- 1Center for Precision Medicine and Division of Cardiovascular Medicine, Department of Medicine, University of Missouri School of Medicine, Columbia, MO, United States
- 2Department of Gastroenterology, The Third Xiangya Hospital, Central South University, Changsha, China
- 3Dalton Cardiovascular Research Center, University of Missouri, Columbia, MO, United States
- 4Department of Medical Pharmacology and Physiology, Columbia, MO, United States
Background: Helicobacter pylori (H. pylori) infection increases the risk for atherosclerosis, and ROS are critical to endothelial dysfunction and atherosclerosis. CagA is a major H. pylori virulence factor associated with atherosclerosis. The present study aimed to test the hypothesis that CagA+ H. pylori effectively colonizes gastric mucosa, and CagA+ H. pylori, but not CagA– H. pylori, infection impairs endothelial function through exosomes-mediated ROS formation.
Methods: C57BL/6 were used to determine the colonization ability of CagA+ H. pylori and CagA– H. pylori. ROS production, endothelial function of thoracic aorta and atherosclerosis were measured in CagA+ H. pylori and CagA– H. pylori infected mice. Exosomes from CagA+ H. pylori and CagA– H. pylori or without H. pylori infected mouse serum or GES-1 were isolated and co-cultured with bEND.3 and HUVECs to determine how CagA+ H. pylori infection impairs endothelial function. Further, GW4869 was used to determine if CagA+H. pylori infection could lead to endothelial dysfunction and atherosclerosis through an exosomes-mediated mechanism.
Results: CagA+ H. pylori colonized gastric mucosa more effectively than CagA– H. pylori in mice. CagA+ H. pylori, not CagA– H. pylori, infection significantly increased aortic ROS production, decreased ACh-induced aortic relaxation, and enhanced early atherosclerosis formation, which were prevented with N-acetylcysteine treatment. Treatment with CagA-containing exosomes significantly increased intracellular ROS production in endothelial cells and impaired their function. Inhibition of exosomes secretion with GW4869 effectively prevented excessive aortic ROS production, endothelial dysfunction, and atherosclerosis in mice with CagA+ H. pylori infection.
Conclusion: These data suggest that CagA+ H. pylori effectively colonizes gastric mucosa, impairs endothelial function, and enhances atherosclerosis via exosomes-mediated ROS formation in mice.
Introduction
Helicobacter pylori (H. pylori) colonizes the human gastric epithelium in a significant portion of the general population, ranging from 30 to 50% in developed countries and to approximately 80% in developing countries especially in Asia (1, 2). H. pylori has multiple strains, and based on the presence of cytotoxin-associated gene antigen (CagA), H. pylori is divided into two major categories: CagA-positive and CagA-negative (3, 4). The majority of patients in East Asian countries with H. pylori infection (>90%) are infected with CagA-positive H. pylori (5). CagA is a major virulence factor in H. pylori, which encodes the CagA protein and can be translocated into host cells through the type IV secretion system (T4SS) (5). Epidemiological data and meta-analysis reveal a much stronger correlation between infection with CagA+ H. pylori strains and atherosclerosis in patients compared to that of CagA– H. pylori strains (6). However, it is not clear why CagA+ H. pylori is the dominant strain in patient infections, and how it is associated with extra gastrointestinal conditions including atherosclerosis.
Endothelial dysfunction contributes to the development and progression of atherosclerosis (7). H. pylori infection significantly increases the risk for cardiovascular diseases including atherosclerosis and hypertension (8, 9). Recent studies with both human subjects and animal models have demonstrated that H. pylori infection significantly impairs endothelial function through a pathway involving exosomes (10). Exosomes are known to be critically involved in cell-to-cell communication and cell functions through various mechanisms including regulation of extra- and intracellular redox states via direct and/or indirect modification (either increase or decrease) of reactive oxygen species (ROS) content (11–13). The present study was to test the hypotheses that: (1) CagA+ H. pylori colonizes gastric mucosa more effectively than CagA– H. pylori; and (2) CagA+ H. pylori infection, but not CagA– H. pylori infection, impairs endothelial function through CagA-containing exosomes-mediated ROS formation. The objectives were to determine: (1) if there was a significant difference in gastric colonization between CagA+ H. pylori and CagA– H. pylori; (2) if there were significant differences in endothelial function and atherosclerosis between mice infected with CagA+ H. pylori and CagA– H. pylori infection; (3) if a significant difference in exosomes production was evident in human gastric epithelial cells (GES-1) co-cultured with either CagA+ H. pylori or CagA– H. pylori; and 4) if CagA-containing exosomes impair endothelial function and enhance development of atherosclerosis via increased ROS formation.
Materials and Methods
Helicobacter pylori Culture
CagA+ H. pylori in the present study was isolated from the gastric specimens of a gastric ulcer patient during gastroscopy at the Third Xiangya Hospital of Central South University (Changsha, Hunan, China), and its identity confirmed using the complete sequence data of H. pylori 16s rRNA gene from GenBank data and positive biochemical tests as described (10). CagA– H. pylori (ATCC 51932) were purchased from American Type Culture Collection (ATCC, Manassas, VA, United States). Both strains were cultured for 3–4 days on Columbia blood agar plates supplemented with antibiotics and 10% sheep blood (Fisher Scientific 50863755, Waltham, MA, United States) under a microaerophilic milieu (5% O2, 10% CO2, and 85% N2) at 37°C. The concentration of H. pylori was determined by measuring the optical density at OD 600 nm, where 1 unit of OD 600 nm corresponds to about 2 × 108 colony-forming unit (CFU)/ml (10).
Cell Culture and Cell-Bacteria Co-culture
Human gastric epithelial cell line (GES-1) was obtained from Professor Canxia Xu in Department of Gastroenterology, the Third Xiangya Hospital, Central South University; Changsha, Hunan, China. Human umbilical vein endothelial cells (HUVECs) and mouse brain microvascular endothelial cells (bEnd.3) were purchased from ATCC and cultured in RPMI-1640 (Gibco, Grand Island, NY, United States), endothelial cell medium (Sciencell Research Laboratories, Carlsbad, CA, United States), and DMEM (Gibco, Grand Island, NY, United States), respectively, supplemented with 10% fetal bovine serum (FBS) (Gibco, Grand Island, NY, United States), 100 U/ml penicillin, and 100 mg/ml streptomycin in a controlled humidified incubator with 5% CO2 and 95% room air. To evaluate the effect of exosomes on endothelial cells, exosomes (100 ug/ml) from conditioned media of GES-1 co-cultured with CagA+ H. pylori, CagA– H. pylori or PBS without H. pylori, or exosomes from the serum of mice with CagA+ H. pylori, CagA– H. pylori infection or without H. pylori infection were cultured with HUVECs or bEND.3. After being cultured for 4 h, HUVECs or bEND.3 were tested for ROS production using the fluorescent dye 2′,7′-dichlorodihydrofluorescein diacetate (H2DCFDA, Invitrogen D399, Waltham, MA, United States) as described (14). GES-1 was cultured with H. pylori at a MOI (multiplicity of infection) of 100 for 12 h as described (10).
Animal Models
All animal experiments were performed in accordance with the “Guide for the Care and Use of Laboratory Animals of the US National Institutes of Health.” The experimental protocols were reviewed and approved by the Institutional Animal Care and Use Committee of the University of Missouri School of Medicine, Columbia, MO, United States. Specific-pathogen-free 4–6 week-old male C57BL/6 wild-type mice (WT) and LDLR knockout mice (LDLR–/–) were from Jackson Lab (ME, United States), and were fed rodent diet and water ad libitum.
After fasting overnight, mice were given 0.2 ml of PBS, CagA– H. pylori or CagA+ H. pylori inoculums (approximate 4 × 109 CFU/ml) by intragastric gavage as described (10). The presence of H. pylori infection was assessed at 1-week post-infection or at the time when mice were sacrificed, using Rapid Urease Test (RUT) and Giemsa staining of gastric mucosa. To evaluate the effect of H. pylori infection on the development of early atherosclerosis, LDLR–/– mice were fed a high fat diet (HFD) (Envigo TD.88137, Indianapolis, IN, United States) beginning 1 week after H. pylori gavage. WT mice were sacrificed 1 week after the last gavage to collect thoracic aorta to determine endothelium-dependent and -independent vascular relaxation responses and ROS production (see details below). LDLR–/– mice were sacrificed after week 3, 5, and 12 of HFD feeding to collect whole aorta and aortic root for atherosclerotic lesion analysis (see details below).
Evaluation of Vascular Endothelium Relaxation in Mice
Thoracic aorta was isolated from mice to evaluate endothelium-dependent and -independent vascular relaxation responses as described (10). The thoracic aorta was cut into 2–3 mm segments and mounted onto a four-channel Wire Myograph System (610M; DMT, Aarhus, Denmark). Aortic segments were equilibrated with a resting tension of 4.9 mN for 45–60 min in a temperature-controlled tissue bath filled with 5 ml of Krebs’ solution and bubbled continuously with 95% O2 and 5% CO2, at 37°C. The aortic preparations were then tested for maximal contraction with 50 mM KCl, and concentration-dependent vasocontractile responses to phenylephrine (PE). After adequate washout with Krebs solution and equilibration, the aortic tissues were examined for endothelium-dependent relaxation to cumulative doses of acetylcholine (ACh, 10–9–10–5M) and endothelium-independent relaxation to cumulative doses of nitroglycerin (NTG, 10–9–10–5M) after submaximal contraction with PE.
Administration of N-Acetylcysteine
To further confirmed the role of oxidative stress on endothelial dysfunction caused by H. pylori infection, the antioxidant NAC was used to treat the mice in vivo and endothelial cells in vitro. NAC is an FDA-approved drug and has been traditionally considered an antioxidant that effectively attenuates ROS production (15).
Mice in the NAC treatment group received NAC (Sigma-Aldrich, MO, United States, 1 mg/ml in drinking water) 3 days before the first gavage until the end of the experiment (16). NAC was changed every other day and covered with aluminum foil to avoid exposure to direct light. For the in vitro study, endothelial cells were incubated with 10 mM NAC as described (17).
Quantification of Atherosclerotic Lesions
Atherosclerotic burden in thoracic aorta and cross sections of aortic root was quantified with Oil Red O staining as described (18). Briefly, the thoracic aorta was collected immediately from euthanized mice and carefully prepared with the removal of periadventitial fat after perfusion with sterile phosphate-buffered saline (PBS). Thoracic aorta was cut open longitudinally after being washed twice with 60% isopropanol and images taken with a digital camera after being stained with Oil Red O dye for 30 min at room temperature. Aortic roots were frozen with optimum cutting temperature O.C.T. Compound (Fisher, Waltham, MA, United States) and serial sections (10-μm thick) were cut through the aortic valve as described (18). The sections were stained with Oil Red O for plaque quantification in the cross-section areas. The total lesion area and lesion area percentage were analyzed and quantified using Image J software.
Determination of Reactive Oxygen Species Formation in Cryostat Sections of Aorta
Dihydroethidium (DHE, Invitrogen D23107, Waltham, MA, United States) was used to assess ROS formation in cryostat sections of aorta using fluorescence microscopy (19). Cryostat sections (5 μm) of mouse aortic rings were incubated with 5 μM DHE in normal physiological saline solution (NPSS, composition in mmol L-1: NaCl 140, KCl 5, CaCl2 1, MgCl2 1, glucose 10, and HEPES 5) for 7 min as described (19). Aortic rings were washed three times to remove DHE after incubation, and then examined with a fluorescence microscope. The fluorescence intensity was evaluated with 518 nm excitation and 606 nm emission, and the images were analyzed using Image J software.
Measurement of Intracellular Reactive Oxygen Species Production
The level of intracellular ROS in HUVECs or bEND.3 was evaluated using the fluorescent dye 2’7’-dichlorodihydrofluorescein diacetate (H2DCFDA; Invitrogen D399) (14) after treatment with exosomes from conditioned media or mouse serum as describe (14). H2DCFDA is a non-polar compound that is converted by cellular esterase to the polar and membrane impermeable derivative H2DCF. H2DCF is non-fluorescent but becomes highly fluorescent 2’,7’-dichlorofluorescein (DCF) when oxidized in the presence of intracellular ROS. After treatment with exosomes for 4 h, endothelial cells were washed twice with pre-warmed PBS, and then incubated with 15 uM H2DCFDA for 30 min at 37°C in the dark. Cells were washed twice with pre-warmed PBS after removing the dye. The fluorimetric signal in the cells was examined and analyzed using a fluorescence microscope. The fluorescence intensity was evaluated using ImageJ software.
Exosome Isolation and Characterization
To prepare exosomes from conditioned media, human gastric epithelium cells (GES-1) were cultured with PBS, CagA– H. pylori or CagA+ H. pylori at MOI of 100 for 12 h. The conditioned media and mice serum were collected to isolate the exosomes as described (20). Briefly, the conditioned media were successive centrifuged at 4°C (300 × g for 10 min, 2,000 × g for 20 min, and 10,000 × g for 30 min) to eliminate the cells and cell debris. The supernatant was then ultracentrifuged at 100,000 × g at 4°C for 70 min for two times (Beckman Coulter, Indianapolis, IN, United States). Exosome pellets were re-suspended in PBS for further analysis. Serum from mice with CagA– H. pylori or CagA+ H. pylori infection and control mice was collected for exosome isolation similar to conditioned media as described above (20). Exosomes identification of morphologies, size distribution, and biomarkers were assessed using a transmission electron microscopy (TECNAI G2 Spirit; FEI, Hillsboro, OR, United States), dynamic light scattering with a particle and molecular size analyzer (Zetasizer Nano ZS) and Western blotting as described (10).
Statistical Analysis
Data were expressed as mean ± standard error of the mean (SEM) and analyzed with SPSS statistical software (22.0 for Windows; SPSS, Chicago, IL, United States). A two-tailed unpaired t-test was used for the analysis of two groups of data with normal distribution and equal variance, and a two-tailed unpaired t-test with Welch’s correction for analyzing two groups of data with normal distribution and unequal variance. Mann–Whitney U test was used for comparisons between two groups of data with abnormal distributions. One-way analysis of variance (ANOVA) was used for three or more groups of data analysis with normal distributions and equal variance, and the Kruskal–Wallis test with Dunn post-hoc multiple comparison tests was used for three or more groups of data analysis with normal distributions and unequal variance or abnormal distributions. P < 0.05 was considered significant.
Results
CagA+ Helicobacter pylori Colonized Gastric Mucosa More Effectively Than CagA– Helicobacter pylori in Mice
As over 90% of Asian H. pylori patients are infected with CagA+ H. pylori, we hypothesized that CagA+ H. pylori would exhibit greater gastric colonization than CagA– H. pylori. A total of 110 male C57BL/6 mice were divided into 11 groups (10 mice in each group) and infected with either CagA+H. pylori or CagA– H. pylori using 4 different infection protocols (Table 1). Phospho-buffered saline (PBS) treatment served as negative control. Animals were considered infected when both RUT and Giemsa staining were positive. A 100% infection rate was achieved in mice receiving three daily doses of CagA+ H. pylori, whereas six doses of CagA– H. pylori were required to achieve a 100% infection rate (Table 1). CagA+ H. pylori exhibited a higher gastric colonization rate than CagA– H. pylori under all infection conditions. All control mice were negative for H. pylori infection, confirming that no H. pylori contamination existed in the animal facility.
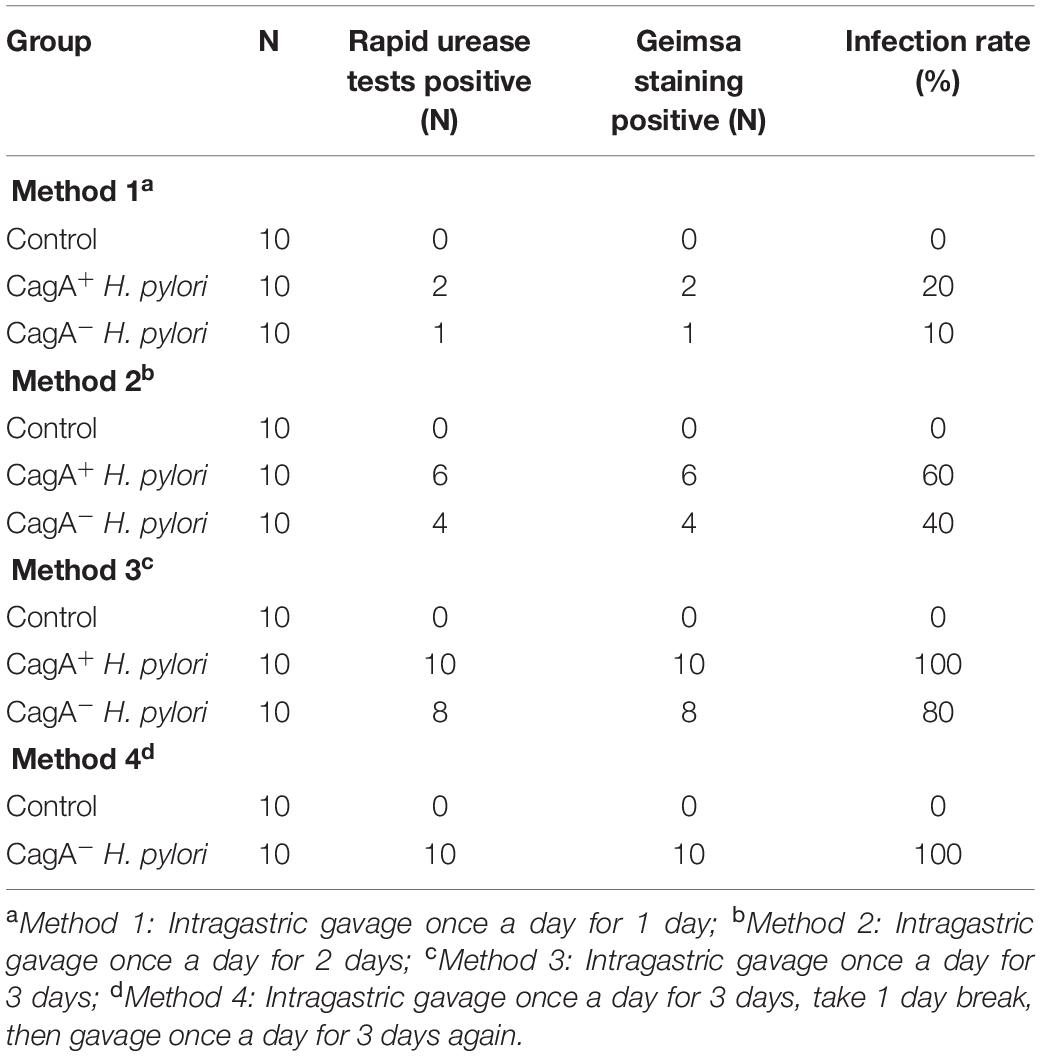
Table 1. Infection rate of CagA+ Helicobacter pylori and CagA– H. pylori 1 week after last intragastric gavage.
CagA+ Helicobacter pylori, Not CagA– Helicobacter pylori Infection, Induced Endothelial Dysfunction, and Promoted Atherosclerosis
To determine if a significant difference in endothelial dysfunction existed between mice infected with CagA+ H. pylori and CagA– H. pylori, both acute (1 week) and chronic (12 weeks) infection models were established using C57BL/6 mice with PBS as control. Ex vivo acetylcholine (ACh)-induced endothelium-dependent relaxation of aortic rings was significantly decreased in mice with CagA+ H. pylori infection at both 1 week and 12 weeks post-infection compared with control (Figures 1A,B). Endothelium-independent relaxation to nitroglycerin (NTG) was unchanged between the groups (Supplementary Figures 1A,B). In contrast, no significant changes in ACh-induced relaxation (Figures 1C,D) or NTG-induced relaxation (Supplementary Figures 1C,D) were observed in mice with CagA– H. pylori infection compared with control.
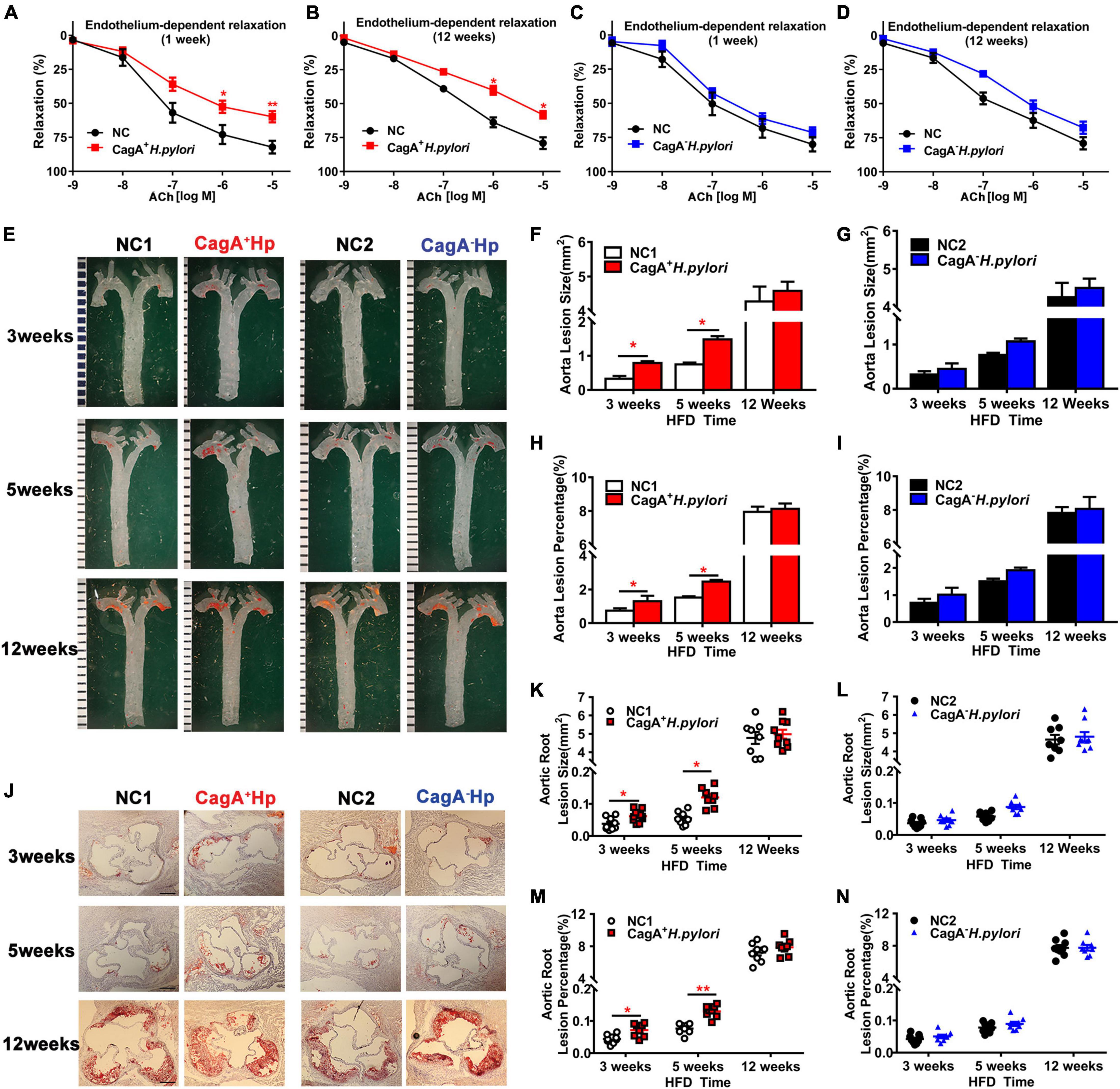
Figure 1. CagA+ Helicobacter pylori, not CagA– H. pylori infection, led to significant endothelial dysfunction, and promoted the development of atherosclerosis. ACh-induced aortic relaxation was significantly reduced in male C57BL/6 mice after 1 week (A) or 12 weeks (B) of CagA+ H. pylori infection compared with control, while no change in ACh-induced aortic relaxation in mice with CagA– H. pylori infected was observed (C,D); (E–I) Representative images and quantification of Oil-red O-stained whole aorta of LDLR–/– mice with PBS, CagA+ H. pylori or CagA– H. pylori infection after 3, 5, and 12 weeks of high-fat diet (HFD). (J–N) Representative images of cross-section histological and quantification of atherosclerotic lesions in aortic roots. NC(1/2): normal control; ACh: acetylcholine; CagA+Hp: CagA+ H. pylori; CagA– Hp: CagA– H. pylori. Data are presented as mean ± SEM. *P < 0.05, **P < 0.01 by t-test, N = 8–10 mice for each group at each time point.
To determine if CagA+ H. pylori infection promotes the development of atherosclerosis, LDLR–/– mice were infected with CagA+ H. pylori or CagA– H. pylori with PBS as control. Infection with CagA+ H. pylori significantly increased aortic atherosclerotic lesion areas in mice compared with PBS-treated controls after 3 and 5 weeks of HFD. However, no significant differences in aortic atherosclerotic lesion areas were present between LDLR–/– mice with CagA– H. pylori infection and PBS-treated controls. After 12 weeks of HFD, all LDLR–/– mice developed extensive atherosclerotic lesions with or without CagA+ H. pylori or CagA– H. pylori infection (Figures 1E–N). These data suggest that CagA+ H. pylori, but not CagA– H. pylori infection, accelerates early atherosclerotic development in LDLR–/– mice on an HFD.
CagA+ Helicobacter pylori Infection Impaired Endothelial Function via Reactive Oxygen Species Production in Aorta
To test the hypothesis that CagA+ H. pylori infection impairs endothelial function through increased ROS production, aortic vasodilation and ROS levels were assessed in C57BL/6 mice infected with CagA+ H. pylori or CagA– H. pylori and controls receiving PBS. CagA+ H. pylori infection significantly decreased ACh-induced aortic relaxation in association with significantly increased aortic ROS production compared with their controls (Figure 2A). In contrast, CagA– H. pylori infection had no significant effect on either aortic ACh-induced relaxation or ROS levels compared with controls (Figure 2A). There was no change in NTG-induced aortic relaxation in mice infected with CagA+ H. pylori or CagA– H. pylori (Supplementary Figure 1). N-acetylcysteine (NAC) treatment effectively blocked excessive ROS production in aortic segments (Figure 2B) and preserved ACh-induced relaxation in mice after 1 or 12 weeks of CagA+ H. pylori infection (Figures 2C,D), without differences in NTG-induced relaxation (Figures 2E,F).
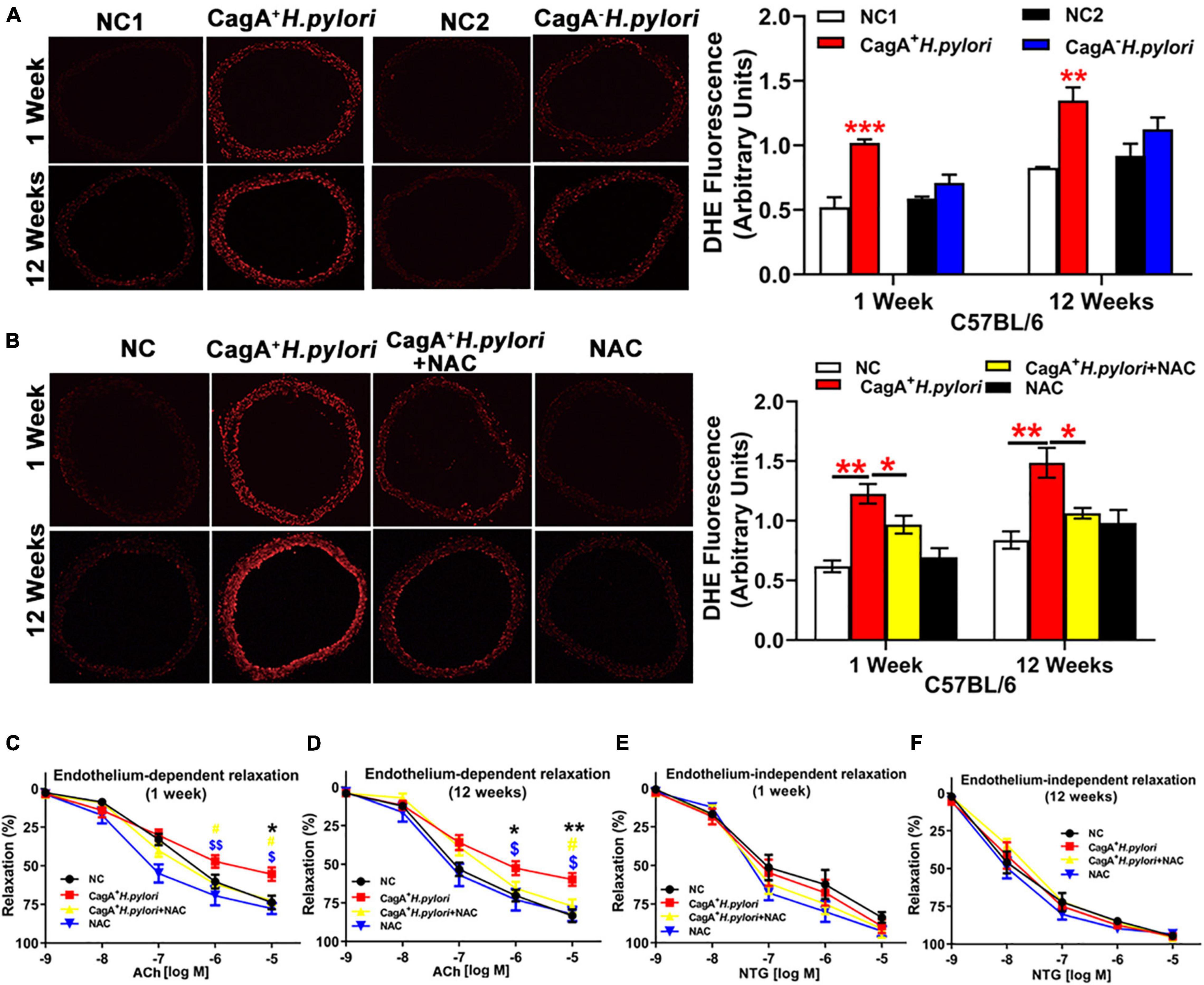
Figure 2. CagA+ H. pylori, not CagA– H. pylori infection, impaired endothelial function through increased ROS production in mice. Representative fluorescent images and quantification of ROS formation in the aorta of male C57BL/6 mice (A) (**P < 0.01, ***P < 0.001 by t-test) with CagA+ H. pylori, CagA– H. pylori or PBS gavage. Aortic ROS production was significantly increased in mice with CagA+ H. pylori infection, not with CagA– H. pylori infection. Treatment with NAC prevented aortic ROS production (B) (*P < 0.05**P < 0.01 by one-way ANOVA) and preserved ACh-induced aortic relaxation (C,D) in mice with 1 or 12 weeks of CagA+ H. pylori infection, without change in NTG-induced aortic relaxation (E,F). *P < 0.05, **P < 0.01 (compared with NC), #P < 0.05 (compared with CagA+ H. pylori + NAC); $P < 0.05, $$P < 0.01 (compared with NAC) by one-way ANOVA. NC(1/2): normal control. NAC: N-acetylcysteine; ACh: acetylcholine; NTG: nitroglycerin. Data are presented as mean ± SEM; N = 8–10 mice for each group at each time point.
CagA+ H. pylori, not CagA– H. pylori infection also significantly increased aortic ROS production in LDLR–/– mice with 3 or 5 weeks of HFD feeding compared with their controls (Supplementary Figures 2A,B). After 12 weeks of HFD feeding, all LDLR–/– mice exhibited increased ROS production with or without CagA+ H. pylori or CagA– H. pylori infection (Supplementary Figure 2C).
CagA-Containing Exosomes Impaired Endothelial Function
To test the hypothesis that CagA+ H. pylori, but not CagA– H. pylori, infection promotes exosomes production, leading to endothelial dysfunction, serum exosomes were prepared from mice with CagA+ H. pylori and CagA– H. pylori infection as well as non-infected control mice. Exosomes were characterized using transmission electron microscopy (TEM), nanoparticle tracking analysis (NTA), and western blotting (Figures 3A–C). Although there was no significant difference in serum exosomes level from mice infected with either H. pylori strain or controls (Figure 3D), treatment with serum exosomes from mice infected with CagA+ H. pylori significantly inhibited the function of mouse bEND.3 cells in vitro with decreases in migration, tube formation and proliferation compared with control exosomes (Figures 3E–G). Culture with serum exosomes from mice with CagA– H. pylori infection had no significant effect on endothelial function (Figures 3E–G).
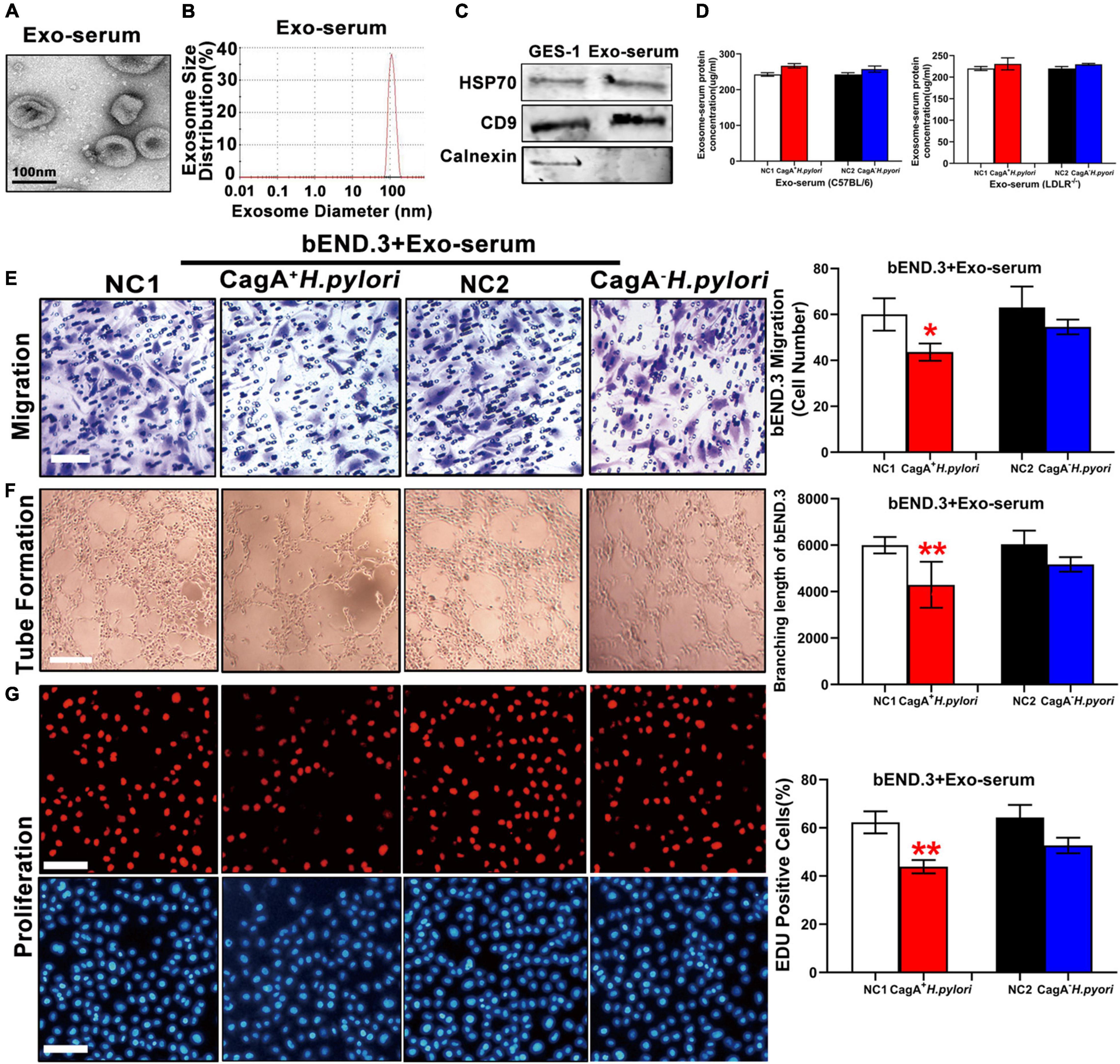
Figure 3. Serum exosomes from CagA+ H. pylori infected mice impaired endothelial function. Mouse serum exosomes displayed typical features for exosomes including morphology on transmission electron microscopy (A) and size distribution (B). Western blotting analysis showed that the exosomes markers HSP70 and CD9 were present in the exosomes without the presence of calnexin (C). Although there was no significant difference in serum exosomes levels from mice infected with H. pylori compared to the controls (D), treatment with serum exosomes from mice with CagA+ H. pylori infection significantly inhibited the function of mouse bEND.3 cells in vitro with decreased migration (E, scale bars = 25 μm), tube formation (F, scale bars = 25 μm), and proliferation (G, scale bars = 100 μm). Exo, Exosomes; Exo-serum: exosomes isolated from mouse serum; NC(1/2): normal control. Data are resented as mean ± SEM; *P < 0.05; **P < 0.01 by t-test, N = 8–10 mice for each group. Experiment was repeated 3 times for every measurement.
Exosomes from conditioned medium of human gastric epithelial cells (GES-1) cultured with CagA+ H. pylori or with CagA– H. pylori were prepared and similarly characterized by TEM, NTA and western blotting (Figures 4A–C). Co-culture of GES-1 with CagA+ H. pylori, not CagA– H. pylori, significantly increased the exosomes level in conditioned media (Figure 4D). When using the same amount of exosomes (by protein level), exosomes from GES-1 co-cultured with CagA+ H. pylori significantly inhibited the functional properties of HUVECs in vitro with decreased proliferation, migration, and tube formation compared with non-infected control, while exosomes from GES-1 co-cultured with CagA– H. pylori did not significantly impact endothelial cell function (Figures 4E–G).
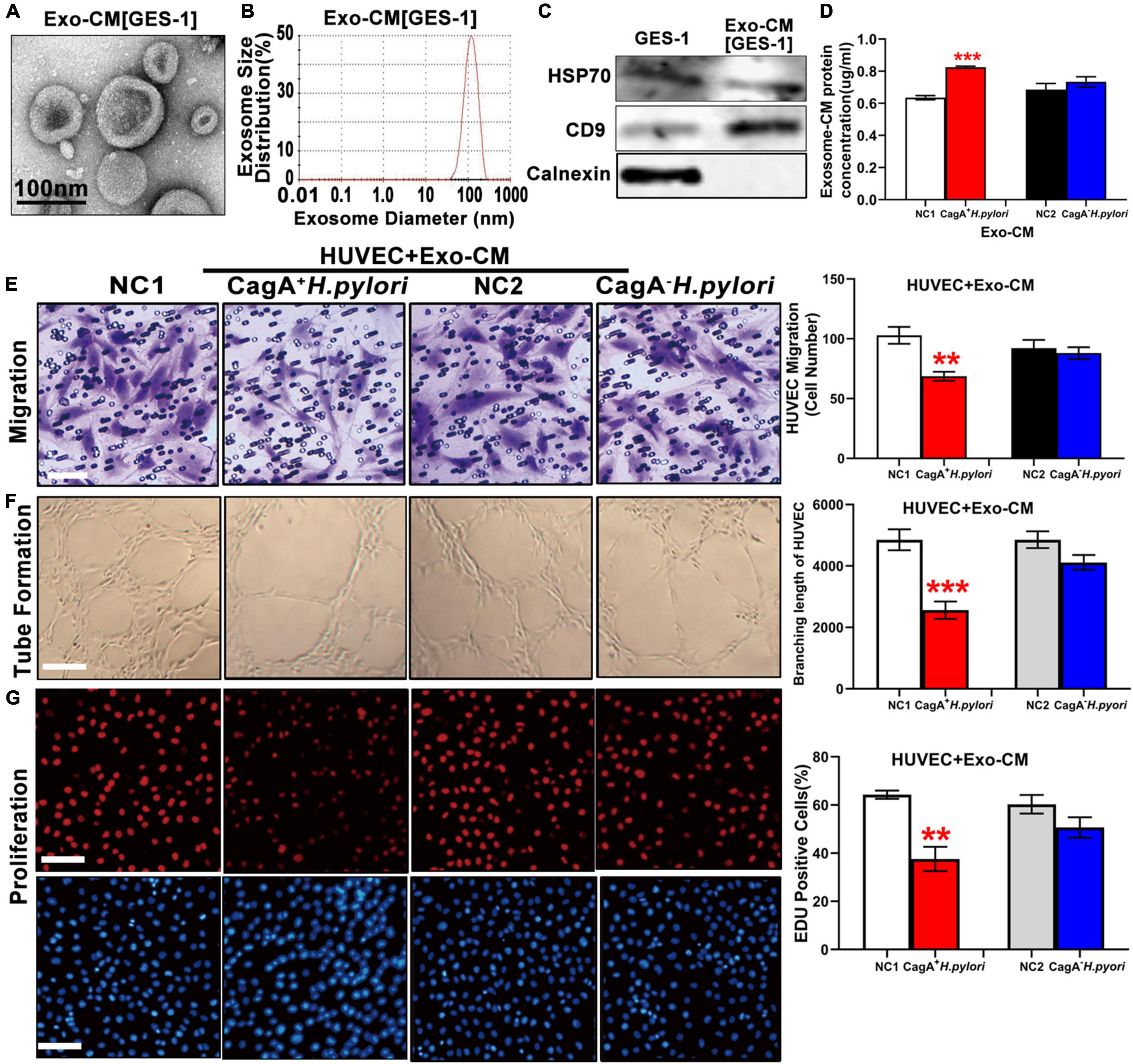
Figure 4. Exosomes from conditioned medium of human gastric epithelial cells (GES-1) cultured with CagA+ H. pylori, not with CagA– H. pylori, impaired endothelial function in vitro. Exosomes from conditioned medium of GES-1 cultured with CagA+ H. pylori exhibited typical exosome morphology (A) and size distribution (B). Western blotting analysis confirmed the presence of exosomes markers (HSP70, CD9) and absence of calnexin in exosomes (C). Exosome protein concentration was significantly higher in the conditioned medium of GES-1 cultured with CagA+ H. pylori than that cultured with CagA– H. pylori (D). Treatment of HUVECs with exosomes-CM (100 ug/ml) from CagA+ H. pylori, not from CagA– H. pylori, infected GES-1 significantly inhibited the function of HUVECs with decreased migration (E, scale bars = 25 μm), tube formation (F, scale bars = 100 μm), and proliferation (G, scale bars = 100 μm). NC(1/2): normal control; GES-1: human gastric epithelial cells; HUVEC: human umbilical vein endothelial cell; Exo-CM: Exosomes from conditioned medium. Data are presented as mean ± SEM; **P < 0.01; ***P < 0.001 by t-test. Experiment was repeated 3 times for every measurement.
CagA-Containing Exosomes Impaired Endothelial Function via Reactive Oxygen Species Production
To test the hypothesis that CagA-containing exosomes impair endothelial function via ROS-mediated mechanisms, mouse bEND.3 cells were treated with serum exosomes from mice infected with CagA+ H. pylori, or CagA– H. pylori or from non-infected control mice. HUVECs were treated with exosomes from conditioned medium of GES-1 co-cultured with CagA+ H. pylori, CagA– H. pylori or PBS. H2DCFDA assay showed that serum exosomes from mice with CagA+ H. pylori, not CagA– H. pylori infection, significantly increased intracellular ROS formation in mouse bEND.3 cells compared to exosomes from non-infected controls (Figure 5A). Similarly, exosomes from conditioned media of GES-1 co-cultured with CagA+ H. pylori, not CagA– H. pylori, significantly increased intracellular ROS levels in HUVECs (Figure 5B). CagA-containing exosomes-induced increase in intracellular ROS formation was associated with decreased endothelial function with reduced proliferation, migration, and tube formation in both bEND.3 (Figures 5C–F) and HUVECs (Figures 5G–J). NAC treatment decreased intracellular ROS production, and preserved function of bEND.3 (Figure 5C) and HUVECs (Figure 5G) in the presence of CagA-containing exosomes (Figures 5D–F,H–J). These data suggest that the effect of CagA-containing exosomes on endothelial function was indeed mediated via ROS formation.
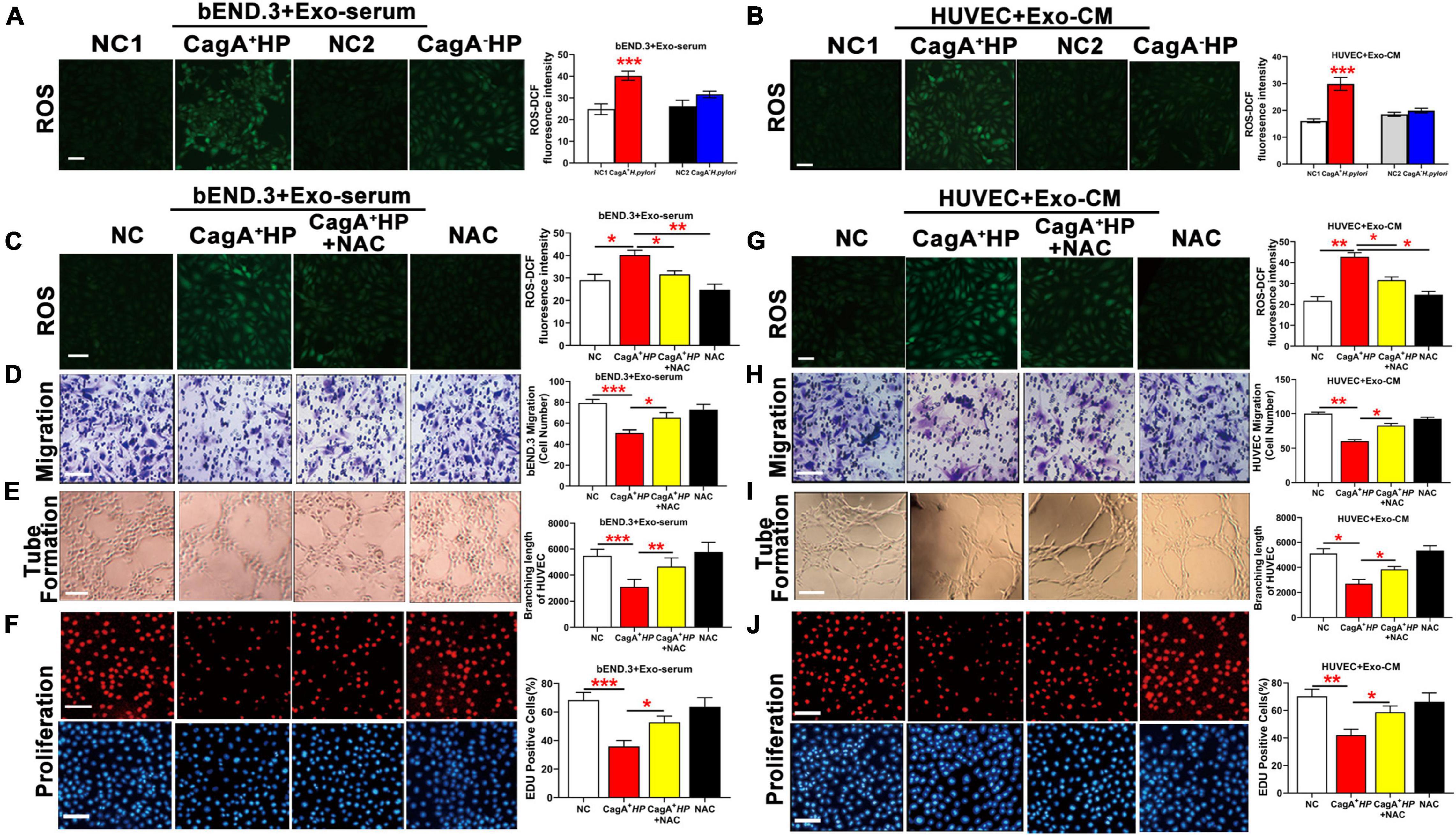
Figure 5. CagA-containing exosome impaired endothelial function via increased ROS formation. (A) Representative fluorescent images and quantification of ROS formation in bEND.3 co-cultured with serum exosomes from mice infected with CagA+ H. pylori, CagA– H. pylori or PBS. Intracellular ROS formation was significantly increased in bEND.3 co-cultured with serum exosomes from mice infected with CagA+ H. pylori, not from mice infected with CagA– H. pylori or PBS. (B) Representative fluorescent images and quantification of ROS formation in HUVECs co-cultured with exosomes from conditioned media of GES-1 cultured with CagA+ H. pylori, CagA– H. pylori or PBS. Intracellular ROS formation was significantly increased in HUVECs co-cultured with exosomes from GES-1 cultured with CagA+ H. pylori, not from GES-1 co-cultured with CagA– H. pylori or PBS. NAC treatment significantly decreased intracellular ROS production and preserved endothelial function of bEND.3 (C–F) and HUVECs (G–J) treated with CagA-containing exosomes. NC(1/2): normal control; Exo-serum: exosomes from mouse serum; Exo-CM: Exosomes from conditioned medium; CagA+ HP: CagA+ H. pylori; CagA– HP: CagA– H. pylori.; NAC: N-acetylcysteine. Data were presented as mean ± SEM; *P < 0.05; **P < 0.01; ***P < 0.001 by t-test or one-way ANOVA. Experiment was repeated 3 times for every measurement. Scale bars (all, except for D,H,E) = 100 μm. Scale bars (D,H) = 25 μm. Scale bars (E) = 10 μm.
Treatment With GW4869 Prevented CagA+ Helicobacter pylori Infection-Induced Reactive Oxygen Species Production, Endothelial Dysfunction, and Atherosclerosis
To further test the hypothesis that CagA+ H. pylori infection impairs endothelial function through CagA-containing exosomes-mediated ROS formation, C57BL/6 mice were pre-treated with GW4869 to block exosomes release in vivo. As detailed earlier, CagA+ H. pylori infection significantly increased aortic ROS level (Figure 2A) in mice in association with impaired ACh-induced relaxation (Figures 1A,C). Treatment with GW4869 significantly reduced serum exosomes levels (Figure 6A), prevented excessive aortic ROS production (Figures 6B,C) and preserved ACh-induced relaxation in mice with CagA+ H. pylori infection (Figures 6D,E).
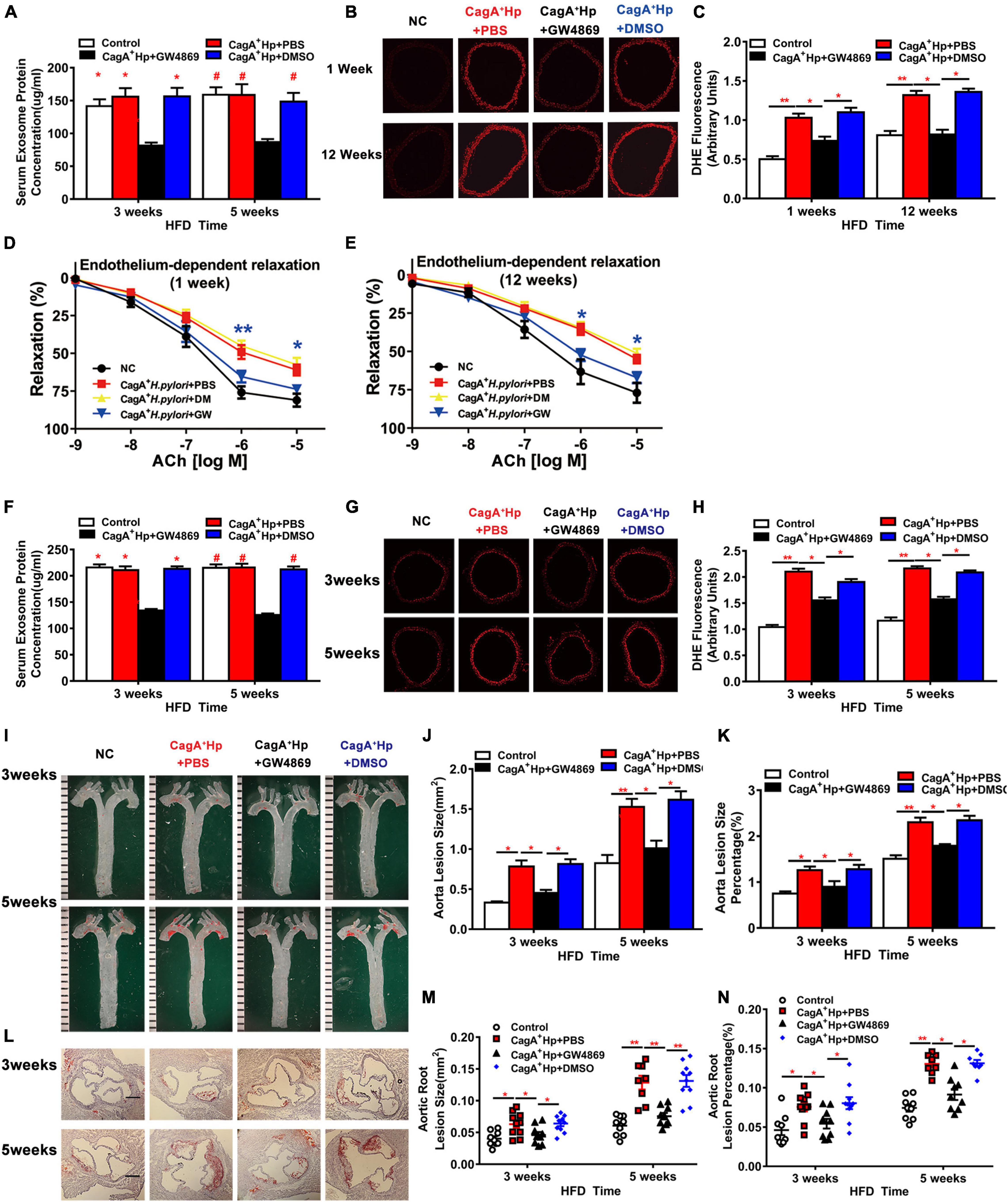
Figure 6. Blocking exosomes release with GW4869 prevented endothelial dysfunction and atherosclerosis in mice with CagA+ H. pylori infection. Treatment with GW4869 significantly decreased the serum exosome level (A) and ROS formation in thoracic aorta (B–C) with improved ACh-induced aortic relaxation (D,E) (*P < 0.05, **P < 0.01, CagA+ H. pylori vs. CagA+ H. pylori + NAC by one-way ANOVA) in C57BL/6 mice with CagA+ H. pylori infection. GW4869 treatment also significantly decreased serum exosomes level in LDLR–/– mice with CagA+ H. pylori infection (F). After 3 or 5 weeks of high-fat diet (HFD), ROS formation (G,H) and atherosclerotic plaque formation in aorta and aortic root (I–N) were significantly increased in LDLR–/–mice with CagA+ H. pylori infection that were prevented with GW4869 treatment. NC: normal control; ACh: acetylcholine; CagA+ Hp: CagA+ H. pylori; DMSO: dimethylsulfoxide (solvent for GW4869). Data are presented as mean ± SEM. *P < 0.05, **P < 0.01 by one-way ANOVA, N = 8–10 mice for each group at each time point.
Treatment with GW4869 significantly decreased serum exosomes level in LDLR–/– mice with H. pylori infection (Figure 6F), and effectively prevented excessive aortic ROS formation (Figures 6G,H). Blocking exosomes release with GW4869 also prevented CagA+ H. pylori infection-induced increases in aortic atherosclerotic burden. At three and 5 weeks of HFD feeding, there were no differences in the prevalence of aortic atherosclerotic lesion and total lesion areas between LDLR–/– mice with CagA+ H. pylori infection with GW4869 treatment and non-infected LDLR–/– control mice (Figures 6I–N). No significant effects of DMSO (vehicle for GW4869) on aortic ROS formation or aortic atherosclerotic burden were observed in hyperlipidemic LDLR–/– mice with CagA+ H. pylori infection (Figures 6A–N). Treatment with GW4869 had no significant effect on atherosclerosis in hyperlipidemic LDLR–/– mice without CagA+ H. pylori infection (Supplementary Figure 3). These data suggest that CagA+ H. pylori infection leads to significant endothelial dysfunction and increased atherosclerosis through CagA-containing exosomes-mediated ROS formation.
Discussion
The data from the present study demonstrated: (1) CagA+ H. pylori achieved gastric colonization more effectively than CagA– H. pylori; (2) CagA+ H. pylori infection induced endothelial dysfunction and promoted atherosclerosis; (3) exosomes from the serum of mice with CagA+ H. pylori infection, and exosomes from conditioned media of GES-1 co-cultured with CagA+ H. pylori significantly increased intracellular ROS and decreased endothelial function that were prevented with NAC treatment; and (4) NAC or GW4869 treatment effectively prevented aortic ROS production and aortic endothelial dysfunction in mice with CagA+ H. pylori infection. Importantly, these effects appear specific to CagA+ H. pylori and were not evident following CagA– H. pylori infection. Collectively, these data suggest that CagA+ H. pylori infection impairs endothelial function through ROS production induced by CagA-containing exosomes.
The finding that CagA+ H. pylori colonized gastric mucosa more effectively than CagA– H. pylori may provide an explanation for the clinical observation that over 90% of H. pylori patients are infected with the CagA+ H. pylori strain. Colonization in gastric epithelial cells is the critical initial event for H. pylori invasion and survival, and subsequent pathological changes in vasculature and other organ systems. With pH values as low as 1.5 – 2.5, the stomach fluid has been recognized as a natural antibiotic barrier (21). To colonize and survive in gastric mucous, bacteria have to overcome the extremely acidic and hypoxic environment. H. pylori in general has established multiple mechanisms to adapt and encounter the challenges in the stomach (22). However, CagA protein in CagA+ H. pylori may help their gastric colonization and promote persistent infection. Although some data shows that CagA+ H. pylori may be more susceptible to re-exposure to acidic environment (at pH 3.0) than CagA– H. pylori in culture, CagA expression could increase the acid-tolerance and resistance capabilities of H. pylori (23), enabling the bacteria to survive under the acidic conditions of the stomach. CagA+ H. pylori delivers CagA through a T4SS into gastric epithelial cells. The translocated CagA then dysregulates the homeostatic signal transduction of gastric epithelial cells involved in chronic inflammation and malignancy by changing cell polarity, apoptosis, and proliferation (24). In addition, CagA could activate host cell survival and antiapoptotic pathways to enhance self-renewal of gastric epithelium, helping sustain H. pylori infection (25). CagA could abrogate human β-defensin-3 expression via EGFR dephosphorylation, enhancing the ability to achieve persistent gastric infection of CagA+ H. pylori (24).
Compared to those with CagA– H. pylori infection, patients with CagA+ H. pylori infection have a much higher incidence of CVDs, including atherosclerosis (26–30). Endothelial dysfunction is a key contributing factor for CVDs including atherosclerosis. The present study showed that infection with CagA+ H. pylori, not CagA– H. pylori, significantly impaired endothelial function in C57BL/6 mice, and promoted the development of early atherosclerosis in hyperlipidemic LDLR–/– mice. Atherosclerosis is characterized by chronic inflammation with increased ROS formation (31, 32). CagA+ H. pylori infection produces persistent low-grade systematic inflammation with increased production of pro-inflammatory cytokines, including CRP, IL-6, and IL-18 (33, 34). Immune-mediated responses targeting self-antigens may play an important role in atherosclerosis (35, 36). Interestingly, CagA has been proposed as an antigen that could activate autoimmune mechanisms. The anti-CagA antibodies are able to react with both bacterial CagA and proteins in medium and large arteries (35). Thus, anti-CagA antibodies may cross-react with proteins in vascular smooth muscle cells, fibroblast-like cells, and other cells that are involved in the initiation and progression of atherosclerosis (37). In addition, gut microbiota plays an important role in the development of atherosclerosis, and there is a relationship between gastric and intestinal microbiome and H. pylori infection. It has been demonstrated that there are significant differences in the diversity and number of gut microbiota between H. pylori infected and uninfected individuals (38, 39). It will be important to determine if CagA+ H. pylori infection could impair the population and balance of gut microbiota more than CagA– H. pylori infection, thus leading to endothelial dysfunction and atherosclerosis.
The present study showed that CagA+ H. pylori, but not CagA– H. pylori, infection significantly increased the level of aortic ROS in mice. This is consistent with the concept that CagA+ H. pylori, not CagA– H. pylori, infection attenuates endothelial function and promotes the development of early atherosclerosis, and may provide an explanation for the clinical findings that patients with CagA+ H. pylori infection have significantly increased risk for atherosclerosis as compared with those with CagA– H. pylori infection. This finding is also consistent with the results from our previous study with human subjects that H. pylori infection selectively increases the risk of carotid atherosclerosis for male patients younger than 50 years of age (40). A recent study, using a large database of 208,196 patients, reveals that there is a significant decrease in composite endpoints for CAD and death for younger patients (<65 years old) with early H. pylori eradication therapy, but not for older patients (≥65 years old) or control subjects (41). Further studies are needed to address the important question why H. pylori infection selectively increases the risk of atherosclerosis for young males.
Exosomes are critically involved in cell function and disease development through direct and indirect cell-cell communications and the transfer of bioactive substances including proteins and microRNAs (42, 43). There are extensive interactions between exosomes and ROS. Exosomes can increase or decrease ROS production through various mechanisms, and ROS can regulate exosomes production and their contents as extensively summarized in a recent review by Bodega and colleagues (44). Study shows that PKH67-labeled CagA-containing exosomes readily enter HUVECs, and significantly inhibited cellular function (10). In the present study, serum exosomes from mice with CagA+ H. pylori infection, and exosomes from conditioned media of human GES-1 co-cultured with CagA+ H. pylori increased intracellular ROS production and inhibited the function of endothelial cells. In both situations, the effects of CagA+ H. pylori infection were effectively prevented with NAC treatment. Inhibition of exosomes release with GW4869 effectively prevented aortic ROS production and aortic endothelial dysfunction in mice as well as atherosclerotic burden in hyperlipidemic LDLR–/– mice with CagA+ H. pylori infection. One may argue that GW4869 is a non-specific inhibitor of exosomes release, and thus the effect of GW4869 on atherosclerosis in mice with CagA+ H. pylori infection might be non-specific. However, our previous study showed that GW4869 treatment had no effect on endothelial function in mice without H. pylori infection (10). In the present study, no significant effect of GW4869 on atherosclerosis was observed in hyperlipidemic LDLR–/– mice without CagA+ H. pylori infection. Collectively, these data support a mechanism whereby CagA+ H. pylori infection impairs endothelial function through CagA-containing exosomes-induced ROS production. Soluble components from H. pylori, including CagA, VacA, urease, and neutrophil activating factor A (NapA), could conceivably enter the circulation through exosomes, and trigger inflammatory responses and oxidative stress (45). Exosomal CagA from H. pylori-infected gastric epithelial cells has been shown to induce macrophage foam cell formation (46). Further studies are needed to define the specific molecule(s) in the exosomes that contributes to ROS production in endothelial cells and the consequent impairments in cellular function.
There are many factors that are important for H. pylori infection and the specific strains of infection, including (but not limited to) geographic locations and dietary habits. The prevalence of H. pylori infection varies significantly in the globe due to substantial differences in the population, culture, individual lifestyles, social and economic status, as well as environmental factors. Studies have shown that the prevalence of H. pylori infection are higher in Central/South America and Asia than other regions (47). However, the association between H. pylori infection and dietary habits remains inconsistent. It has been reported that intake of some uncooked vegetables and seafood, such as tomato, pepper, and mussels, correlates significantly with H. pylori infection (48). In contrast, no association is observed between H. pylori infection and intake of fruits, fish, legumes, honey, spices, meats, milk, and milk products. A cross-sectional study has shown no relationship between H. pylori infection and dietary habits (49). Some data suggest that consumption of honey and green/black tea may be associated with decreased prevalence of H. pylori infection (50). Further studies are needed to determine if diet could play a different role in the infection of CagA+ H. pylori vs. CagA– H. pylori.
It is very concerning that cardiovascular mortality has been increasing since 2010 especially for male subjects for unknown reasons (51). Studies suggest that H. pylori infection could be a significant risk factor for endothelial dysfunction, atherosclerosis, and CAD in young patients, and could provide a potential explanation for young patients who develop CAD without a clear etiology (41, 52). It is unclear why H. pylori infection does not increase the risk for atherosclerosis for patients older than 50 years. The pathophysiology of atherosclerosis is very complex and multifactorial that has not been fully understood. The data from the present study and our previous study (10) suggest that H. pylori infection could serve as a trigger to initiate the development of early atherosclerosis by compromising endothelial function at the initial phase of atherosclerosis. Other important factors including diabetes mellitus, hypertension, and hyperlipidemia may play a dominant role that could unmask the contribution of H. pylori infection to atherosclerosis in older patients. Further studies are needed to investigate the mechanism(s) on the selective effect of H. pylori infection on atherosclerosis in young populations. Clinically, it is reasonable to screen young male populations for H. pylori infection once a year and to treat them accordingly as an effective approach for early prevention of CVDs, especially premature atherosclerosis as the majority of patients with H. pylori infection are asymptomatic.
Study Limitations
The limitations in the present study include: (1) only male mice were used; (2) no studies were performed to determine the specific molecules in CagA-containing exosomes that could be primarily responsible for increasing ROS production and endothelial dysfunction and related mechanism(s); and (3) no studies were conducted to define the key pathway(s) that may significantly contribute to increased ROS levels in endothelial cells with CagA+ H. pylori infection.
Conclusion
The data suggested that CagA+ H. pylori colonized gastric mucosa more effectively than CagA– H. pylori. CagA+ H. pylori, but not CagA– H. pylori, infection induced endothelial dysfunction and promoted development of atherosclerosis through CagA-containing exosomes-mediated ROS formation.
Data Availability Statement
The raw data supporting the conclusions of this article will be made available by the authors, without undue reservation.
Ethics Statement
The animal study was reviewed and approved by the Institutional Animal Care and Use Committee of the University of Missouri School of Medicine, Columbia, MO, United States.
Author Contributions
ZL contributed to the conception and designed the study. XX, LZ, HW, FC, XL, YC, QZ, and MW contributed to the data collections and analysis. XX, LZ, HX, and WF contributed to the exosomes preparations and characterization. XX, LZ, LM-L, and MH contributed to the vascular function studies. XX and LZ drafted the manuscript. HH, D-PL, WF, LM-L, MH, CX, and ZL critically reviewed and interpreted the data and revised the manuscript. All authors contributed to the article and approved the submitted version.
Funding
This work was supported by the US National Institutes of Health grant HL148196 (ZL).
Conflict of Interest
The authors declare that the research was conducted in the absence of any commercial or financial relationships that could be construed as a potential conflict of interest.
Publisher’s Note
All claims expressed in this article are solely those of the authors and do not necessarily represent those of their affiliated organizations, or those of the publisher, the editors and the reviewers. Any product that may be evaluated in this article, or claim that may be made by its manufacturer, is not guaranteed or endorsed by the publisher.
Acknowledgments
We thank Thomas Spencer for his technical help on the characterization of exosomes.
Supplementary Material
The Supplementary Material for this article can be found online at: https://www.frontiersin.org/articles/10.3389/fcvm.2022.881372/full#supplementary-material
References
1. Mentis A, Lehours P, Megraud F. Epidemiology and diagnosis of Helicobacter pylori infection. Helicobacter. (2015) 20(Suppl. 1):1–7. doi: 10.1111/hel.12250
2. Eusebi LH, Zagari RM, Bazzoli F. Epidemiology of Helicobacter pylori infection. Helicobacter. (2014) 19(Suppl. 1):1–5. doi: 10.1111/hel.12165
3. Covacci A, Censini S, Bugnoli M, Petracca R, Burroni D, Macchia G, et al. Molecular characterization of the 128-kDa immunodominant antigen of Helicobacter pylori associated with cytotoxicity and duodenal ulcer. Proc Natl Acad Sci USA. (1993) 90:5791–5. doi: 10.1073/pnas.90.12.5791
4. Tummuru MK, Cover TL, Blaser MJ. Cloning and expression of a high-molecular-mass major antigen of Helicobacter pylori: evidence of linkage to cytotoxin production. Infect Immun. (1993) 61:1799–809. doi: 10.1128/iai.61.5.1799-1809.1993
5. Hatakeyama M. Helicobacter pylori CagA – a bacterial intruder conspiring gastric carcinogenesis. Int J Cancer. (2006) 119:1217–23. doi: 10.1002/ijc.21831
6. Shmuely H, Passaro DJ, Vaturi M, Sagie A, Pitlik S, Samra Z, et al. Association of CagA+ Helicobacter pylori infection with aortic atheroma. Atherosclerosis. (2005) 179:127–32. doi: 10.1016/j.atherosclerosis.2004.09.010
7. Gimbrone MA Jr, Garcia-Cardena G. Endothelial cell dysfunction and the pathobiology of atherosclerosis. Circ Res. (2016) 118:620–36. doi: 10.1161/CIRCRESAHA.115.306301
8. Pietroiusti A, Diomedi M, Silvestrini M, Cupini LM, Luzzi I, Gomez-Miguel MJ, et al. Cytotoxin-associated gene-A–positive Helicobacter pylori strains are associated with atherosclerotic stroke. Circulation. (2002) 106:580–4. doi: 10.1161/01.cir.0000023894.10871.2f
9. Xiong X, Chen J, He M, Wu T, Yang H. Helicobacter pylori infection and the prevalence of hypertension in Chinese adults: the Dongfeng-Tongji cohort. J Clin Hypertens (Greenwich). (2020) 22:1389–95. doi: 10.1111/jch.13928
10. Xia X, Zhang L, Chi J, Li H, Liu X, Hu T, et al. Helicobacter pylori infection impairs endothelial function through an exosome-mediated mechanism. J Am Heart Assoc. (2020) 9:e014120. doi: 10.1161/JAHA.119.014120
11. Zhang H, Deng T, Liu R, Ning T, Yang H, Liu D, et al. CAF secreted miR-522 suppresses ferroptosis and promotes acquired chemo-resistance in gastric cancer. Mol Cancer. (2020) 19:43. doi: 10.1186/s12943-020-01168-8
12. Haney MJ, Klyachko NL, Zhao Y, Gupta R, Plotnikova EG, He Z, et al. Exosomes as drug delivery vehicles for Parkinson’s disease therapy. J Control Release. (2015) 207:18–30. doi: 10.1016/j.jconrel.2015.03.033
13. Zhang L, Liu H, Jia L, Lyu J, Sun Y, Yu H, et al. Exosomes mediate hippocampal and cortical neuronal injury induced by hepatic ischemia-reperfusion injury through activating pyroptosis in rats. Oxid Med Cell Longev. (2019) 2019:3753485. doi: 10.1155/2019/3753485
14. Guo H, Zhang J, Boudreau M, Meng J, Yin JJ, Liu J, et al. Intravenous administration of silver nanoparticles causes organ toxicity through intracellular ROS-related loss of inter-endothelial junction. Part Fibre Toxicol. (2016) 13:21. doi: 10.1186/s12989-016-0133-9
15. Sayin VI, Ibrahim MX, Larsson E, Nilsson JA, Lindahl P, Bergo MO. Antioxidants accelerate lung cancer progression in mice. Sci Transl Med. (2014) 6:221ra15. doi: 10.1126/scitranslmed.3007653
16. Cui Y, Liu L, Xiao Y, Li X, Zhang J, Xie X, et al. N-acetylcysteine differentially regulates the populations of bone marrow and circulating endothelial progenitor cells in mice with limb ischemia. Eur J Pharmacol. (2020) 881:173233. doi: 10.1016/j.ejphar.2020.173233
17. Chai Y, Cao Z, Yu R, Liu Y, Yuan D, Lei L. Dexmedetomidine attenuates LPS-induced monocyte-endothelial adherence via inhibiting Cx43/PKC-alpha/NOX2/ROS signaling pathway in monocytes. Oxid Med Cell Longev. (2020) 2020:2930463. doi: 10.1155/2020/2930463
18. Centa M, Ketelhuth DFJ, Malin S, Gistera A. Quantification of atherosclerosis in mice. J Vis Exp. (2019) 148:e59828. doi: 10.3791/59828
19. Lau YS, Tian XY, Mustafa MR, Murugan D, Liu J, Zhang Y, et al. Boldine improves endothelial function in diabetic db/db mice through inhibition of angiotensin II-mediated BMP4-oxidative stress cascade. Br J Pharmacol. (2013) 170:1190–8. doi: 10.1111/bph.12350
20. Thery C, Amigorena S, Raposo G, Clayton A. Isolation and characterization of exosomes from cell culture supernatants and biological fluids. Curr Protoc Cell Biol. (2006) Chapter 3:Unit 3.22. doi: 10.1002/0471143030.cb0322s30
21. Singh A, Barnard TG. Surviving the acid barrier: responses of pathogenic Vibrio cholerae to simulated gastric fluid. Appl Microbiol Biotechnol. (2016) 100:815–24. doi: 10.1007/s00253-015-7067-2
22. Ansari S, Yamaoka Y. Survival of Helicobacter pylori in gastric acidic territory. Helicobacter. (2017) 22:e12386. doi: 10.1111/hel.12386
23. Karita M, Blaser MJ. Acid-tolerance response in Helicobacter pylori and differences between cagA+ and cagA- strains. J Infect Dis. (1998) 178:213–9. doi: 10.1086/515606
24. Backert S, Tegtmeyer N. Type IV secretion and signal transduction of Helicobacter pylori CagA through interactions with host cell receptors. Toxins (Basel). (2017) 9:115. doi: 10.3390/toxins9040115
25. Mimuro H, Suzuki T, Nagai S, Rieder G, Suzuki M, Nagai T, et al. Helicobacter pylori dampens gut epithelial self-renewal by inhibiting apoptosis, a bacterial strategy to enhance colonization of the stomach. Cell Host Microbe. (2007) 2:250–63. doi: 10.1016/j.chom.2007.09.005
26. Wang B, Yu M, Zhang R, Chen S, Xi Y, Duan G. A meta-analysis of the association between Helicobacter pylori infection and risk of atherosclerotic cardiovascular disease. Helicobacter. (2020) 25:e12761. doi: 10.1111/hel.12761
27. Rasmi Y, Rouhrazi H, Khayati-Shal E, Shirpoor A, Saboory E. Association of endothelial dysfunction and cytotoxin-associated gene A-positive Helicobacter pylori in patients with cardiac syndrome X. Biomed J. (2016) 39:339–45. doi: 10.1016/j.bj.2016.01.010
28. Gunn M, Stephens JC, Thompson JR, Rathbone BJ, Samani NJ. Significant association of cagA positive Helicobacter pylori strains with risk of premature myocardial infarction. Heart. (2000) 84:267–71. doi: 10.1136/heart.84.3.267
29. Khodaii Z, Vakili H, Ghaderian SM, Najar RA, Panah AS. Association of Helicobacter pylori infection with acute myocardial infarction. Coron Artery Dis. (2011) 22:6–11. doi: 10.1097/MCA.0b013e3283402360
30. Mayr M, Kiechl S, Mendall MA, Willeit J, Wick G, Xu Q. Increased risk of atherosclerosis is confined to CagA-positive Helicobacter pylori strains: prospective results from the Bruneck study. Stroke. (2003) 34:610–5. doi: 10.1161/01.STR.0000058481.82639.EF
31. Wu X, Zhang H, Qi W, Zhang Y, Li J, Li Z, et al. Nicotine promotes atherosclerosis via ROS-NLRP3-mediated endothelial cell pyroptosis. Cell Death Dis. (2018) 9:171. doi: 10.1038/s41419-017-0257-3
32. Kattoor AJ, Pothineni NVK, Palagiri D, Mehta JL. Oxidative stress in atherosclerosis. Curr Atheroscler Rep. (2017) 19:42. doi: 10.1007/s11883-017-0678-6
33. Ebrahimi A, Soofizadeh B, Ebrahimi F, Moaadab SY, Bonyadi M, Gojazadeh M, et al. Relationship between Helicobacter pylori cytotoxin-associated gene A protein with clinical outcomes in patients with rheumatoid arthritis. Immunol Lett. (2019) 211:49–52. doi: 10.1016/j.imlet.2019.05.014
34. Figura N, Palazzuoli A, Vaira D, Campagna M, Moretti E, Iacoponi F, et al. Cross-sectional study: CagA-positive Helicobacter pylori infection, acute coronary artery disease and systemic levels of B-type natriuretic peptide. J Clin Pathol. (2014) 67:251–7. doi: 10.1136/jclinpath-2013-201743
35. Franceschi F, Sepulveda AR, Gasbarrini A, Pola P, Silveri NG, Gasbarrini G, et al. Cross-reactivity of anti-CagA antibodies with vascular wall antigens: possible pathogenic link between Helicobacter pylori infection and atherosclerosis. Circulation. (2002) 106:430–4. doi: 10.1161/01.cir.0000024100.90140.19
36. George J, Harats D, Shoenfeld Y. Autoimmunity in atherosclerosis. The role of autoantigens. Clin Rev Allergy Immunol. (2000) 18:73–86. doi: 10.1385/CRIAI:18:1:73
37. Rozankovic PB, Huzjan AL, Cupic H, Bencic IJ, Basic S, Demarin V. Influence of CagA-positive Helicobacter pylori strains on atherosclerotic carotid disease. J Neurol. (2011) 258:753–61. doi: 10.1007/s00415-010-5824-9
38. Klymiuk I, Bilgilier C, Stadlmann A, Thannesberger J, Kastner MT, Hogenauer C, et al. The human gastric microbiome is predicated upon infection with Helicobacter pylori. Front Microbiol. (2017) 8:2508. doi: 10.3389/fmicb.2017.02508
39. Suarez-Jaramillo A, Baldeon ME, Prado B, Fornasini M, Cohen H, Flores N, et al. Duodenal microbiome in patients with or without Helicobacter pylori infection. Helicobacter. (2020) 25:e12753. doi: 10.1111/hel.12753
40. Zhang L, Chen Z, Xia X, Chi J, Li H, Liu X, et al. Helicobacter pylori infection selectively increases the risk for carotid atherosclerosis in young males. Atherosclerosis. (2019) 291:71–7. doi: 10.1016/j.atherosclerosis.2019.10.005
41. Wang JW, Tseng KL, Hsu CN, Liang CM, Tai WC, Ku MK, et al. Association between Helicobacter pylori eradication and the risk of coronary heart diseases. PLoS One. (2018) 13:e0190219. doi: 10.1371/journal.pone.0190219
42. Pegtel DM, Gould SJ. Exosomes. Annu Rev Biochem. (2019) 88:487–514. doi: 10.1146/annurev-biochem-013118-111902
43. Thery C, Zitvogel L, Amigorena S. Exosomes: composition, biogenesis and function. Nat Rev Immunol. (2002) 2:569–79. doi: 10.1038/nri855
44. Bodega G, Alique M, Puebla L, Carracedo J, Ramirez RM. Microvesicles: ROS scavengers and ROS producers. J Extracell Vesicles. (2019) 8:1626654. doi: 10.1080/20013078.2019.1626654
45. Jarzab M, Posselt G, Meisner-Kober N, Wessler S. Helicobacter pylori-derived outer membrane vesicles (OMVs): role in bacterial pathogenesis? Microorganisms. (2020) 8:1328. doi: 10.3390/microorganisms8091328
46. Yang S, Xia YP, Luo XY, Chen SL, Li BW, Ye ZM, et al. Exosomal CagA derived from Helicobacter pylori-infected gastric epithelial cells induces macrophage foam cell formation and promotes atherosclerosis. J Mol Cell Cardiol. (2019) 135:40–51. doi: 10.1016/j.yjmcc.2019.07.011
47. Peleteiro B, Bastos A, Ferro A, Lunet N. Prevalence of Helicobacter pylori infection worldwide: a systematic review of studies with national coverage. Dig Dis Sci. (2014) 59:1698–709. doi: 10.1007/s10620-014-3063-0
48. Monno R, De Laurentiis V, Trerotoli P, Roselli AM, Ierardi E, Portincasa P. Helicobacter pylori infection: association with dietary habits and socioeconomic conditions. Clin Res Hepatol Gastroenterol. (2019) 43:603–7. doi: 10.1016/j.clinre.2018.10.002
49. Assaad S, Chaaban R, Tannous F, Costanian C. Dietary habits and Helicobacter pylori infection: a cross sectional study at a Lebanese hospital. BMC Gastroenterol. (2018) 18:48. doi: 10.1186/s12876-018-0775-1
50. Boyanova L, Ilieva J, Gergova G, Vladimirov B, Nikolov R, Mitov I. Honey and green/black tea consumption may reduce the risk of Helicobacter pylori infection. Diagn Microbiol Infect Dis. (2015) 82:85–6. doi: 10.1016/j.diagmicrobio.2015.03.001
51. Tsao CW, Aday AW, Almarzooq ZI, Alonso A, Beaton AZ, Bittencourt MS, et al. Heart disease and stroke statistics-2022 update: a report from the American heart association. Circulation. (2022) 145:e153–639. doi: 10.1161/CIR.0000000000001052
Keywords: CagA, Helicobacter pylori, endothelial dysfunction, atherosclerosis, exosomes, reactive oxygen species
Citation: Xia X, Zhang L, Wu H, Chen F, Liu X, Xu H, Cui Y, Zhu Q, Wang M, Hao H, Li D-P, Fay WP, Martinez-Lemus LA, Hill MA, Xu C and Liu Z (2022) CagA+ Helicobacter pylori, Not CagA– Helicobacter pylori, Infection Impairs Endothelial Function Through Exosomes-Mediated ROS Formation. Front. Cardiovasc. Med. 9:881372. doi: 10.3389/fcvm.2022.881372
Received: 22 February 2022; Accepted: 08 March 2022;
Published: 31 March 2022.
Edited by:
Hong Chen, Boston Children’s Hospital and Harvard Medical School, United StatesReviewed by:
Kalpna Gupta, University of California, Irvine, United StatesLiya Yin, Northeast Ohio Medical University, United States
Copyright © 2022 Xia, Zhang, Wu, Chen, Liu, Xu, Cui, Zhu, Wang, Hao, Li, Fay, Martinez-Lemus, Hill, Xu and Liu. This is an open-access article distributed under the terms of the Creative Commons Attribution License (CC BY). The use, distribution or reproduction in other forums is permitted, provided the original author(s) and the copyright owner(s) are credited and that the original publication in this journal is cited, in accordance with accepted academic practice. No use, distribution or reproduction is permitted which does not comply with these terms.
*Correspondence: Zhenguo Liu, bGl1emhlbmdAaGVhbHRoLm1pc3NvdXJpLmVkdQ==
†These authors have contributed equally to this work and share first authorship