- 1Department of Life Technologies/Biotechnology, University of Turku, Turku, Finland
- 2Heart Center, Turku University Hospital and University of Turku, Turku, Finland
- 3Turku PET Centre, Turku University Hospital, University of Turku, Turku, Finland
- 4Minerva Foundation Institute for Medical Research, Biomedicum, Helsinki, Finland
- 5National Institute for Health and Welfare, Genomics and Biobank Unit, Biomedicum 2U, Helsinki, Finland
Objective: High-density lipoprotein (HDL) is a heterogeneous group of subpopulations differing in protein/lipid composition and in their anti-atherogenic function. There is a lack of assays that can target the functionality of HDL particles related to atherosclerosis. The objective of this study was to construct two-site apolipoprotein A-I (apoA-I) assays and to evaluate their clinical performance in patients with suspected obstructive coronary artery disease (CAD).
Approach and results: Direct two-site apoA-I assays (named 109–121 and 110–525) were developed to identify the presence of apoA-I in the HDL of patients with CAD using apoA-I antibodies as a single-chain variable fragment fused with alkaline phosphatase. ApoA-I109−121 and apoA-I110−525 were measured in 197 patients undergoing coronary computed tomography angiography (CTA) and myocardial positron emission tomography perfusion imaging due to suspected obstructive CAD. Among patients not using lipid-lowering medication (LLM, n = 125), the level of apoA-I110−525 was higher in the presence than in the absence of coronary atherosclerosis [21.88 (15.89–27.44) mg/dl vs. 17.66 (13.38–24.48) mg/dl, P = 0.01)], whereas there was no difference in apoA-I109−121, HDL cholesterol, and apoA-I determined using a polyclonal apoA-I antibody. The levels of apoA-I109−121 and apoA-I110−525 were similar in the presence or absence of obstructive CAD. Among patients not using LLM, apoA-I110−525 adjusted for age and sex identified individuals with coronary atherosclerosis with a similar accuracy to traditional risk factors [area under the curve [AUC] (95% CI): 0.75(0.66–0.84) 0.71 (0.62–0.81)]. However, a combination of apoA-I110−525 with risk factors did not improve the accuracy [AUC (95% CI): 0.73 (0.64–0.82)].
Conclusion: Direct two-site apoA-I assays recognizing heterogeneity in reactivity with apoA-I could provide a potential approach to identify individuals at a risk of coronary atherosclerosis. However, their clinical value remains to be studied in larger cohorts.
Introduction
Atherosclerotic cardiovascular diseases (ASCVDs) remain as major causes of morbidity and mortality throughout the world (1). Plasma high-density lipoprotein cholesterol (HDL-C) levels are inversely associated with the risk of ASCVD (2–4). The major anti-atherogenic functions of HDL include participation in reverse cholesterol transport, and anti-inflammatory and antioxidation processes (5).
The molecular structure of HDL is complex consisting of several lipid classes and up to 85 proteins (6). In circulation, HDL consists of numerous distinct particle subpopulations varying in terms of size, charge, shape, and density (5, 7). Apolipoprotein A-I (apoA-I) is the most abundant structural protein in HDL particles constituting 60–70% of the total protein mass, with the exception of clinical conditions that harbor apoA-I genetic defects (8, 9). HDL participates in anti-atherogenic functions via its protein and/or lipid components (5, 10).
An emerging concept emphasizes that the functionality of HDL over its cholesterol content is reflected in the total circulating concentration of HDL. This concept is based on the failure of pharmacological interventions increasing HDL-C to reduce ASCVD events (11–13). Mendelian randomization studies also show no connection between HDL-C and the risk of coronary artery disease (CAD) or myocardial infarction (MI) (14).
Reportedly, the structural features of apoA-I may define the functional properties of HDL related to atherosclerosis and could, therefore, be explored as risk markers (10, 15). For example, plasma myeloperoxidase (MPO)-modified apoA-I (15) and lysine glycated apoA-I (16) have been shown to have an altered conformation (16). This can generate dysfunctional and pro-atherogenic apoA-I and HDL due to an impaired anti-inflammatory function and a reverse cholesterol transport function; both of which could partly account for an increased risk of CVD (15, 16).
In addition, there is a lack of monoclonal antibodies (mAbs) that could specifically target HDL functionality and could be used to improve the risk estimation of ASCVD. The production of mAbs through conventional animal immunization against a complex molecule like HDL is challenging. The phage display-based universal recombinant antibody libraries provide a platform where antibodies can be produced against almost any target (17). Recently, Huang et al. (15) demonstrated the production of an antibody against dysfunctional MPO-modified apoA-I using phage display.
We have previously developed phage-based two-site apoA-I assays (assay 109–121 and assay 110–525) by using phage-displayed single-chain variable fragment (scFv) antibodies (18) isolated against HDL derived from patients with CAD (19). However, the phage-based two-site assay design (18) was complex due to the complicated structure of the scFv antibodies (fused to a large phage particle) and the need for an additional phage detecting antibody. The aim of this study was to construct simpler forms of the two-site apoA-I assays and evaluate their clinical performance in patients undergoing coronary computed tomography angiography (CTA) and positron emission tomography (PET) myocardial perfusion imaging due to a suspected obstructive CAD. Coronary CTA is a non-invasive imaging modality that accurately detects coronary atherosclerosis and is powerful in ruling out obstructive CAD (20). In turn, PET myocardial perfusion imaging is a functional imaging modality detecting myocardial ischemia caused by obstructive CAD (20, 21). A combination of coronary CTA and PET perfusion imaging provides a comprehensive characterization of CAD, because both the extent of non-obstructive atherosclerosis and the presence of significant obstructive lesions can be evaluated (22). In this study, direct two-site apoA-I assays (assays 109–121 and 110–525) were designed using different single-chain (sc) apoA-I antibodies (sc 109, sc 121, sc 110, and sc 525) as scFv fused to bacterial alkaline phosphatase (scFv-APs). The antibodies were biotinylated or directly labeled and implemented in assay development to capture and identify the apoA-I on HDL by binding at two different sites. Therefore, these assays are referred to as direct two-site apoA-I assays.
Materials and methods
Clinical samples
We prospectively recruited 252 symptomatic patients referred to Turku University Hospital for coronary CTA due to suspected obstructive CAD from March 2016 to January 2019 (Figure 1). Blood samples were drawn from these patients before imaging and serum samples were prepared according to standard protocol. All the samples were stored at −70°C prior to use. The study protocols for the collection of blood samples were approved by the Local Ethics Committee of the Hospital District of Southwest Finland, and the study was conducted in accordance with the Declaration of Helsinki as revised in 2013. All samples were collected after the participants gave their informed consent. A total of 55 patients were excluded from this study due to a hemolyzed blood sample (N = 21), missing information on the use of lipid-lowering medication (LLM; N = 16), a failed or non-diagnostic coronary CTA (N = 11), or indications of coronary CTA other than an evaluation of a suspected CAD (N = 7). Hence, 197 samples remained for clinical evaluation of the direct two-site apoA-I assays.
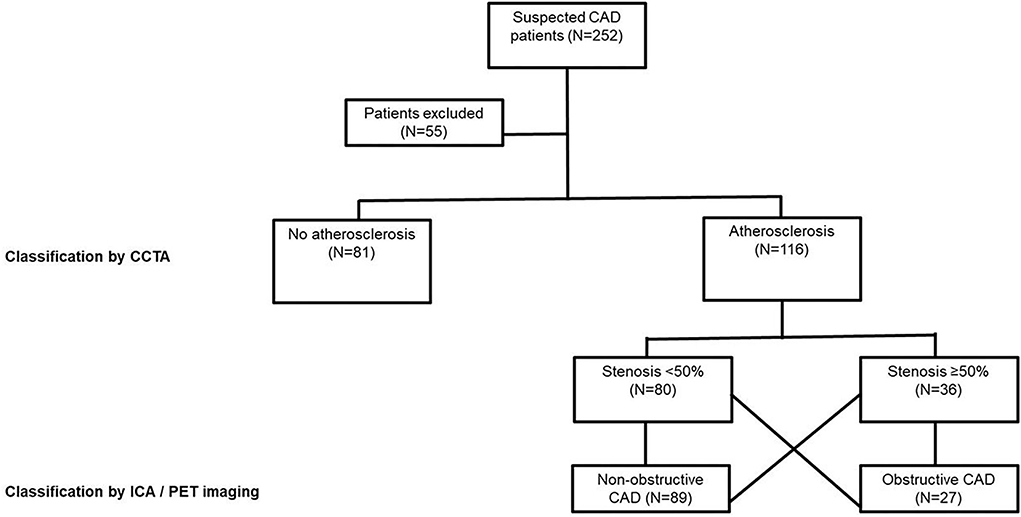
Figure 1. Study population. Obstructive CAD is defined as a coronary atherosclerosis associated with an abnormal stress myocardial blood flow (MBF) on a CCTA or >70% coronary stenosis on an ICA. N, number of individuals; CAD, coronary artery disease; CCTA, coronary computed tomography angiography (CCTA); PET, positron emission tomography; ICA, invasive coronary angiography.
The clinical characteristics of the patients and findings of invasive coronary angiography (ICA) were collected from electronic medical records. The Framingham Risk Score (FRS) was utilized in the calculation of a 10-year coronary heart disease (CHD) risk (23). As predictors, the FRS uses age, diabetes and smoking status, blood pressure, total cholesterol (TC), HDL-C, and low-density lipoprotein cholesterol (LDL-C).
Serum samples of randomly selected and routinely analyzed non-cardiac patients from Helsinki University Hospital (Finland) were also collected (N = 200). Some of these samples were used as assay controls during the optimization and validation of the direct two-site apoA-I assays.
Coronary CTA and PET image acquisition
Coronary CTA was performed with a 128-row hybrid PET-CT scanner (GE Discovery D690 or MI, General Electric Medical Systems, Waukesha, WI, USA), as previously described (24, 25). Before the coronary CTA, metoprolol (0–30 mg) was given intravenously to achieve a target heart rate of <60 beats/min, and an isosorbide dinitrate aerosol (1.25 mg) was administered. A coronary artery calcium scan was performed before the coronary CTA. The coronary CTA was performed using an intravenously administered low-osmolality iodine contrast agent (60–80 ml; 320–400 mg iodine/ml; injection velocity of 4–5 ml/s) followed by a saline flush. A prospectively triggered acquisition was applied whenever feasible.
As previously described (25), in the routine clinical practice at Turku University Hospital, patients initially undergo a coronary CTA using a hybrid PET-CT scanner. Immediately after the coronary CTA, the attending physician makes an initial assessment of the CTA scan to decide whether a PET myocardial perfusion imaging study is needed. Briefly, if the obstructive CAD is excluded by coronary CTA, no further imaging procedure is performed; however, in the presence of a suspected obstructive coronary lesion on the CTA (≥50% diameter stenosis), a PET myocardial perfusion imaging is performed. The PET myocardial perfusion imaging uses a 15O-water tracer during adenosine stress to assess the hemodynamic significance of the stenosis. In our cohort, 47 patients underwent a PET perfusion study, whereas eight patients with an obstructive CAD on the CTA were directly referred to an ICA due either to a contra-indication to the PET perfusion study (N = 5) or to logistic reasons (N = 3).
Scans were performed after an overnight fast. Patients were instructed to abstain from alcohol and caffeine for 24 h before the PET myocardial perfusion study. The adenosine infusion was started 2 min before the stress PET scan and continued at a rate of 140 μg/kg/min until the scan was complete. 15O-water (Radiowater Generator, Hidex Oy, Turku, Finland) was injected as an intravenous bolus (injected activity of 500–600 MBq) over 15 s, and a dynamic PET acquisition was performed (14 × 5 s, 3 × 10 s, 3 × 20 s, and 4 × 30 s).
Image analysis and interpretation
The coronary CTA images were analyzed according to the 17-segment vessel system using the GE ADW 4.4 Workstation software (General Electric Medical Systems, Waukesha, Wisconsin). The presence of coronary atherosclerosis and the diameter of the stenosis were evaluated in all segments. An Agatston coronary artery calcium score was measured in the non-enhanced scan.
The PET data were analyzed quantitatively using the Carimas software (developed at Turku PET Centre, Turku, Finland) (21, 26). Absolute stress myocardial blood flow (MBF) was quantified (in ml/g/min) individually for each of the standard 17 myocardial segments. Stress MBF of <2.4 ml/g/min was considered abnormally low, reflecting hemodynamically significant obstructive CAD based on our previous validation study (21). The analysis was performed by an experienced physician and recorded in a standardized reporting system.
In this study, obstructive CAD was defined as the presence of atherosclerosis on coronary CTA accompanied by an abnormally stressed MBF or, alternatively, the presence of > 70% stenosis on the ICA. The rest of the patients were classified as having a non-obstructive CAD or normal coronary arteries (no atherosclerosis) based on the CTA findings.
Reagents
The total HDL (= HDL2 + HDL3 subclasses) from the serum of a healthy individual was isolated, as described earlier (19). Affinity-purified scFv-APs (sc 109 and sc 110) were biotinylated with 20-fold molar excess of EZ-Link-NHS-PEG4-Biotin (Thermo Scientific, USA) according to the manufacturer's instructions. Europium (Eu+3) chelate of tetra-tert-butyl 2,2′,2″,2‴-[((((4-((4-aminophenyl)ethynyl)-pyridine-2,6-diyl)bis(methylene))bis((2-(tert-butoxy)-2-oxoethyl) azanediyl))bis(ethane-2,1-diyl))bis(azanetriyl)]-tetraacetate (referred to as Eu+3-WN) was synthesized according to Wang et al. (27). Affinity-purified scFv-APs (sc 121 and sc 525) were labeled with 25- and 50-fold molar excess of Eu+3-WN, respectively, in phosphate-buffered saline (PBS; pH 7.4) at 4°C, overnight with shaking using a modified protocol of Eriksson et al. (28). Wash buffer, streptavidin-coated plates (low fluorescence and bovine serum albumin (BSA) blocked), and europium fluorescence intensifier (EFI) were purchased from Kaivogen, Finland. The HDL assay buffer contained 50 mM Tris–hydrochloride, pH 7.75, 150 mM sodium chloride, 0.05% sodium azide, 20 μM diethylenetriaminepentaacetic acid (DTPA), 20 μg/ml cherry red, 0.05% bovine γ-globulin, and 2.3% or 4% BSA.
Measurement of biochemical parameters in clinical samples
In the samples of the CAD-suspected individuals, the biochemical parameters were measured including HDL-C, apoA-I, phospholipids (PLs), triglycerides (TG), TC, PL transfer protein (PLTP) activity, paraoxonase activity (PON-I), and serum-free glycerol. TG and TC were measured using enzymatic methods (Roche Diagnostics, Germany). HDL-C was analyzed using a phosphotungstate-MgCl2-precipitation method, whereby apoB-containing lipoproteins were precipitated and HDL-C could be analyzed from the supernatant after light centrifugation (29). ApoA-I was measured using a polyclonal anti-apoA-I-based ELISA instrument, as described earlier (30). Briefly, the wells were coated with a polyclonal rabbit antibody against human apoA-I, and the bound protein was detected with a horseradish peroxidase (HRP)-conjugated rabbit anti-human apoA-I immunoglobulin G (IgG) (30). LDL-C was calculated using the Friedewald formula, where LDL-C = TC-(TG/2.2)-HDL-C. PLTP activity was determined with a radiometric method as described by Jauhiainen et al. (31). PON-1 activity was measured with a chromogenic method (32). The serum concentration of free glycerol was determined by a commercial enzymatic colorimetric assay (free glycerol FS; DiaSys, Diagnostic Systems GmbH, Holzheim, Germany). Serum PL [choline-containing PL, i.e., phosphatidylcholine (PC), lysoPC, and sphingomyelin] was analyzed using the Phospholipids B Kit (Wako Chemicals, Osaka, Japan) or Pureauto S PL-Kit (Daiichi Pure Chemicals, Tokyo, Japan).
ScFv-APs antibodies for direct two-site apoA-I assays
Two distinct pairs of recombinant scFv apoA-I antibodies (sc 109–121 and sc 110–525), which were previously tested with an optimized phage-based two-site apoA-I immunoassay and had shown diagnostic/predictive value for MI and mortality (18), were used for the development of the direct two-site apoA-I assay. The assay utilized recombinant apoA-I antibodies as scFv-AP fusion proteins, which were produced and purified as described previously (19). Briefly, DNA encoded apoA-I scFv antibodies were inserted at the Sfi-I restriction site of the chloramphenicol-resistant vector, then expressed into vector pLK06H, and purified by Ni-NTA chromatography. The purified scFv-APs were stored at 4°C in PBS.
Final optimized direct two-site apoA-I-assays
The final optimized assays used the scFv-AP apoA-I antibody pairs 109–121 and 110–525. A schematic representation of the principle of these direct two-site apoA-I assays is shown in Figure 2A. Each assay used two different apoA-I antibodies, namely, the capture antibodies (scFv-AP) sc 109 and sc 110 and the corresponding Eu+3-WN labeled detection antibodies (scFv-AP) sc 121 and sc 525, respectively. Assay 109–121 was done with an HDL-assay buffer supplemented with 4% BSA and assay 110–525 was performed with an HDL-assay buffer containing 2.3% BSA on streptavidin plates. The total HDL (16–2,564 ng/ml as HDL total protein), i.e., HDL2 + HDL3 subpopulations isolated from the serum of a healthy individual was used as an assay calibrator or standard.
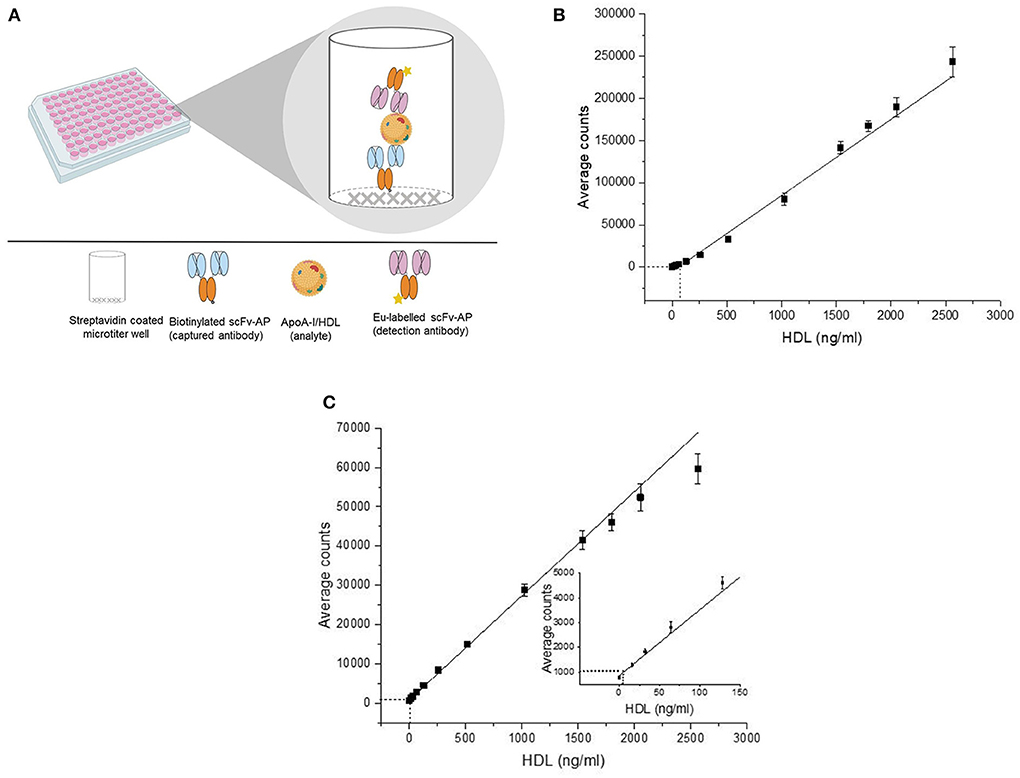
Figure 2. Schematic representation of the principle of the direct two-site apoA-I immunoassay (A) and their standard curve (B,C). The detection of the direct two-site apoA-I immunoassays is based on time-resolved fluorescence of europium attached to single-chain variable fragment-alkaline phosphatase fusion protein (scFv-AP) (A). In the standard curve (B,C), the X-axis represents the concentration of the standard (HDL) and the Y-axis represents the average of the counts obtained from the replicates of the standard. The error bars represent the standard deviation (SD) of the average counts. The curve was fitted with a linear fitting function. The analytical sensitivity (background + 5*SD) of the assay 109–121 (63.8 ng/ml) (B) and assay 110–525 (6.8 ng/ml) (C) is illustrated as dash-line (———). ApoA-I, apolipoprotein A-I; HDL, high-density lipoprotein; Eu, europium.
Biotinylated-captured antibodies sc 109 and sc 110 (100 ng/50 μl/well) were immobilized on separate streptavidin-coated microtiter plates and incubated for 1 h at room temperature (RT) with shaking followed by two washes. For both assays, a calibrator and sample were added in four replicates and incubated for 1 h at RT with shaking. After two washes, the Eu+3-WN labeled detection antibodies sc 121 (100 ng/50 μl/well) and sc 525 (200 ng/50 μl/well) were added to the respective assays and incubated for 1 h at RT with shaking. Finally, the wells were washed four times. EFI (200 μl/well) was added to the well, incubated for 10 min with shaking, and the TRF of the europium was measured with a Victor plate reader (PerkinElmer, USA).
Assay performance and clinical evaluation
The analytical sensitivity of the direct two-site apoA-I assays was evaluated by running eleven levels of the standard (HDL protein range: 16–2,564 ng/ml) and the blanks (HDL = 0 ng/ml) through several replicates, i.e., N = 15 for the blanks and N = 12 per level for the standard in assays 109–121, and N = 20 for the blanks and N = 16 per level for the standard in assay 110–525. The analytical sensitivity was calculated by adding five times the standard deviation (SD) to the average count for the blank sample (blank + 5*SD) and performing a linear fitting between the concentration of the calibrator and the corresponding average counts. Within 3 days, the inter-assay variation of the assays was tested with eight routinely analyzed serum samples (HDL-C ranging from 32.5 to 49.5 mg/dl, i.e., 0.84–1.28 mmol/L), using four replicates (N) per sample, i.e., N = 12 per sample. The linearity of the assay was assessed by diluting the four serum samples (HDL-C ranging from 38.67 to 70.38 mg/dl, i.e., 1.0–1.8 mmol/L) from 62.5- to 1,000-fold, using three replicates per sample (N = 3).
The clinical performance of the optimized direct two-site apoA-I immunoassays was evaluated using serum samples from the cohort of patients with suspected obstructive CAD (N = 197). Comparisons were done between the following groups (Figure 1) that were categorized based on imaging findings: (i) patients without any coronary atherosclerosis (normal) vs. those with atherosclerosis (i.e., either non-obstructive or obstructive CAD), (ii) patients without obstructive CAD (i.e., either normal coronary arteries or non-obstructive CAD) vs. those with obstructive CAD, and (iii) patients without any coronary atherosclerosis (normal) vs. those with non-obstructive CAD vs. those with obstructive CAD.
Statistical analysis
The statistical analysis was done using Origin 2015 (OriginLab Corporation, Wellesley Hills, USA), JMP Pro 13 (SAS Institute Inc., Cary, NC, USA), and IBM® SPSS® Statistics version 25 (IBM Corp., Armonk, NY). The normality distribution was checked with a Shapiro–Wilk test and a Q-Q plot. Categorical variables are presented as numbers (percentage) and analyzed by a chi-squared test or a Fisher's exact test, as appropriate. Continuous variables are presented as a median (25th percentile; 75th percentile). The concentration of the apoA-I obtained from the direct two-site apoA-I assays and the concentration of biochemical parameters were used after a natural log transformation was performed in order to enable parametric statistical testing.
A suitable Pearson's or Spearman's correlation was used to test the correlation of apoA-I110−525 or apoA-I109−121 levels with age, with the Agatston coronary calcium score and with conventional apoA-I ELISA (done by using polyclonal anti-apoA-I antibody). Biochemical parameters and the FRS for the CHD were compared between the two groups (no atherosclerosis vs. atherosclerosis and obstructive CAD vs. non-obstructive atherosclerosis) by using an independent t-test. For a comparison between the three groups (no atherosclerosis, non-obstructive CAD, and obstructive CAD), a one-way ANOVA was used followed, if required, by a multiple pair comparison using a Tukey's test.
A logistic regression analysis was performed to estimate the odds ratios (ORs) and 95% confidence intervals (CIs), with atherosclerosis and obstructive CAD as the dependent variables, and the apoA-I110−525 or apoA-I109−121 levels combined with age and gender (Model 1) or age, gender, smoking, diabetes, hypertension, LDL-C, and HDL-C (Model 2) as the independent variables. The apoA-I concentrations obtained from the two-site apoA-I assays were used as a categorical variable (below and above the median). An analysis of the area under the receiving-operating characteristic (ROC) curve was performed to test the diagnostic clinical value of the apoA-I110−525 or apoA-I109−121 levels and the FRS CHD. The ROC curves were compared using the DeLong's method. The results were analyzed for the whole cohort as well as separately in patients using and not using LLM. All the test results were considered statistically significant for P-value of < 0.05.
Results
Assay validation
The standard curves of the direct two-site apoA-I assays where the X-axis represents the HDL protein concentration (standard or calibrator) and the Y-axis represents the average counts (signal) of the standard are shown in Figures 2B,C, respectively. The measurement range of assay 109–121 and assay 110–525 was decided to be between an HDL (protein) concentration of 128–2,564 ng/ml (r2 = 0.98) and 6.8–2,564 ng/ml (r2 = 0.99). The inter-assay variation within 3 days with assay 109–121 was between 2–9% and 4–16% in the standards and samples; the inter-assay variation with apoA-I assay 110-525 in the standards and samples was 3–22% and 7–26%, respectively (Supplementary Table S1). The intra-assay variation was between 0.7–6.6% and 2.4–18.4% with assays 109–121 and 110–525, respectively. Sample dilution of 62.5- to 1,000-fold (r2 = 0.99) and 250- to 1,000-fold (r2 = 0.99) was suitable for assays 109–121 and 110–525, respectively.
Clinical evaluation of assays
Characteristics of the patients
The median age of the 197 patients was 63 years and 43% were males. The most common symptom was atypical chest pain (52%). The baseline characteristics of the patients are displayed in Table 1. At the time of enrollment in the study, 72 (36.5%) patients were taking LLM. The patients taking LLM were significantly older, had more risk factors for cardiovascular disease, and were more often taking antithrombotic medication than patients without LLM. Individuals who were not using LLM had significantly higher levels of serum TC, PL, and LDL-C than those who were using LLM; however, the concentration of HDL-C, apoA-I (determined with ELISA), and other biochemical parameters was similar (Table 1).
Biochemical parameters and FRS were compared between patients with and without coronary atherosclerosis (Table 2A) and between patients with and without obstructive CAD (Table 2B). Among the biochemical parameters, PL (in all patients altogether, P = 0.007) and PON-I (in patients not using LLM, P = 0.04) were higher in patients without any coronary atherosclerosis than in those with atherosclerosis (Table 2A). In the whole cohort, TC (P = 0.01) and LDL-C (P = 0.01) were higher in patients without obstructive CAD than in those with obstructive CAD (Table 2B). Among individuals not using LLM and using LLM, HDL-C (P = 0.03) and PLTP (P = 0.04) were significantly lower in patients with obstructive CAD than in those without obstructive CAD (Table 2B). Finally, FRS was significantly higher (P < 0.0001) in patients with coronary atherosclerosis than in those with normal coronary arteries (Table 2A), but there was no significant difference between patients with and without obstructive CAD (Table 2B).
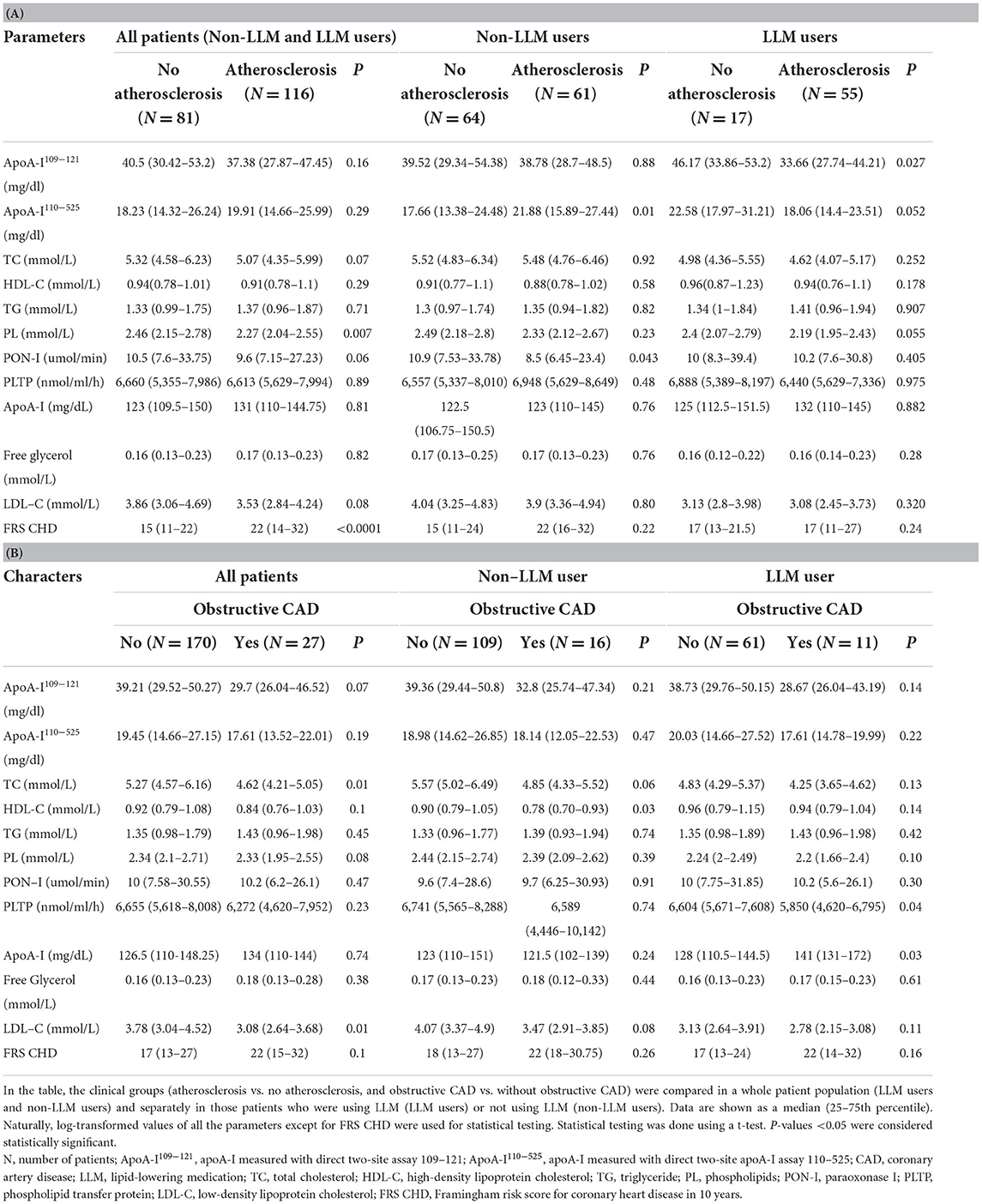
Table 2. Comparison of the direct two-site apoA-I assays (assay 109–121 and assay 110–525), biochemical parameters, and FRS CHD between patients with and without atherosclerosis (A), and with and without obstructive CAD (B).
ApoA-I110−525 (apoA-I measured by the direct two-site apoA-I assay 110–525) did not show any significant correlation (Pearson's r) with a conventional ELISA-based apoA-I assay (whole patient population: r = 0.05, P = 0.41; patients using LLM: r = −0.12, P = 0.29; patients not using LLM: r = 0.14, P = 0.09; Supplementary Figures S1A–C). In contrast, apoA-I109−121 (apoA-I measured by the direct two-site apoA-I assay 109–121) showed a weak positive correlation (whole patient population: r = 0.28, P < 0.001; patients using LLM: r = 0.17, P = 0.14; patients not using LLM: r = 0.33, P < 0.0001; Supplementary Figures S1D–F). ApoA-I109−121 and apoA-I110−525 showed a positive correlation (Spearman's r) with each other (whole patient population: rs = 0.37, P < 0.001; patients not using LLM: rs = 0.39, P < 0.001; patients using LLM: rs = 0.332, P = 0.0042; Supplementary Figures S1G–I).
Direct two-site apoA-I assays and coronary atherosclerosis
Compared to patients without coronary atherosclerosis, the level of apoA-I110−525 was higher in patients with coronary atherosclerosis among individuals not taking LLM (P = 0.01, Table 2A). However, in the whole cohort, the level of apoA-I110−525 was similar in patients with and without coronary atherosclerosis (P = 0.29), since apoA-I110−525 tended to be lower in the presence of atherosclerosis with a borderline significance (P = 0.05) among patients taking LLM.
The level of apoA-I109−121 was significantly higher in patients without any coronary atherosclerosis than in those with coronary atherosclerosis among LLM users (P = 0.03) but not in patients not taking LLM (P = 0.88) or in the whole cohort (P = 0.16; Table 2A).
Neither of the two direct apoA-I assays showed a correlation (P > 0.05) with the extent of coronary atherosclerosis measured by the coronary calcium score.
Direct two-site apoA-I assays and obstructive CAD
The levels of apoA-I109−121 were not significantly different between the patients with and without obstructive CAD, in the whole patient population (P = 0.07) and separately in LLM users (P = 0.14) and non-LLM users (P = 0.21; refer to Table 2B). Similarly, the levels of apoA-I110−525 were also not significantly different between these patients, in the whole patient population (P = 0.19) and separately in LLM users (P = 0.22) and non-LLM users (P = 0.47; refer to Table 2B).
We further compared the concentration of apoA-I109−121 and apoA-I110−525 between patients with obstructive CAD, with non-obstructive CAD, or without any coronary atherosclerosis, in the whole patient cohort (Supplementary Table S2A) and separately in patients using LLM and not using LLM (Supplementary Table S2B). Among individuals who were not using LLM, the level of apoA-I110−525 was higher in patients with non-obstructive CAD than in those patients without atherosclerosis (P = 0.01) (Supplementary Table S2B). However, there was no difference in the level of apoA-I110−525 between patients with non-obstructive and obstructive CAD (P = 0.16) or obstructive CAD and without atherosclerosis (P = 0.99). ApoA-I109−121 (all patients altogether, P = 0.14; patients not using LLM, P = 0.44; patients using LLM, P = 0.06) could not discriminate between any of these groups (Supplementary Table S2).
Direct two-site apoA-I assays and cardiovascular risk factors
The median (25–75th percentile) apoA-I109−121 level was higher in females than in males [females: 42.07 (33.62–56.6) mg/dl, males: 31.6 (25.18–42.63) mg/dl; P < 0.001; Supplementary Figure S2A]. Similarly, median apoA-I110−525 was significantly higher in females than in males [females: 21.04 (16.36–28.82) mg/dl, males: 17.25 (12.84–22.40) mg/dl; P < 0.001; Supplementary Figure S2B].
We found a weak positive correlation between age and apoA-I109−121 levels in the whole patient population (r = 0.22, P = 0.002; Figure S3A) that was driven by a positive correlation among patients not using LLM (r = 0.22, P = 0.002; Supplementary Figure S3C); however, there was no correlation among patients using LLM (r = 0.11, P = 0.31; Supplementary Figure S3B). There was also a positive correlation between age and apoA-I110−525 among patients not using LLM (r = 0.20, P = 0.02; Supplementary Figure S3F), but no correlation was found in the whole patient population (r = 0.11, P = 0.10; Supplementary Figure S3D) or in patients using LLM (r = −0.04, P = 0.70; Supplementary Figure S3E).
Association between the direct two-site apoA-I assays and both the presence of atherosclerosis (Table 3A; Supplementary Table S3A) and obstructive CAD (Table 3B; Supplementary Table S3B) was determined in the overall study population and separately in individuals using LLM and not using LLM using models. A simple model, referred to as Model 1, included age and gender and a complex model, referred to as Model 2, included the variables in Model 1 and known risk factors for CAD including smoking, hypertension, diabetes, as well as levels of HDL-C and LDL-C. Only the simpler model (Model 1) was evaluated when estimating the association with obstructive CAD due to the small number of patients with obstructive CAD.
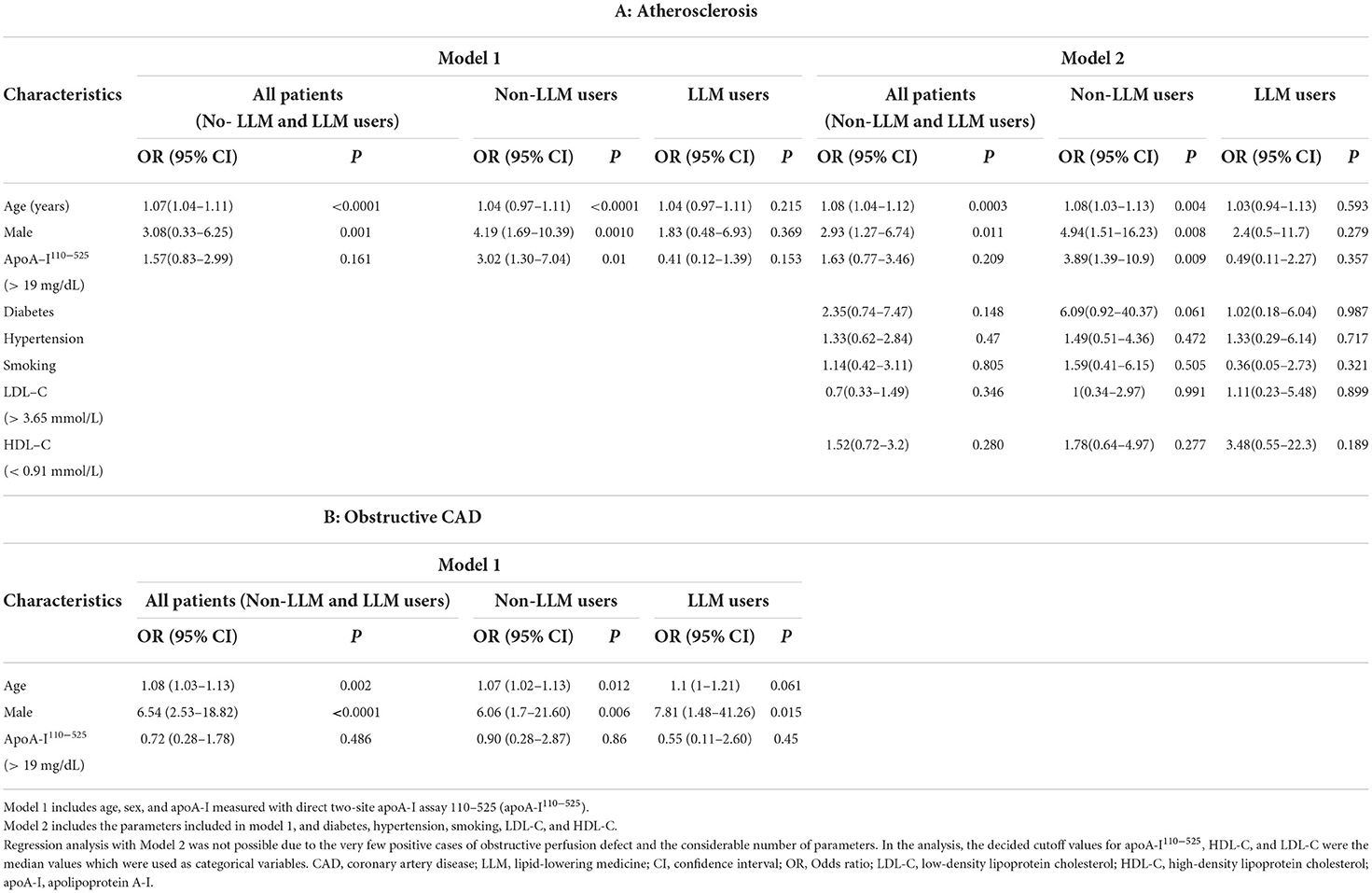
Table 3. Multivariate logistic regression analysis of the direct two-site apoA-I assay 110–525 for the presence of atherosclerosis (A) and obstructive CAD (B) in all the patients and separately for patients taking LLM (LLM users) and not taking LLM (non-LLM users).
There were no significant associations between apoA-I109−121 and the presence of coronary atherosclerosis (Supplementary Table S3A) or obstructive CAD (Supplementary Table S3B) when combined with age and sex (Model 1) or age, sex, and risk factors for CAD (Model 2) in the whole study cohort. However, a high level of apoA-I110−525 (>19 mg/dl, i.e., higher than the median value) was significantly associated with the presence of any form of coronary atherosclerosis when combined with age and sex [Model 1, OR (95% CI): 3.02 (1.30–7.04); P = 0.01] as well as age, sex, and risk factors for CAD [Model 2, OR (95% CI): 3.89 (1.39–10.9); P = 0.009] in patients not using LLM (Table 3A). There was no significant association between obstructive CAD and apoA-I110−525 (Table 3B).
ApoA-I110−525 combined with age and sex [ApoA-I110−525; Model 1] provided a slightly better but not statistically significant prediction than FRS [AUC (95% CI): 0.72 (0.65–0.80) vs. 0.64 (0.57–0.72)] in the whole population, [0.64 (0.50–0.78) vs. 0.55 (0.40–0.69)] in LLM users, and [0.75 (0.66–0.84) vs. 0.71 (0.62–0.81)] in patients not using LLM (P-values on comparison between ROCs > 0.05) (Figure 3). ApoA-I110−525 combined with FRS predicted the presence of coronary atherosclerosis with a similar accuracy to FRS alone [AUC (95% CI): 0.65 (0.57–0.72) vs. 0.64 (0.57–0.72)] in the whole population, [0.60 (0.46–0.75) vs. 0.55 (0.41–0.69)] in LLM users, and [0.73 (0.64–0.82) vs. 0.71 (0.62–0.81)] in patients not using LLM (P-values on comparison between ROCs > 0.05) (Figure 3).
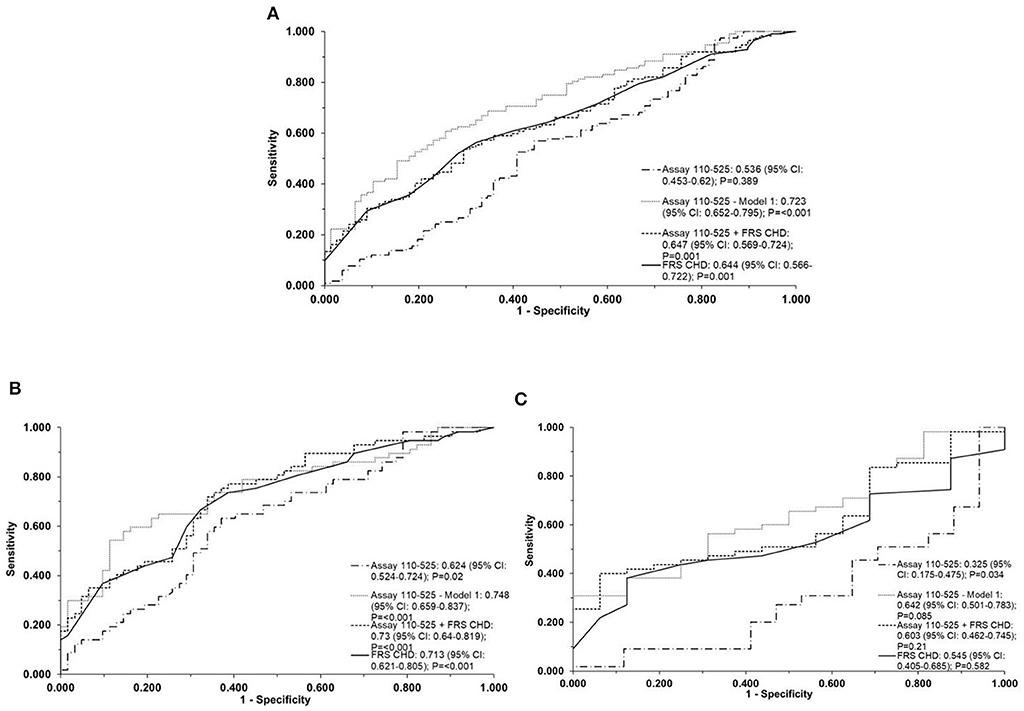
Figure 3. Direct two-site apoA-I assay 110-525 as predictors of coronary atherosclerosis. The ROC curve of the direct two-site apoA-I assay 110-525, assay 110-525 combined with age and sex (Model 1), direct two-site apoA-I assay 110-525 combined with a 10-year coronary heart disease risk using the Framingham Risk Score (FRS CHD), and the FRS CHD alone for detection of coronary atherosclerosis in the whole population (A), in patients without LLM (B) and in patients on LLM (C). The area under the curve (AUC) (95% CI) and the P-values are represented in the bottom right-hand corner of the figure. The assay 110-525, Model-1, used age and gender as the covariates. The FRS CHD estimation included age, gender, smoking, hypertension, diabetes, HDL-C, and LDL-C. ROC, receiver operator characteristic; LLM, lipid-lowering medication; HDL-C, high-density lipoprotein cholesterol; LDL-C, low-density lipoprotein cholesterol.
An ROC curve was not drawn for the prediction of atherosclerosis using the direct two-site apoA-I assay 109–121 since the data from this assay were not significantly associated with atherosclerosis in the logistic regression analysis (Supplementary Table S3A).
Discussion
This study presents the development and clinical evaluation of time-resolved fluorescence (TRF) based on direct two-site apoA-I immunoassays (assays 109–121 and 110–525); the assays were established using recombinant apoA-I antibodies previously generated against the intact isolated HDL particles from plasma of patients with CAD (19). The apoA-I antibodies (sc 109, sc 121, sc 110, and sc 525) used in these direct two-site apoA-I assays were scFv fragments fused to a bacterial alkaline phosphatase (scFv-APs). In the assays, total HDL (= HDL2 and HDL3 subpopulation from normolipidemic subjects) was used as the calibrator since the apoA-I reactive epitopes of these anti-apoA-I antibodies were unknown. The optimized assays were very sensitive and a serum dilution of up to 1,000-fold was found to be sufficient for these assays. We evaluated whether differences detected by the new recombinant apoA-I antibodies and the respective direct two-site apoA-I assays are related to coronary atherosclerosis and its severity in patients with suspected obstructive CAD.
At present, LLM (mainly statins) is widely used for the prevention of cardiovascular disease by reducing LDL-C and TG (33) and its effect on HDL particles (34, 35). As these drugs also affect HDL (the effect is not systematically similar in each subject), we analyzed the data separately for patients with and without the use of LLM, in addition to the whole cohort. In line with the prescription of LLM according to the composite risk of ASCVD (36), subjects who were using LLM were older and had more risk factors for cardiovascular disease than patients not taking LLM. Subjects not using LLM displayed a higher level of TC, PL, and LDL-C, but interestingly there were no significant differences in levels of HDL-C or apoA-I determined by using the apoA-I ELISA method (30). The apoA-I level determined with the direct two-site apoA-I assay 109–121 (referred to as apoA-I109−121 ) and assay 110–525 (referred to as apoA-I110−525) was significantly higher in females compared to males. We found a positive correlation between direct two-site apoA-I assays and age specifically in patients not using LLM. The direct two-site apoA-I assays 110–525 did not show any significant correlation with a conventional ELISA-based apoA-I test; however, the assays 109–121 (in all the patients altogether and in patients without LLM) showed a weak positive correlation. It is worth noting that the ELISA-based apoA-I assay was performed using polyclonal antibodies against purified apoA-I (30). However, the direct two-site apoA-I assays use antibodies derived from the phage display library against the whole HDL particle and the epitopes of these antibodies are not yet characterized. Since these direct two-site apoA-I assays use as a calibrator HDL derived from the normolipidemic, disease-free subject, this indicates that the epitopes reacting with these mAbs also exist in HDL particles not modified by the disease itself. However, it is possible that among the different patient groups, the reacting epitopes could be sterically hindered/or induced to interact with these mAbs.
Among all the measured biochemical parameters (namely TG, TC, PL, HDL-C, LDL-C, apoA-I, PON-I, PLTP, and free glycerol), only the total PL serum and PON-I activity were significantly lower in patients with coronary atherosclerosis, which is in accordance with previous studies (37, 38). The reduced PL in HDL might reflect a reduced efflux capacity in the cholesterol from macrophage-foam cells; however, we have measured only the total serum PLs and not separately in each of the isolated HDL particles. The reduced PON-I activity indicates that anti-oxidative capacity mediated via the function of PON-1 is compromised in these subjects. The PON-1 function is physiologically important to attenuate the LDL oxidation generating minimally modified oxLDL which is related to atherogenesis (39). Notably, neither HDL-C nor LDL-C was significantly associated with atherosclerosis in the multivariable analyses. This finding further highlights, especially in the case of HDL, that the quantity of HDL-C alone has a limited value in the prediction of the presence of atherosclerosis. Moreover, in the case of LDL-C, evidence of the superiority of apoB-100 concentration over LDL-C in the evaluation of CHD risk is increasingly gaining strength; for instance, strong evidence has been shown in the study on the Framingham Offspring Cohort (40).
Previous studies have demonstrated that a low level of serum apoA-I is associated with CAD and MI (41), (42). Other studies have identified modified forms of apoA-I as poor acceptors of cholesterol from macrophage-foam cells during the process of reverse cholesterol transport (43–45), which is an important atheroprotective mechanism facilitated by HDL. Increased levels of modified apoA-I forms, such as chlorinated (46) and oxidized apoA-I (47), have been found in patients with CAD and acute coronary syndromes. In this study, the levels of apoA-I (determined with a conventional ELISA assay) were similar among patients with and without coronary atherosclerosis. However, a higher level of apoA-I110−525 was associated with coronary atherosclerosis in patients not using LLM. This association was present in multivariable models including age, gender, and traditional CAD risk factors. We also tested whether apoA-I110−525 could improve the identification of patients with coronary atherosclerosis as compared to traditional risk factors. FRS is a well-established method to evaluate the 10-year risk of having CHD (23). ApoA-I110−525 in combination with FRS predicted coronary atherosclerosis with a similar diagnostic accuracy as FRS alone. Notably, apoA-I110−525 adjusted for age and sex performed slightly better than FRS (based on an incremental AUC) in individuals not using LLM than in those taking LLM. Whether this approach could be used to estimate the likelihood of coronary atherosclerosis in LLM naive individuals remains to be tested in a larger cohort. In contrast to apoA-I110−525, apoA-I109−121 was lower in the presence of atherosclerosis among LLM users, indicating that these antibodies recognize different epitopes. The difference in the apoA-I109−121 levels was not significant when the risk factors were included.
The amount of coronary artery calcium reflects the extent of the coronary atherosclerosis and allows a risk prediction for the general population (48). The two-site apoA-I assays were not correlated/associated with the extent of coronary artery calcium or the presence of obstructive CAD (abnormal stress MBF or stenosis >70%). The lack of correlation with obstructive CAD might also be due to the small number of individuals with obstructive CAD in our study population, especially after stratification according to the use of LLM. The lack of correlation with the coronary calcium score might be explained by the fact that calcification represents a relatively late phenomenon in the progression of atherosclerosis, whereas serum lipid derangements will contribute to earlier stages of atherosclerosis. However, the coronary calcium score can be seen as an early marker of atherosclerotic disease in an individual as it shows the disease when it is in an early clinical phase. We do not have any specific explanation for our observation and this issue needs/requires further study.
There are some limitations to this study, which must be considered. Due to the small number of patients, the findings may be viewed as exploratory, but they demonstrate the proof-of-concept that recombinant apoA-I antibodies derived from the phage display library against CAD HDL particles may be used to improve the estimation of the risk of coronary atherosclerosis. However, the findings need to be confirmed in a larger cohort. With regard to the antibodies used in the direct two-site assays, they have already been characterized for their various properties as a part of the study where they were discovered and further investigated (18, 19). SDS-PAGE analysis demonstrated that the antibodies had adequate purity after affinity chromatography purification and the correct molecular size (19). Moreover, the DNA and translated polypeptide sequences of the antibodies are known. In addition, the purified biotinylated or europium chelate-labeled antibodies have been stored at +4°C for months without observed aggregates. However, any systematic study on their long-term stability in storage has unfortunately not been conducted. The antibodies used in this study and the aforementioned previous study (18) are in the form of single-chain antibody fragment (scFv) fused to bacterial alkaline phosphatase, i.e., scFv-AP. Although this construct corresponds to an IgG molecule in terms of certain relevant features such as its bivalent nature (i.e., alkaline phosphatase is a homodimer) and size (~150 kDa of scFv-AP dimer vs. 160 kDa of IgG), scFv-AP can still be a less optimal format for an immunoassay reagent than the intact IgG, which is considered a standard antibody format used in immunoassays. This can be a reason behind the somewhat high variation observed, especially with the direct two-site assay 110–525, and therefore also calls for/requires further optimization of this assay. Conversion of these antibodies to intact IgG, i.e., the format typically used in immunoassays, could enhance the robustness of these antibodies and help to make them more predictable as reagents. However, the conversion includes a risk of altering the binding properties of the antibodies, and the process should, therefore, involve careful characterization of binding properties and the possibility for additional genetic engineering of the binders. Unfortunately, this was out of the scope of this study but can be considered for future research work. In addition, these antibodies were characterized and studied for their ability to bind several different HDL forms and some HDL-associated proteins (19); however, their exact epitopes on apoA-I polypeptide are not known. Detailed characterization of the epitope responsible for the binding of antibodies as well as a functional characterization of the detected HDL particles would help to better understand the significance of the assays. For instance, dysfunctional apoA-I could be associated with an impaired ability of HDL to act as a cholesterol acceptor from macrophage-foam cells.
In summary, TRF-based direct two-site apoA-I immunoassays were developed using novel recombinant apoA-I antibody pairs (sc 109–121 and sc 110–525). The assays were clinically evaluated and compared with other traditional risk predictors (HDL-C, LDL-C, and FRS) with a well-characterized clinical cohort of patients with suspected obstructive CAD. In patients not using LLM, a high level of apoA-I identified with the antibody pair 110–525 was associated with the presence of coronary atherosclerosis; however, HDL-C and apoA-I levels measured using a polyclonal anti-apoA-I-based ELISA test were not. The direct two-site apoA-I assay 110–525 showed a similar prediction of atherosclerosis to FRS. In conclusion, assays targeting heterogeneity in HDL's main apolipoprotein, apoA-I, could provide a potential approach to improving the identification of patients with a risk of developing coronary atherosclerosis.
Data availability statement
The raw data supporting the conclusions of this article will be made available by the authors, without undue reservation.
Ethics statement
The studies involving human participants were reviewed and approved by Local Ethics Committee of the Hospital District of Southwest Finland. The patients/participants provided their written informed consent to participate in this study.
Author contributions
KP, JL, UL, MJ, JK, and AS contributed to the study concept and design. PN, TH, KV, and JM performed the laboratory work. PN and AS contributed to data analysis. AS, ET, WN, TM, and JK contributed to the collection of patients' samples or medical information or image acquisition and interpretation. PN, AS, and JM wrote the first draft of the manuscript. All authors contributed to the article and approved the submitted version.
Funding
This research was supported by the Doctoral Program of Clinical Investigation (CLIDP), University of Turku Graduate School (to PN), the Academy of Finland (grant # 257545 to MJ, grant # 310136 to AS), and the Finnish Foundation for Cardiovascular Research (to MJ and AS).
Acknowledgments
We sincerely express our gratitude to the individuals who provided their samples for this study. Riikka Lankinen is acknowledged for her technical assistance. The authors would like to acknowledge Eliisa Löyttyniemi (Department of Biostatistics, University of Turku, Turku, Finland) for her guidance in performing the statistical analysis.
Conflict of interest
Author AS declares fees for lectures or consultancy from Abbott, Amgen, Astra Zeneca (AS and JK), Boehringer Ingelheim, Bayer and Pfizer, Novartis (JK), GE Healthcare (JK).
The remaining authors declare that the research was conducted in the absence of any commercial or financial relationships that could be construed as a potential conflict of interest.
Publisher's note
All claims expressed in this article are solely those of the authors and do not necessarily represent those of their affiliated organizations, or those of the publisher, the editors and the reviewers. Any product that may be evaluated in this article, or claim that may be made by its manufacturer, is not guaranteed or endorsed by the publisher.
Supplementary material
The Supplementary Material for this article can be found online at: https://www.frontiersin.org/articles/10.3389/fcvm.2022.912578/full#supplementary-material
References
1. Mozaffarian D, Benjamin EJ, Go AS, Arnett DK, Blaha MJ, Cushman M, et al. Heart disease and stroke statistics-2015 update : a report from the American Heart Association. Circulation. (2015) 131:29−39. doi: 10.1161/CIR.0000000000000152
2. Gordon T, Castelli WP, Hjortland MC, Kannel WB, Dawber TR. High-density lipoprotein as a protective factor against coronary heart disease. The Framingham study. Am J Med Sci. (1977) 62:707–14. doi: 10.1016/0002-9343(77)90874-9
3. Gordon DJ, Probstfield JL, Garrison RJ, Neaton JD, Castelli WP, Knoke JD, et al. High-density lipoprotein cholesterol and cardiovascular disease. Four prospective American studies. Circulation. (1989) 79:8–15. doi: 10.1161/01.CIR.79.1.8
4. Robins S. Relation of gemfibrozil treatment and lipid levels with major coronary events: VA-HIT: a randomized controlled trial. JAMA. (2001) 28:1585–91. doi: 10.1001/jama.285.12.1585
5. Rye KA, Bursill CA, Lambert G, Tabet F, Barter PJ. The metabolism and anti-atherogenic properties of HDL. J Lipid Res. (2009) 50:S195–200. doi: 10.1194/jlr.R800034-JLR200
6. Shah AS, Tan L, Long JL, Davidson WS. Proteomic diversity of high-density lipoproteins: our emerging understanding of its importance in lipid transport and beyond. J Lipid Res. (2013) 54:2575–85. doi: 10.1194/jlr.R035725
7. Zannis VI, Fotakis P, Koukos G, Kardassis D, Ehnholm C, Jauhiainen M, et al. HDL biogenesis, remodeling, and catabolism. In:von Eckardstein A, Kardassis D, , editors. High-Density Lipoproteins From Biological Understanding to Clinical Exploitation. (2015). p. 53–111. doi: 10.1007/978-3-319-09665-0_2
8. Rached F, Santos RD, Camont L, Miname MH, Lhomme M, Dauteuille C, et al. Defective functionality of HDL particles in familial apoA-I deficiency: relevance of alterations in HDL lipidome and proteome. J Lipid Res. (2015) 55:2509–20. doi: 10.1194/jlr.M051631
9. Schaefer EJ, Santos RD, Asztalos BF. Marked HDL deficiency and premature coronary heart disease. Curr Opin Lipidol. (2010) 21:289–97. doi: 10.1097/MOL.0b013e32833c1ef6
10. Zannis V, Kateifides A, Fotakis P, Zanni E, Kardassis D. Pleiotropic functions of HDL lead to protection from atherosclerosis and other diseases. In:Kelishadi R, , editor. Dyslipidemia - From Prevention to Treatment. (2012). p. 173–96. doi: 10.5772/30054
11. Barter PJ, Caulfield M, Eriksson M, Grundy SM, Kastelein JJP, Komajda M, et al. Effects of torcetrapib in patients at high risk for coronary events. N Engl J Med. (2007) 357:2109–22. doi: 10.1056/NEJMoa0706628
12. Boden WE, Probstfield JL, Anderson T, Chaitman BR, Desvignes-Nickens P, Koprowicz K, et al. Niacin in patients with low HDL cholesterol levels receiving intensive statin therapy. N Engl J Med. (2011) 365:2255–67. doi: 10.1056/NEJMoa1107579
13. Schwartz GG, Olsson AG, Abt M, Ballantyne CM, Barter PJ, Brumm J, et al. Effects of dalcetrapib in patients with a recent acute coronary syndrome. N Engl J Med. (2012) 367:2089–99. doi: 10.1056/NEJMoa1206797
14. Voight BF, Peloso GM, Orho-Melander M, Frikke-Schmidt R, Barbalic M, Jensen MK, et al. Plasma HDL cholesterol and risk of myocardial infarction: a mendelian randomisation study. Lancet. (2012) 380:572–80. doi: 10.1016/S0140-6736(12)60312-2
15. Huang Y, Didonato JA, Levison BS, Schmitt D, Li L, Wu Y, et al. An abundant dysfunctional apolipoprotein A1 in human atheroma. Nat Med. (2014) 20:193–203. doi: 10.1038/nm.3459
16. Liu D, Ji L, Zhao M, Wang Y, Guo Y, Li L, et al. Lysine glycation of apolipoprotein A-I impairs its anti-inflammatory function in type 2 diabetes mellitus. J Mol Cell Cardiol. (2018) 122:47–57. doi: 10.1016/j.yjmcc.2018.08.001
17. Bradbury ARM, Sidhu S, Dübel S, McCafferty J. Beyond natural antibodies: the power of in vitro display technologies. Nat Biotechnol. (2011) 29:245–54. doi: 10.1038/nbt.1791
18. Negi P, Heikkilä T, Tallgren T, Malmi P, Lund J, Sinisalo J, et al. Three two-site apoA-I immunoassays using phage expressed detector antibodies – Preliminary clinical evaluation with cardiac patients. J Pharm Biomed Anal. (2021) 194:113772. doi: 10.1016/j.jpba.2020.113772
19. Negi P, Lövgren J, Malmi P, Sirkka N, Metso J, Huovinen T, et al. Identification and analysis of anti-HDL scFv-antibodies obtained from phage display based synthetic antibody library. Clin Biochem. (2016) 49:472–9. doi: 10.1016/j.clinbiochem.2015.11.020
20. Knuuti J, Ballo H, Juarez-Orozco LE, Saraste A, Kolh P, Rutjes AWS, et al. The performance of non-invasive tests to rule-in and rule-out significant coronary artery stenosis in patients with stable angina: a meta-analysis focused on post-test disease probability. Eur Heart J. (2018) 39:3322–30. doi: 10.1093/eurheartj/ehy267
21. Danad I, Uusitalo V, Kero T, Saraste A, Raijmakers PG, Lammertsma AA, et al. Quantitative assessment of myocardial perfusion in the detection of significant coronary artery disease: cutoff values and diagnostic accuracy of quantitative [15O]H2O PET imaging. J Am Coll Cardiol. (2014) 64:1464–75. doi: 10.1016/j.jacc.2014.05.069
22. Danad I. Raijmakers, Pieter G, Driessen RS, Leipsic J, Raju R, Naoum C, Knuuti J, et al. Comparison of coronary CT angiography, SPECT, PET, and hybrid imaging for diagnosis of ischemic heart disease determined by fractional flow reserve. JAMA Cardiol. (2017) 2:1100–7. doi: 10.1001/jamacardio.2017.2471
23. Wilson PWF, D'Agostino RB, Levy D, Belanger AM, Silbershatz H, Kannel WB. Prediction of coronary heart disease using risk factor categories. Circulation. (1998) 97:1837–47. doi: 10.1161/01.CIR.97.18.1837
24. Uusitalo V, Kamperidis V, de Graaf MA, Maaniitty T, Stenström I, Broersen A, et al. Coronary computed tomography angiography derived risk score in predicting cardiac events. J Cardiovasc Comput Tomogr. (2017) 11:274–80. doi: 10.1016/j.jcct.2017.04.010
25. Maaniitty T, Stenström I, Bax JJ, Uusitalo V, Ukkonen H, Kajander S, et al. Prognostic value of coronary CT angiography with selective PET perfusion imaging in coronary artery disease. JACC Cardiovasc Imaging. (2017) 10:1361–70. doi: 10.1016/j.jcmg.2016.10.025
26. Nesterov SV, Han C, Mäki M, Kajander S, Naum AG, Helenius H. Myocardial perfusion quantitation with 15O-labelled water PET: high reproducibility of the new cardiac analysis software (CarimasTM). Eur J Nucl Med Mol Imaging. (2009) 36:1594–602. doi: 10.1007/s00259-009-1143-8
27. Wang Q, Nchimi Nono K, Syrjänpää M, Charbonnieìre LJ, Hovinen J, Härmä H. Stable and highly fluorescent europium(III) chelates for time-resolved immunoassays. Inorg Chem. (2013) 52:8461–6. doi: 10.1021/ic400384f
28. Eriksson S, Junikka M, Laitinen P, Majamaa-Voltti K, Alfthan H, Pettersson K. Negative interference in cardiac troponin I immunoassays from a frequently occurring serum and plasma component. Clin Chem. (2003) 49:1095–104. doi: 10.1373/49.7.1095
29. Warnick GR, Cheung MC, Albers JJ. Comparison of current methods for high-density lipoprotein cholesterol quantitation. Clin Chem. (1979) 25:596–604. doi: 10.1093/clinchem/25.4.596
30. Siggins S, Jauhiainen M, Olkkonen VM, Tenhunen J, Ehnholm C. PLTP secreted by HepG2 cells resembles the high-activity PLTP form in human plasma. J Lipid Res. (2003) 44:1698–704. doi: 10.1194/jlr.M300059-JLR200
31. Jauhiainen M, Ehnholm C. Determination of human plasma phospholipid transfer protein mass and activity. Methods. (2005) 36:97–101. doi: 10.1016/j.ymeth.2004.11.006
32. Kleemola P, Freese R, Jauhiainen M, Pahlman R, Alfthan G, Mutanen M. Dietary determinants of serum paraoxonase activity in healthy humans. Atherosclerosis. (2002) 160:425–32. doi: 10.1016/S0021-9150(01)00594-9
33. Jones PH, Davidson MH, Stein EA, Bays HE, McKenney JM, Miller E, et al. Comparison of the efficacy and safety of rosuvastatin vs. atorvastatin, simvastatin, and pravastatin across doses (STELLAR* Trial). Am J Cardiol. (2003) 92:152–60. doi: 10.1016/S0002-9149(03)00530-7
34. Miyamoto-Sasaki M, Yasuda T, Monguchi T, Nakajima H, Mori K, Toh R, et al. Pitavastatin increases HDL particles functionally preserved with cholesterol efflux capacity and antioxidative actions in dyslipidemic patients. J Atheroscler Thromb. (2013) 20:708–16. doi: 10.5551/jat.17210
35. Triolo M, Annema W, de Boer JF, Tietge UJF, Dullaart RPF. Simvastatin and bezafibrate increase cholesterol efflux in men with type 2 diabetes. Eur J Clin Invest. (2014) 44:240–8. doi: 10.1111/eci.12226
36. Mortensen MB, Falk E. Primary prevention with statins in the elderly. J Am Coll Cardiol. (2018) 71:85–94. doi: 10.1016/j.jacc.2017.10.080
37. Fan Y, Li Y, Chen Y, Zhao YJ, Liu LW Li J, et al. Comprehensive metabolomic characterization of coronary artery diseases. J Am Coll Cardiol. (2016) 68:1281–93. doi: 10.1016/j.jacc.2016.06.044
38. Wang M, Lang X, Cui S, Zou L, Cao J, Wang S, et al. Quantitative assessment of the influence of paraoxonase 1 activity and coronary heart disease risk. DNA Cell Biol. (2012) 31:975–82. doi: 10.1089/dna.2011.1478
39. Khatana C, Saini NK, Chakrabarti S, Saini V, Sharma A, Saini RV, et al. Mechanistic insights into the oxidized low-density lipoprotein-induced atherosclerosis. Oxid Med Cell Longev. (2020) 2020:5245308. doi: 10.1155/2020/5245308
40. Pencina MJ, D'Agostino RB, Zdrojewski T, Williams K, Thanassoulis G, Furberg CD, et al. Apolipoprotein B improves risk assessment of future coronary heart disease in the Framingham heart study beyond LDL-C and non-HDL-C. Eur J Prev Cardiol. (2015) 22:1321–7. doi: 10.1177/2047487315569411
41. Thompson A, Danesh J. Associations between apolipoprotein B, apolipoprotein AI, the apolipoprotein B/AI ratio and coronary heart disease: a literature-based meta-analysis of prospective studies. Intern Med J. (2006). 259:481–92. doi: 10.1111/j.1365-2796.2006.01644.x
42. Walldius G, Jungner I, Holme I, Aastveit AH, Kolar W, Steiner E. High apolipoprotein B, low apolipoprotein A-I, and improvement in the prediction of fatal myocardial infarction (AMORIS study): a prospective study. Lancet. (2001) 358:2026–33. doi: 10.1016/S0140-6736(01)07098-2
43. Urundhati A, Huang Y, Lupica JA, Smith JD, DiDonato JA, Hazen SL. Modification of high-density lipoprotein by myeloperoxidase generates a pro-inflammatory particle. J Biol Chem. (2009) 284:30825–35. doi: 10.1074/jbc.M109.047605
44. Nicholls SJ, Zheng L, Hazen SL. Formation of dysfunctional high-density lipoprotein by myeloperoxidase. Trends Cardiovasc Med. (2005) 15:212–9. doi: 10.1016/j.tcm.2005.06.004
45. Bergt C, Fu X, Huq NP, Kao J, Heinecke JW. Lysine residues direct the chlorination of tyrosines in YXXK motifs of apolipoprotein A-I when hypochlorous acid oxidizes high-density lipoprotein. J Biol Chem. (2004) 279:7856–66. doi: 10.1074/jbc.M309046200
46. Shao B, Bergt C, Fu X, Green P, Voss JC Oda MN, et al. Tyrosine 192 in apolipoprotein A-I is the major site of nitration and chlorination by myeloperoxidase, but only chlorination markedly impairs ABCA1-dependent cholesterol transport. J Biol Chem. (2005) 280:5983–93. doi: 10.1074/jbc.M411484200
47. Shao B, Cavigiolio G, Brot N, Oda MN, Heinecke JW. Methionine oxidation impairs reverse cholesterol transport by apolipoprotein A-I. Proc Natl Acad Sci U S A. (2008) 105:12224–9. doi: 10.1073/pnas.0802025105
Keywords: coronary artery disease, phage display, high-density lipoprotein, apolipoprotein A-I, immunoassay, single-chain variable fragment, coronary computed tomography angiography, positron emission tomography
Citation: Negi P, Heikkilä T, Vuorenpää K, Tuunainen E, Nammas W, Maaniitty T, Knuuti J, Metso J, Lövgren J, Jauhiainen M, Lamminmäki U, Pettersson K and Saraste A (2022) Time-resolved fluorescence based direct two-site apoA-I immunoassays and their clinical application in patients with suspected obstructive coronary artery disease. Front. Cardiovasc. Med. 9:912578. doi: 10.3389/fcvm.2022.912578
Received: 04 April 2022; Accepted: 20 September 2022;
Published: 14 October 2022.
Edited by:
Michail Papafaklis, University Hospital of Ioannina, GreeceReviewed by:
Santosh Karnewar, University of Virginia, United StatesMark Borja, California State University, East Bay, United States
Copyright © 2022 Negi, Heikkilä, Vuorenpää, Tuunainen, Nammas, Maaniitty, Knuuti, Metso, Lövgren, Jauhiainen, Lamminmäki, Pettersson and Saraste. This is an open-access article distributed under the terms of the Creative Commons Attribution License (CC BY). The use, distribution or reproduction in other forums is permitted, provided the original author(s) and the copyright owner(s) are credited and that the original publication in this journal is cited, in accordance with accepted academic practice. No use, distribution or reproduction is permitted which does not comply with these terms.
*Correspondence: Priyanka Negi, cHJpeWFua2EubWljcm9AZ21haWwuY29t; Antti Saraste, YW50c2FyYXNAdXR1LmZp