- 1Laboratory for Regenerative Tissue Repair, Texas Children's Hospital, Houston, TX, United States
- 2Center for Congenital Cardiac Research, Texas Children's Hospital, Houston, TX, United States
- 3Division of Congenital Heart Surgery, Texas Children's Hospital, Houston, TX, United States
- 4Department of Surgery, Baylor College of Medicine, Houston, TX, United States
- 5Division of Pediatric Surgery, Department of Surgery, Texas Children's Hospital, Houston, TX, United States
- 6Department of Mechanical Engineering, Kennesaw State University, Marietta, GA, United States
- 7Division of Pediatric Cardiology, Texas Children's Hospital, Houston, TX, United States
Hypoplastic left heart syndrome (HLHS) is a complex congenital heart condition in which a neonate is born with an underdeveloped left ventricle and associated structures. Without palliative interventions, HLHS is fatal. Treatment typically includes medical management at the time of birth to maintain patency of the ductus arteriosus, followed by three palliative procedures: most commonly the Norwood procedure, bidirectional cavopulmonary shunt, and Fontan procedures. With recent advances in surgical management of HLHS patients, high survival rates are now obtained at tertiary treatment centers, though adverse neurodevelopmental outcomes remain a clinical challenge. While surgical management remains the standard of care for HLHS patients, innovative treatment strategies continue to be developing. Important for the development of new strategies for HLHS patients is an understanding of the genetic basis of this condition. Another investigational strategy being developed for HLHS patients is the injection of stem cells within the myocardium of the right ventricle. Recent innovations in tissue engineering and regenerative medicine promise to provide important tools to both understand the underlying basis of HLHS as well as provide new therapeutic strategies. In this review article, we provide an overview of HLHS, starting with a historical description and progressing through a discussion of the genetics, surgical management, post-surgical outcomes, stem cell therapy, hemodynamics and tissue engineering approaches.
Introduction
Hypoplastic left heart syndrome (HLHS) is a congenital heart condition in which a pediatric patient is born with an underdeveloped left ventricle and associated structures (1). This condition affects ~1,000 patients annually in the US (1). If not aggressively treated and managed at the time of birth, HLHS is fatal (1). There have been excellent reviews in recent literature covering specific topics related to HLHS, to include stem cell therapy (2, 3), regenerative medicine approaches (4, 5), tissue engineering strategies (6), and treatment approaches (7). These review articles each provide an excellent overview of a very focused area related to HLHS. The current review serves to provide a comprehensive overview of HLHS, starting with a historical perspective, followed by a review of genetics, stem cell therapy, clinical outcomes, neurodevelopmental aspects, hemodynamics, and proposed tissue engineering therapies.
Surgical management of HLHS patients
Since the pioneering work by Dr. Norwood and the development of the staged palliative surgical approach (8), high survival rates are now accomplished in tertiary treatment centers (9). HLHS can be diagnosed in utero during a routine fetal echocardiography as early as the second trimester and allows for surgical planning at the time of birth (7). At the time of birth, a series of medical management strategies are used to stabilize the patient prior to surgical intervention, as described below.
One of the first tasks is stabilization with prostaglandins to maintain ductal patency. During fetal circulation, the placenta is the primary source of oxygen-exchange with the maternal circulation (10). Since the fetus does not rely on the lungs for oxygen, the ductus arteriosus shunts the blood away from the lungs and provides a flow pathway from the pulmonary artery directly to the descending aorta (10). After birth, this circulation changes and the ductus arteriosus rapidly begins and the pulmonary vascular resistance falls and the neonate now relies on the pulmonary circulation for oxygenation (10). However, in HLHS hearts, prograde aortic flow is minimal or absent and the right side of the heart delivers the majority of the cardiac output to the lungs and through the ductus arteriosus to the body (7). Pharmacologically maintaining patency of the ductus arteriosus with infusion of prostaglandin E1 (PGE-1), a naturally occurring prostaglandin and a known vasodilator, is required to maintain patency of the ductus arteriosus at the time of birth prior to surgical intervention (10).
Once the patient has been stabilized through the use of PGE-1, the next steps include three palliative interventions, the first stage palliation, the second stage palliation and the Fontan procedure (7). Surgical intervention was developed on the philosophy that the right side of the neonatal heart can support both systematic and pulmonary circulation. To achieve this surgically, the right side of the neonatal heart must deliver blood to the lungs for oxygenation and through the systematic circulation to the whole body. A series of staged palliative surgeries were developed to accomplish this task by Dr. Norwood; they were published in his landmark paper in 1983 (8) and were described in an earlier section of this review.
The characteristics of HLHS is underdeveloped left heart structures including the mitral valve, left ventricle, aortic valve, and ascending aorta and aortic arch (Figures 1A,B), all of which severely limit the ability of the neonatal heart to support systematic circulation (7). The most immediate need is to support both systematic and pulmonary circulation independent of the ductus arteriosus and develop a patent aorta for blood flow through the RV. The four basic objectives of first stage palliation are to: 1. Provide unobstructed systemic cardiac output; 2. Provide a controlled source of pulmonary blood flow; 3. Provide a reliable source of coronary blood flow; 4. Provide unobstructed egress of blood from the pulmonary veins (Figures 1C,D) (8). During the Norwood, the main pulmonary artery is divided amalgamated with the ascending aorta and the reconstructed aortic arch to provide cardiac output to the body and a reliable source of coronary circulation (8). The second objective achieved with the Norwood is to provide a controlled source of pulmonary blood flow which can be provided by a modified Blalock-Taussig-Thomas (mBTTS) shunt, which directs blood from the innominate artery to the pulmonary artery (Figure 1C) (11, 12). An alternative is the Sano shunt, which provides pulmonary blood flow through a conduit between the RV and the pulmonary artery (Figure 1D) (13). These steps result in a parallel circulation where the cardiac output is divided between the systemic and pulmonary circulations. During the Norwood procedure, the atrial septal defect is enlarged to ensure unobstructed egress of oxygenated blood from the left atrium to the right atrium. In addition, the atrial septal defect is enlarged to ensure unobstructed egress of oxygenated blood from the left atrium to the right atrium (14).
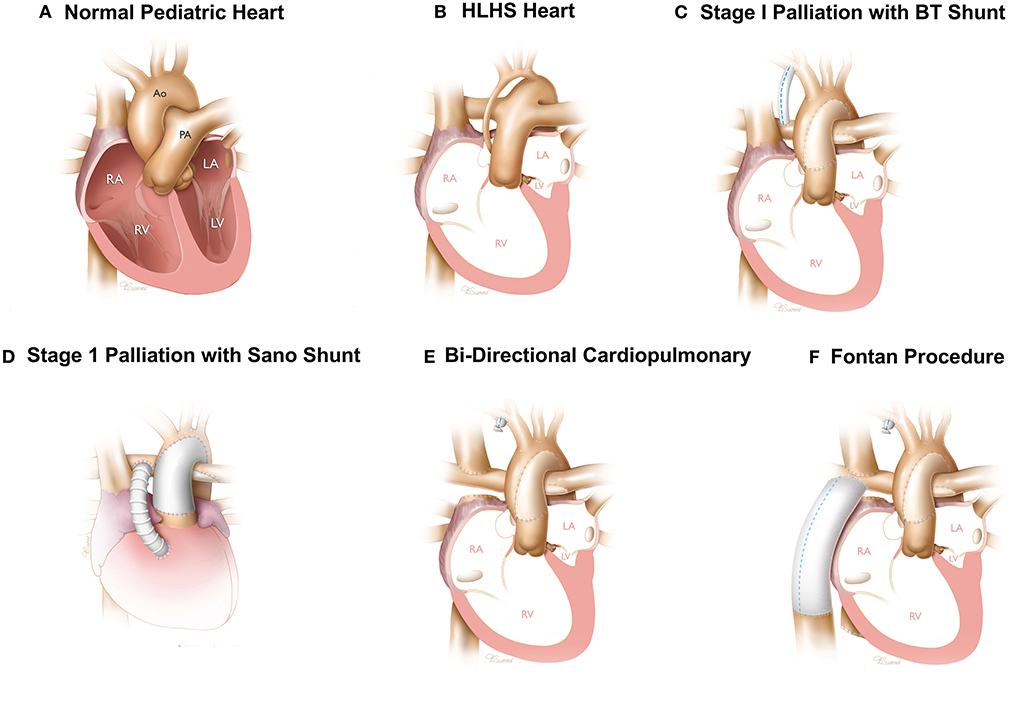
Figure 1. Surgical reconstruction of HLHS hearts—Normal (A) and HLHS pediatric heart (B). Three stage surgical reconstruction starting with stage one palliation using a BT shunt (C) or sano-shunt (D), bidirectional cavopulmonary shunt (E), and Fontan procedure (F). Images printed with permissions from Texas Children's Hospital.
The second surgery, known as the bidirectional cavopulmonary shunt, is performed about 4–6 months after the Norwood procedure (15). The objective of the Glenn procedure is to reduce the load on the RV to support both the pulmonary and systematic circulation through the Norwood (15). During the Glenn procedure, the superior vena cava (SVC) is divided from the right atrium and anastomosed directly to the pulmonary artery (Figure 1E) (15). This provides a pathway for venous blood from the upper extremities and head to be directed to the pulmonary circulation directly, thereby unloading the RV of this burden achieved by converting an in-parallel circulation to an in-series circulation. The objective of the second stage procedure is to maintain oxygenation and to convert from an in-parallel to an in-series circulation as an interim step prior to a Fontan procedure.
The third surgery is known as the Fontan (16) and performed 3–4 years after the Glenn procedure. During the Fontan surgery, the inferior vena cava (IVC) is divided and anastomosed to the pulmonary artery using a conduit or a tunnel comprised of a patch and the lateral wall of the right atrium. In both configurations, the physiologic result is inclusion of venous blood flow from the IVC into the pulmonary circulation (Figure 1F) (16).
While the staged palliation surgical management approach is the most common, alternative strategies are also used. A hybrid approach has been used and, in some cases, HLHS patients are directly listed for a heart transplantation, though with the scarcity of donor organs, this strategy becomes challenging. Mechanical support devices have also been used as a bridge to transplantation.
Genetic basis of HLHS
Genetic studies can be grouped into three categories and are discussed in subsequent sections: (1) Linkage analysis and heritage analysis mapping HLHS to specific regions of the chromosomes. (2) Mutation analysis to identify individual genes responsible for HLHS. (3) Use of induced pluripotent stem cells to elucidate the genetic basis of HLHS.
Linkage analysis of HLHS patients
Linkage analysis is a powerful tool that identifies the chromosomal location of genes that are responsible for a particular disease (17). This technique has been used in the case of HLHS and several examples are presented here. In one study involving 353 patients, linkage analysis was used to connect the inheritance of bicuspid aortic valve (BAV) dysfunction, a common disorder observed in congenital heart patients, including HLHS, to chromosomes 18q, 5q, and 13q, suggesting the presence of genes whose mutations are responsible for BAV dysfunction (18). Another study, also relying on linkage analysis using 289 patients, demonstrated chromosomal linkage of HLHS abnormalities to chromosome 2p15 (19). Additional studies provided evidence and demonstrated that HLHS was linked to mutations in chromosomes 10q22 and 6q (20) and 21q22.3 (21) and also 11q23 deletion (22). While there is no doubt that linkage analysis has provided valuable insights into the chromosomal locations of mutations related to HLHS, such a strategy has done little to develop a pathway for therapeutic approaches. This strategy was used during an earlier timeframe, prior to the recent and powerful advances in RNA-seq, single-cell seq, and other related strategies that can identify individual genes responsible for HLHS.
Mutation analysis to identify individual genes responsible for HLHS
Identifying individuals and families of genes responsible for the pathophysiology of HLHS is critical in developing effective therapeutic strategies to treat this patient population. While there is now an abundance of rich literature describing many different gene candidates, the molecular mechanisms leading to HLHS remain poorly studied and understood. One of the earliest reports observed in HLHS patients showed mutations in connexin43 (23). In this study, HLHS patients undergoing heart transplant were analyzed based on PCR analysis of tissue biopsies obtained at the time of transplant. In this group of patients, eight out of 14, or 57.1%, had mutation in connexin43 (23). Given the critical role of connexin43 in intercellular communication, this result was not surprising (24). In addition, NOTCH1 (25–28) and NKX2.5 mutations (29), deletion of ETS1 (30), impaired adrenergic signaling (31, 32), and upregulation of cTnI (33) have all been linked to HLHS. In another study, 87,355 chemically mutagenized mice were screened and whole exome sequencing was performed, identifying 91 recessive mutations in 61 genes that included 34 cilia-related genes and 16 genes involved in cilia transduced cell signaling (34), highlighting the role of the cilia mutations in congenital heart disorders. In yet another recent study, mouse forward genetics was used to link Sap130 and Pcdha9 in mediating left ventricle hypoplasia and increased penetrance of aortic valve abnormalities, both of which are associated with HLHS (35). Changes in micro-RNA expression has been implicated in HLHS (36), including upregulation of miR-486 in certain cases (37). In addition, one study performed a genome-wide exon array analysis to determine differentially expressed genes and alternatively spliced transcripts in the right ventricle (RV) of six neonates with HLHS, compared to the RV and left ventricle (LV) from non-diseased control subjects (38). In HLHS, more than 180 genes were differentially expressed and 1,800 were differentially spliced, leading to changes in a variety of biological processes involving cell metabolism, cytoskeleton, and cell adherence (38).
HLHS is caused by multiple genes
Recent literature suggests that HLHS is caused by mutations in multiple genes and is not the result of a single mutation only. Examples of genes shown to be cause HLHS include HAND1, GJA1, ZIC3, NKX2.5, NOTCH1, MCTP2, and MYH6 (39). The exact nature of the relative contribution of these genes and how they affect the etiologies observed in HLHS, it is becoming increasing evident that a multitude of genes acting in tanden lead to the anatomical abnormalities seen in HLHS (39).
Single cell analysis
More recent work has focused on single cell analysis and 3D patch engineering to decipher the role of various genes in HLHS (40). The single cell analysis showed significant changes in many pathways related to cardiomyocyte contraction, heart development, striated muscle differentiation, and cytoskeleton organization. Deficiencies in heart muscle contractility were shown based on work using 3D patches (40). Overall, this work provided a broader perspective of the genetic basis of HLHS showing that a family of genes are responsible, rather than a single gene mutation.
Induced pluripotent stem cells and genetics of HLHS
Induced pluripotent stem cell derived cardiomyocytes (iPSC-CMs) have been used extensively as a disease model in many cardiac disorders (41), though not extensively in the congenital space and much less so in the study of HLHS. In one study, iPSC-CMs were used to demonstrate the role of hypoxia in the development of HLHS through upregulation of the master regulator hypoxia inducible factor (HIF-1α), oncogene-associated cellular senescence, TGF-β1-associated fibrosis and impaired vasculogenesis (42). In a more recent study, whole genome sequencing of iPSC-CMs from three related individuals, identified LDL receptor-related protein LRP2 as a key modulator of cardiomyocyte proliferation and development during embryogenesis, mutagenesis of which could lead to HLHS (43).
Future studies
The genetic basis of HLHS has been an area of investigation, though a clear signaling pathway that leads to the anatomical malformations of the fetal heart is yet to be established. Understanding the genetic basis of HLHS and the role of hemodynamics is essential to develop effective therapeutic strategies. The current body of literature lacks a cohesive understanding of the genetic basis of HLHS; rather, alterations in the expression of a few select genes are linked to HLHS. The current set of models used to study HLHS development will need to be expanded, and recent advances in bioengineering approaches related to iPSC-CMs and patch engineering will prove to be valuable in these studies.
iPSC-CMs technology has not been leveraged to its full potential as a tool to significantly understand the key modulators of HLHS. The main advantage of iPSC-CMs is the ability to obtain patient-specific information on the genetic modulators of HLHS. In addition, iPSC-CMs can be subjected to known master regulators of HLHS, like volume overload and cyanosis, and the responsiveness of these cells can be characterized in an isolated in vitro monolayer culture system. The ability to bioengineer three-dimensional heart muscle from these cells further adds to the advantages of this technology as the genetic modulators and response to external stimuli can now be investigated in three-dimensional tissue (44).
HLHS outcomes
Table 1 includes a list of reports describing 1-year mortality after the Norwood procedure (9, 45–57). Scanning Table 1 provides some insight into post-surgical outcomes for HLHS patients. A review of the data shows large variations in outcomes with no clear trends. The range of mortalities is broad, from a low of 15% from 157 patients during the period 1996–2007 at University Hospital Schleswig-Holstein in Germany (52) to a high of 60% from 129 patients during the period 1983–2004 at Royal Children's Hospital in Australia (50). Based on an analysis of the outcomes data presented in Table 1, the HLHS patient survival 1 year after stage one palliation surgery has improved significantly in the current decade compared with survival 1990–2010. Further advances in management of HLHS include stem cell therapies and bioengineering solutions, which have the potential to increase survival of HLHS patients. While surgical management will continue to be the standard of care for HLHS patients in the near-term, use of innovative therapies (stem cell therapy and bioengineering) are essential tools in increasing patient survival and improving outcomes.
HLHS cavopulmonary hemodynamics
The selection of the optimal shunt during the Norwood procedure and the construction of the optimal cavopulmonary connection configuration during the Glenn and Fontan surgeries rely on multiple patient-specific factors such as RV function, cardiac and vessel anatomies, inflow conditions and respiration rates, and their relations with the systemic and pulmonary flow dynamics generated at each surgical stage (58). Experimental approaches based on state-of-the-art in vitro flow diagnostic techniques, as well as computational fluid dynamics (CFD) strategies combining patient-specific flow models with lumped parameter networks have been successfully implemented to improve surgical planning in HLHS patients (59). As a result, the bulk of the literature published to date on HLHS hemodynamics has mostly focused on the extracardiac hemodynamics generated by the different palliative surgical stages and the use of this knowledge in surgical planning. In contrast, the pre- and post-surgical intracardiac hemodynamics of the single RV, which are key predictors of HLHS patient survival (60), remain largely unexplored. This section describes the important contributions made to the characterization of HLHS hemodynamics to date.
Norwood hemodynamics
While post-operative complications of the Norwood procedure may be linked to pre-operative patient characteristics (e.g., presence of non-cardiac/genetic abnormalities, weight <2.5 kg, right dominant single ventricle) (61), the specific hemodynamics and flow resistance of the systemic-pulmonary arterial shunt has also been suggested as an important prognostic factor for patient survival. As demonstrated in an early in vitro study, which evaluated pressure-flow relationships in systemic-to-pulmonary Blalock-Taussig shunts of different diameters, an increase in shunt diameter resulted in reduced pressure gradients at both the proximal and distal anastomoses (62). More recently, a computational parametric investigation conducted in an idealized Blalock-Taussig shunt geometry revealed that not only shunt diameter but also anastomosis placement was critical to oxygen delivery to both systemic and coronary circulations (63). Lastly, multiscale computational flow modeling has also been used to compare the hemodynamic performance of different shunt configurations. The simulations suggested that the Sano shunt consistently generated more favorable hemodynamics (i.e., lower RV systolic and diastolic pressures, lower pulmonary-to-systemic flow ratios, and higher coronary perfusion pressure).
The surgical management of HLHS consists of redirecting the venous deoxygenated blood toward the lungs while bypassing the RV via a staged surgical approach. A challenge raised by the construction of this bypass is the requirement to achieve a balanced flow split between both lungs while minimizing flow energy loss. The dominant objective in single ventricle management is to achieve the Fontan circulation, which depends upon kinetic energy to propel blood through the lungs without a sub-pulmonary ventricle. The concept of energy is critical to the success of this staged palliative surgery. In fact, the single RV must eject blood with sufficient kinetic energy to overcome energy losses caused by the viscous friction along the entire vasculature and the hemodynamic disturbances (e.g., mixing, collision, separation, and recirculation) generated at each stage of the surgical cardiac and vascular reconstructions (64). Therefore, optimization of the staged surgery is critical to produce efficient hemodynamics necessary for positive long-term clinical outcomes (65).
Glenn and Fontan hemodynamics
The hemodynamics of the Glenn and Fontan surgical reconstructions have been documented in many in vitro and CFD studies. In vitro measurements in realistic glass models mimicking a bidirectional Glenn cavopulmonary connection suggested the benefits of this connection over a dilated atriopulmonary connection by demonstrating its ability to reduce fluid energy dissipation, achieve physiologic distribution of total flow, and maintain some hepatic venous flow to each lung (66). Pulsatile flow simulations in hemi-Fontan and bidirectional Glenn geometries reconstructed from magnetic resonance, angiocardiogram, and echocardiogram anatomic data complemented the previous experimental findings by indicating no substantial difference in power loss and flow distribution to each lung (67).
However, the bulk of the literature published to date on Glenn and Fontan hemodynamics has focused on the identification of key geometrical parameters affecting the hydraulics of the Fontan total cavopulmonary connection (TCPC). Experimental particle image velocimetry measurements in realistic stereolithography intra-atrial connection models have revealed the existence of complex, unsteady, and highly three-dimensional flow structures, suggesting a substantial degree of energy loss in this type of connection (68). Those results are supported by 4D-flow MRI and patient-specific CFD studies, which have evidenced the existence of high vorticity magnitudes and atrial recirculation in the intra-atrial connection (69), and the hydraulic superiority of the lateral tunnel Fontan operation relative to any other method (67).
Another important finding suggested by the literature on TCPC hemodynamics is the stronger dependence of energy loss on the TCPC topology and geometrical features than on the Fontan surgical option. In fact, a retrospective analysis of CFD and in vitro flow data revealed that the minimum cross-sectional area of the pulmonary arteries at the TCPC outlets was a stronger predictor of energy loss characteristics than the surgical procedure (extra- vs. intra-cardiac conduit) (70). Another CFD analysis using realistic pulsatile flow boundary conditions and TCPC geometries featuring extra- and intra-cardiac conduits demonstrated that power dissipation was primarily influenced by the actual cross-sectional area of the inferior vena cava anastomosis (71). Lastly, steady flow pressure measurements and flow visualization conducted in idealized TCPC glass models suggested that caval offsets and anastomotic flaring could reduce the hydraulic power loss by half relative to no offset and by at least 45% relative to no flaring, respectively (72, 73). Altogether, those studies suggest that patient-specific flow modeling could be an effective surgical planning tool toward the improvement of HLHS patient outcome and the reduction of surgical risks (74).
RV hemodynamics during staged palliation
While the re-engineering of the right side of the heart is a critical requirement to support both pulmonary and systematic circulations in HLHS patients (7), RV function is a key determinant of HLHS patient survival (60). Mathematical and computational approaches with various degrees of sophistication have been proposed to estimate RV hemodynamics and function in HLHS. Flow simulations in a post-Norwood RV geometry confirmed the hemodynamic superiority of the Sano shunt over the Blalock-Taussig shunt, as suggested by the predicted reduction in RV workload and no substantial difference in systemic blood flow (75). Another computational model based on patient-specific RV anatomies reconstructed from magnetic resonance imaging revealed the strong dependence of interventricular pressure gradients, filling dynamics and capacity on RV shape and temporal deformation patterns (76). To date, the only quantification of the native pre-surgical HLHS RV hemodynamics was performed numerically in a fetal heart in the context of cardiac development (77). This investigation, which assessed fetal blood flow using 4D spatiotemporal image correlation ultrasound and numerical modeling, revealed that despite a larger right-ventricular cavity size and a greater cardiac output, HLHS fetal hearts generated essentially the same global interventricular hemodynamics as a normal heart.
Future studies
The synergies suggested by previous RV studies between interventricular RV flow dynamics, cardiac function, and TCPC power loss suggest that the knowledge of pre- and post-surgical RV hemodynamics on a patient-specific basis could guide clinical decision making and promote patient survival (78). However, determining the exact role played by RV hemodynamics in the long-term outcome of the right heart surgical reconstruction requires the assessment of the hemodynamic alterations experienced by the RV during the course of the staged Fontan palliation. While this knowledge gap has not been addressed yet, computational strategies have been designed and successfully implemented to assess the potential mechanical changes of other cardiac defects such as discrete subaortic stenosis (DSS) (79–81). Cine-magnetic resonance imaging data was used in tandem with state-of-the-art fluid-structure interaction modeling to predict native blood flow patterns in patient-specific left-ventricular models featuring normal and DSS-prone outflow tract anatomies, and to characterize the resulting myocardial mechanical stresses. A similar modeling strategy could be deployed to elucidate RV hemodynamics in patient-specific post-Norwood RV anatomies, and to identify particular blood flow patterns and mechanical stresses associated with good clinical outcomes.
Stem cell therapy in HLHS patients
Stem cell therapy is conceptually based on the idea of delivery of isolated cells to the region of injury in an attempt to promote repair and/or regeneration (82). Stem cell therapy has been evaluated in many different fields, particularly in the realm of myocardial infarction in the adult population (83). In comparison, there is a much smaller, though growing body of literature, with varying degree of success, in the pediatric congenital heart field, particularly related to HLHS patients (84–89). Important considerations in stem cell therapy are the source of cells, bone marrow derived mesenchymal stem cells (BMMSCs) being a common choice, mode of delivery (intra-muscular vs. intra-coronary), the number of cells, and timing of stem cell delivery relative to disease progression. Our discussion on this topic is divided into the following sections: (1) lessons learnt from stem cell therapy in adult heart patients, (2) stem cell therapy for pediatric HLHS patients, (3) summary and future perspective.
Lessons learnt from stem cell therapy in the adult heart
Stem cell therapy for myocardial infarction in adult hearts is now a large and expansive field, with numerous lessons being learnt (82). However, our goal is to focus this discussion on the potential mode of action of injected cells. How are the injected cells acting on the host tissue to provide a functional benefit? Initially, it was hypothesized that injected cells would integrate with host myocardium, transform to become contracting cardiomyocytes, electromechanically couple with host cardiomyocytes, and provide direct functional improvement to an otherwise failing heart (82). Conceptually, this was the basis of the field and if realized, would truly be revolutionary in the field of adult cardiac regeneration. However, this was not the case for many reasons, perhaps the most significant of which was the low rate of local cell retention: <5% of injected cells were retained at the site of injury (90). Irrespective of the challenges in the field, many lessons have been learnt regarding the potential mode of action of injected stem cells. It is now hypothesized that injected stem cells act through one of several mechanisms, including the release of paracrine signaling factors, promoting neovascularization and/or recruitment of resident or circulating stem cells, all of which serve to either increase the number of functional cardiomyocytes or promote neovascularization (90). With such a rich literature in the adult cardiac stem cell transplantation space, it provides a strong background to initiate similar studies in the pediatric congenital cardiac space, particularly related to HLHS.
Stem cell therapy for pediatric HLHS patients
Many of the factors discussed earlier apply to stem cell therapy for HLHS patients, to include the source and number of cells and mode of delivery. However, specific to HLHS patients is the timing of stem cell delivery. The surgical palliation of HLHS is a complex three-stage process, progressing through the Norwood, Glenn, and Fontan surgeries. Therefore, the timing of stem cell delivery becomes crucial and most studies making use of stem cell therapy have been at the time of stage two palliation.
Table 2 provides an overview of several studies describing stem cell therapy in HLHS patients, and these are discussed in subsequent sections. Table 2 is designed to serve as a survey of the recent literature on stem cell therapy in HLHS, rather than an exhaustive list of all published studies. Earlier studies in 2010 (89) and 2015 (87) were designed to provide safety of injected cells in single HLHS patients at the time of the Glenn surgery, followed by the Phase I TICAP trial in 2015 (87) and Phase II PERSEUS trial in 2017 (86), all of which are discussed below.
One of the earlier case studies was described in 2010, in which intracoronary injection of bone marrow mesenchymal stem cells (BMMSCs) during stage II procedure in a single pediatric HLHS patient proved to be safe (89). This was an initial proof of concept study to demonstrate the feasibility of BMMSCs at the time of Glenn, without much detail to any potential functional benefit and/or mode of action. While the results of this study demonstrated safety of BMMSCs delivery in a single HLHS patient at the time of Glenn, many unanswered questions remained. A second case study was published in 2015, using mononuclear cells from umbilical cord blood, again injected during stage II surgery, increasing RV ejection fraction from 30–35 to 50% (84).
Phase I results of the TICAP trial were published in 2015, making use of intracoronary infusion of cardiac progenitor cells (CPCs) in 14 patients, seven undergoing staged palliation with CPCs infusion and an equal amount with only staged palliation (87). Patients treated with CPCs showed RV ejection fraction improvement from baseline to 3-month follow-up (46.9 ± 4.6% to 52.1 ± 2.4%; P = 0.008) (87). Compared with controls at 18 months, cardiac MRI analysis of CPC-treated patients showed a higher right ventricular ejection fraction (31.5 ± 6.8% vs. 40.4 ± 7.6%; P = 0.049) (87). This was followed up by a Phase II clinical trial, PERSEUS with 41 patients; at 3 months, the absolute changes in ventricular function were significantly greater in the CPC-treated group than in the controls (35.3%+/-9.2% to 41.7%+/-7.4%; P = 0.0002) (86).
Another recent study made use of autologous bone marrow derived mononuclear cells administered via cardiac catheterization to the coronary circulation in a single patient 23 years after the Fontan Surgery, showing decrease in ventricular size 3 months after cell injection (88).
A more recent study published in 2019 showed the feasibility of intramyocardial injections of mononuclear cells derived from umbilical cord bloods in a Phase I clinical trial with 10 patients, though there were no functional benefits resulting from this intervention (85).
Summary and future perspective
Stem cell therapy in HLHS patients is at a stage of infancy, and very few studies have been conducted in a very small patient population. The mode of delivery has been either intracoronary or intramyocardial, and the number and source of cells has varied. The time of delivery has been consistent, with most studies conducted at the time of the second stage palliation. Source and number of cells, along with the timing of delivery, must be optimized, and the modes of action must be elucidated. Furthermore, a combinational therapy consisting of stem cells coupled with growth factors to increase the contractile function of the RV may also prove to be beneficial.
Tissue engineering and regenerative medicine
Recent advances in bioengineered functional cardiovascular tissue have provided a novel opportunity for new technologies, both to increase our understanding of HLHS and to provide therapeutic options. The definition of tissue engineering has been very elegantly presented in a recent publication (91): Tissue engineering is a multidisciplinary field bringing together experts from engineering, life sciences and medicine, utilizing the building blocks of cells, biomaterials and bioreactors for the development of three-dimensional artificial tissue and organs which can be used to augment, repair and/or replace damaged and/or diseased tissue. Or in simpler terms, tissue engineering is the field focused on fabricating three-dimensional tissue, and applied to the cardiac space, this includes heart muscle (92), ventricles (93), Purkinje networks (94), and whole hearts (95).
The tissue fabrication process has been presented in a very methodological manner in a recent publication (96). In summary, iPSCs are generated from peripheral blood mononuclear cells, isolated from a routine blood draw and converted to cardiomyocytes (iPSC-CMs), using an established protocol (97). iPSC-CMs are cultured within a 3D matrix, resulting in the formation of contractile heart muscle tissue (92). Recent developments in the field of 3D bioprinting have provided a powerful tool to fabricate patient-specific tissue, to perfectly fit the geometry of the defect or to match the patient's anatomy (91). Electromechanical stimulation is used for the maturation and development of iPSC-CMs (98), and subsequent vascularization of the bioengineered tissue is required to support metabolic activity.
While presented in a very simple manner, fabricating cardiovascular tissue is not without its challenges. Some of the challenges include the inability to generate large numbers of mature iPSC-CMs, fabricating heart muscle with high contractility, and optimized bioreactors for electromechanical stimulation. Recent advances point to the development of highly functional 3D cardiac patches, though functional integration with host tissue remains a challenge.
There are two potential applications of bioengineered cardiovascular models in HLHS: (1) tools to understand the underlying molecular mechanisms and (2) potential therapeutic strategies, as detailed in a recent review (6).
Tools to understand the underlying molecular mechanisms in HLHS
As one example, there is a high incidence of progressive myocardial dysfunction after the Norwood surgery, as discussed in an earlier section. The goal is to identify molecular indicators responsible for myocardial dysfunction. To accomplish this, blood samples from patients at the time of Norwood can be used to generate iPSCs and cellular difference can be used to correlate with observed clinical outcomes. These iPSCs can further be used to bioengineer 3D patches and that can be used to model tissue level contractility in post-surgical response of HLHS patients. Bioengineered right ventricles (93) can be used to study the effect of volume overload on heart muscle function and subsequent myocardial dysfunction. iPSCs-CMs, patches and ventricles can be used as powerful tools to provide mechanistic information at the cell, tissue, and organ levels.
Potential therapeutic strategies
Bioengineered models may someday be used as potential therapies to support HLHS hearts. As an example, biological contractile pumps (99) can be used in the Fontan circuit to provide pulsatile support for blood, as opposed to the inert grafts currently in use which only serve a passive role. These biological pumps can support the flow of blood through the Fontan circuit. Another example is the use of bioengineered heart muscle, which can be used to add musculature to the right ventricle, thereby augmenting contractile function to support the increased blood volume after the Norwood surgery. Heart transplantation is another important therapeutic approach, but the current scarcity of donor hearts precludes heart transplant as routine management strategy. However, in the future, if bioengineered hearts become available, heart transplantation may be expanded as a treatment option for all HLHS patients, or at minimum, expanded to patients considered high risk for palliative surgeries.
Summary
Our understanding of HLHS has increased over the past two decades, including recent advances in stem cell therapy, dissecting the genetic basis of HLHS, and management of progressive myocardial dysfunction using ventricular assist devices and heart transplantation. Recent advances in tissue engineering and regeneration provide promise to develop new therapies based on stem cell therapy and bioengineered cardiovascular tissue, including patches, ventricles, biological pumps, and whole hearts.
The goal of new therapies is to improve the survival of HLHS patients. This can be accomplished based on an understanding of the genetic basis of the disease, as the master regulator(s), once identified, can be blocked or chemically inhibited. Furthermore, based on an understanding of the molecular basis predictive tools can be developed to quantify the risk of mortality for HLHS patients. Tissue engineering tools, are very powerful, and can be used to increase our understanding of the basic mechanisms and can also be used for repair of the HLHS heart and in the future, bioengineered hearts can be used for HLHS patients and alleviate current shortage of donor hearts.
Author contributions
AB prepared the section on historical perspective, introduction, and abstract. WS, OO, and CC prepared the section on surgical management of HLHS. SL prepared the introduction. RB the prepared sections on genetic basis of HLHS, tissue engineering, regenerative medicine, and summary. SL and SK prepared section on HLHS outcomes. PS and JS prepared the section on hemodynamics. AP the prepared section on stem cell therapy in HLHS patients. All authors contributed to the article and approved the submitted version.
Acknowledgments
SK would like to acknowledge financial support from NIH R01 4R01-HL140305.
Conflict of interest
The authors declare that the research was conducted in the absence of any commercial or financial relationships that could be construed as a potential conflict of interest.
Publisher's note
All claims expressed in this article are solely those of the authors and do not necessarily represent those of their affiliated organizations, or those of the publisher, the editors and the reviewers. Any product that may be evaluated in this article, or claim that may be made by its manufacturer, is not guaranteed or endorsed by the publisher.
References
1. Kritzmire SM, Cossu AE. Hypoplastic Left Heart Syndrome. Treasure Island: StatPearls Publishing Copyright © 2021, StatPearls Publishing LLC (2021).
2. Kobayashi J, Sano S, Oh H. Dissecting the left heart hypoplasia by pluripotent stem cells. In: Nakanishi T, Markwald RR, Baldwin HS, Keller BB, Srivastava D, Yamagishi H, editors. Etiology and Morphogenesis of Congenital Heart Disease: From Gene Function and Cellular Interaction to Morphology. Tokyo: Springer (2016). p. 337–9. doi: 10.1007/978-4-431-54628-3_47
3. Jiang W, Lan F, Zhang H. Human induced pluripotent stem cells for inherited cardiovascular diseases modeling. Curr Stem Cell Res Ther. (2016) 11:533–41. doi: 10.2174/1574888X09666141016170856
4. Ambastha C, Bittle GJ, Morales D, Parchment N, Saha P, Mishra R, et al. Regenerative medicine therapy for single ventricle congenital heart disease. Transl Pediatr. (2018) 7:176–87. doi: 10.21037/tp.2018.04.01
5. Chery J, Wong J, Huang S, Wang S, Si MS. Regenerative medicine strategies for hypoplastic left heart syndrome. Tissue Eng Part B Rev. (2016) 22:459–69. doi: 10.1089/ten.teb.2016.0136
6. Williams SK, Birla RK. Tissue engineering solutions to replace contractile function during pediatric heart surgery. Tissue Cell. (2020) 67:101452. doi: 10.1016/j.tice.2020.101452
7. Rai V, Gladki M, Dudynska M, Skalski J. Hypoplastic left heart syndrome [HLHS]: treatment options in present era. Indian J Thorac Cardiovasc Surg. (2019) 35:196–202. doi: 10.1007/s12055-018-0742-z
8. Norwood WI, Lang P, Hansen DD. Physiologic repair of aortic atresia-hypoplastic left heart syndrome. N Engl J Med. (1983) 308:23–6. doi: 10.1056/NEJM198301063080106
9. Stasik CN, Gelehrter S, Goldberg CS, Bove EL, Devaney EJ, Ohye RG. Current outcomes and risk factors for the Norwood procedure. J Thorac Cardiovasc Surg. (2006) 131:412–7. doi: 10.1016/j.jtcvs.2005.09.030
10. Roehl SL, Townsend RJ. Alprostadil (Prostin VR Pediatric Sterile Solution, The Upjohn Company). Drug Intell Clin Pharm. (1982) 16:823–32. doi: 10.1177/106002808201601103
11. Doble M, Makadia N, Pavithran S, Kumar RS. Analysis of explanted ePTFE cardiovascular grafts (modified BT shunt). Biomed Mater. (2008) 3:034118. doi: 10.1088/1748-6041/3/3/034118
12. Quandt D, Ramchandani B, Penford G, Stickley J, Bhole V, Mehta C, et al. Right ventricular outflow tract stent vs. BT shunt palliation in Tetralogy of Fallot. Heart. (2017) 103:1985–91. doi: 10.1136/heartjnl-2016-310620
13. Kanazawa T, Shimizu K, Iwasaki T, Baba K, Otsuki S, Kotani Y, et al. Perioperative milrinone infusion improves one-year survival after the norwood-sano procedure. J Cardiothorac Vasc Anesth. (2021) 2:17. doi: 10.1053/j.jvca.2021.02.017
14. Mohanty SR, Patel A, Kundan S, Radhakrishnan HB, Rao SG. Hypoplastic left heart syndrome: current modalities of treatment and outcomes. Indian J Thorac Cardiovasc Surg. (2021) 37:26–35. doi: 10.1007/s12055-019-00919-7
15. Salik I, Mehta B, Ambati S. Bidirectional Glenn Procedure or Hemi-Fontan. Treasure Island: StatPearls (2021).
16. Gewillig M, Brown SC. The Fontan circulation after 45 years: update in physiology. Heart. (2016) 102:1081–6. doi: 10.1136/heartjnl-2015-307467
17. Susgun S, Kasan K, Yucesan E. Gene hunting approaches through the combination of linkage analysis with whole-exome sequencing in mendelian diseases: from darwin to the present day. Public Health Genom. (2021) 2021:1–11. doi: 10.1159/000517102
18. Martin LJ, Ramachandran V, Cripe LH, Hinton RB, Andelfinger G, Tabangin M, et al. Evidence in favor of linkage to human chromosomal regions 18q, 5q and 13q for bicuspid aortic valve and associated cardiovascular malformations. Hum Genet. (2007) 121:275–84. doi: 10.1007/s00439-006-0316-9
19. McBride KL, Zender GA, Fitzgerald-Butt SM, Koehler D, Menesses-Diaz A, Fernbach S, et al. Linkage analysis of left ventricular outflow tract malformations (aortic valve stenosis, coarctation of the aorta, and hypoplastic left heart syndrome). Eur J Hum Genet. (2009) 17:811–9. doi: 10.1038/ejhg.2008.255
20. Hinton RB, Martin LJ, Rame-Gowda S, Tabangin ME, Cripe LH, Benson DW. Hypoplastic left heart syndrome links to chromosomes 10q and 6q and is genetically related to bicuspid aortic valve. J Am Coll Cardiol. (2009) 53:1065–71. doi: 10.1016/j.jacc.2008.12.023
21. Ciocca L, Digilio MC, Lombardo A, D'Elia G, Baban A, Capolino R, et al. Hypoplastic left heart syndrome and 21q22.3 deletion. Am J Med Genet A. (2015) 167A:579–86. doi: 10.1002/ajmg.a.36914
22. Chen CP, Wang LK, Wu PC, Chang TY, Chern SR, Wu PS, et al. Molecular cytogenetic characterization of Jacobsen syndrome (11q233-q25 deletion) in a fetus associated with double outlet right ventricle, hypoplastic left heart syndrome and ductus venosus agenesis on prenatal ultrasound Taiwan. J Obstet Gynecol. (2017) 56:102–5. doi: 10.1016/j.tjog.2016.12.004
23. Dasgupta C, Martinez AM, Zuppan CW, Shah MM, Bailey LL, Fletcher WH. Identification of connexin43 (alpha1) gap junction gene mutations in patients with hypoplastic left heart syndrome by denaturing gradient gel electrophoresis (DGGE). Mutat Res. (2001) 479:173–86. doi: 10.1016/S0027-5107(01)00160-9
24. Epifantseva I, Shaw RM. Intracellular trafficking pathways of Cx43 gap junction channels. Biochim Biophys Acta Biomembr. (2018) 1860:40–7. doi: 10.1016/j.bbamem.2017.05.018
25. McBride KL, Riley MF, Zender GA, Fitzgerald-Butt SM, Towbin JA, Belmont JW, et al. NOTCH1 mutations in individuals with left ventricular outflow tract malformations reduce ligand-induced signaling. Hum Mol Genet. (2008) 17:2886–93. doi: 10.1093/hmg/ddn187
26. Iascone M, Ciccone R, Galletti L, Marchetti D, Seddio F, Lincesso AR, et al. Identification of de novo mutations and rare variants in hypoplastic left heart syndrome. Clin Genet. (2012) 81:542–54. doi: 10.1111/j.1399-0004.2011.01674.x
27. Theis JL, Hrstka SC, Evans JM, O'Byrne MM, de Andrade M, O'Leary PW, et al. Compound heterozygous NOTCH1 mutations underlie impaired cardiogenesis in a patient with hypoplastic left heart syndrome. Hum Genet. (2015) 134:1003–11. doi: 10.1007/s00439-015-1582-1
28. Hrstka SC, Li X, Nelson TJ, Wanek Program Genetics Pipeline G. NOTCH1-dependent nitric oxide signaling deficiency in hypoplastic left heart syndrome revealed through patient-specific phenotypes detected in bioengineered cardiogenesis. Stem Cells. (2017) 35:1106–19. doi: 10.1002/stem.2582
29. Stallmeyer B, Fenge H, Nowak-Gottl U, Schulze-Bahr E. Mutational spectrum in the cardiac transcription factor gene NKX25 (CSX) associated with congenital heart disease. Clin Genet. (2010) 78:533–40. doi: 10.1111/j.1399-0004.2010.01422.x
30. Ye M, Coldren C, Liang X, Mattina T, Goldmuntz E, Benson DW, et al. Deletion of ETS-1, a gene in the Jacobsen syndrome critical region, causes ventricular septal defects and abnormal ventricular morphology in mice. Hum Mol Genet. (2010) 19:648–56. doi: 10.1093/hmg/ddp532
31. Miyamoto SD, Stauffer BL, Polk J, Medway A, Friedrich M, Haubold K, et al. Gene expression and beta-adrenergic signaling are altered in hypoplastic left heart syndrome. J Heart Lung Transplant. (2014) 33:785–93. doi: 10.1016/j.healun.2014.02.030
32. Ramroop R, Manase G, Lu D, Manase D, Chen S, Kim R, et al. Adrenergic receptor genotypes influence postoperative outcomes in infants in the Single-Ventricle Reconstruction Trial. J Thorac Cardiovasc Surg. (2017) 154:1703–10 e3. doi: 10.1016/j.jtcvs.2017.06.041
33. Eerola A, Poutanen T, Savukoski T, Pettersson K, Sairanen H, Jokinen E, et al. Cardiac troponin I, cardiac troponin-specific autoantibodies and natriuretic peptides in children with hypoplastic left heart syndrome. Interact Cardiovasc Thorac Surg. (2014) 18:80–5. doi: 10.1093/icvts/ivt430
34. Li Y, Klena NT, Gabriel GC, Liu X, Kim AJ, Lemke K, et al. Global genetic analysis in mice unveils central role for cilia in congenital heart disease. Nature. (2015) 521:520–4. doi: 10.1038/nature14269
35. Liu X, Yagi H, Saeed S, Bais AS, Gabriel GC, Chen Z, et al. The complex genetics of hypoplastic left heart syndrome. Nat Genet. (2017) 49:1152–9. doi: 10.1038/ng.3870
36. Sucharov CC, Sucharov J, Karimpour-Fard A, Nunley K, Stauffer BL, Miyamoto SD. Micro-RNA expression in hypoplastic left heart syndrome. J Card Fail. (2015) 21:83–8. doi: 10.1016/j.cardfail.2014.09.013
37. Lange S, Banerjee I, Carrion K, Serrano R, Habich L, Kameny R, et al. miR-486 is modulated by stretch and increases ventricular growth. JCI Insight. (2019) 4:e125507. doi: 10.1172/jci.insight.125507
38. Ricci M, Xu Y, Hammond HL, Willoughby DA, Nathanson L, Rodriguez MM, et al. Myocardial alternative RNA splicing and gene expression profiling in early stage hypoplastic left heart syndrome. PLoS ONE. (2012) 7:e29784. doi: 10.1371/journal.pone.0029784
39. Parker LE, Landstrom AP. Genetic etiology of left-sided obstructive heart lesions: a story in development. J Am Heart Assoc. (2021) 10:e019006. doi: 10.1161/JAHA.120.019006
40. Krane M, Dressen M, Santamaria G, My I, Schneider CM, Dorn T, et al. Sequential defects in cardiac lineage commitment and maturation cause hypoplastic left heart syndrome. Circulation. (2021) 144:1409–28. doi: 10.1161/CIRCULATIONAHA.121.056198
41. Azar J, Bahmad HF, Daher D, Moubarak MM, Hadadeh O, Monzer A, et al. The use of stem cell-derived organoids in disease modeling: an update. Int J Mol Sci. (2021) 22:147667. doi: 10.3390/ijms22147667
42. Gaber N, Gagliardi M, Patel P, Kinnear C, Zhang C, Chitayat D, et al. Fetal reprogramming and senescence in hypoplastic left heart syndrome and in human pluripotent stem cells during cardiac differentiation. Am J Pathol. (2013) 183:720–34. doi: 10.1016/j.ajpath.2013.05.022
43. Theis JL, Vogler G, Missinato MA, Li X, Nielsen T, Zeng XI, et al. Patient-specific genomics and cross-species functional analysis implicate LRP2 in hypoplastic left heart syndrome. Elife. (2020) 9:sa2. doi: 10.7554/eLife.59554.sa2
44. Augustine R, Dan P, Hasan A, Khalaf IM, Prasad P, Ghosal K, et al. Stem cell-based approaches in cardiac tissue engineering: controlling the microenvironment for autologous cells. Biomed Pharmacother. (2021) 138:111425. doi: 10.1016/j.biopha.2021.111425
45. Kern JH, Hayes CJ, Michler RE, Gersony WM, Quaegebeur JM. Survival and risk factor analysis for the Norwood procedure for hypoplastic left heart syndrome. Am J Cardiol. (1997) 80:170–4. doi: 10.1016/S0002-9149(97)00313-5
46. Andrews R, Tulloh R, Sharland G, Simpson J, Rollings S, Baker E, et al. Outcome of staged reconstructive surgery for hypoplastic left heart syndrome following antenatal diagnosis. Arch Dis Child. (2001) 85:474–7. doi: 10.1136/adc.85.6.474
47. Chang RK, Chen AY, Klitzner TS. Clinical management of infants with hypoplastic left heart syndrome in the United States, 1988-1997. Pediatrics. (2002) 110:292–8. doi: 10.1542/peds.110.2.292
48. Krasemann T, Fenge H, Kehl HG, Rukosujew A, Schmid C, Scheld HH, et al. decade of staged Norwood palliation in hypoplastic left heart syndrome in a midsized cardiosurgical center. Pediatr Cardiol. (2005) 26:751–5. doi: 10.1007/s00246-005-0908-5
49. McGuirk SP, Stickley J, Griselli M, Stumper OF, Laker SJ, Barron DJ, et al. Risk assessment and early outcome following the Norwood procedure for hypoplastic left heart syndrome. Eur J Cardiothorac Surg. (2006) 29:675–81. doi: 10.1016/j.ejcts.2006.01.061
50. Tibballs J, Kawahira Y, Carter BG, Donath S, Brizard C, Wilkinson J. Outcomes of surgical treatment of infants with hypoplastic left heart syndrome: an institutional experience 1983-2004. J Paediatr Child Health. (2007) 43:746–51. doi: 10.1111/j.1440-1754.2007.01164.x
51. Sano S, Huang SC, Kasahara S, Yoshizumi K, Kotani Y, Ishino K. Risk factors for mortality after the Norwood procedure using right ventricle to pulmonary artery shunt. Ann Thorac Surg. (2009) 87:178–85. doi: 10.1016/j.athoracsur.2008.08.027
52. Furck AK, Uebing A, Hansen JH, Scheewe J, Jung O, Fischer G, et al. Outcome of the Norwood operation in patients with hypoplastic left heart syndrome: a 12-year single-center survey. J Thorac Cardiovasc Surg. (2010) 139:359–65. doi: 10.1016/j.jtcvs.2009.07.063
53. Knirscha W, Liamlahia R, Hugb MI, Hoopa R, Rheinc M, Prêtred R, et al. Mortality and neurodevelopmental outcome at 1 year of age comparing hybrid and Norwood procedures. Eur J Cardio Thoracic Surg. (2012) 42:33–9. doi: 10.1093/ejcts/ezr286
54. Pasquali SK, Jacobs JP, He X, Hornik CP, Jaquiss RDB, Jacobs ML, et al. The complex relationship between center volume and outcome in patients undergoing the norwood operation. Ann Thorac Surg. (2012) 93:1556–62. doi: 10.1016/j.athoracsur.2011.07.081
55. Lloyd DF, Cutler L, Tibby SM, Vimalesvaran S, Qureshi SA, Rosenthal E, et al. Analysis of preoperative condition and interstage mortality in Norwood and hybrid procedures for hypoplastic left heart syndrome using the Aristotle scoring system. Heart. (2014) 100:775–80. doi: 10.1136/heartjnl-2013-304759
56. Rai V, Mroczek T, Szypulski A, Pac A, Gladki M, Dudynska M, et al. Outcome of Norwood operation for hypoplastic left heart syndrome. Indian J Thorac Cardiovasc Surg. (2018) 34:337–44. doi: 10.1007/s12055-017-0603-1
57. Mascio CE, Irons ML, Ittenbach RF, Gaynor JW, Fuller SM, Kaplinski M, et al. Thirty years and 1663 consecutive Norwood procedures: has survival plateaued? J Thorac Cardiovasc Surg. (2019) 158:220–9. doi: 10.1016/j.jtcvs.2018.12.117
58. de Zelicourt DA, Marsden A, Fogel MA, Yoganathan AP. Imaging and patient-specific simulations for the Fontan surgery: current methodologies and clinical applications. Prog Pediatr Cardiol. (2010) 30:31–44. doi: 10.1016/j.ppedcard.2010.09.005
59. Migliavacca F, Balossino R, Pennati G, Dubini G, Hsia TY, de Leval MR, et al. Multiscale modelling in biofluidynamics: application to reconstructive paediatric cardiac surgery. J Biomech. (2006) 39:1010–20. doi: 10.1016/j.jbiomech.2005.02.021
60. Altmann K, Printz BF, Solowiejczky DE, Gersony WM, Quaegebeur J, Apfel HD. Two-dimensional echocardiographic assessment of right ventricular function as a predictor of outcome in hypoplastic left heart syndrome. Am J Cardiol. (2000) 86:964–8. doi: 10.1016/S0002-9149(00)01131-0
61. Hornik CP, He X, Jacobs JP Li JS, Jaquiss RD, Jacobs ML, O'Brien SM, et al. Complications after the Norwood operation: an analysis of The Society of Thoracic Surgeons Congenital Heart Surgery Database. Ann Thorac Surg. (2011) 92:1734–40. doi: 10.1016/j.athoracsur.2011.05.100
62. Pennati G, Fiore GB, Migliavacca F, Lagana K, Fumero R, Dubini G. In vitro steady-flow analysis of systemic-to-pulmonary shunt haemodynamics. J Biomech. (2001) 34:23–30. doi: 10.1016/S0021-9290(00)00167-6
63. Moghadam ME, Migliavacca F, Vignon-Clementel IE, Hsia TY, Marsden AL. Optimization of shunt placement for the Norwood surgery using multi-domain modeling. J Biomech Eng. (2012) 134:e51002. doi: 10.1115/1.4006814
64. Rijnberg FM, Hazekamp MG, Wentzel JJ, de Koning PJH, Westenberg JJM, Jongbloed MRM, et al. Energetics of blood flow in cardiovascular disease: concept and clinical implications of adverse energetics in patients with a fontan circulation. Circulation. (2018) 137:2393–407. doi: 10.1161/CIRCULATIONAHA.117.033359
65. Troianowski G, Taylor CA, Feinstein JA, Vignon-Clementel IE. Three-dimensional simulations in Glenn patients: clinically based boundary conditions, hemodynamic results and sensitivity to input data. J Biomech Eng. (2011) 133:111006. doi: 10.1115/1.4005377
66. Lardo AC, Webber SA, Iyengar A, del Nido PJ, Friehs I, Cape EG. Bidirectional superior cavopulmonary anastomosis improves mechanical efficiency in dilated atriopulmonary connections. J Thorac Cardiovasc Surg. (1999) 118:681–91. doi: 10.1016/S0022-5223(99)70014-0
67. Bove EL, de Leval MR, Migliavacca F, Guadagni G, Dubini G. Computational fluid dynamics in the evaluation of hemodynamic performance of cavopulmonary connections after the Norwood procedure for hypoplastic left heart syndrome. J Thorac Cardiovasc Surg. (2003) 126:1040–7. doi: 10.1016/S0022-5223(03)00698-6
68. de Zelicourt DA, Pekkan K, Wills L, Kanter K, Forbess J, Sharma S, et al. In vitro flow analysis of a patient-specific intraatrial total cavopulmonary connection. Ann Thorac Surg. (2005) 79:2094–102. doi: 10.1016/j.athoracsur.2004.12.052
69. Roldán-Alzate A, García-Rodríguez S, Anagnostopoulos PV, Srinivasan S, Wieben O, François CJ. Hemodynamic study of TCPC using in vivo and in vitro 4D Flow MRI and numerical simulation. J Biomech. (2015) 48:1325–30. doi: 10.1016/j.jbiomech.2015.03.009
70. Dasi LP, Krishnankuttyrema R, Kitajima HD, Pekkan K, Sundareswaran KS, Fogel M, et al. Fontan hemodynamics: importance of pulmonary artery diameter. J Thorac Cardiovasc Surg. (2009) 137:560–4. doi: 10.1016/j.jtcvs.2008.04.036
71. Migliavacca F, Dubini G, Bove EL, de Leval MR. Computational fluid dynamics simulations in realistic 3-D geometries of the total cavopulmonary anastomosis: the influence of the inferior caval anastomosis. J Biomech Eng. (2003) 125:805–13. doi: 10.1115/1.1632523
72. Sharma S, Goudy S, Walker P, Panchal S, Ensley A, Kanter K, et al. In vitro flow experiments for determination of optimal geometry of total cavopulmonary connection for surgical repair of children with functional single ventricle. J Am Coll Cardiol. (1996) 27:1264–9. doi: 10.1016/0735-1097(95)00598-6
73. Ensley AE, Lynch P, Chatzimavroudis GP, Lucas C, Sharma S, Yoganathan AP. Toward designing the optimal total cavopulmonary connection: an in vitro study. Ann Thorac Surg. (1999) 68:1384–90. doi: 10.1016/S0003-4975(99)00560-3
74. Haggerty CM, de Zelicourt DA, Restrepo M, Rossignac J, Spray TL, Kanter KR, et al. Comparing pre- and post-operative Fontan hemodynamic simulations: implications for the reliability of surgical planning. Ann Biomed Eng. (2012) 40:2639–51. doi: 10.1007/s10439-012-0614-4
75. Mroczek T, Malota Z, Wojcik E, Nawrat Z, Skalski J. Norwood with right ventricle-to-pulmonary artery conduit is more effective than Norwood with Blalock-Taussig shunt for hypoplastic left heart syndrome: mathematic modeling of hemodynamics. Eur J Cardiothorac Surg. (2011) 40:1412–7. doi: 10.1016/j.ejcts.2011.03.033
76. de Vecchi A, Nordsletten DA, Remme EW, Bellsham-Revell H, Greil G, Simpson JM, et al. Inflow typology and ventricular geometry determine efficiency of filling in the hypoplastic left heart. Ann Thorac Surg. (2012) 94:1562–9. doi: 10.1016/j.athoracsur.2012.05.122
77. Zebhi B, Wiputra H, Howley L, Cuneo B, Park D, Hoffman H, et al. Right ventricle in hypoplastic left heart syndrome exhibits altered hemodynamics in the human fetus. J Biomech. (2020) 112:110035. doi: 10.1016/j.jbiomech.2020.110035
78. Doost SN, Ghista D, Su B, Zhong L, Morsi YS. Heart blood flow simulation: a perspective review. Biomed Eng Online. (2016) 15:101. doi: 10.1186/s12938-016-0224-8
79. Shar JA, Brown KN, Keswani SG, Grande-Allen J, Sucosky P. Impact of aortoseptal angle abnormalities and discrete subaortic stenosis on left-ventricular outflow tract hemodynamics: preliminary computational assessment. Front Bioeng Biotechnol. (2020) 8:114. doi: 10.3389/fbioe.2020.00114
80. Shar JA, Keswani SG, Grande-Allen KJ, Sucosky P. Computational assessment of valvular dysfunction in discrete subaortic stenosis: a parametric study. Cardiovasc Eng Technol. (2021) 2021:1900665. doi: 10.1080/24748706.2021.1900665
81. Masse DD, Shar JA, Brown KN, Keswani SG, Grande-Allen KJ, Sucosky P. Discrete subaortic stenosis: perspective roadmap to a complex disease. Front Cardiovasc Med. (2018) 5:122. doi: 10.3389/fcvm.2018.00122
82. Elde S, Wang H, Woo YJ. Navigating the crossroads of cell therapy and natural heart regeneration. Front Cell Dev Biol. (2021) 9:674180. doi: 10.3389/fcell.2021.674180
83. Tohyama S, Fukuda K. Safe and effective cardiac regenerative therapy with human-induced pluripotent stem cells: how should we prepare pure cardiac myocytes? Circ Res. (2017) 120:1558–60. doi: 10.1161/CIRCRESAHA.116.310328
84. Burkhart HM, Qureshi MY, Peral SC, O'Leary PW, Olson TM, Cetta F, et al. Regenerative therapy for hypoplastic left heart syndrome: first report of intraoperative intramyocardial injection of autologous umbilical-cord blood-derived cells. J Thorac Cardiovasc Surg. (2015) 149:e35–7. doi: 10.1016/j.jtcvs.2014.10.093
85. Burkhart HM, Qureshi MY, Rossano JW, Cantero Peral S, O'Leary PW, Hathcock M, et al. Autologous stem cell therapy for hypoplastic left heart syndrome: safety and feasibility of intraoperative intramyocardial injections. J Thorac Cardiovasc Surg. (2019) 158:1614–23. doi: 10.1016/j.jtcvs.2019.06.001
86. Ishigami S, Ohtsuki S, Eitoku T, Ousaka D, Kondo M, Kurita Y, et al. Intracoronary cardiac progenitor cells in single ventricle physiology: the PERSEUS (Cardiac Progenitor Cell Infusion to Treat Univentricular Heart Disease) Randomized Phase 2 Trial. Circ Res. (2017) 120:1162–73. doi: 10.1161/CIRCRESAHA.116.310253
87. Ishigami S, Ohtsuki S, Tarui S, Ousaka D, Eitoku T, Kondo M, et al. Intracoronary autologous cardiac progenitor cell transfer in patients with hypoplastic left heart syndrome: the TICAP prospective phase 1 controlled trial. Circ Res. (2015) 116:653–64. doi: 10.1161/CIRCRESAHA.116.304671
88. Qureshi MY, Cabalka AK, Khan SP, Hagler DJ, Haile DT, Cannon BC, et al. Cell-based therapy for myocardial dysfunction after fontan operation in hypoplastic left heart syndrome. Mayo Clin Proc Innov Qual Outcomes. (2017) 1:185–91. doi: 10.1016/j.mayocpiqo.2017.07.002
89. Rupp S, Zeiher AM, Dimmeler S, Tonn T, Bauer J, Jux C, et al. Regenerative strategy for heart failure in hypoplastic left heart syndrome: intracoronary administration of autologous bone marrow-derived progenitor cells. J Heart Lung Transplant. (2010) 29:574–7. doi: 10.1016/j.healun.2009.10.006
90. Rheault-Henry M, White I, Grover D, Atoui R. Stem cell therapy for heart failure: medical breakthrough, or dead end? World J Stem Cells. (2021) 13:236–59. doi: 10.4252/wjsc.v13.i4.236
91. Birla RK, Williams SK. 3D bioprinting and its potential impact on cardiac failure treatment: an industry perspective. APL Bioeng. (2020) 4:e10903. doi: 10.1063/1.5128371
92. Abbasgholizadeh R, Islas JF, Navran S, Potaman VN, Schwartz RJ, Birla RK, et al. Highly conductive 3D cardiac patch fabricated using cardiac myocytes reprogrammed from human adipogenic mesenchymal stem cells. Cardiovasc Eng Technol. (2020) 11:205–18. doi: 10.1007/s13239-019-00451-0
93. Patel NM, Birla RK. The bioengineered cardiac left ventricle. ASAIO J. (2018) 64:56–62. doi: 10.1097/MAT.0000000000000642
94. Tracy E, Gettler B, Zakhari J, Schwartz RJ, Williams SK, Birla R. 3D bioprinting the cardiac purkinje system using human adipogenic mesenchymal stem cell derived purkinje cells. Cardiovasc Eng Technol. (2020) 478:8. doi: 10.1007/s13239-020-00478-8
95. Tao ZW, Mohamed M, Hogan M, Salazar B, Patel NM, Birla RK. Establishing the framework for fabrication of a bioartificial heart. ASAIO J. (2015) 61:429–36. doi: 10.1097/MAT.0000000000000233
96. Birla RK. A methodological nine-step process to bioengineer heart muscle tissue. Tissue Cell. (2020) 67:101425. doi: 10.1016/j.tice.2020.101425
97. Lian X, Zhang J, Azarin SM, Zhu K, Hazeltine LB, Bao X, et al. Directed cardiomyocyte differentiation from human pluripotent stem cells by modulating Wnt/beta-catenin signaling under fully defined conditions. Nat Protoc. (2013) 8:162–75. doi: 10.1038/nprot.2012.150
98. Ronaldson-Bouchard K, Ma SP, Yeager K, Chen T, Song L, Sirabella D, et al. Advanced maturation of human cardiac tissue grown from pluripotent stem cells. Nature. (2018) 556:239–43. doi: 10.1038/s41586-018-0016-3
Keywords: stem cells, congenital heart defect (CHD), genetics, hemodynamic, regenerative medicine
Citation: Birla AK, Brimmer S, Short WD, Olutoye OO II, Shar JA, Lalwani S, Sucosky P, Parthiban A, Keswani SG, Caldarone CA and Birla RK (2022) Current state of the art in hypoplastic left heart syndrome. Front. Cardiovasc. Med. 9:878266. doi: 10.3389/fcvm.2022.878266
Received: 17 February 2022; Accepted: 30 August 2022;
Published: 28 October 2022.
Edited by:
Gian Marco Rosa, San Martino Hospital (IRCCS), ItalyReviewed by:
Tomasz Marek Mroczek, Jagiellonian University Medical College, PolandLuc Mertens, University of Toronto, Canada
Gianluca Santamaria, Technical University of Munich, Germany
Copyright © 2022 Birla, Brimmer, Short, Olutoye, Shar, Lalwani, Sucosky, Parthiban, Keswani, Caldarone and Birla. This is an open-access article distributed under the terms of the Creative Commons Attribution License (CC BY). The use, distribution or reproduction in other forums is permitted, provided the original author(s) and the copyright owner(s) are credited and that the original publication in this journal is cited, in accordance with accepted academic practice. No use, distribution or reproduction is permitted which does not comply with these terms.
*Correspondence: Ravi K. Birla, cmF2aS5iaXJsYUBiY20uZWR1
†These authors share senior authorship