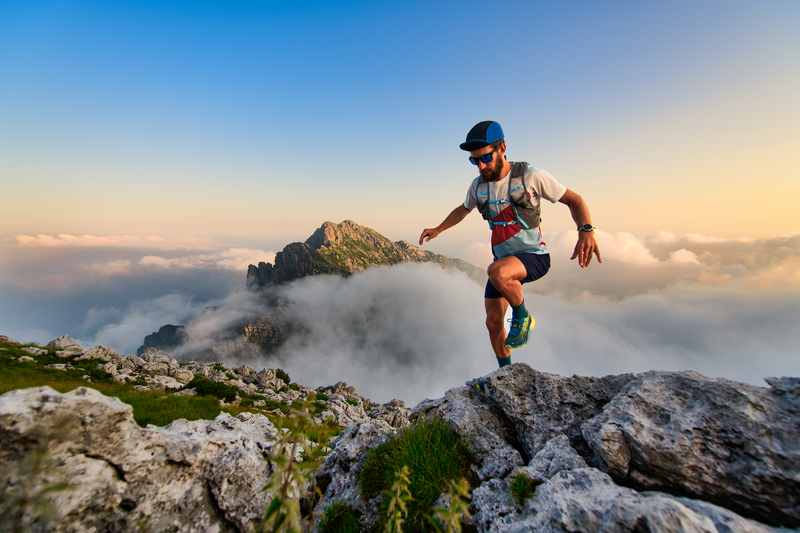
94% of researchers rate our articles as excellent or good
Learn more about the work of our research integrity team to safeguard the quality of each article we publish.
Find out more
MINI REVIEW article
Front. Cardiovasc. Med. , 20 May 2022
Sec. General Cardiovascular Medicine
Volume 9 - 2022 | https://doi.org/10.3389/fcvm.2022.868658
This article is part of the Research Topic Cardiorenal Syndromes: From pathogenesis to clinical research View all 10 articles
Heart and kidney failure often co-exist and confer high morbidity and mortality. The complex bi-directional nature of heart and kidney dysfunction is referred to as cardiorenal syndrome, and can be induced by acute or chronic dysfunction of either organ or secondary to systemic diseases. The five clinical subtypes of cardiorenal syndrome are categorized by the perceived primary precipitant of organ injury but lack precision. Traditional biomarkers such as serum creatinine are also limited in their ability to provide an early and accurate diagnosis of cardiorenal syndrome. Novel biomarkers have the potential to assist in the diagnosis of cardiorenal syndrome and guide treatment by evaluating the relative roles of implicated pathophysiological pathways such as hemodynamic dysfunction, neurohormonal activation, endothelial dysfunction, inflammation and oxidative stress, and fibrosis. In this review, we assess the utility of biomarkers that correlate with kidney and cardiac (dys)function, inflammation/oxidative stress, fibrosis, and cell cycle arrest, as well as emerging novel biomarkers (thrombospondin-1/CD47, glycocalyx and interleukin-1β) that may provide prediction and prognostication of cardiorenal syndrome, and guide potential development of targeted therapeutics.
Heart failure (HF) and chronic kidney disease (CKD) are increasing public health issues with a prevalence of 4% and 9% respectively, and a global rise in attributable deaths by 41% for both diseases since 1990 (1, 2). Cardiorenal syndrome (CRS) refers to the concurrent dysfunction of the heart and kidney, which can initiate and perpetuate disease in the other organ through hemodynamic, neurohormonal, and immunological and/or biochemical feedback pathways (3). Combined HF and CKD is associated with high morbidity and mortality (4–9). In people with HF, every 10 ml/min decrease in estimated glomerular filtration rate (eGFR) increases the risk of all-cause death by 7%, while HF hospitalization in people with CKD increases the risk of all-cause death 3–7-fold (8, 9).
Currently, CRS is classified into 5 different subtypes based on the perceived primary precipitant of organ injury: (10)
1. CRS type 1: rapid decline in cardiac function (e.g., cardiogenic shock or acute decompensation of chronic HF) resulting in acute kidney injury (AKI).
2. CRS type 2: chronic cardiac dysfunction (e.g., chronic HF) causing progressive decline in kidney function and CKD.
3. CRS type 3: acute decline in kidney function (e.g., AKI or glomerulonephritis) causing acute cardiac dysfunction (e.g., HF, arrhythmia, or myocardial infarction).
4. CRS type 4: CKD causing progressive decline in cardiac function (e.g., left ventricular hypertrophy, HF, or myocardial infarction).
5. CRS type 5: combine cardiac and kidney dysfunction caused by an acute or chronic systemic disorder (e.g., sepsis or diabetes mellitus).
While this classification system is clinical intuitive, it may be difficult to identify the initial insult. Furthermore, this classification system does not incorporate the pathophysiological pathways implicated in CRS such as hemodynamic dysfunction, neurohormonal activation, endothelial dysfunction, inflammation, and fibrosis (11, 12).
Current biomarkers of kidney function such as serum creatinine also have limited sensitivity and specificity. A rise in serum creatinine only occurs after a significant decline in GFR (e.g., 48–72 h after AKI or after 50% of function is lost chronically), is non-specific to the underlying disease process, and is affected by clinical characteristics (e.g., age, weight, gender, ethnicity, volume status, and medication use) that do not reflect true parenchymal injury (13). In this review, we summarize the evidence for different biomarkers in CRS (Figure 1 and Table 1) as well as promising emerging biomarkers that may inform on future management in this debilitating condition.
Figure 1. Biomarkers in the pathways of injury in cardiorenal syndrome. Created with Biorender. Cardiorenal syndrome involves the complex bidirectional nature of heart and kidney dysfunction. The pathways involved include hemodynamic dysfunction, neurohormonal activation (primarily the renin-angiotensin-aldosterone system), inflammation and oxidative stress, endothelial dysfunction, and ultimately injury and fibrosis to both heart and kidney. We have outlined the pathways which each biomarker is associated with as well as indicated biomarkers with the most clinical applicability based on the evidence quality of existing literature (underline) vs. biomarkers requiring further research and validation in larger cohort of patients with cardiorenal syndrome (italics). Of note, biomarker performance will depend on kidney function, timing of collection and type of cardiorenal syndrome. Furthermore, a combination of biomarkers may have superior performance to a single biomarker. Therefore, further research is required to identify the type and timing of biomarker(s) analysis in the prediction and prognostication of cardiorenal syndrome. NGAL, neutrophil gelatinase-associated lipocalin; KIM-1, kidney injury molecule-1; L-FABP, liver fatty acid-binding protein; NAG, N-acetyl-beta-D-glucosaminidase; BNP, brain natriuretic peptide; NT-proBNP, N-terminal pro-brain natriuretic peptide; IL, interleukin; TIMP2, tissue inhibitor of metalloproteinases-1 and -2; IGFBP7, insulin-like growth factor-binding protein-7; TSP-1, thrombospondin-1.
Albuminuria is a cheap and widely available biomarker, which may not only reflect glomerular injury but also endothelial dysfunction (14). In three large chronic HF trials, microalbuminuria (30–299 mg/g) and macroalbuminuria (≥ 300 mg/g) were associated with a 1.4–1.8-fold increased risk of all-cause death, cardiovascular death, or HF hospitalization (15–17). Albuminuria predicted these outcomes independent of serum creatinine, HbA1c and left ventricular ejection fraction (LVEF) (16). While treatments exist to reduce albuminuria [e.g., renin-angiotensin-aldosterone system (RAAS) inhibitors], whether targeting albuminuria improves prognosis in CRS requires further study.
Cystatin-C, a 13-kDa cysteine protease inhibitor, is produced at a constant rate by all nucleated cells and is freely filtered through the glomerulus, almost completely reabsorbed, and not secreted by the renal tubules. Cystatin-C inhibits collagen- and elastin-degrading cysteine proteases of the cathepsin family and protects against atherosclerosis in apolipoprotein E–deficient mice, though its role in CRS is unclear (18). In both acute and chronic HF, elevated plasma or urinary cystatin-C was associated with a 2–3-fold increased risk of all-cause death, independent of serum creatinine or eGFR (19–22). In acute HF, plasma cystatin-C modestly predicted AKI [area under the receiver operating characteristic curve (AUC-ROC) 0.68] and all-cause death or HF hospitalization (AUC-ROC, 95% confidence interval: 0.73, 0.66–0.80) (23, 24), providing prognostic value in addition to N-terminal prohormone of brain natriuretic peptide (NT-proBNP) and troponin (25). In elderly patients with chronic HF, the highest quartile of cystatin-C doubled the risk of all-cause death, outperforming serum creatinine in multivariate analyses (21). After cardiac surgery, plasma cystatin-C modestly predicted AKI (AUC-ROC 0.68), (26) while urinary cystatin-C has not consistently predicted AKI (27, 28).
Unlike serum creatinine, plasma cystatin-C is not affected by muscle mass but both are affected by volume status. In the Renal Optimization Strategies Evaluation–Acute Heart Failure (ROSE-HF) trial, protocol-driven aggressive diuresis caused worsening of renal function (WRF), based on ≥ 20% decrease in eGFR using cystatin C, in 21% of participants but was not associated with an increase in kidney tubular injury markers (29). This suggests tubular markers may have utility in differentiating AKI due to diuretic-induced volume depletion or parenchymal injury in CRS.
Galectin-3 is a 30-kDa glycoprotein synthesized by cardiac macrophages in response to angiotensin II and aldosterone, which mediates collagen deposition by fibroblasts resulting in cardiac fibrosis (12). Plasma galectin-3 levels are also inversely related to renal function and therefore represents a biomarker for both cardiac fibrosis and GFR. In acute HF, while NT-proBNP was superior to galectin-3 for diagnosis, galectin-3 may be superior to NT-proBNP at predicting 60-day mortality (AUC-ROC 0.74 vs. 0.67, p = 0.05) and was associated with a 14-fold increased risk of all-cause death or HF hospitalization in multivariate analysis (30). In chronic HF, galectin-3 only modestly predicted all-cause death (AUC-ROC 0.612, 0.538–0.685) and all-cause death or HF hospitalization (AUC-ROC 0.58, 0.55–0.61). Pooled analysis of chronic HF trials (mean eGFR 54–58 ml/min/1.73 m2) showed elevated galectin-3 was associated with a 1.6–2-fold increased risk of all-cause death or HF hospitalization (31–33).
Proenkephalin A is an endogenous opioid secreted by cardiac cells and mediates negative inotropic effects via the delta opioid receptor. Plasma proenkephalin is inversely proportional to GFR and associated with a 1.5-fold increased risk of CKD (highest vs lowest tertile) (34). In acute HF, proenkephalin A modestly predicted AKI (AUC-ROC 0.69) and was independently associated with a 27% increased risk of 1-year mortality or HF hospitalization (35).
Neutrophil gelatinase-associated lipocalin (NGAL) is a 25-kDa protein secreted by immature neutrophils, epithelial cells (including renal tubular epithelium) and cardiomyocytes in response to inflammation. While NGAL has been extensively studied as a marker of renal tubular injury, it also plays a role in mineralocorticoid-mediated cardiovascular fibrosis. NGAL knockout mice demonstrated blunted vascular fibrosis in response to an aldosterone-salt challenge, and reduced cardiac fibrosis, inflammation and left ventricular dysfunction in a myocardial infarction model (36, 37). However, no treatments exist to inhibit NGAL for evaluation in CRS.
In neonatal and pediatric cardiac surgery, plasma and urinary NGAL were detectable 2 h post-surgery (compared to 1–3 days for serum creatinine) and performed exceptionally at predicting AKI (AUC-ROC 0.92–0.998) (38, 39). A small study in adult cardiac surgery demonstrated similar predictive ability for detecting AKI (AUC-ROC 0.98), (40) but this has not been consistent replicated. In a meta-analysis of NGAL in diagnosing AKI, subgroup analysis of 10 studies reporting cardiac surgery-related AKI demonstrated plasma and/or urinary NGAL moderately predicted AKI (AUC-ROC 0.775, 0.669–0.867) (41). In a large cohort study of adults undergoing cardiac surgery, plasma (but not urinary) NGAL significantly improved risk prediction of AKI over the clinical models using demographic factors, surgical factors, eGFR and patient comorbidities (42). These differences highlight the importance of considering time and baseline kidney function when assessing biomarkers. Indeed, for the diagnosis of AKI in 529 critically ill patients in the intensive care unit, cystatin-C, interleukin-18 (IL-18), NGAL, kidney injury molecule-1 (KIM-1), and γ-glutamyltranspeptidase demonstrated optimal performance earlier (≤ 12 h after injury) in patients with preserved kidney function (eGFR ≥ 60 ml/min) and later (12–36 h after injury) in patients with reduced kidney function (eGFR < 60 ml/min) (43). In acute HF, plasma and urinary NGAL were associated with a 1.3–2-fold increased risk of long-term mortality, (22, 44) and plasma NGAL outperformed cystatin-C at predicting AKI (AUC-ROC 0.93 vs. 0.68) (23). Plasma NGAL kinetics may also improve its ability to predict AKI (AUC-ROC 0.91 for delta NGAL change vs. 0.69 for NGAL at baseline) (45). Lastly, urinary NGAL performed 2 days after hospitalization for acute HF differentiated true WRF from pseudo-WRF based on AKI with or without clinical improvement (AUC-ROC 0.83, 0.73–0.93), (22) was associated with a 5-fold risk of AKI, (46) but failed to predict AKI-to-CKD transition (47).
Kidney injury molecule-1 (KIM-1) is a transmembrane glycoprotein with immunoglobulin and mucin domains, which is expressed by renal proximal tubule epithelium in response to injury and facilitates phagocytosis of apoptotic tubular cells. In chronic HF, elevated urinary KIM-1 was modestly associated with a 10–15% increased risk of all-cause death or HF hospitalization but did not predict AKI nor AKI-to-CKD transition in acute HF (22, 47–49). In contrast, urinary KIM-1 measured 12 h after cardiac surgery predicted AKI with good performance (AUC-ROC 0.83–0.88), (50, 51) and a high cut-off for urinary KIM-1 (highest vs. lowest tertile) was associated with a doubling of 3-year mortality risk, independent of post-operative AKI (52).
Interleukin-18 (IL-18) is an 18-kDa pro-inflammatory cytokine produced by immune cells (e.g., macrophages) and non-immune cells (e.g., vascular endothelial cells and renal proximal tubular cells) in response to tissue injury via activation of the NLRP3-inflammasome, which causes programmed cell death in both cardiomyocytes and renal tubular cells (53–55). In acute HF, elevated urinary IL-18 was associated with a 3.6-fold increased risk of AKI in multivariate analysis, (46) and modestly predicted AKI-to-CKD transition at 6 months (AUC-ROC 0.674, 0.543-0.805) (47). In people undergoing cardiac surgery, urinary IL-18 also modestly predicted AKI (AUC-ROC 0.61 at 4 h post-surgery, 0.75 at 12 h, and 0.73 at 24 h), (56) which was superior to clinical models using eGFR, (42) and was associated with a modest 1.2-fold increased risk of long-term mortality (52).
Fatty-acid binding proteins (FABPs) are a family of 15-kDa cytoplasmic proteins that are involved in the intracellular transport of long-chain fatty acids. L-FABP is located on the renal proximal tubular cells may reduce oxidative stress by binding fatty acid oxidation products (57). NAG is a large lysosomal brush border enzyme (>130 kDa), predominantly expressed on proximal tubular cells, and is not freely filtered. In patients undergoing cardiac surgery, combining urinary L-FABP and NAG at 4 h after surgery with pre-operative clinical factors (including demographics, comorbidities and serum creatinine) improved AKI prediction compared to pre-operative clinical factors alone (AUC-ROC 0.86, 0.74–0.93 vs. 0.79, 0.66–0.88, p < 0.05) (58). In chronic HF, elevated urinary NAG was also associated with a 1.2-fold increased risk of HF hospitalization and 1.3–1.4-fold increased risk of all-cause death in multivariate models (48, 49). In acute HF, urinary angiotensinogen also predicted AKI (AUC-ROC 0.78) and all-cause death (AUC-ROC 0.85) (46).
Cardiac troponin I (cTnI) and T (cTnT) are established diagnostic and prognostic biomarkers in myocardial infarction and HF (59). While troponin levels are elevated in people with CKD due to reduced plasma clearance, elevated cTNT was associated with a 2–6-fold increased risk of cardiovascular events, (60, 61) but not all-cause death after adjusting for kidney function (62). Adjusting cTnT cut-off retained good performance at detecting acute myocardial infarction in people with CKD (cTnT > 350 ng/L (standard cut-off > 14 ng/L) for eGFR < 15 ml/min/1.73 m2: sensitivity 95%, specificity 97%; cTnT cut-off > 43.2 ng/L for eGFR < 60 ml/min/1.73 m2: sensitivity 92%, specificity: 88%) (63, 64).
Pro-BNP is secreted by cardiomyocytes in the ventricle and atria in response to ventricular wall stretch and cleaved into active BNP and inactive NT-proBNP, which are both established biomarkers in HF (65). While NT-proBNP is elevated in people with CKD, elevated NT-proBNP was still associated with a 1.3-fold increased risk of cardiovascular events and 1.6-fold increased risk of all-cause death after adjusting for kidney function (60). In people with CKD, both BNP and NT-proBNP demonstrated moderate predictive ability for all-cause death and/or cardiovascular events at different cut-offs. For stage 1–3 CKD, BNP > 90.8 pg/ml (AUC-ROC 0.699) and NT-proBNP > 259.7 pg/ml (AUC-ROC 0.702) predicted all-cause death. In comparison, for stage 4–5 CKD, BNP > 157.0 pg/ml (AUC-ROC 0.666) and NT-proBNP > 5,111.5 pg/ml (AUC-ROC 0.720) predicted all-cause death or cardiovascular events (66). NT-proBNP kinetics may have improved clinical utility in the CKD population with a doubling of NT-proBNP associated with a 1.4-fold increased risk of cardiovascular events in African-Americans with CKD in multivariate analysis (67).
TIMP2 and IGFBP7 are involved in G1 cell-cycle arrest during early phases of cell injury and the product of urinary TIMPIGFBP7 concentrations (NephroCheck) is the first Food and Drug Administration-approved test to assess the risk of AKI based on studies in critically ill patients in the intensive care unit (68–70). Small studies have demonstrated urinary [TIMP2]•[IGFBP7] predict AKI after cardiac surgery (AUC-ROC 0.84), (71) and in acute HF, where it outperformed KIM-1 (AUC-ROC 0.75, 0.61–0.88 vs. 0.54, 0.37–0.70) (72).
The forementioned biomarkers demonstrate diagnostic utility for AKI and/or cardiac events though implementation remains challenging. Key suggestions for biomarker use from the 23rd Acute Disease Quality Initiative meeting included combining damage and functional biomarkers to identify patients at high-risk of AKI, to improve the diagnostic accuracy of AKI, discriminate AKI etiology, and to assess AKI severity (73). In CRS, biomarkers may assist our understanding of the interaction of heart-kidney injury. Kidney damage biomarkers (e.g., plasma/urinary NGAL, urinary IL-18, urinary KIM-1, urinary L-FABP, urinary NAG and urinary angiotensinogen) and cell cycle arrest biomarkers (e.g., urinary [TIMP2]•[IGFBP7]) may inform in CRS type 1, and cardiac damage biomarkers (e.g., troponin and BNP) in CRS type 3 and 4. In contrast, the utility of biomarkers in CRS type 2 and 5 require further study though potentially more sensitive markers of GFR (e.g., plasma cystatin-C and proenkephalin A) may be inform in CRS type 2, plasma galectin-3 may inform in both CRS type 2 and 4 since it is both a marker of GFR and cardiac fibrosis, and CRS type 5 likely requires a combination of biomarkers since it reflects simultaneous heart and kidney injury. However, interventions in CRS remain limited and few existing biomarkers (e.g., albuminuria, plasma galectin-3, urinary angiotensinogen, plasma BNP and NT-proBNP) offer insights into therapeutic targets that may benefit patients with CRS.
Loop diuretics remain the first-line treatment for fluid removal in HF, however, animal studies raise concerns regarding frusemide-mediated RAAS activation and subsequent myocardial and renal fibrosis (3). While aldosterone-mediated fibrosis in CRS suggest RAAS inhibitors and mineralocorticoid receptor antagonists may be beneficial, the risk of hyperkalemia or AKI may limit their use (74). The thrombospondin-1/CD47 axis, glycocalyx and IL-1β are emerging biomarkers in cardiovascular disease which may also provide prognostic value and therapeutic targets in CRS.
TSP-1 is a 480 kDa matricellular protein secreted by tissue in response to hypoxia and binds to the ubiquitously expressed CD47 to limit nitric oxide (NO)-mediated vasodilation, thereby limiting tissue perfusion (75, 76). The TSP-1/CD47 axis has been implicated in renal ischemia reperfusion injury (IRI), (77, 78) atherosclerosis, (79) endothelial dysfunction, (80, 81) pulmonary hypertension, (82) and vaso-occlusive events in sickle cell anemia (83). In microarray analysis of peripheral blood samples, TSP-1 outperformed BNP and cTnT at predicting HF after myocardial infarction (AUC-ROC 0.82 vs. 0.63), (84) and plasma TSP-1 levels are also increased in people with CKD (85).
In human hearts after autopsy and experimental myocardial infarction in mice, CD47 is upregulated on cardiomyocytes and inhibited phagocytosis of apoptotic cardiomyocytes by macrophages (86). CD47 inhibition ameliorated myocardial infarction in mice and rats by enhancing myocardial phagocytosis, resolving monocyte infiltration, increasing endothelial nitric oxide synthase activity and reducing oxidative stress, resulting in reduced infarct size, reduced cardiac fibrosis, and improved left ventricular ejection fraction (86, 87). Anti-CD47 blockade also successfully ameliorated kidney fibrosis in a renal IRI model and reduced expression of fibrosis markers such as transforming growth factor (TGF)-β, SMAD2 and connective tissue growth factor (85). More relevant to CRS, microRNA-221 inhibits TSP-1 upregulation, reduces TGF-β1-mediated cardiac fibrosis, and improves cardiac function and survival in 5/6 nephrectomy rats, a model of CKD (88). Overall, the TSP-1/CD47 axis represents a promising biomarker and therapeutic target in CRS.
The glycocalyx is a 0.5–8 μm thick carbohydrate-rich structure composed of glycoproteins (e.g., syndecan-1) bound to glycosaminoglycan side-chains (e.g., heparan sulfate and hyaluronan), which overlies vascular endothelial cells and governs its barrier function as well as antiadhesive and anticoagulant properties (89, 90). Degradation of the glycocalyx has been proposed as an early marker of endothelial dysfunction, which has been increasingly recognized as a critical process in CRS (11, 91–93). In acute HF, elevated serum syndecan-1 at hospital admission predicted AKI (AUC-ROC 0.741), severe AKI (AUC-ROC 0.812) and in-hospital death (AUC-ROC 0.788), and was associated with a 1.3-fold increased risk of all-cause death at 6 months in multivariate analysis (94). In chronic HF, elevated serum syndecan-1 was prognostic in HF with preserved ejection fraction (2-fold increased risk of all-cause death or hospitalization) but not HF with reduced ejection fraction (95).
Restoration of the glycocalyx using a novel selectin-targeting glycocalyx mimetic (DS-IkL) reduced selectin-mediated neutrophil and macrophage infiltration, endothelial cell and fibroblast proliferation, and cardiac fibrosis after myocardial infarction in mice (96). Therapies with proven safety in humans repurposed for glycocalyx regeneration (e.g., albumin, sulodexide, fresh frozen plasma, hydrocortisone, etanercept, rosuvastatin, metformin, and heparin) may also represent potential novel treatments in CRS (97–111).
Similar to IL-18, IL-1β is involved in activation of the NLRP3-inflammasome in myocardial and kidney injury. Rademaker et al. recently performed RNA sequencing on serial kidney biopsies in an ovine model of acute HF and identified 675 differentially expressed genes with human homologs that were enriched for 9 pathways, of which IL-1β was the top-predicted upstream regulator gene (112). Canakinumab, a humanized monoclonal antibody targeting IL-1β, reduced cardiovascular events by 15% in 10,061 patients with previous myocardial infarction though at the cost of increased fatal infections (113). Similar results were reported in the CKD subgroup (114). Whether canakinumab or other biomarkers identified in the study by Rademaker et al. have a role in the treatment of CRS require further study.
Cardiorenal syndrome is an increasingly common condition in the aging multimorbid population with significant health burden and few effective treatments. Current studies of biomarkers in CRS have largely focused on prognostication and clinical translation has been limited by sparse data comparing them to traditional biomarkers such as serum creatinine. Furthermore, few biomarkers offer insights into the mechanistic basis of disease needed to inform therapeutic strategies. In this review, we propose the TSP-1/CD47 axis, glycocalyx and IL-1β as promising areas for future research in CRS, which have the potential to prognosticate and direct treatments in this complex condition.
EC, NR, and SA conceptualized review topic. EC, KT, JL, SH, ZE, NR, and SA contributed to data interpretation. ZE, NR, and SA contributed to supervision. All authors contributed to important intellectual content during manuscript drafting or revision and agrees to be personally accountable for the individual’s own contributions and to ensure that questions pertaining to the accuracy or integrity of any portion of the work, even one in which the author was not directly involved, are appropriately investigated and resolved, including with documentation in the literature if appropriate.
EC is supported by the NHMRC Postgraduate Research Scholarship (APP 2014037).
The authors declare that the research was conducted in the absence of any commercial or financial relationships that could be construed as a potential conflict of interest.
The handling editor YL declared a past collaboration with the author EC.
All claims expressed in this article are solely those of the authors and do not necessarily represent those of their affiliated organizations, or those of the publisher, the editors and the reviewers. Any product that may be evaluated in this article, or claim that may be made by its manufacturer, is not guaranteed or endorsed by the publisher.
1. Ziaeian B, Fonarow GC. Epidemiology and aetiology of heart failure. Nat Rev Cardiol. (2016) 13:368–78.
2. Bikbov B, Purcell CA, Levey AS, Smith M, Abdoli A, Abebe M, et al. Global, regional, and national burden of chronic kidney disease, 1990-2017: a systematic analysis for the global burden of disease study 2017. Lancet. (2020) 395:709–33. doi: 10.1016/S0140-6736(20)30045-3
3. Bock JS, Gottlieb SS. Cardiorenal syndrome: new perspectives. Circulation. (2010) 121:2592–600. doi: 10.1161/CIRCULATIONAHA.109.886473
4. Dries DL, Exner DV, Domanski MJ, Greenberg B, Stevenson LW. The prognostic implications of renal insufficiency in asymptomatic and symptomatic patients with left ventricular systolic dysfunction. J Am Coll Cardiol. (2000) 35:681–9. doi: 10.1016/s0735-1097(99)00608-7
5. Hillege HL, Nitsch D, Pfeffer MA, Swedberg K, McMurray JJ, Yusuf S, et al. Renal function as a predictor of outcome in a broad spectrum of patients with heart failure. Circulation. (2006) 113:671–8. doi: 10.1161/circulationaha.105.580506
6. Mann JF, Gerstein HC, Pogue J, Bosch J, Yusuf S. Renal insufficiency as a predictor of cardiovascular outcomes and the impact of ramipril: the HOPE randomized trial. Ann Intern Med. (2001) 134:629–36. doi: 10.7326/0003-4819-134-8-200104170-00007
7. Shlipak MG, Katz R, Kestenbaum B, Siscovick D, Fried L, Newman A, et al. Rapid decline of kidney function increases cardiovascular risk in the elderly. J Am Soc Nephrol. (2009) 20:2625–30. doi: 10.1681/ASN.2009050546
8. Smith GL, Lichtman JH, Bracken MB, Shlipak MG, Phillips CO, DiCapua P, et al. Renal impairment and outcomes in heart failure: systematic review and meta-analysis. J Am Coll Cardiol. (2006) 47:1987–96. doi: 10.1016/j.jacc.2005.11.084
9. Sud M, Tangri N, Pintilie M, Levey AS, Naimark DM. ESRD and death after heart failure in CKD. J Am Soc Nephrol. (2015) 26:715–22. doi: 10.1681/ASN.2014030253
10. Ronco C, Haapio M, House AA, Anavekar N, Bellomo R. Cardiorenal syndrome. J Am Coll Cardiol. (2008) 52:1527–39.
11. Hatamizadeh P, Fonarow GC, Budoff MJ, Darabian S, Kovesdy CP, Kalantar-Zadeh K. Cardiorenal syndrome: pathophysiology and potential targets for clinical management. Nat Rev Nephrol. (2013) 9:99–111. doi: 10.1038/nrneph.2012.279
12. Ronco C, Cicoira M, McCullough PA. Cardiorenal syndrome type 1: pathophysiological crosstalk leading to combined heart and kidney dysfunction in the setting of acutely decompensated heart failure. J Am Coll Cardiol. (2012) 60:1031–42. doi: 10.1016/j.jacc.2012.01.077
13. Slocum JL, Heung M, Pennathur S. Marking renal injury: can we move beyond serum creatinine? Transl Res. (2012) 159:277–89. doi: 10.1016/j.trsl.2012.01.014
14. Bouquegneau A, Krzesinski JM, Delanaye P, Cavalier E. Biomarkers and physiopathology in the cardiorenal syndrome. Clin Chim Acta. (2015) 443:100–7. doi: 10.1016/j.cca.2014.10.041
15. Anand IS, Bishu K, Rector TS, Ishani A, Kuskowski MA, Cohn JN. Proteinuria, chronic kidney disease, and the effect of an angiotensin receptor blocker in addition to an angiotensin-converting enzyme inhibitor in patients with moderate to severe heart failure. Circulation. (2009) 120:1577–84. doi: 10.1161/CIRCULATIONAHA.109.853648
16. Jackson CE, Solomon SD, Gerstein HC, Zetterstrand S, Olofsson B, Michelson EL, et al. Albuminuria in chronic heart failure: prevalence and prognostic importance. Lancet. (2009) 374:543–50. doi: 10.1016/S0140-6736(09)61378-7
17. Masson S, Latini R, Milani V, Moretti L, Rossi MG, Carbonieri E, et al. Prevalence and prognostic value of elevated urinary albumin excretion in patients with chronic heart failure: data from the GISSI-heart failure trial. Circ Heart Fail. (2010) 3:65–72. doi: 10.1161/CIRCHEARTFAILURE.109.881805
18. Bengtsson E, To F, Håkansson K, Grubb A, Brånén L, Nilsson J, et al. Lack of the cysteine protease inhibitor cystatin C promotes atherosclerosis in apolipoprotein E-deficient mice. Arterioscler Thromb Vasc Biol. (2005) 25:2151–6. doi: 10.1161/01.ATV.0000179600.34086.7d
19. Arimoto T, Takeishi Y, Niizeki T, Takabatake N, Okuyama H, Fukui A, et al. Cystatin C, a novel measure of renal function, is an independent predictor of cardiac events in patients with heart failure. J Card Fail. (2005) 11:595–601. doi: 10.1016/j.cardfail.2005.06.001
20. Lassus J, Harjola VP, Sund R, Siirilä-Waris K, Melin J, Peuhkurinen K, et al. Prognostic value of cystatin C in acute heart failure in relation to other markers of renal function and NT-proBNP. Eur Heart J. (2007) 28:1841–7. doi: 10.1093/eurheartj/ehl507
21. Shlipak MG, Katz R, Fried LF, Jenny NS, Stehman-Breen C, Newman AB, et al. Cystatin-C and mortality in elderly persons with heart failure. J Am Coll Cardiol. (2005) 45:268–71. doi: 10.1016/j.jacc.2004.09.061
22. Sokolski M, Zymliński R, Biegus J, Siwołowski P, Nawrocka-Millward S, Todd J, et al. Urinary levels of novel kidney biomarkers and risk of true worsening renal function and mortality in patients with acute heart failure. Eur J Heart Fail. (2017) 19:760–7. doi: 10.1002/ejhf.746
23. Alvelos M, Pimentel R, Pinho E, Gomes A, Lourenço P, Teles MJ, et al. Neutrophil gelatinase-associated lipocalin in the diagnosis of type 1 cardio-renal syndrome in the general ward. Clin J Am Soc Nephrol. (2011) 6:476–81. doi: 10.2215/CJN.06140710
24. Carrasco-Sánchez FJ, Galisteo-Almeda L, Páez-Rubio I, Martínez-Marcos FJ, Camacho-Vázquez C, Ruiz-Frutos C, et al. Prognostic value of cystatin C on admission in heart failure with preserved ejection fraction. J Card Fail. (2011) 17:31–8. doi: 10.1016/j.cardfail.2010.07.248
25. Manzano-Fernández S, Boronat-Garcia M, Albaladejo-Otón MD, Pastor P, Garrido IP, Pastor-Pérez FJ, et al. Complementary prognostic value of cystatin C, N-terminal pro-B-type natriuretic peptide and cardiac troponin T in patients with acute heart failure. Am J Cardiol. (2009) 103:1753–9. doi: 10.1016/j.amjcard.2009.02.029
26. Wald R, Liangos O, Perianayagam MC, Kolyada A, Herget-Rosenthal S, Mazer CD, et al. Plasma cystatin C and acute kidney injury after cardiopulmonary bypass. Clin J Am Soc Nephrol. (2010) 5:1373–9. doi: 10.2215/CJN.06350909
27. Koyner JL, Vaidya VS, Bennett MR, Ma Q, Worcester E, Akhter SA, et al. Urinary biomarkers in the clinical prognosis and early detection of acute kidney injury. Clin J Am Soc Nephrol. (2010) 5:2154–65. doi: 10.2215/CJN.00740110
28. Liangos O, Tighiouart H, Perianayagam MC, Kolyada A, Han WK, Wald R, et al. Comparative analysis of urinary biomarkers for early detection of acute kidney injury following cardiopulmonary bypass. Biomarkers. (2009) 14:423–31. doi: 10.1080/13547500903067744
29. Ahmad T, Jackson K, Rao VS, Tang WHW, Brisco-Bacik MA, Chen HH, et al. Worsening renal function in patients with acute heart failure undergoing aggressive diuresis is not associated with tubular injury. Circulation. (2018) 137:2016–28. doi: 10.1161/circulationaha.117.030112
30. van Kimmenade RR, Januzzi JL Jr, Ellinor PT, Sharma UC, Bakker JA, Low AF, et al. Utility of amino-terminal pro-brain natriuretic peptide, galectin-3, and apelin for the evaluation of patients with acute heart failure. J Am Coll Cardiol. (2006) 48:1217–24. doi: 10.1016/j.jacc.2006.03.061
31. Lok DJ, Van Der Meer P, de la Porte PW, Lipsic E, Van Wijngaarden J, Hillege HL, et al. Prognostic value of galectin-3, a novel marker of fibrosis, in patients with chronic heart failure: data from the DEAL-HF study. Clin Res Cardiol. (2010) 99:323–8. doi: 10.1007/s00392-010-0125-y
32. Meijers WC, Januzzi JL, deFilippi C, Adourian AS, Shah SJ, van Veldhuisen DJ, et al. Elevated plasma galectin-3 is associated with near-term rehospitalization in heart failure: a pooled analysis of 3 clinical trials. Am Heart J. (2014) 167:853–60.e4. doi: 10.1016/j.ahj.2014.02.011
33. van der Velde AR, Gullestad L, Ueland T, Aukrust P, Guo Y, Adourian A, et al. Prognostic value of changes in galectin-3 levels over time in patients with heart failure: data from CORONA and COACH. Circ Heart Fail. (2013) 6:219–26. doi: 10.1161/CIRCHEARTFAILURE.112.000129
34. Schulz CA, Christensson A, Ericson U, Almgren P, Hindy G, Nilsson PM, et al. High level of fasting plasma proenkephalin-a predicts deterioration of kidney function and incidence of CKD. J Am Soc Nephrol. (2017) 28:291–303. doi: 10.1681/ASN.2015101177
35. Ng LL, Squire IB, Jones DJL, Cao TH, Chan DCS, Sandhu JK, et al. Proenkephalin, renal dysfunction, and prognosis in patients with acute heart failure: a GREAT network study. J Am Coll Cardiol. (2017) 69:56–69. doi: 10.1016/j.jacc.2016.10.038
36. Tarjus A, Martínez-Martínez E, Amador C, Latouche C, El Moghrabi S, Berger T, et al. Neutrophil gelatinase-associated lipocalin, a novel mineralocorticoid biotarget, mediates vascular profibrotic effects of mineralocorticoids. Hypertension. (2015) 66:158–66. doi: 10.1161/HYPERTENSIONAHA.115.05431
37. Martínez-Martínez E, Buonafine M, Boukhalfa I, Ibarrola J, Fernández-Celis A, Kolkhof P, et al. Aldosterone target NGAL (neutrophil gelatinase-associated lipocalin) is involved in cardiac remodeling after myocardial infarction through NFκB pathway. Hypertension. (2017) 70:1148–56. doi: 10.1161/HYPERTENSIONAHA.117.09791
38. Mishra J, Dent C, Tarabishi R, Mitsnefes MM, Ma Q, Kelly C, et al. Neutrophil gelatinase-associated lipocalin (NGAL) as a biomarker for acute renal injury after cardiac surgery. Lancet. (2005) 365:1231–8. doi: 10.1016/S0140-6736(05)74811-X
39. Krawczeski CD, Woo JG, Wang Y, Bennett MR, Ma Q, Devarajan P. Neutrophil gelatinase-associated lipocalin concentrations predict development of acute kidney injury in neonates and children after cardiopulmonary bypass. J Pediatr. (2011) 158:1009–15.e1. doi: 10.1016/j.jpeds.2010.12.057
40. Prabhu A, Sujatha DI, Ninan B, Vijayalakshmi MA. Neutrophil gelatinase associated lipocalin as a biomarker for acute kidney injury in patients undergoing coronary artery bypass grafting with cardiopulmonary bypass. Ann Vasc Surg. (2010) 24:525–31. doi: 10.1016/j.avsg.2010.01.001
41. Haase M, Bellomo R, Devarajan P, Schlattmann P, Haase-Fielitz A. Accuracy of neutrophil gelatinase-associated lipocalin (NGAL) in diagnosis and prognosis in acute kidney injury: a systematic review and meta-analysis. Am J Kidney Dis. (2009) 54:1012–24. doi: 10.1053/j.ajkd.2009.07.020
42. Parikh CR, Devarajan P, Zappitelli M, Sint K, Thiessen-Philbrook H, Li S, et al. Postoperative biomarkers predict acute kidney injury and poor outcomes after pediatric cardiac surgery. J Am Soc Nephrol. (2011) 22:1737–47. doi: 10.1681/ASN.2010111163
43. Endre ZH, Pickering JW, Walker RJ, Devarajan P, Edelstein CL, Bonventre JV, et al. Improved performance of urinary biomarkers of acute kidney injury in the critically ill by stratification for injury duration and baseline renal function. Kidney Int. (2011) 79:1119–30. doi: 10.1038/ki.2010.555
44. van Deursen VM, Damman K, Voors AA, van der Wal MH, Jaarsma T, van Veldhuisen DJ, et al. Prognostic value of plasma neutrophil gelatinase-associated lipocalin for mortality in patients with heart failure. Circ Heart Fail. (2014) 7:35–42. doi: 10.1161/CIRCHEARTFAILURE.113.000242
45. Mortara A, Bonadies M, Mazzetti S, Fracchioni I, Delfino P, Chioffi M, et al. Neutrophil gelatinase-associated lipocalin predicts worsening of renal function in acute heart failure: methodological and clinical issues. J Cardiovasc Med (Hagerstown). (2013) 14:629–34. doi: 10.2459/JCM.0b013e3283629ca6
46. Chen C, Yang X, Lei Y, Zha Y, Liu H, Ma C, et al. Urinary biomarkers at the time of AKI diagnosis as predictors of progression of AKI among patients with acute cardiorenal syndrome. Clin J Am Soc Nephrol. (2016) 11:1536–44. doi: 10.2215/CJN.00910116
47. Verbrugge FH, Dupont M, Shao Z, Shrestha K, Singh D, Finucan M, et al. Novel urinary biomarkers in detecting acute kidney injury, persistent renal impairment, and all-cause mortality following decongestive therapy in acute decompensated heart failure. J Card Fail. (2013) 19:621–8.
48. Damman K, Masson S, Hillege HL, Maggioni AP, Voors AA, Opasich C, et al. Clinical outcome of renal tubular damage in chronic heart failure. Eur Heart J. (2011) 32:2705–12. doi: 10.1093/eurheartj/ehr190
49. Damman K, Van Veldhuisen DJ, Navis G, Vaidya VS, Smilde TD, Westenbrink BD, et al. Tubular damage in chronic systolic heart failure is associated with reduced survival independent of glomerular filtration rate. Heart. (2010) 96:1297–302. doi: 10.1136/hrt.2010.194878
50. Liang XL, Liu SX, Chen YH, Yan LJ, Li H, Xuan HJ, et al. Combination of urinary kidney injury molecule-1 and interleukin-18 as early biomarker for the diagnosis and progressive assessment of acute kidney injury following cardiopulmonary bypass surgery: a prospective nested case-control study. Biomarkers. (2010) 15:332–9. doi: 10.3109/13547501003706558
51. Han WK, Waikar SS, Johnson A, Betensky RA, Dent CL, Devarajan P, et al. Urinary biomarkers in the early diagnosis of acute kidney injury. Kidney Int. (2008) 73:863–9.
52. Coca SG, Garg AX, Thiessen-Philbrook H, Koyner JL, Patel UD, Krumholz HM, et al. Urinary biomarkers of AKI and mortality 3 years after cardiac surgery. J Am Soc Nephrol. (2014) 25:1063–71. doi: 10.1681/ASN.2013070742
53. Anders HJ, Muruve DA. The inflammasomes in kidney disease. J Am Soc Nephrol. (2011) 22:1007–18. doi: 10.1681/asn.2010080798
54. O’Brien LC, Mezzaroma E, Van Tassell BW, Marchetti C, Carbone S, Abbate A, et al. Interleukin-18 as a therapeutic target in acute myocardial infarction and heart failure. Mol Med. (2014) 20:221–9. doi: 10.2119/molmed.2014.00034
55. Miao N, Yin F, Xie H, Wang Y, Xu Y, Shen Y, et al. The cleavage of gasdermin D by caspase-11 promotes tubular epithelial cell pyroptosis and urinary IL-18 excretion in acute kidney injury. Kidney Int. (2019) 96:1105–20. doi: 10.1016/j.kint.2019.04.035
56. Parikh CR, Mishra J, Thiessen-Philbrook H, Dursun B, Ma Q, Kelly C, et al. Urinary IL-18 is an early predictive biomarker of acute kidney injury after cardiac surgery. Kidney Int. (2006) 70:199–203. doi: 10.1038/sj.ki.5001527
57. Veerkamp JH, Paulussen RJ, Peeters RA, Maatman RG, van Moerkerk HT, van Kuppevelt TH. Detection, tissue distribution and (sub)cellular localization of fatty acid-binding protein types. Mol Cell Biochem. (1990) 98:11–8. doi: 10.1007/BF00231362
58. Katagiri D, Doi K, Honda K, Negishi K, Fujita T, Hisagi M, et al. Combination of two urinary biomarkers predicts acute kidney injury after adult cardiac surgery. Ann Thorac Surg. (2012) 93:577–83. doi: 10.1016/j.athoracsur.2011.10.048
59. Yancy CW, Jessup M, Bozkurt B, Butler J, Casey DE Jr, Colvin MM, et al. 2017 ACC/AHA/HFSA focused update of the 2013 ACCF/AHA guideline for the management of heart failure: a report of the American college of cardiology/American heart association task force on clinical practice guidelines and the heart failure society of America. Circulation. (2017) 136:e137–61.
60. Scheven L, de Jong PE, Hillege HL, Lambers Heerspink HJ, van Pelt LJ, Kootstra JE, et al. High-sensitive troponin T and N-terminal pro-B type natriuretic peptide are associated with cardiovascular events despite the cross-sectional association with albuminuria and glomerular filtration rate. Eur Heart J. (2012) 33:2272–81. doi: 10.1093/eurheartj/ehs163
61. Hasegawa M, Ishii J, Kitagawa F, Kanayama K, Takahashi H, Ozaki Y, et al. Prognostic value of highly sensitive troponin T on cardiac events in patients with chronic kidney disease not on dialysis. Heart Vessels. (2013) 28:473–9. doi: 10.1007/s00380-012-0273-2
62. Chrysochou C, Manzoor S, Wright J, Roberts SA, Wood G, McDowell G, et al. Role of renal function and cardiac biomarkers (NT-proBNP and Troponin) in determining mortality and cardiac outcome in atheromatous renovascular disease. Kidney Blood Press Res. (2009) 32:373–9. doi: 10.1159/000254337
63. Ryu DR, Park JT, Chung JH, Song EM, Roh SH, Lee JM, et al. A more appropriate cardiac troponin T level that can predict outcomes in end-stage renal disease patients with acute coronary syndrome. Yonsei Med J. (2011) 52:595–602. doi: 10.3349/ymj.2011.52.4.595
64. Chenevier-Gobeaux C, Meune C, Freund Y, Wahbi K, Claessens YE, Doumenc B, et al. Influence of age and renal function on high-sensitivity cardiac troponin T diagnostic accuracy for the diagnosis of acute myocardial infarction. Am J Cardiol. (2013) 111:1701–7. doi: 10.1016/j.amjcard.2013.02.024
65. Panteghini M, Clerico A. Understanding the clinical biochemistry of N-terminal pro-B-type natriuretic peptide: the prerequisite for its optimal clinical use. Clin Lab. (2004) 50:325–31.
66. Horii M, Matsumoto T, Uemura S, Sugawara Y, Takitsume A, Ueda T, et al. Prognostic value of B-type natriuretic peptide and its amino-terminal proBNP fragment for cardiovascular events with stratification by renal function. J Cardiol. (2013) 61:410–6. doi: 10.1016/j.jjcc.2013.01.015
67. Astor BC, Yi S, Hiremath L, Corbin T, Pogue V, Wilkening B, et al. N-terminal prohormone brain natriuretic peptide as a predictor of cardiovascular disease and mortality in blacks with hypertensive kidney disease: the African American study of kidney disease and hypertension (AASK). Circulation. (2008) 117:1685–92. doi: 10.1161/CIRCULATIONAHA.107.724187
68. Bihorac A, Chawla LS, Shaw AD, Al-Khafaji A, Davison DL, Demuth GE, et al. Validation of cell-cycle arrest biomarkers for acute kidney injury using clinical adjudication. Am J Respir Crit Care Med. (2014) 189:932–9. doi: 10.1164/rccm.201401-0077OC
69. Gunnerson KJ, Shaw AD, Chawla LS, Bihorac A, Al-Khafaji A, Kashani K, et al. TIMP2IGFBP7 biomarker panel accurately predicts acute kidney injury in high-risk surgical patients. J Trauma Acute Care Surg. (2016) 80:243–9. doi: 10.1097/TA.0000000000000912
70. Kashani K, Al-Khafaji A, Ardiles T, Artigas A, Bagshaw SM, Bell M, et al. Discovery and validation of cell cycle arrest biomarkers in human acute kidney injury. Crit Care. (2013) 17:R25. doi: 10.1186/cc12503
71. Meersch M, Schmidt C, Van Aken H, Martens S, Rossaint J, Singbartl K, et al. Urinary TIMP-2 and IGFBP7 as early biomarkers of acute kidney injury and renal recovery following cardiac surgery. PLoS One. (2014) 9:e93460. doi: 10.1371/journal.pone.0093460
72. Atici A, Emet S, Cakmak R, Yuruyen G, Alibeyoglu A, Akarsu M, et al. Type I cardiorenal syndrome in patients with acutely decompensated heart failure: the importance of new renal biomarkers. Eur Rev Med Pharmacol Sci. (2018) 22:3534–43. doi: 10.26355/eurrev_201806_15180
73. Ostermann M, Zarbock A, Goldstein S, Kashani K, Macedo E, Murugan R, et al. Recommendations on acute kidney injury biomarkers from the acute disease quality initiative consensus conference: a consensus statement. JAMA Netw Open. (2020) 3:e2019209. doi: 10.1001/jamanetworkopen.2020.19209
74. Zannad F, Rossignol P. Cardiorenal syndrome revisited. Circulation. (2018) 138:929–44. doi: 10.1161/circulationaha.117.028814
75. Isenberg JS, Romeo MJ, Abu-Asab M, Tsokos M, Oldenborg A, Pappan L, et al. Increasing survival of ischemic tissue by targeting CD47. Circ Res. (2007) 100:712–20. doi: 10.1161/01.RES.0000259579.35787.4e
76. Rogers NM, Yao M, Novelli EM, Thomson AW, Roberts DD, Isenberg JS. Activated CD47 regulates multiple vascular and stress responses: implications for acute kidney injury and its management. Am J Physiol Renal Physiol. (2012) 303:F1117–25. doi: 10.1152/ajprenal.00359.2012
77. Rogers NM, Thomson AW, Isenberg JS. Activation of parenchymal CD47 promotes renal ischemia-reperfusion injury. J Am Soc Nephrol. (2012) 23:1538–50. doi: 10.1681/ASN.2012020137
78. Yao M, Rogers NM, Csányi G, Rodriguez AI, Ross MA, St Croix C, et al. Thrombospondin-1 activation of signal-regulatory protein-α stimulates reactive oxygen species production and promotes renal ischemia reperfusion injury. J Am Soc Nephrol. (2014) 25:1171–86. doi: 10.1681/ASN.2013040433
79. Riessen R, Kearney M, Lawler J, Isner JM. Immunolocalization of thrombospondin-1 in human atherosclerotic and restenotic arteries. Am Heart J. (1998) 135:357–64. doi: 10.1016/s0002-8703(98)70105-x
80. Bauer EM, Qin Y, Miller TW, Bandle RW, Csanyi G, Pagano PJ, et al. Thrombospondin-1 supports blood pressure by limiting eNOS activation and endothelial-dependent vasorelaxation. Cardiovasc Res. (2010) 88:471–81. doi: 10.1093/cvr/cvq218
81. Moura R, Tjwa M, Vandervoort P, Cludts K, Hoylaerts MF. Thrombospondin-1 activates medial smooth muscle cells and triggers neointima formation upon mouse carotid artery ligation. Arterioscler Thromb Vasc Biol. (2007) 27:2163–9. doi: 10.1161/ATVBAHA.107.151282
82. Rogers NM, Ghimire K, Calzada MJ, Isenberg JS. Matricellular protein thrombospondin-1 in pulmonary hypertension: multiple pathways to disease. Cardiovasc Res. (2017) 113:858–68. doi: 10.1093/cvr/cvx094
83. Novelli EM, Kato GJ, Ragni MV, Zhang Y, Hildesheim ME, Nouraie M, et al. Plasma thrombospondin-1 is increased during acute sickle cell vaso-occlusive events and associated with acute chest syndrome, hydroxyurea therapy, and lower hemolytic rates. Am J Hematol. (2012) 87:326–30. doi: 10.1002/ajh.22274
84. Devaux Y, Azuaje F, Vausort M, Yvorra C, Wagner DR. Integrated protein network and microarray analysis to identify potential biomarkers after myocardial infarction. Funct Integr Genomics. (2010) 10:329–37. doi: 10.1007/s10142-010-0169-0
85. Julovi SM, Sanganeria B, Minhas N, Ghimire K, Nankivell B, Rogers NM. Blocking thrombospondin-1 signaling via CD47 mitigates renal interstitial fibrosis. Lab Invest. (2020) 100:1184–96. doi: 10.1038/s41374-020-0434-3
86. Zhang S, Yeap XY, DeBerge M, Naresh NK, Wang K, Jiang Z, et al. Acute CD47 blockade during ischemic myocardial reperfusion enhances phagocytosis-associated cardiac repair. JACC Basic Transl Sci. (2017) 2:386–97. doi: 10.1016/j.jacbts.2017.03.013
87. Wang HB, Yang J, Ding JW, Chen LH, Li S, Liu XW, et al. RNAi-mediated down-regulation of CD47 protects against ischemia/reperfusion-induced myocardial damage via activation of eNOS in a rat model. Cell Physiol Biochem. (2016) 40:1163–74. doi: 10.1159/000453170
88. Zhou Y, Ng DYE, Richards AM, Wang P. microRNA-221 inhibits latent TGF-β1 activation through targeting thrombospondin-1 to attenuate kidney failure-induced cardiac fibrosis. Mol Ther Nucleic Acids. (2020) 22:803–14. doi: 10.1016/j.omtn.2020.09.041
89. Liew H, Roberts MA, Pope A, McMahon LP. Endothelial glycocalyx damage in kidney disease correlates with uraemic toxins and endothelial dysfunction. BMC Nephrol. (2021) 22:21. doi: 10.1186/s12882-020-02219-4
90. Kim YH, Nijst P, Kiefer K, Tang WH. Endothelial glycocalyx as biomarker for cardiovascular diseases: mechanistic and clinical implications. Curr Heart Fail Rep. (2017) 14:117–26. doi: 10.1007/s11897-017-0320-5
91. Cohen L. The cardiorenal syndrome: pathophysiologic crosstalk, outcomes, and treatment targets. Cardiovasc Hematol Disord Drug Targets. (2014) 14:170–6. doi: 10.2174/1871529x14666140701100913
92. Jia G, Aroor AR, Sowers JR. Arterial stiffness: a nexus between cardiac and renal disease. Cardiorenal Med. (2014) 4:60–71. doi: 10.1159/000360867
93. Zhang J, Bottiglieri T, McCullough PA. The central role of endothelial dysfunction in cardiorenal syndrome. Cardiorenal Med. (2017) 7:104–17. doi: 10.1159/000452283
94. Neves FM, Meneses GC, Sousa NE, Menezes RR, Parahyba MC, Martins AM, et al. Syndecan-1 in acute decompensated heart failure–association with renal function and mortality. Circ J. (2015) 79:1511–9.
95. Tromp J, van der Pol A, Klip IT, de Boer RA, Jaarsma T, van Gilst WH, et al. Fibrosis marker syndecan-1 and outcome in patients with heart failure with reduced and preserved ejection fraction. Circ Heart Fail. (2014) 7:457–62. doi: 10.1161/CIRCHEARTFAILURE.113.000846
96. Dehghani T, Thai PN, Sodhi H, Ren L, Sirish P, Nader CE, et al. Selectin-targeting glycosaminoglycan-peptide conjugate limits neutrophil mediated cardiac reperfusion injury. Cardiovasc Res. (2020) 118:267–81. doi: 10.1093/cvr/cvaa312
97. Jacob M, Paul O, Mehringer L, Chappell D, Rehm M, Welsch U, et al. Albumin augmentation improves condition of guinea pig hearts after 4 hr of cold ischemia. Transplantation. (2009) 87:956–65. doi: 10.1097/TP.0b013e31819c83b5
98. Adamson RH, Clark JF, Radeva M, Kheirolomoom A, Ferrara KW, Curry FE. Albumin modulates S1P delivery from red blood cells in perfused microvessels: mechanism of the protein effect. Am J Physiol Heart Circ Physiol. (2014) 306:H1011–7. doi: 10.1152/ajpheart.00829.2013
99. Zeng Y, Adamson RH, Curry FR, Tarbell JM. Sphingosine-1-phosphate protects endothelial glycocalyx by inhibiting syndecan-1 shedding. Am J Physiol Heart Circ Physiol. (2014) 306:H363–72. doi: 10.1152/ajpheart.00687.2013
100. Broekhuizen LN, Lemkes BA, Mooij HL, Meuwese MC, Verberne H, Holleman F, et al. Effect of sulodexide on endothelial glycocalyx and vascular permeability in patients with type 2 diabetes mellitus. Diabetologia. (2010) 53:2646–55. doi: 10.1007/s00125-010-1910-x
101. Song JW, Zullo JA, Liveris D, Dragovich M, Zhang XF, Goligorsky MS. Therapeutic restoration of endothelial glycocalyx in sepsis. J Pharmacol Exp Ther. (2017) 361:115–21. doi: 10.1124/jpet.116.239509
102. Li T, Liu X, Zhao Z, Ni L, Liu C. Sulodexide recovers endothelial function through reconstructing glycocalyx in the balloon-injury rat carotid artery model. Oncotarget. (2017) 8:91350–61. doi: 10.18632/oncotarget.20518
103. Torres LN, Sondeen JL, Ji L, Dubick MA, Torres Filho I. Evaluation of resuscitation fluids on endothelial glycocalyx, venular blood flow, and coagulation function after hemorrhagic shock in rats. J Trauma Acute Care Surg. (2013) 75:759–66. doi: 10.1097/TA.0b013e3182a92514
104. Haywood-Watson RJ, Holcomb JB, Gonzalez EA, Peng Z, Pati S, Park PW, et al. Modulation of syndecan-1 shedding after hemorrhagic shock and resuscitation. PLoS One. (2011) 6:e23530. doi: 10.1371/journal.pone.0023530
105. Chappell D, Hofmann-Kiefer K, Jacob M, Rehm M, Briegel J, Welsch U, et al. TNF-alpha induced shedding of the endothelial glycocalyx is prevented by hydrocortisone and antithrombin. Basic Res Cardiol. (2009) 104:78–89. doi: 10.1007/s00395-008-0749-5
106. Chappell D, Jacob M, Hofmann-Kiefer K, Bruegger D, Rehm M, Conzen P, et al. Hydrocortisone preserves the vascular barrier by protecting the endothelial glycocalyx. Anesthesiology. (2007) 107:776–84. doi: 10.1097/01.anes.0000286984.39328.96
107. Nieuwdorp M, Meuwese MC, Mooij HL, van Lieshout MH, Hayden A, Levi M, et al. Tumor necrosis factor-alpha inhibition protects against endotoxin-induced endothelial glycocalyx perturbation. Atherosclerosis. (2009) 202:296–303. doi: 10.1016/j.atherosclerosis.2008.03.024
108. Meuwese MC, Mooij HL, Nieuwdorp M, van Lith B, Marck R, Vink H, et al. Partial recovery of the endothelial glycocalyx upon rosuvastatin therapy in patients with heterozygous familial hypercholesterolemia. J Lipid Res. (2009) 50:148–53. doi: 10.1194/jlr.P800025-JLR200
109. Eskens BJ, Zuurbier CJ, van Haare J, Vink H, van Teeffelen JW. Effects of two weeks of metformin treatment on whole-body glycocalyx barrier properties in db/db mice. Cardiovasc Diabetol. (2013) 12:175. doi: 10.1186/1475-2840-12-175
110. Targosz-Korecka M, Malek-Zietek KE, Kloska D, Rajfur Z, Stepien E, Grochot-Przeczek A, et al. Metformin attenuates adhesion between cancer and endothelial cells in chronic hyperglycemia by recovery of the endothelial glycocalyx barrier. Biochim Biophys Acta Gen Subj. (2020) 1864:129533. doi: 10.1016/j.bbagen.2020.129533
111. Lipowsky HH, Lescanic A. Inhibition of inflammation induced shedding of the endothelial glycocalyx with low molecular weight heparin. Microvasc Res. (2017) 112:72–8. doi: 10.1016/j.mvr.2017.03.007
112. Rademaker MT, Pilbrow AP, Ellmers LJ, Palmer SC, Davidson T, Mbikou P, et al. Acute decompensated heart failure and the kidney: physiological, histological and transcriptomic responses to development and recovery. J Am Heart Assoc. (2021) 10:e021312.
113. Ridker PM, Everett BM, Thuren T, MacFadyen JG, Chang WH, Ballantyne C, et al. Antiinflammatory therapy with canakinumab for atherosclerotic disease. N Engl J Med. (2017) 377:1119–31.
Keywords: cardiorenal syndrome (CRS), biomarker (BM), heart failure, chronic kidney disease, prognosis
Citation: Chung EYM, Trinh K, Li J, Hahn SH, Endre ZH, Rogers NM and Alexander SI (2022) Biomarkers in Cardiorenal Syndrome and Potential Insights Into Novel Therapeutics. Front. Cardiovasc. Med. 9:868658. doi: 10.3389/fcvm.2022.868658
Received: 03 February 2022; Accepted: 29 April 2022;
Published: 20 May 2022.
Edited by:
Yong Liu, Guangdong Provincial People’s Hospital, ChinaReviewed by:
Kevin Shah, The University of Utah, United StatesCopyright © 2022 Chung, Trinh, Li, Hahn, Endre, Rogers and Alexander. This is an open-access article distributed under the terms of the Creative Commons Attribution License (CC BY). The use, distribution or reproduction in other forums is permitted, provided the original author(s) and the copyright owner(s) are credited and that the original publication in this journal is cited, in accordance with accepted academic practice. No use, distribution or reproduction is permitted which does not comply with these terms.
*Correspondence: Edmund Y. M. Chung, ZWRtdW5kLmNodW5nQGhlYWx0aC5uc3cuZ292LmF1
Disclaimer: All claims expressed in this article are solely those of the authors and do not necessarily represent those of their affiliated organizations, or those of the publisher, the editors and the reviewers. Any product that may be evaluated in this article or claim that may be made by its manufacturer is not guaranteed or endorsed by the publisher.
Research integrity at Frontiers
Learn more about the work of our research integrity team to safeguard the quality of each article we publish.