundant resident immune cells in the brain (66–68). Sharma et al. found that the inhibition of microglial activation in PVN inhibited sympathetic activation, and lowered BP in Ang II-induced rodent hypertension (22). Toral et al. demonstrated that the alteration of intestinal microbiota influences PVN NADPH oxidase activity, neuroinflammation, and sympathetic activity, subsequently impacting BP levels (57). The activated microglia release a variety of pro-inflammatory and toxic products, including ROS and cytokines (69), which indirectly promote sympathetic activation and increase BP (70). Erny et al. found that defective microglia can be repaired by microbiota or SCFAs (71). Meanwhile, intestinal dysbiosis can also promote Th1 cell infiltration, allowing local crosstalk with M1 microglia, which in turn triggers the differentiation of microglia to a pro-inflammatory state (68). Moreover, products of the intestinal microbiota, such as indoxyl sulfate, increase neuroinflammation (72). Intestinal dysbiosis also decreased levels of H2S, which is an endogenous vasoactive factor and a neuromodulatory and neuroprotective molecule that plays an antihypertensive and anti-neuroinflammatory role (70).
The kidney plays important roles in the pathogenesis of hypertension via renal sympathetic regulation, humoral regulation, and vasoactive substances (e.g., RAAS, nitric oxide, and endothelin). Recently, the intestinal microbiota has been identified as a substantial participant in regulating the progression of kidney disease through the gut–kidney axis (16). Intestinal dysbiosis contributes to the overproduction and accumulation of uremic toxins, p-cresyl, phenols, advanced glycation end products, and indoles, which all impair the intestinal barrier and increase intestinal permeability, and infiltrate the kidneys via the circulation, leading to the progression of kidney disease (73, 74). Moreover, the intestinal inflammatory cells and cytokines contribute to renal inflammation and injury via circulation (16).
In addition, L-carnitine, choline, and lecithin can be converted into trimethylamine (TMA) by intestinal microbiota, such as Clostridia, Enterobacteriaceae, Anaerococcushydrogenalis, and Edwardsiella tarda (75, 76). Subsequently, TMA was converted to trimethylamine-N-oxide (TMAO) by flavin monooxygenases (FMO) in the liver and excreted through the kidneys. Important Ang II-induced hypertensive mouse model showed a higher TMAO levels which were intimately associated with CVD and CKD (58, 77). Animal studies showed that increased TMAO in a long-term diet directly contributes to progressive renal fibrosis and dysfunction (75, 77) and the vasoconstriction of renal afferent arterioles (78). TMAO induces the production of pro-inflammatory cytokines, such as TNF-α and IL-1B, and inhibits the production of anti-inflammatory cytokines, such as IL-10 (79). Studies have disclosed that the circulating TMAO level was positively correlated with BP, which may be associated with endothelial dysfunction, oxidative stress (11, 80, 81), and the prolonged hypertensive effects of Ang II (82, 83). Moreover, the elevated level of TMAO was suggested as an increased risk for myocardial infarction, heart failure, peripheral artery disease, and stroke (10). Recently, Jaworska et al. demonstrated that TMA, but not TMAO, can affect the viability of human vascular smooth muscle cells, thereby exerting a booster effect of hypertension (84). Hong-Bao Li et al. demonstrated that the Faecalibacterium genus was significantly depleted in patients with CKD and hypertension (CKD-hypertension) compared with the healthy controls. The supplementation of Faecalibacterium prausnitzii to CKD mice reduced renal dysfunction and inflammation by the Faecalibacterium prausnitzii-induced butyrate–renal GPR-43 axis (85), whereas F. prausnitzii supplementation may alleviate BP in patients with CKD-induced hypertension.
In the gut, indigestible dietary fiber is fermented into SCFAs, primarily involving acetate, propionate, and butyrate, by specific intestinal microbiota, including Roseburia, Ruminococcaceae, and Faecalibacterium spp (9, 14, 86); these intestinal microbiota have been demonstrated to be associated with moderate BP maintenance and shown to be less abundant in patients with hypertension (37, 44, 50, 87). In addition, the administration of a high-fiber diet and acetate supplementation showed significant alteration of the intestinal microbiota components, causing a remarkable increase in the proportion of Bacteroides acidifaciens, and led to a reduction in systolic BP (SBP) and diastolic BP (DBP) (3, 51).
Molecular mechanism studies have revealed that SCFAs regulate BP through a number of GPCRs, which mainly include the fatty acid receptor (FFAR)-2 (GPR43), FFAR 3 (GPR41), GPR109A (HCAR2), and olfactory receptor 78 (Olfr78) (9, 50, 62) (Figure 2). Animal studies showed that the activation of GPR41 in vascular endothelial cells stimulated by SCFAs leads to BP lowering and that mice lacking GPR42 (Gpr41−/−) have systolic hypertension, aortic thickening, and collagen deposition (88, 89). Similarly, GPR43 is widely expressed in a variety of tissues and plays a role in lowering BP in response to the stimulation of SCFAs (50). Waghulde et al. demonstrated that salt-sensitive hypertensive rats lack G protein-coupled estrogen receptor 1 (Gper1−/−), was accompanies the amelioration of hypertension, as well as the intestinal dysbiosis indicated by the diminished Firmicutes/Bacteroidetes ratio (90). Although the exact molecular mechanism by which the deletion of Gper1 mitigates hypertension remains unclear, the researchers speculated that this process may be mediated by the gut microbiota (90). Olfr78 is localized in the renal afferent arteriole and vascular smooth muscle cells. In response, SCFAs, which mainly contain acetate and propionate, perform a unique role in facilitating renin secretion, eventually leading to high renin concentration and hypertension (50, 91, 92). Moreover, Olfr78 activation induced a counteraction of the hypotensive effect induced by GPR41 (93).
SCFAs are regarded as an important link between the intestinal microbiota and the immune system (3, 94), and selectively support the development of Th1, Th17, and Treg cells according to the cytokine and immune milieu (95). Studies revealed that SCFAs, particularly butyrate, mediate anti-inflammatory effects by inhibiting histone deacetylase (HDAC), and the inhibition of HDAC reduces pro-inflammatory and hypertensive responses by decreasing the production of ROS and the expression of Ang II type1 receptor (AT1r) in the myocardium (96, 97). Recently, Robles-Vera et al. demonstrated that acetate or butyrate supplementation prevented the development of hypertension in SHR, and restored Th17/Treg balance in the aorta (98). Furthermore, SCFAs can dampen glial inflammatory responses and subsequently decrease BP (70). Studies have demonstrated that SCFAs and FFA2 receptors improve the development of hypertension by inhibiting monocyte and dendritic cell activity (99).
In addition, vagal afferent receptors express SCFAs sensing to participate in BP modulation. SCFAs have also been suspected to participate in gut–brain communication, thereby becoming involved in the neural regulation of BP. FMT from normotensive to hypertensive animals has been shown to ameliorate BP levels in hypertensive animals and was accompanied by increased expression of GPR41 and GPR43 in PVN (64). Yang et al. showed that excessive colonic acetate levels can lower BP by activating parasympathetic nerves. Meanwhile, the diminished expression of butyrate-sensing receptors in the hypothalamus contributed to destroying the BP alleviation induced by butyrate administration in SHR (100). Therefore, the reduction in the availability of circulating SCFAs and SCFA-sensing receptors contributes to the pathophysiology of hypertension.
The immune system and exaggerated inflammation have been demonstrated to play important roles in the hypertension process (101–103). Recently, the intestinal microbiota was indicated as a key regulator of the immune and inflammatory response (104) (Figure 2). The intestine is the largest immune organ in the body, with a complex mucosal immune system, lymphocytes, and innate immune cells spread throughout the epithelial layer (16). Moreover, the development of the intestinal immune system depends on the intestinal microbiota (105). The lack of intestinal microbiota can lead to inadequate gut-associated lymphoid tissues (GALTs) development and systemic and central immune abnormalities (16, 106, 107). Germ-free animals are accompanied by a significant reduction in the richness of TH17 cells, B cells, and disequilibrium of TH1 and TH2 responses, and impaired Treg cell function (16). Preclinical studies have shown that T lymphocyte subsets, such as Th1, Th2, Th17, and Treg cells, participate in the regulation of BP and end-organ damage (3, 108). Th1 and Th17 cells release pro-inflammatory cytokines, such as IFN-γ, TNF-α, and IL-17a, which are prominent pathogens in hypertension models (104, 109) while Treg cell inhibits Ang II-induced hypertension by releasing anti-inflammatory cytokines, which mainly include IL-10 and TGF-β (110).
The intestinal dysbiosis, including the increased Bilophila wadsworthia and Clostridium cocleatum and the decreased Bifidobacterium and Bacteroides, leads to an increase in LPS production and permeability of the intestine (111). LPS infiltrates into the circulation across the highly permeable intestinal wall to form a complex with LBP, which binds to CD14 on monocytes, contributing to the production of pro-inflammatory cytokines, such as TNF-α, IL-1, and IL-6 (50, 112). Additionally, LPS also interacts with PRR (TLR4) to induce inflammatory responses and increase arterial pressure and vascular dysfunction (113–116). In animal models, LPS administration caused an increase in heart rate, norepinephrine level, and neuroinflammation, as well as decreased baroreflex sensitivity, which was confirmed by increased expression of TLR and TNF-α in the PVN (117). Wang et al. discovered that the inhibition of TLR4 in PVN caused amelioration of hypertension in SHR via a decrease in ROS production and pro-inflammation cytokines (118). Moreover, intestinal dysbiosis also directly increases the production of pro-inflammatory cytokines and ROS, which leads to the production of ox-LDL (13). Subsequently, Ox-LDL has an inhibitory function in the production of nitric oxide (NO), a well-recognized vasodilator, and endothelin-1, leading to the exacerbation of hypertension (119). The intestinal microbiota at least partially contributes to Ang II-induced vascular dysfunction and hypertension by supporting MPC-1/IL-17-driven vascular immune cell infiltration and inflammation (120). SNS directly innervates the primary immune organ (bone marrow), and sympathetic activation can induce bone marrow hemopoietic stem cells into a pro-inflammatory state through the brain–gut–bone marrow axis (121, 122). Bone marrow-derived immune cells can be activated by the intestinal microbiota, leading to low-grade chronic inflammation, which is a recognized risk factor for hypertension (16, 101).
Recent evidence suggests that intestinal microbiota-related strategies, such as FMT, probiotics, prebiotics, and synbiotics, may be considered as promising strategies for the prevention and treatment of hypertension (10, 123, 124). In high-salt-induced hypertensive mice, FMT administration decreased the richness of Allobaculum, Dubosiella, and Alloprevotella, but increased the relative abundance of Lachnospiraceae_UCG-006 and Lachnoclostridium (125). Adnan et al. transplanted the fecal microbiota extracted from spontaneously hypertensive stroke-prone rats into WKY rats and found an increase of 26 mmHg in SBP in WKY rats, accompanied by a significantly increased ratio of Firmicutes/Bacteroidetes (28). Importantly, the transplantation of microbiota from normotensive into hypertensive animals has caused the amelioration of BP levels in animal models of hypertension and was accompanied by an increase of SCFAs receptors expression in PVN (64). Toral et al. found that FMT from WKY rats to SHR rats decreased basal SBP, restored the Th17/Treg imbalance, improved endothelial dysfunction, and alleviated vascular oxidative stress (53). Unfortunately, no studies have been published on the treatment of hypertension with FMT.
Probiotics, containing SCFAs-producing microbiota, are defined as living microorganisms that can modify the composition of the microbiome to benefit the host (11, 15). Probiotics supplementation is conducive to intestinal barrier function, reduces endotoxemia and increases butyrate (15, 98), and further significantly lowers SBP and DBP (49). Robles-Vera et al. demonstrated that probiotic Bifidobacterium breve CECT7263 (BFM) prevented DOCA-salt hypertension and renal damage by increasing acetate and reducing TMA production (23). Similarly, Wilck et al. found that salt-sensitive mice supplemented with Lactobacillus murinus had ameliorated hypertension compared with controls (25, 126). Nonetheless, the antihypertensive activity of probiotics depends on the specific strain; even for the same strain of probiotics, the antihypertensive effect varies in different hypertension models (49). Intervention with Lactiplantibacillus plantarum strains SR37-3 (PFM-SR37-3) and SR61-2 (PFM-SR61-2) significantly lowered the BP of NG-nitro-L-arginine methyl ester induced hypertensive rats and attenuated renal injury (127).
Prebiotics are defined as a healthy matrix that is selectively utilized by host microorganisms to promote the growth of beneficial intestinal microorganisms (128, 129). Kaye et al. found that the administration of prebiotic fiber has protective effects on hypertension and cardiac hypertrophy, mediated by GPR43/GPR109A (9). Hsu et al. reported that the prebiotic treatment prevented BP elevation and diminished the ratio of Firmicutes/Bacteroidetes (130). Meanwhile, the renal mRNA expression of ACE and plasma TMAO levels were concomitantly decreased (130).
The word synbiotics refers to a mixture of living microorganisms and substrates that are selectively utilized by the host microorganisms and beneficial to the host's health (131). A systematic review and meta-analysis of clinical trials conducted by Hadi et al. showed that synbiotic interventions improved SBP in patients with hypertension (128). Similarly, Bartolomaeus et al. showed that synbiotic management increased intestinal SCFAs production and significantly reduced BP levels in patients (132).
Hypertension presents a significant public health challenge and is a major risk factor for CVD, cerebrovascular, and CKD (133). Accumulating evidence in recent years supports that the intestinal microbiota and its metabolites are essential regulators of hypertension and its complications (11). Alterations in the intestinal microbial composition associated with disease and potential virulent metabolites have been considered a contributor to the development of hypertension. Sympathetic activation and neuroinflammation, induced by intestinal dysbiosis, have been recognized as key players in hypertension. Similarly, intestinal microbial metabolism, such as SCFAs, TMAO, and endotoxins (LPS), has a substantial influence on hypertension through its effect on undulating vasomotion, renal function, neural activation, and inflammation.
Consequently, modification of the intestinal microbiota is considered a promising tactic to improve personalized BP control. Nevertheless, owing to past technical limitations and insufficient understanding of intestinal microbiota, the current in-depth research on intestinal microbiota and hypertension remains limited. Currently, evidence in humans is circumspect and indeterminate, and the understanding of the mechanisms is widely based on rodent models. In many cases, the causal relationship between dysbiosis and hypertension is still inconclusive. In addition, the specific mechanism by which intestinal microbiota affects the progression of hypertension remains largely ambiguous. The lack of longitudinal studies makes it extremely challenging to identify the specific alternations in the intestinal microbiota of patients with hypertension; hence, individualized treatment consisting of modulation of the microbiota remains largely challenging. Furthermore, the safety and effectiveness of preventing and treating hypertension via intervention in the intestinal microbiota require further evaluation. Therefore, the management of the intestinal microbiome for the prevention and treatment of patients with hypertension is full of prospective challenges and potential.
In the future, genome-wide correlation studies should be combined with intestinal microbiome analysis to provide personalized data on the individual composition of the microbiota. The mechanism through which the intestinal microbiota affects hypertension requires further investigation, particularly regarding the specific bacterial species. In addition, more clinical evidence is required to validate the results of experiments in rodent models. It is extremely important to emphasize that the main site for sensing pro-hypertensive signals is the brain or the intestine, or both contribute equally.
In conclusion, the implementation of individualized intestinal microbiota intervention therapy strategies for hypertension is expected to occur. The use of intestinal microbiota modulation as a therapeutic option for hypertension has excellent potential in the development of personalized strategies for hypertension management.
LH and JY contributed to the conception and design of the study. DS and HX wrote the first draft of the manuscript. All authors reviewed and edited the manuscript. All authors contributed to the article and approved the submitted version.
This work was supported by Wuhan No. 1 Hospital, Wuhan Hospital of Traditional Chinese and Western Medicine.
The authors declare that the research was conducted in the absence of any commercial or financial relationships that could be construed as a potential conflict of interest.
All claims expressed in this article are solely those of the authors and do not necessarily represent those of their affiliated organizations, or those of the publisher, the editors and the reviewers. Any product that may be evaluated in this article, or claim that may be made by its manufacturer, is not guaranteed or endorsed by the publisher.
1. Worldwide trends in blood pressure from 1975 to 2015: a pooled analysis of 1479 population-based measurement studies with 19·1 million participants. Lancet. (2017) 389:37–55. doi: 10.1016/S0140-6736(16)31919-5
2. Liu ZZ, Jose PA, Yang J, Zeng C. Importance of extracellular vesicles in hypertension. Exp Biol Med. (2021) 246:342–53. doi: 10.1177/1535370220974600
3. Marques FZ, Mackay CR, Kaye DM. Beyond gut feelings: how the gut microbiota regulates blood pressure. Nat Rev Cardiol. (2018) 15:20–32. doi: 10.1038/nrcardio.2017.120
4. Whelton PK, Carey RM, Aronow WS, Casey DE Jr, Collins KJ, Dennison Himmelfarb C, et al. 2017 ACC/AHA/AAPA/ABC/ACPM/AGS/APhA/ASH/ASPC/NMA/PCNA Guideline for the prevention, detection, evaluation, and management of high blood pressure in adults: a report of the American College of Cardiology/American Heart Association Task Force on Clinical Practice Guidelines. Hypertension. (2018) 71:e13–115. doi: 10.1161/HYP.0000000000000065
5. Wang Z, Chen Z, Zhang L, Wang X, Hao G, Zhang Z, et al. Status of hypertension in China: results from the china hypertension survey, 2012–2015. Circulation. (2018) 137:2344–56. doi: 10.1161/CIRCULATIONAHA.117.032380
6. Lu J, Lu Y, Wang X, Li X, Linderman GC, Wu C, et al. Prevalence, awareness, treatment, and control of hypertension in China: data from 1·7 million adults in a population-based screening study (China PEACE Million Persons Project). Lancet. (2017) 390:2549–58. doi: 10.1016/S0140-6736(17)32478-9
7. Giri A, Hellwege JN, Keaton JM, Park J, Qiu C, Warren HR, et al. Trans-ethnic association study of blood pressure determinants in over 750,000 individuals. Nat Genet. (2019) 51:51–62. doi: 10.1038/s41588-018-0303-9
8. Smiljanec K, Lennon SL. Sodium, hypertension, and the gut: does the gut microbiota go salty? Am J Physiol Heart Circ Physiol. (2019) 317:H1173–82. doi: 10.1152/ajpheart.00312.2019
9. Kaye DM, Shihata WA, Jama HA, Tsyganov K, Ziemann M, Kiriazis H, et al. Deficiency of prebiotic fiber and insufficient signaling through gut metabolite-sensing receptors leads to cardiovascular disease. Circulation. (2020) 141:1393–403. doi: 10.1161/CIRCULATIONAHA.119.043081
10. Jin L, Shi X, Yang J, Zhao Y, Xue L, Xu L, et al. Gut microbes in cardiovascular diseases and their potential therapeutic applications. Protein Cell. (2021) 12:346–59. doi: 10.1007/s13238-020-00785-9
11. Muralitharan RR, Jama HA, Xie L, Peh A, Snelson M, Marques FZ. Microbial peer pressure: the role of the gut microbiota in hypertension and its complications. Hypertension. (2020) 76:1674–87. doi: 10.1161/HYPERTENSIONAHA.120.14473
12. Aron-Wisnewsky J, Clément K. The gut microbiome, diet, and links to cardiometabolic and chronic disorders. Nat Rev Nephrol. (2016) 12:169–81. doi: 10.1038/nrneph.2015.191
13. Duttaroy AK. Role of gut microbiota and their metabolites on atherosclerosis, hypertension and human blood platelet function: a review. Nutrients. (2021) 13:144. doi: 10.3390/nu13010144
14. Jones RM, Neish AS. Gut microbiota in intestinal and liver disease. Annu Rev Pathol. (2021) 16:251–75. doi: 10.1146/annurev-pathol-030320-095722
15. Naqvi S, Asar TO, Kumar V, Al-Abbasi FA, Alhayyani S, Kamal MA, et al. A cross-talk between gut microbiome, salt and hypertension. Biomed Pharmacother. (2021) 134:111156. doi: 10.1016/j.biopha.2020.111156
16. Yang T, Richards EM, Pepine CJ, Raizada MK. The gut microbiota and the brain-gut-kidney axis in hypertension and chronic kidney disease. Nat Rev Nephrol. (2018) 14:442–56. doi: 10.1038/s41581-018-0018-2
17. Fan Y, Pedersen O. Gut microbiota in human metabolic health and disease. Nat Rev Microbiol. (2021) 19:55–71. doi: 10.1038/s41579-020-0433-9
18. Xiang H, Sun D, Liu X, She ZG, Chen Y. The role of the intestinal microbiota in nonalcoholic steatohepatitis. Front Endocrinol. (2022) 13:812610. doi: 10.3389/fendo.2022.812610
19. Raizada MK, Joe B, Bryan NS, Chang EB, Dewhirst FE, Borisy GG, et al. Report of the national heart, lung, and blood institute working group on the role of microbiota in blood pressure regulation: current status and future directions. Hypertension. (2017) 70:479–85. doi: 10.1161/HYPERTENSIONAHA.117.09699
20. Xia WJ, Xu ML, Yu XJ, Du MM, Li XH, Yang T, et al. Antihypertensive effects of exercise involve reshaping of gut microbiota and improvement of gut-brain axis in spontaneously hypertensive rat. Gut Microbes. (2021) 13:1–24. doi: 10.1080/19490976.2020.1854642
21. Abais-Battad JM, Saravia FL, Lund H, Dasinger JH, Fehrenbach DJ, Alsheikh AJ, et al. Dietary influences on the Dahl SS rat gut microbiota and its effects on salt-sensitive hypertension and renal damage. Acta Physiol. (2021) 232:e13662. doi: 10.1111/apha.13662
22. Sharma RK, Yang T, Oliveira AC, Lobaton GO, Aquino V, Kim S, et al. Microglial cells impact gut microbiota and gut pathology in angiotensin ii-induced hypertension. Circ Res. (2019) 124:727–36. doi: 10.1161/CIRCRESAHA.118.313882
23. Robles-Vera I, de la Visitación N, Toral M, Sánchez M, Romero M, Gómez-Guzmán M, et al. Probiotic bifidobacterium breve prevents DOCA-salt hypertension. FASEB J. (2020) 34:13626–40. doi: 10.1096/fj.202001532R
24. Bier A, Braun T, Khasbab R, Di Segni A, Grossman E, Haberman Y, et al. A high salt diet modulates the gut microbiota and short chain fatty acids production in a salt-sensitive hypertension rat model. Nutrients. (2018) 10:1154. doi: 10.3390/nu10091154
25. Wilck N, Matus MG, Kearney SM, Olesen SW, Forslund K, Bartolomaeus H, et al. Salt-responsive gut commensal modulates T(H)17 axis and disease. Nature. (2017) 551:585–9. doi: 10.1038/nature24628
26. Yan X, Jin J, Su X, Yin X, Gao J, Wang X, et al. Intestinal flora modulates blood pressure by regulating the synthesis of intestinal-derived corticosterone in high salt-induced hypertension. Circ Res. (2020) 126:839–53. doi: 10.1161/CIRCRESAHA.119.316394
27. Yang T, Santisteban MM, Rodriguez V, Li E, Ahmari N, Carvajal JM, et al. Gut dysbiosis is linked to hypertension. Hypertension. (2015) 65:1331–40. doi: 10.1161/HYPERTENSIONAHA.115.05315
28. Adnan S, Nelson JW, Ajami NJ, Venna VR, Petrosino JF, Bryan RM Jr, et al. Alterations in the gut microbiota can elicit hypertension in rats. Physiol Genomics. (2017) 49:96–104. doi: 10.1152/physiolgenomics.00081.2016
29. Cheema MU, Pluznick JL. Gut microbiota plays a central role to modulate the plasma and fecal metabolomes in response to angiotensin II. Hypertension. (2019) 74:184–93. doi: 10.1161/HYPERTENSIONAHA.119.13155
30. Vital M, Howe AC, Tiedje JM. Revealing the bacterial butyrate synthesis pathways by analyzing (meta)genomic data. mBio. (2014) 5:e00889. doi: 10.1128/mBio.00889-14
31. Cookson TA. Bacterial-induced blood pressure reduction: mechanisms for the treatment of hypertension via the gut. Front Cardiovasc Med. (2021) 8:721393. doi: 10.3389/fcvm.2021.721393
32. Robles-Vera I, de la Visitación N, Toral M, Sánchez M, Romero M, Gómez-Guzmán M, et al. Changes in gut microbiota induced by doxycycline influence in vascular function and development of hypertension in DOCA-salt rats. Nutrients. (2021) 13:2971. doi: 10.3390/nu13092971
33. Hsu CN, Chan JYH, Wu KLH, Yu HR, Lee WC, Hou CY, et al. Altered gut microbiota and its metabolites in hypertension of developmental origins: exploring differences between fructose and antibiotics exposure. Int J Mol Sci. (2021) 22:2674. doi: 10.3390/ijms22052674
34. Wang B, Liu J, Lei R, Xue B, Li Y, Tian X, et al. Cold exposure, gut microbiota, and hypertension: a mechanistic study. Sci Total Environ. (2022) 833:155199. doi: 10.1016/j.scitotenv.2022.155199
35. Durgan DJ, Ganesh BP, Cope JL, Ajami NJ, Phillips SC, Petrosino JF, et al. Role of the gut microbiome in obstructive sleep apnea-induced hypertension. Hypertension. (2016) 67:469–74. doi: 10.1161/HYPERTENSIONAHA.115.06672
36. Robles-Vera I, Toral M, Duarte J. Microbiota and hypertension: role of the sympathetic nervous system and the immune system. Am J Hypertens. (2020) 33:890–901. doi: 10.1093/ajh/hpaa103
37. Huart J, Leenders J, Taminiau B, Descy J, Saint-Remy A, Daube G, et al. Gut microbiota and fecal levels of short-chain fatty acids differ upon 24-hour blood pressure levels in men. Hypertension. (2019) 74:1005–13. doi: 10.1161/HYPERTENSIONAHA.118.12588
38. Jackson MA, Verdi S, Maxan ME, Shin CM, Zierer J, Bowyer RCE, et al. Gut microbiota associations with common diseases and prescription medications in a population-based cohort. Nat Commun. (2018) 9:2655. doi: 10.1038/s41467-018-05184-7
39. Verhaar BJH, Collard D, Prodan A, Levels JHM, Zwinderman AH, Bäckhed F, et al. Associations between gut microbiota, faecal short-chain fatty acids, and blood pressure across ethnic groups: the HELIUS study. Eur Heart J. (2020) 41:4259–67. doi: 10.1093/eurheartj/ehaa704
40. Takagi T, Naito Y, Kashiwagi S, Uchiyama K, Mizushima K, Kamada K, et al. Changes in the gut microbiota are associated with hypertension, hyperlipidemia, and type 2 diabetes mellitus in japanese subjects. Nutrients. (2020) 12:2996. doi: 10.3390/nu12102996
41. Li J, Zhao F, Wang Y, Chen J, Tao J, Tian G, et al. Gut microbiota dysbiosis contributes to the development of hypertension. Microbiome. (2017) 5:14. doi: 10.1186/s40168-016-0222-x
42. Sun S, Lulla A, Sioda M, Winglee K, Wu MC, Jacobs DR Jr., et al. Gut microbiota composition and blood pressure. Hypertension. (2019) 73:998–1006. doi: 10.1161/HYPERTENSIONAHA.118.12109
43. Dan X, Mushi Z, Baili W, Han L, Enqi W, Huanhu Z, et al. Differential analysis of hypertension-associated intestinal microbiota. Int J Med Sci. (2019) 16:872–81. doi: 10.7150/ijms.29322
44. Kim S, Goel R, Kumar A, Qi Y, Lobaton G, Hosaka K, et al. Imbalance of gut microbiome and intestinal epithelial barrier dysfunction in patients with high blood pressure. Clin Sci. (2018) 132:701–18. doi: 10.1042/CS20180087
45. Yan Q, Gu Y, Li X, Yang W, Jia L, Chen C, et al. Alterations of the gut microbiome in hypertension. Front Cell Infect Microbiol. (2017) 7:381. doi: 10.3389/fcimb.2017.00381
46. Calderón-Pérez L, Gosalbes MJ, Yuste S, Valls RM, Pedret A, Llauradó E, et al. Gut metagenomic and short chain fatty acids signature in hypertension: a cross-sectional study. Sci Rep. (2020) 10:6436. doi: 10.1038/s41598-020-63475-w
47. Palmu J, Salosensaari A, Havulinna AS, Cheng S, Inouye M, Jain M, et al. Association between the gut microbiota and blood pressure in a population cohort of 6953 individuals. J Am Heart Assoc. (2020) 9:e016641. doi: 10.1161/JAHA.120.016641
48. Silveira-Nunes G, Durso DF, Jr L, Cunha EHM, Maioli TU, Vieira AT, et al. Hypertension Is associated with intestinal microbiota dysbiosis and inflammation in a Brazilian population. Front Pharmacol. (2020) 11:258. doi: 10.3389/fphar.2020.00258
49. Li J, Yang X, Zhou X, Cai J. The role and mechanism of intestinal flora in blood pressure regulation and hypertension development. Antioxid Redox Signal. (2021) 34:811–30. doi: 10.1089/ars.2020.8104
50. Verhaar BJH, Prodan A, Nieuwdorp M, Muller M. Gut microbiota in hypertension and atherosclerosis: a review. Nutrients. (2020) 12:2982. doi: 10.3390/nu12102982
51. Marques FZ, Nelson E, Chu PY, Horlock D, Fiedler A, Ziemann M, et al. High-fiber diet and acetate supplementation change the gut microbiota and prevent the development of hypertension and heart failure in hypertensive mice. Circulation. (2017) 135:964–77. doi: 10.1161/CIRCULATIONAHA.116.024545
52. Toral M, Romero M, Rodríguez-Nogales A, Jiménez R, Robles-Vera I, Algieri F, et al. Lactobacillus fermentum improves tacrolimus-induced hypertension by restoring vascular redox state and improving eNOS coupling. Mol Nutr Food Res. (2018) 62:e1800033. doi: 10.1002/mnfr.201800033
53. Toral M, Robles-Vera I, de la Visitación N, Romero M, Sánchez M, Gómez-Guzmán M, et al. Role of the immune system in vascular function and blood pressure control induced by faecal microbiota transplantation in rats. Acta Physiol. (2019) 227:e13285. doi: 10.1111/apha.13285
54. Blacher E, Levy M, Tatirovsky E, Elinav E. Microbiome-modulated metabolites at the interface of host immunity. J Immunol. (2017) 198:572–80. doi: 10.4049/jimmunol.1601247
55. Sun D, Yang X, Wu B, Zhang XJ, Li H, She ZG. Therapeutic potential of g protein-coupled receptors against nonalcoholic steatohepatitis. Hepatology. (2021) 74:2831–8. doi: 10.1002/hep.31852
56. O'Connor KM, Lucking EF, Cryan JF, O'Halloran KD. Bugs, breathing and blood pressure: microbiota-gut-brain axis signalling in cardiorespiratory control in health and disease. J Physiol. (2020) 598:4159–79. doi: 10.1113/JP280279
57. Toral M, Robles-Vera I, de la Visitación N, Romero M, Yang T, Sánchez M, et al. Critical role of the interaction gut microbiota - sympathetic nervous system in the regulation of blood pressure. Front Physiol. (2019) 10:231. doi: 10.3389/fphys.2019.00231
58. Tang WH, Kitai T, Hazen SL. Gut microbiota in cardiovascular health and disease. Circ Res. (2017) 120:1183–96. doi: 10.1161/CIRCRESAHA.117.309715
59. Burnstock G, Loesch A. Sympathetic innervation of the kidney in health and disease: emphasis on the role of purinergic cotransmission. Auton Neurosci. (2017) 204:4–16. doi: 10.1016/j.autneu.2016.05.007
60. Drummond GR, Vinh A, Guzik TJ, Sobey CG. Immune mechanisms of hypertension. Nat Rev Immunol. (2019) 19:517–32. doi: 10.1038/s41577-019-0160-5
61. Muller PA, Schneeberger M, Matheis F, Wang P, Kerner Z, Ilanges A, et al. Microbiota modulate sympathetic neurons via a gut-brain circuit. Nature. (2020) 583:441–6. doi: 10.1038/s41586-020-2474-7
62. Felizardo RJF, Watanabe IKM, Dardi P, Rossoni LV, Câmara NOS. The interplay among gut microbiota, hypertension and kidney diseases: the role of short-chain fatty acids. Pharmacol Res. (2019) 141:366–77. doi: 10.1016/j.phrs.2019.01.019
63. Yano JM, Yu K, Donaldson GP, Shastri GG, Ann P, Ma L, et al. Indigenous bacteria from the gut microbiota regulate host serotonin biosynthesis. Cell. (2015) 161:264–76. doi: 10.1016/j.cell.2015.02.047
64. Zubcevic J, Richards EM, Yang T, Kim S, Sumners C, Pepine CJ, et al. Impaired autonomic nervous system-microbiome circuit in hypertension. Circ Res. (2019) 125:104–16. doi: 10.1161/CIRCRESAHA.119.313965
65. Richards EM, Li J, Stevens BR, Pepine CJ, Raizada MK. Gut microbiome and neuroinflammation in hypertension. Circ Res. (2022) 130:401–17. doi: 10.1161/CIRCRESAHA.121.319816
66. Kim C, Ho DH, Suk JE, You S, Michael S, Kang J, et al. Neuron-released oligomeric α-synuclein is an endogenous agonist of TLR2 for paracrine activation of microglia. Nat Commun. (2013) 4:1562. doi: 10.1038/ncomms2534
67. Sampson TR, Debelius JW, Thron T, Janssen S, Shastri GG, Ilhan ZE, et al. Gut microbiota regulate motor deficits and neuroinflammation in a model of Parkinson's disease. Cell. (2016) 167:1469–80.e12. doi: 10.1016/j.cell.2016.11.018
68. Wang X, Sun G, Feng T, Zhang J, Huang X, Wang T, et al. Sodium oligomannate therapeutically remodels gut microbiota and suppresses gut bacterial amino acids-shaped neuroinflammation to inhibit Alzheimer's disease progression. Cell Res. (2019) 29:787–803. doi: 10.1038/s41422-019-0216-x
69. Megur A, Baltriukiene D, Bukelskiene V, Burokas A. The microbiota-gut-brain axis and alzheimer's disease: neuroinflammation is to blame? Nutrients. (2020) 13:37. doi: 10.3390/nu13010037
70. Donertas Ayaz B, Zubcevic J. Gut microbiota and neuroinflammation in pathogenesis of hypertension: a potential role for hydrogen sulfide. Pharmacol Res. (2020) 153:104677. doi: 10.1016/j.phrs.2020.104677
71. Fung TC, Olson CA, Hsiao EY. Interactions between the microbiota, immune and nervous systems in health and disease. Nat Neurosci. (2017) 20:145–55. doi: 10.1038/nn.4476
72. Adesso S, Magnus T, Cuzzocrea S, Campolo M, Rissiek B, Paciello O, et al. Indoxyl sulfate affects glial function increasing oxidative stress and neuroinflammation in chronic kidney disease: interaction between astrocytes and microglia. Front Pharmacol. (2017) 8:370. doi: 10.3389/fphar.2017.00370
73. Wikoff WR, Anfora AT, Liu J, Schultz PG, Lesley SA, Peters EC, et al. Metabolomics analysis reveals large effects of gut microflora on mammalian blood metabolites. Proc Natl Acad Sci USA. (2009) 106:3698–703. doi: 10.1073/pnas.0812874106
74. Aronov PA, Luo FJ, Plummer NS, Quan Z, Holmes S, Hostetter TH, et al. Colonic contribution to uremic solutes. J Am Soc Nephrol. (2011) 22:1769–76. doi: 10.1681/ASN.2010121220
75. Antza C, Stabouli S, Kotsis V. Gut microbiota in kidney disease and hypertension. Pharmacol Res. (2018) 130:198–203. doi: 10.1016/j.phrs.2018.02.028
76. Bäckhed F. Meat-metabolizing bacteria in atherosclerosis. Nat Med. (2013) 19:533–4. doi: 10.1038/nm.3178
77. Tang WH, Wang Z, Kennedy DJ, Wu Y, Buffa JA, Agatisa-Boyle B, et al. Gut microbiota-dependent trimethylamine N-oxide (TMAO) pathway contributes to both development of renal insufficiency and mortality risk in chronic kidney disease. Circ Res. (2015) 116:448–55. doi: 10.1161/CIRCRESAHA.116.305360
78. Jiang S, Shui Y, Cui Y, Tang C, Wang X, Qiu X, et al. Gut microbiota dependent trimethylamine N-oxide aggravates angiotensin II-induced hypertension. Redox Biol. (2021) 46:102115. doi: 10.1016/j.redox.2021.102115
79. Chen K, Zheng X, Feng M, Li D, Zhang H. Gut Microbiota-dependent metabolite trimethylamine n-oxide contributes to cardiac dysfunction in western diet-induced obese mice. Front Physiol. (2017) 8:139. doi: 10.3389/fphys.2017.00139
80. Heianza Y, Ma W, Manson JE, Rexrode KM, Qi L. Gut microbiota metabolites and risk of major adverse cardiovascular disease events and death: a systematic review and meta-analysis of prospective studies. J Am Heart Assoc. (2017) 6:e004947. doi: 10.1161/JAHA.116.004947
81. Brunt VE, Gioscia-Ryan RA, Casso AG, VanDongen NS, Ziemba BP, Sapinsley ZJ, et al. Trimethylamine-N-oxide promotes age-related vascular oxidative stress and endothelial dysfunction in mice and healthy humans. Hypertension. (2020) 76:101–12. doi: 10.1161/HYPERTENSIONAHA.120.14759
82. Ufnal M, Jazwiec R, Dadlez M, Drapala A, Sikora M, Skrzypecki J. Trimethylamine-N-oxide: a carnitine-derived metabolite that prolongs the hypertensive effect of angiotensin II in rats. Can J Cardiol. (2014) 30:1700–5. doi: 10.1016/j.cjca.2014.09.010
83. Liu C, Kraja AT, Smith JA, Brody JA, Franceschini N, Bis JC, et al. Meta-analysis identifies common and rare variants influencing blood pressure and overlapping with metabolic trait loci. Nat Genet. (2016) 48:1162–70. doi: 10.1038/ng.3660
84. Jaworska K, Bielinska K, Gawrys-Kopczynska M, Ufnal M. TMA (trimethylamine), but not its oxide TMAO (trimethylamine-oxide), exerts haemodynamic effects: implications for interpretation of cardiovascular actions of gut microbiome. Cardiovasc Res. (2019) 115:1948–9. doi: 10.1093/cvr/cvz231
85. Li HB, Xu ML, Xu XD, Tang YY, Jiang HL, Li L, et al. Faecalibacterium prausnitzii attenuates CKD via butyrate-renal GPR43 axis. Circ Res. (2022) 131:e120–34. doi: 10.1161/CIRCRESAHA.122.320184
86. Avery EG, Bartolomaeus H, Maifeld A, Marko L, Wiig H, Wilck N, et al. The gut microbiome in hypertension: recent advances and future perspectives. Circ Res. (2021) 128:934–50. doi: 10.1161/CIRCRESAHA.121.318065
87. Gomez-Arango LF, Barrett HL, McIntyre HD, Callaway LK, Morrison M, Dekker Nitert M. Increased systolic and diastolic blood pressure is associated with altered gut microbiota composition and butyrate production in early pregnancy. Hypertension. (2016) 68:974–81. doi: 10.1161/HYPERTENSIONAHA.116.07910
88. Santisteban MM, Qi Y, Zubcevic J, Kim S, Yang T, Shenoy V, et al. Hypertension-linked pathophysiological alterations in the gut. Circ Res. (2017) 120:312–23. doi: 10.1161/CIRCRESAHA.116.309006
89. Natarajan N, Hori D, Flavahan S, Steppan J, Flavahan NA, Berkowitz DE, et al. Microbial short chain fatty acid metabolites lower blood pressure via endothelial G protein-coupled receptor 41. Physiol Genomics. (2016) 48:826–34. doi: 10.1152/physiolgenomics.00089.2016
90. Waghulde H, Cheng X, Galla S, Mell B, Cai J, Pruett-Miller SM, et al. Attenuation of microbiotal dysbiosis and hypertension in a CRISPR/Cas9 gene ablation rat model of GPER1. Hypertension. (2018) 72:1125–32. doi: 10.1161/HYPERTENSIONAHA.118.11175
91. Wang L, Zhu Q, Lu A, Liu X, Zhang L, Xu C, et al. Sodium butyrate suppresses angiotensin II-induced hypertension by inhibition of renal (pro)renin receptor and intrarenal renin-angiotensin system. J Hypertens. (2017) 35:1899–908. doi: 10.1097/HJH.0000000000001378
92. Pluznick JL, Protzko RJ, Gevorgyan H, Peterlin Z, Sipos A, Han J, et al. Olfactory receptor responding to gut microbiota-derived signals plays a role in renin secretion and blood pressure regulation. Proc Natl Acad Sci USA. (2013) 110:4410–5. doi: 10.1073/pnas.1215927110
93. Pluznick JL. Renal and cardiovascular sensory receptors and blood pressure regulation. Am J Physiol Renal Physiol. (2013) 305:F439–44. doi: 10.1152/ajprenal.00252.2013
94. Khalesi S, Sun J, Buys N, Jayasinghe R. Effect of probiotics on blood pressure: a systematic review and meta-analysis of randomized, controlled trials. Hypertension. (2014) 64:897–903. doi: 10.1161/HYPERTENSIONAHA.114.03469
95. Park J, Kim M, Kang SG, Jannasch AH, Cooper B, Patterson J, et al. Short-chain fatty acids induce both effector and regulatory T cells by suppression of histone deacetylases and regulation of the mTOR-S6K pathway. Mucosal Immunol. (2015) 8:80–93. doi: 10.1038/mi.2014.44
96. Cardinale JP, Sriramula S, Pariaut R, Guggilam A, Mariappan N, Elks CM, et al. HDAC inhibition attenuates inflammatory, hypertrophic, and hypertensive responses in spontaneously hypertensive rats. Hypertension. (2010) 56:437–44. doi: 10.1161/HYPERTENSIONAHA.110.154567
97. Säemann MD, Böhmig GA, Osterreicher CH, Burtscher H, Parolini O, Diakos C, et al. Anti-inflammatory effects of sodium butyrate on human monocytes: potent inhibition of IL-12 and up-regulation of IL-10 production. FASEB J. (2000) 14:2380–2. doi: 10.1096/fj.00-0359fje
98. Robles-Vera I, Toral M, de la Visitación N, Sánchez M, Gómez-Guzmán M, Romero M, et al. Probiotics prevent dysbiosis and the rise in blood pressure in genetic hypertension: role of short-chain fatty acids. Mol Nutr Food Res. (2020) 64:e1900616. doi: 10.1002/mnfr.201900616
99. Gusovsky F, Yasumoto T, Daly JW. Maitotoxin stimulates phosphoinositide breakdown in neuroblastoma hybrid NCB-20 cells. Cell Mol Neurobiol. (1987) 7:317–22. doi: 10.1007/BF00711308
100. Yang T, Magee KL, Colon-Perez LM, Larkin R, Liao YS, Balazic E, et al. Impaired butyrate absorption in the proximal colon, low serum butyrate and diminished central effects of butyrate on blood pressure in spontaneously hypertensive rats. Acta Physiol. (2019) 226:e13256. doi: 10.1111/apha.13256
101. Zhang RM, McNerney KP, Riek AE, Bernal-Mizrachi C. Immunity and hypertension. Acta Physiol. (2021) 231:e13487. doi: 10.1111/apha.13487
102. Mouton AJ, Li X, Hall ME, Hall JE. Obesity, hypertension, and cardiac dysfunction: novel roles of immunometabolism in macrophage activation and inflammation. Circ Res. (2020) 126:789–806. doi: 10.1161/CIRCRESAHA.119.312321
103. Dixon DL, Wohlford GFt, Abbate A. Inflammation and hypertension: causal or not? Hypertension. (2020) 75:297–8. doi: 10.1161/HYPERTENSIONAHA.119.14195
104. Jama HA, Beale A, Shihata WA, Marques FZ. The effect of diet on hypertensive pathology: is there a link via gut microbiota-driven immunometabolism? Cardiovasc Res. (2019) 115:1435–47. doi: 10.1093/cvr/cvz091
105. Furusawa Y, Obata Y, Fukuda S, Endo TA, Nakato G, Takahashi D, et al. Commensal microbe-derived butyrate induces the differentiation of colonic regulatory T cells. Nature. (2013) 504:446–50. doi: 10.1038/nature12721
106. Hooper LV, Littman DR, Macpherson AJ. Interactions between the microbiota and the immune system. Science. (2012) 336:1268–73. doi: 10.1126/science.1223490
107. Erny D, Hrabě de Angelis AL, Jaitin D, Wieghofer P, Staszewski O, David E, et al. Host microbiota constantly control maturation and function of microglia in the CNS. Nat Neurosci. (2015) 18:965–77. doi: 10.1038/nn.4030
108. Turnbaugh PJ, Ley RE, Mahowald MA, Magrini V, Mardis ER, Gordon JI. An obesity-associated gut microbiome with increased capacity for energy harvest. Nature. (2006) 444:1027–31. doi: 10.1038/nature05414
109. Madhur MS, Lob HE, McCann LA, Iwakura Y, Blinder Y, Guzik TJ, et al. Interleukin 17 promotes angiotensin II-induced hypertension and vascular dysfunction. Hypertension. (2010) 55:500–7. doi: 10.1161/HYPERTENSIONAHA.109.145094
110. Barhoumi T, Kasal DA, Li MW, Shbat L, Laurant P, Neves MF, et al. T regulatory lymphocytes prevent angiotensin II-induced hypertension and vascular injury. Hypertension. (2011) 57:469–76. doi: 10.1161/HYPERTENSIONAHA.110.162941
111. Natividad JM, Lamas B, Pham HP, Michel ML, Rainteau D, Bridonneau C, et al. Bilophila wadsworthia aggravates high fat diet induced metabolic dysfunctions in mice. Nat Commun. (2018) 9:2802. doi: 10.1038/s41467-018-05249-7
112. Wright SD, Ramos RA, Tobias PS, Ulevitch RJ, Mathison JC. CD14, a receptor for complexes of lipopolysaccharide (LPS) and LPS binding protein. Science. (1990) 249:1431–3. doi: 10.1126/science.1698311
113. Pevsner-Fischer M, Blacher E, Tatirovsky E, Ben-Dov IZ, Elinav E. The gut microbiome and hypertension. Curr Opin Nephrol Hypertens. (2017) 26:1–8. doi: 10.1097/MNH.0000000000000293
114. Grylls A, Seidler K, Neil J. Link between microbiota and hypertension: focus on LPS/TLR4 pathway in endothelial dysfunction and vascular inflammation, and therapeutic implication of probiotics. Biomed Pharmacother. (2021) 137:111334. doi: 10.1016/j.biopha.2021.111334
115. Larsson E, Tremaroli V, Lee YS, Koren O, Nookaew I, Fricker A, et al. Analysis of gut microbial regulation of host gene expression along the length of the gut and regulation of gut microbial ecology through MyD88. Gut. (2012) 61:1124–31. doi: 10.1136/gutjnl-2011-301104
116. Brown JM, Hazen SL. The gut microbial endocrine organ: bacterially derived signals driving cardiometabolic diseases. Annu Rev Med. (2015) 66:343–59. doi: 10.1146/annurev-med-060513-093205
117. Jamar G, Ribeiro DA, Pisani LP. High-fat or high-sugar diets as trigger inflammation in the microbiota-gut-brain axis. Crit Rev Food Sci Nutr. (2021) 61:836–54. doi: 10.1080/10408398.2020.1747046
118. Wang ML, Yu XJ, Li XG, Pang DZ, Su Q, Saahene RO, et al. Blockade of TLR4 Within the paraventricular nucleus attenuates blood pressure by regulating ROS and inflammatory cytokines in prehypertensive rats. Am J Hypertens. (2018) 31:1013–23. doi: 10.1093/ajh/hpy074
119. Subah Packer C. Estrogen protection, oxidized LDL, endothelial dysfunction and vasorelaxation in cardiovascular disease: new insights into a complex issue. Cardiovasc Res. (2007) 73:6–7. doi: 10.1016/j.cardiores.2006.11.013
120. Karbach SH, Schönfelder T, Brandão I, Wilms E, Hörmann N, Jäckel S, et al. Gut microbiota promote angiotensin ii-induced arterial hypertension and vascular dysfunction. J Am Heart Assoc. (2016) 5:e003698. doi: 10.1161/JAHA.116.003698
121. Santisteban MM, Ahmari N, Carvajal JM, Zingler MB, Qi Y, Kim S, et al. Involvement of bone marrow cells and neuroinflammation in hypertension. Circ Res. (2015) 117:178–91. doi: 10.1161/CIRCRESAHA.117.305853
122. Zubcevic J, Jun JY, Kim S, Perez PD, Afzal A, Shan Z, et al. Altered inflammatory response is associated with an impaired autonomic input to the bone marrow in the spontaneously hypertensive rat. Hypertension. (2014) 63:542–50. doi: 10.1161/HYPERTENSIONAHA.113.02722
123. Qi Y, Kim S, Richards EM, Raizada MK, Pepine CJ. Gut microbiota: potential for a unifying hypothesis for prevention and treatment of hypertension. Circ Res. (2017) 120:1724–6. doi: 10.1161/CIRCRESAHA.117.310734
124. Ganesh BP, Nelson JW, Eskew JR, Ganesan A, Ajami NJ, Petrosino JF, et al. Prebiotics, probiotics, and acetate supplementation prevent hypertension in a model of obstructive sleep apnea. Hypertension. (2018) 72:1141–50. doi: 10.1161/HYPERTENSIONAHA.118.11695
125. Liu TH, Tao WC, Liang QE, Tu WQ, Xiao Y, Chen LG. Gut microbiota-related evidence provides new insights into the association between activating transcription factor 4 and development of salt-induced hypertension in mice. Front Cell Dev Biol. (2020) 8:585995. doi: 10.3389/fcell.2020.585995
126. Gomez-Guzman M, Toral M, Romero M, Jimenez R, Galindo P, Sanchez M, et al. Antihypertensive effects of probiotics Lactobacillus strains in spontaneously hypertensive rats. Mol Nutr Food Res. (2015) 59:2326–36. doi: 10.1002/mnfr.201500290
127. Yuan L, Li Y, Chen M, Xue L, Wang J, Ding Y, et al. Antihypertensive activity of milk fermented by Lactiplantibacillus plantarum SR37–3 and SR61–2 in L-NAME-induced hypertensive rats. Foods. (2022) 11:2332. doi: 10.3390/foods11152332
128. Hadi A, Pourmasoumi M, Kazemi M, Najafgholizadeh A, Marx W. Efficacy of synbiotic interventions on blood pressure: a systematic review and meta-analysis of clinical trials. Crit Rev Food Sci Nutr. (2021) 62:5582–91. doi: 10.1080/10408398.2021.1888278
129. Gibson GR, Hutkins R, Sanders ME, Prescott SL, Reimer RA, Salminen SJ, et al. Expert consensus document: the International Scientific Association for Probiotics and Prebiotics (ISAPP) consensus statement on the definition and scope of prebiotics. Nat Rev Gastroenterol Hepatol. (2017) 14:491–502. doi: 10.1038/nrgastro.2017.75
130. Hsu CN, Hou CY, Chan JYH, Lee CT, Tain YL. Hypertension programmed by perinatal high-fat diet: effect of maternal gut microbiota-targeted therapy. Nutrients. (2019) 11:2908. doi: 10.3390/nu11122908
131. Swanson KS, Gibson GR, Hutkins R, Reimer RA, Reid G, Verbeke K, et al. The international scientific association for probiotics and prebiotics (ISAPP) consensus statement on the definition and scope of synbiotics. Nat Rev Gastroenterol Hepatol. (2020) 17:687–701. doi: 10.1038/s41575-020-0344-2
132. Bartolomaeus H, Avery EG, Bartolomaeus TUP, Kozhakhmetov S, Zhumadilov Z, Müller DN, et al. Blood pressure changes correlate with short-chain fatty acid production potential shifts under a synbiotic intervention. Cardiovasc Res. (2020) 116:1252–3. doi: 10.1093/cvr/cvaa083
Keywords: hypertension, intestinal microbiota, intestinal dysbiosis, SCFAs, inflammation
Citation: Sun D, Xiang H, Yan J and He L (2022) Intestinal microbiota: A promising therapeutic target for hypertension. Front. Cardiovasc. Med. 9:970036. doi: 10.3389/fcvm.2022.970036
Received: 15 June 2022; Accepted: 28 October 2022;
Published: 15 November 2022.
Edited by:
Elise Peery Gomez-Sanchez, University of Mississippi Medical Center, United StatesReviewed by:
Xiaoguang Liu, Guangzhou Sport University, ChinaCopyright © 2022 Sun, Xiang, Yan and He. This is an open-access article distributed under the terms of the Creative Commons Attribution License (CC BY). The use, distribution or reproduction in other forums is permitted, provided the original author(s) and the copyright owner(s) are credited and that the original publication in this journal is cited, in accordance with accepted academic practice. No use, distribution or reproduction is permitted which does not comply with these terms.
*Correspondence: Liqun He, bGlxdW5oZTA5MDJAMTYzLmNvbQ==; Jiangtao Yan, anR5YW5fdGpoQDE2My5jb20=
†These authors have contributed equally to this work and share first authorship
Disclaimer: All claims expressed in this article are solely those of the authors and do not necessarily represent those of their affiliated organizations, or those of the publisher, the editors and the reviewers. Any product that may be evaluated in this article or claim that may be made by its manufacturer is not guaranteed or endorsed by the publisher.
Research integrity at Frontiers
Learn more about the work of our research integrity team to safeguard the quality of each article we publish.