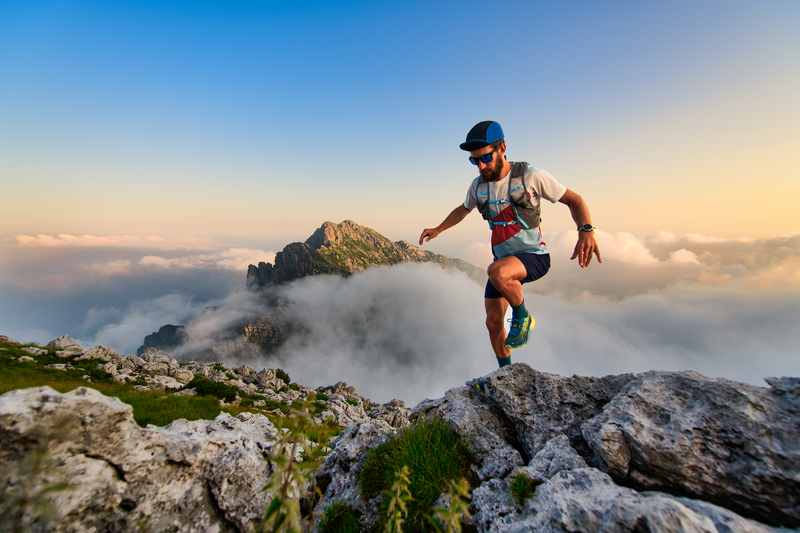
94% of researchers rate our articles as excellent or good
Learn more about the work of our research integrity team to safeguard the quality of each article we publish.
Find out more
REVIEW article
Front. Cardiovasc. Med. , 09 February 2022
Sec. Heart Valve Disease
Volume 9 - 2022 | https://doi.org/10.3389/fcvm.2022.829120
This article is part of the Research Topic Vascular and Valvular Tissue Engineering: Treating and modeling vasculopathies and valvulopathies View all 13 articles
The Ross, or pulmonary autograft, procedure presents a fascinating mechanobiological scenario. Due to the common embryological origin of the aortic and pulmonary root, the conotruncus, several authors have hypothesized that a pulmonary autograft has the innate potential to remodel into an aortic phenotype once exposed to systemic conditions. Most of our understanding of pulmonary autograft mechanobiology stems from the remodeling observed in the arterial wall, rather than the valve, simply because there have been many opportunities to study the walls of dilated autografts explanted at reoperation. While previous histological studies provided important clues on autograft adaptation, a comprehensive understanding of its determinants and underlying mechanisms is needed so that the Ross procedure can become a widely accepted aortic valve substitute in select patients. It is clear that protecting the autograft during the early adaptation phase is crucial to avoid initiating a sequence of pathological remodeling. External support in the freestanding Ross procedure should aim to prevent dilatation while simultaneously promoting remodeling, rather than preventing dilatation at the cost of vascular atrophy. To define the optimal mechanical properties and geometry for external support, the ideal conditions for autograft remodeling and the timeline of mechanical adaptation must be determined. We aimed to rigorously review pulmonary autograft remodeling after the Ross procedure. Starting from the developmental, microstructural and biomechanical differences between the pulmonary artery and aorta, we review autograft mechanobiology in relation to distinct clinical failure mechanisms while aiming to identify unmet clinical needs, gaps in current knowledge and areas for further research. By correlating clinical and experimental observations of autograft remodeling with established principles in cardiovascular mechanobiology, we aim to present an up-to-date overview of all factors involved in extracellular matrix remodeling, their interactions and potential underlying molecular mechanisms.
The aortic valve opens and closes continuously, upwards of 100,000 times per day. Smooth functioning of the valve throughout a lifetime is enabled by its innate remodeling ability (1). Yet, the valve may need to be replaced in cases of unrepairable aortic valve disease, as, for example, in the setting of a bicuspid aortic valve. Especially in young adults with a long life expectancy, an aortic valve substitute should optimally restore aortic root biomechanics and hemodynamic function.
Disappointing outcomes of the first prosthetic valves led to the search for biological alternatives and in 1962, Donald N. Ross commenced implanting aortic valve homografts in patients (2). The lack of availability of homografts in all sizes, the lack of growth potential in children, and their limited durability prompted the quest for a living valve alternative (3). In the Ross procedure, first performed in a patient in 1967, the diseased aortic valve is replaced by the patient's own pulmonary valve and a pulmonary homograft is implanted in the pulmonary position (Figure 1) (5). As the so-called pulmonary autograft is a native tissue substitute, it offers an excellent hemodynamic profile and resistance to endocarditis without the need for anticoagulant therapy (6, 7). This translates into superior exercise capacity and freedom from valve-related complications when compared to mechanical or bioprosthetic valve replacement (8–10). Therefore, the Ross procedure is the only aortic valve replacement that can restore long-term survival and quality-of-life to that of the age-matched population (11–13). Yet, due to a perceived risk of increased operative mortality and the fear of complex reoperations on two valves, there remains skepticism toward the procedure (14, 15).
Figure 1. The 3 main techniques of the Ross procedure. 1. Freestanding root replacement technique with implantation of the entire pulmonary root into the left ventricular outflow tract. 2. Subcoronary technique: implantation of the pulmonary valve only within the native aortic annulus. 3. Autologous/native inclusion technique with implantation of the pulmonary autograft within the native aortic wall to prevent dilatation. Figure reproduced from Sievers (4), journal ceased publication no permission could be requested.
The Ross procedure presents a fascinating mechanobiological scenario. After devascularizing the autograft, it is placed in the systemic circulation and suddenly exposed to a five- to eight-fold greater blood pressure. In the freestanding root technique (Figure 1.1), the pulmonary autograft often dilates immediately (16). Nevertheless, many patients have a well-functioning neo-aortic valve multiple decades post-operatively, indicating a living, remodeling valve (17, 18). As the aorta and pulmonary root share a common embryological origin, the conotruncus, several authors have hypothesized that the pulmonary autograft has the innate ability to remodel into an aortic phenotype (19–22). Unfortunately, there are few data on successfully remodeled tissue. Rather, most ex vivo studies on human autografts evaluated tissue acquired at reoperation and even detailed histological reports of well-functioning autografts are scarce. Therefore, the adaptive mechanisms of the pulmonary autograft are poorly understood. It appears, however, that stress-shielding the pulmonary autograft using meticulous surgical technique, blood pressure control, or external support may further promote adaptation to the systemic circulation (19, 23).
Previous clinical studies and animal models have shown an increase in collagen in both autograft walls and leaflets (24–26). Remodeling of collagen, a key mechanism in cardiovascular biology that can increase tensile stiffness and strength, is also seen in pulmonary hypertension, systemic hypertension and aortic aneurysms (27–29). Therefore, it is likely an essential component of successful remodeling after the Ross procedure. Additional mechanisms such as an increase in cell-extracellular matrix (ECM) connections or collagen cross-linking may contribute to autograft remodeling, but have not yet been identified for the Ross procedure. Proteomic characterization of dilated autografts suggests a unique (mal)adaptive process that differs from that of ascending aortic aneurysms (30). Because all mechanisms aiming to restore tissue stress levels have the greatest chance of succeeding before overt dilatation, the early remodeling phase appears crucial. Furthermore, it is uncertain whether the pulmonary valve has a greater inherent remodeling ability than the wall, or if the wall is just more likely to suffer maladaptation in the freestanding Ross procedure because it sits unrestrained.
Prior reviews on the Ross procedure have focused mainly on patient selection and surgical technique yet speculate about autograft remodeling (19, 23). Nevertheless, long-term success of the Ross procedures relies in the first place on a living, remodeling autograft. Therefore, a comprehensive understanding of autograft adaptation and its determinants is needed so that we can identify patient-specific strategies to promote remodeling and make the pulmonary autograft a permanent aortic valve substitute. Starting with a comparison between the pulmonary artery and aorta, we review autograft mechanobiology in relation to the distinct clinical failure mechanisms. Furthermore, we evaluate the evidence regarding strategies to promote pulmonary autograft adaptation. By correlating clinical and experimental observations of autograft remodeling with established principles in cardiovascular mechanobiology, we aim to present an overview of factors involved in ECM remodeling and their interactions. Simultaneously, we aim to indicate unmet clinical needs, gaps in current knowledge, and areas for further research.
The Ross procedure was first performed with the scalloped pulmonary autograft implanted in subcoronary position, avoiding the need for coronary reimplantation (Figure 1.2) (31). The freestanding root replacement technique was introduced in 1974, once reimplantation of the coronary arteries became technically feasible (3). As this iteration is more reproducible and applicable over a wide range of anatomies, it is the most commonly used today (32). Furthermore, this technique enables neo-aortic root expansion during somatic growth in children.
Upon realizing the risk of dilatation with the root technique, the autologous inclusion technique was introduced whereby the autograft is included within the native aortic wall (Figure 1.3). Although this technique reliably prevents dilatation, it is not applicable in cases of severe size mismatch (16, 18, 33). Prosthetic external support also has the potential to prevent neo-aortic dilatation and is nowadays most commonly performed by placing the autograft within a cylinder of Dacron vascular graft (Figure 2B) (34–36). The long-term effect on reoperation rate and mechanobiological adaptation have yet to be determined (13). The use of external subvalvular annuloplasty and sinotubular junction (STJ) stabilization in patients with an enlarged annulus or STJ was introduced in a systematic fashion by Ismail El-Hamamsy (Figure 2A) (19). Using this “tailored approach” combined with strict blood pressure control, it appears possible to mitigate dilatation while avoiding the potentially deleterious hemodynamic and histological effects of total external support (37).
Figure 2. Most commonly used strategies to externally support the freestanding pulmonary autograft. (A) External subvalvular annuloplasty and sinotubular junction (STJ) stabilization in patients with risk factors for autograft dilatation. (B) Wrapping of the entire autograft within a cylinder of vascular tube graft. Figure adapted with permission from Mazine et al. (19).
Clinical autograft failure, and subsequent reoperation, can be related to wall dilatation, leaflet degeneration, or both. Occurring exclusively after the root replacement technique, non-structural valve degeneration is defined as greater than moderate aortic regurgitation (AR ≥ 3/4) caused by dilatation or autograft wall dilatation beyond 50 mm, with or without associated AR (38). Structural valve degeneration, defined as greater than moderate AR caused by leaflet degeneration or prolapse, is the most common mode of failure for the subcoronary technique, yet it can occur in all variations of the Ross procedure (38). An overview of possible failure patterns, based on anatomical site and underlying mechanism, and correlation with the functional classification of AR as proposed by the group of El Khoury is shown in Figure 3 (39). As the wall and leaflets can degenerate concomitantly, combinations of the described mechanisms are possible, depending on the specific failure phenotype.
Figure 3. Classification of the failure mechanisms of the Ross procedure and correlation with El Khoury's functional classification of aortic regurgitation. AR, aortic regurgitation; SVD, structural valvular degeneration; NSVD, non-structural valvular degeneration. Illustrations adapted with permission from Boodhwani et al. (39).
In expert hands, durability of the subcoronary technique may exceed that of the root replacement technique, without the risk of dilatation. As a leader in his field, Dr. Hans-Hinrich Sievers reports excellent freedom from reoperation of 89.8% at 20 years post-operatively (17). On the other hand, by maximally respecting leaflet anatomy and relations, the root replacement technique has early superiority over the subcoronary and inclusion techniques (18). For any technique, imperfect surgical implantation may result in early AR, progressive in nature due to increased leaflet stress, and potentially lead to early technical failure.
For the root replacement technique, initial elastic dilatation occurs immediately upon release of the aortic cross-clamp due to the pulmonary artery's compliance (Figure 5) (40, 41). Furthermore, up to 60% of the dilatation that is present at 1 year manifested prior to hospital discharge (42). An intuitive hypothesis dictates that in patients with pronounced early dilatation, in itself leading to thinning of the wall, a cycle of pathological remodeling is initiated as the autograft is not permitted to adapt; dilatation begets dilatation (37). Progressive annular or STJ dilatation is known to cause leaflet malcoaptation with a central regurgitant jet (Figure 3) (39, 43, 44). Isolated autograft sinus dilatation on the other hand, is less likely to lead to AR (45).
Although chronic dilatation, related to tissue remodeling, appears to be a slow process in most patients, autograft diameter will exceed 40 mm in up to 50% of patients at 12 years post-operatively (33, 46). At 15 years, up to 24% of patients will require a reoperation for non-structural valve degeneration (13, 32, 38, 47). For the root replacement technique, risk factors for dilatation and subsequent reoperation are pre-operative isolated AR, a large aortic annulus, size mismatch between aortic and pulmonary annulus, pre-existing aortic dilatation, younger age, male sex and post-operative hypertension (13, 32, 38, 47, 48). These variables should be kept in mind when selecting candidates for the Ross procedure (19).
While less straightforward than for genetically determined aortic aneurysms, there appears to be an association between autograft diameter and dissection. Pulmonary autograft dissection has been described in at least 9 cases, occurring between 5 and 18 years after index surgery at an autograft diameter of 54–64 mm (49–57). All patients presented with aortic insufficiency related to a bicuspid aortic valve (BAV) at initial operation. Furthermore, a common feature was pronounced early or sudden dilatation, for example during pregnancy, indicating compromised mechanical homeostasis. In 6 out of 9 cases, the dissection originated in the non-coronary cusp, possibly related to elevated local wall stress. As all dissections were localized without crossing suture-lines, most were incidental findings on imaging, and histopathological assessment confirmed the subacute-to-chronic nature of these dissections (51, 53, 54). In one case, the non-coronary sinus ruptured (56). The critical size threshold for reoperation, when the risk of dissection exceeds the risk of reoperation, is still uncertain. It seems that the risk of autograft dissection or rupture is very low for a diameter below 50 mm, indicating that the decision to reoperate for dilatation should be tailored individually.
A thorough understanding of pulmonary autograft mechanobiology begins with studying the differences between the pulmonary and aortic root. As both vessels have the same embryological origin, the conotruncus, their basic histological composition and anatomy are initially similar (58). Due to diverging hemodynamic conditions post-natally, after the ductus arteriosus closes, the aorta and pulmonary artery and their respective valves develop distinct microstructural and mechanical properties (59).
Both arteries are said to arise from an “annulus,” but the shape of the ventriculo-aortic junction does not constitute a true circle. The aortic root has a crown like band of fibrous tissue at its base with the ventriculo-aortic junction embedded centrally in the heart and inserted on both the atrioventricular valves and the thick left ventricular myocardium (Figure 4). The pulmonary root on the other hand, has no fibrous annulus and originates from the right ventricular infundibulum, a freestanding rim of muscle seated on the right ventricle and septum (61). Both valves consist of three semilunar leaflets, inserted on the annulus in a crown like fashion and meeting at the level of the STJ, forming the commissures (60, 61).
Figure 4. The aortic annulus (red crescent) is embedded within the fibrous skeleton of the heart whereas the pulmonary annulus (blue crescent) consists of a freestanding rim of infundibular muscle lifting the pulmonary leaflets away from the interventricular septum. LVOT, left ventricular outflow tract; RVOT, right ventricular outflow tract. Figure adapted with permission from Ho (60).
In healthy individuals, the aortic STJ diameter is 10–15% smaller than the annulus diameter and ±25% smaller than the maximal sinus diameter (62, 63). Furthermore, the pulmonary valve diameter is about 2 mm greater than that of the aortic valve and both are closely related in height (64). However, in patients undergoing the Ross operation, the relation between pulmonary artery and aorta may be distorted.
Both arteries possess a tunica media rich with elastin, endowing the wall with resilience and elastic recoil, and a tunica adventitia consisting primarily of thick collagen fibers, providing strength (58). Compared to the pulmonary artery, the aorta has a thicker wall with a greater content of structural proteins. Furthermore, its tunica media has a greater number of elastic laminae which are more organized with a denser weave (61, 65–67). As most functional elastic fibers are assembled before adulthood, they are susceptible to mechanical fatigue and proteolytic degradation. Collagen fibers, on the other hand, have a short half-life and are subject to constant turn-over in response to changes in wall stress (27). Therefore, via the action of mainly fibroblasts, the adventitia plays a crucial role in maintaining mechanical homeostasis. The tunica media consists of concentric elastic laminae interspaced with reticular collagen and smooth muscle cells (SMCs), the latter making up ~35% of the wall by dry weight. Finally, a modest amount of glycosaminoglycans contributes to compressive stiffness and likely mechanosensing. Because the contractile filaments of the SMCs are connected to elastic fibers by focal adhesions, forming so-called elastin-contractile units, the SMCs are the main mechanosensing cells of the tunica media (68). While SMCs are typically considered to have either a contractile or synthetic phenotype, with the latter representing a matrix-remodeling function, it seems that these are two ends of a spectrum (69, 70). Furthermore, the SMC population in the aortic and pulmonary media is inhomogenous with cells from various lineages possessing different matrix-producing abilities in response to changes in wall stress or hypoxia (71).
The aortic and pulmonary leaflets, or cusps, are richly innervated and capable of actively responding to changes in mechanical load (19, 72, 73). The cusps are delineated by endothelium on both the arterial and ventricular side. Their core consists of three layers: the collagen-rich fibrosa on the arterial side, the central spongiosa consisting mainly of glycosaminoglycans, and the ventricularis on the ventricular side, rich in elastin sheets (60). Valvular endothelial cells play an important role in the valve's response to changes in flow-induced shear stress by modulating inflammation, calcification, and ECM remodeling (72, 74). They are different from vascular endothelial cells due to their high proliferation rate, unique gene expression profile and orientation perpendicular to blood flow (72). Valve interstitial cells (VICs), present in all three layers of the leaflet, are the primary matrix remodeling cells that maintain structure and function. The mechanical environment of the VICs is sensed by, amongst others, mechanosensitive ion channels (21). When compared to pulmonary VICs, aortic VICs are stiffer and display a greater ability to contract the ECM (75). Additional functional differences include the greater potential for a pro-inflammatory and pro-osteogenic response in aortic VICs, indicating why valve calcification is common in the aortic valve yet rare in the pulmonary position (76).
The pulmonary autograft undergoes a radical change in environment after the Ross procedure due to differences in hemodynamic conditions. The blood pressure in the aorta is around 120/80 mmHg at rest whereas that in the pulmonary artery is around 25/10 mmHg (77). In the healthy pulmonary and aortic root, blood flow is laminar with sinus vortices behind the leaflets, acting as low-pressure zones to facilitate smooth opening and closing (78, 79). The blood flow acceleration and peak velocity in the aorta are approximately double that of the pulmonary root (80). Furthermore, powerful left ventricular contractions subject the aortic root to cyclic elongation and torsional deformation (81). Sufficient aortic distensibility is required to reduce cardiac workload and provide diastolic coronary flow (82). Cyclic expansion of aortic root volume is nearly twice that of the pulmonary root (37.7 vs. 20.9 %), with the greatest distension occurring at the STJ and commissures (82).
Both arterial walls display non-linear mechanical behavior and are most compliant in their physiological pressure ranges because their ECM components are deposited and interlinked at vessel-specific levels of transmural pressure and stretch. At physiological arterial pulsatility, the mechanical load is carried mainly by elastic fibers. With increasing distension, collagen fibers are recruited and the artery stiffens (66, 70). As the pulmonary sinuses are most compliant within the physiological transmural pressure range of 0-30 mmHg, the greatest diameter changes are seen in this range (Figure 5). Beyond 30 mmHg, proportionally less distension is seen with increasing pressures as the wall stiffens (40, 83). Therefore, once exposed to systemic pressures after the Ross procedure, and before any remodeling takes place, the autograft wall will behave significantly stiffer than the aorta, evident by a steeper incline of the pressure-diameter curve (Figure 5) (40, 66, 84, 85).
Figure 5. Pressure-diameter behaviors for the healthy aortic and pulmonary root illustrating non-linear mechanical behavior. In the aortic pressure range of 80–120 mmHg (dotted lines), the pulmonary artery behaves very stiff, evident by the steep incline. Figure recreated using data available in the article by Nagy et al. (40).
The leaflets of the semilunar valves are exposed to flexural and shear stress in systole and tensile and compressive stress in diastole (1). To accommodate this complex cyclical loading, the leaflets are highly anisotropic: they are stiffer in the circumferential than axial direction, related to the circumferential orientation of collagen fibers as opposed to axially oriented elastin bundles (1, 86). Due to the trans-aortic pressure drop of 60–100 mmHg, the autograft leaflets will suddenly experience a far greater tensile and compressive stress on their arterial surface post-operatively. While the microstructure of pulmonary and aortic leaflets is similar, the latter are typically 50–60% thicker and contain more collagen (87, 88). The mechanical properties of both valves also seem to be similar, yet discrepancies in testing protocols between different studies make it challenging to draw definitive conclusions (86, 87, 89, 90). It seems nonetheless that the pulmonary valve is mechanically sound as an aortic valve substitute, given it is implanted symmetrically with perfect coaptation.
The relation between annular and STJ dimensions determine leaflet coaptation (39, 43, 44). A dilated aortic annulus (≥27 mm) may indicate an underlying connective tissue problem. Furthermore, in cases of size mismatch, implantation of the autograft within a larger aortic annulus may impart additional pre-stretch. To ensure that the leaflets remain constrained within the aortic annulus, the autograft should be implanted deep within the annulus so that it can benefit from support of the fibrous skeleton of heart. In patients with a large annulus, an external annuloplasty using a band of prosthetic material may be indicated to further stabilize the annulus (19).
There are regional biomechanical differences within both arterial walls: the ascending aorta and main pulmonary artery are more compliant than their respective sinuses due to a greater elastin content (61, 66). Furthermore, because aortic expansion during the cardiac cycle is most pronounced at the level of the commissures, the STJ is at risk for dilatation after the freestanding root Ross (82). The autograft must be trimmed distally, leaving at most 2 mm of pulmonary wall above the commissures. In patients with pre-operative aortic dilatation, the STJ can be stabilized using a prosthetic interposition graft (91). Externally supporting the STJ with a resorbable band of polydioxanone (PDS) has shown to reduce the incidence of neo-aortic regurgitation in children, further confirming the importance of the STJ.
There appear to be no histological or biomechanical differences between the 3 pulmonary sinuses (66). However, during autograft harvesting, the left-facing, septal autograft sinus is stripped of most of its adventitial tissue where it is adherent to the aorta. In the freestanding Ross, this sinus is therefore usually placed in the left coronary sinus so that it benefits from support of the surrounding heart structures (91).
The underlying pathology forming the clinical indication for the Ross procedure may affect the pulmonary autograft characteristics. In the general population, degenerative aortic valve stenosis in the elderly is the primary indication for valve replacement (92). BAV disease, characterized by abnormal fusion of the aortic leaflets so that only 2 functional leaflets exist, is of special interest to the Ross procedure. While BAV occurs in 0.5–1.2% of the population, it is present in up to 74% of patients undergoing the Ross procedure as it typically manifests at a younger age than degenerative aortic stenosis (32, 38, 93).
Histological features in the aortic wall of BAV include SMC apoptosis and degeneration of ECM (94). While there is evidence for a genetic basis, increased leaflet stress and abnormal flow patterns influence both the development of BAV and its clinical manifestation (95). For the aorta and pulmonary root, both the leaflets and the cells populating the sinus walls are derived from neural crest and second heart field lineages (96). This provides a developmental link between pathologies affecting the leaflets and sinus walls like the aortic dilatation in up to 50% of patients with BAV disease (97). Neural crest cells, implicated in BAV and congenital aortic stenosis, are less commonly seen in the pulmonary than in the aortic root in murine embryological studies (96). This may explain why anatomic pulmonary valve anomalies, precluding use as an autograft, are rare (incidence of 0.1%) and usually associated with other congenital heart defects (98–100).
While de Sa et al. observed degenerative histological features in the main pulmonary artery of BAV patients (101), the association between aortic and pulmonary degenerative features was not confirmed by other groups (94, 102). Furthermore, when assessed by planar biaxial mechanical testing, there is no difference in mechanical properties of the pulmonary artery according to aortic valve phenotype (67). On the other hand, when assessed in vivo by echocardiography, elastic properties of the pulmonary artery are impaired with a correlation between aortic and pulmonary stiffness and diameter in BAV patients (103, 104).
Importantly, the presence of BAV in itself is not a risk factor for autograft failure or dilatation after the freestanding Ross. There are, however, risk factors for dilatation that are associated with BAV and may indicate an underlying connective tissue anomaly, such as aortic insufficiency, a large aortic annulus, or pre-operative aortic dilatation (13, 47, 48). This is in keeping with the observation that the aortic and main pulmonary artery walls of patients with predominantly AR are more compliant than those of patients with predominantly aortic stenosis or mixed stenosis/regurgitation (67). Furthermore, all described cases of autograft dissection occurred in patients with BAV disease. These data highlight the importance of understanding autograft mechanobiology to guide patient selection and predict the risk of dilatation.
Marfan syndrome (MFS) is the most common genetic form of thoracic aortic aneurysm disease, caused by pathogenic variants of the microfibrillar protein fibrillin-1, an ECM component acting as scaffold for elastin, as well as contributing to TGF-β signaling (105). The aorta of patients with MFS is susceptible to dissection or rupture because of its increased stiffness and impaired remodeling ability. MFS and other genetically driven aortopathies are a contra-indication for the Ross procedure because the underlying histologic anomalies, medial degeneration with elastic fiber fragmentation and loss of SMCs may also affect the pulmonary wall (106). Indeed, arterial elastic properties are impaired in MFS, and patients have a larger pulmonary artery diameter with an aneurysm in up to 15.3% (107). If the pulmonary artery of these patients potentially cannot withstand pulmonary pressures, it does not appear fit for use as an aortic autograft.
In 2006, Sir Magdi Yacoub wrote: “An evolutionary point in the Ross operation is the inherent capacity of the autograft to adapt to the new environment by altering its structure and physical properties” (108). The potential longevity of the pulmonary autograft, several decades in many patients, strongly supports this notion (17, 18). If the autograft leaflets would not remodel and function simply as passive structures, it is highly unlikely that they would be able to withstand the systemic circulation for several decades.
For the freestanding Ross, it seems that repetitive supraphysiological distension of the pulmonary autograft may lead to progressive wall damage, while also eliciting a mechanobiological response. Clinical explants of autograft walls acquired within the first 3 months after the Ross procedure show SMC loss and fragmented elastic fibers yet also an increase in myofibroblasts (24). Late explants acquired at reoperation for dilatation show fragmentation of elastic fibers and deposition of mucoid material as well as hyperplastic intimal remodeling, marked adventitial fibrosis and an increase in synthetic SMCs and myofibroblasts (24, 25, 109–112). Yacoub et al. investigated dilated autograft wall samples with a mean implantation period of 14.1 ± 4.1 years and contrary to previous studies, they observed a seemingly well-remodeled and revascularized arterial wall with preserved architecture and a mixture of increased organization of elastic lamellae and degenerative features (20). Table 1 provides an overview of the main histological reports evaluating autograft samples acquired from patients and their key findings.
Table 1. Overview of the main histological reports evaluating autograft samples acquired from patients and their key findings (20, 24, 25, 109–111, 113).
Based on in vivo imaging at 1–5 years post-operatively in adults and children who underwent the Ross procedure as a root technique, the pulmonary autograft sinuses appeared significantly stiffer than the native aorta of healthy controls (85, 114, 115). Stiffening of the pulmonary autograft wall upon dilatation is easily explained by the non-linear mechanical behavior of the intramural constituents, and is likely an inevitable early consequence of the Ross procedure. On the other hand, mechanical testing of dilated, “failed” autograft walls shows that they are not only less stiff than healthy aorta, they are also less stiff than normal pulmonary root at aortic and pulmonary pressure ranges, respectively (116, 117). An artery's mechanical behavior in vivo is determined by its inherent material stiffness but also by its structural stiffness, related to its anatomical configuration and pre-stretch. As it is uncertain if the described mechanical tests of each unique arterial sample were performed at representative levels of in vivo pre-stretch, it is challenging to correlate the mechanical data of these studies with expected in vivo behavior. It is unknown if long-term ECM remodeling can restore autograft stiffness to normal values in well-functioning autografts. Furthermore, the long-term implications of wall stiffness on valve and ventricular function remain uncertain.
Several investigators had the opportunity to investigate autograft leaflets explanted at reoperation, transplantation or at autopsy. While leaflets usually retained their typical trilayered architecture, increased leaflet thickness mainly due to the apposition of an extra layer of tissue/pannus on the ventricular side was consistently observed in multiple reports of both failed and well-functioning valves (20, 24, 25, 109, 113). This layer was characterized by intimal hyperplasia, dense collagen and the presence of myofibroblasts and matrix-remodeling enzymes. Even in “failed” autografts, overall leaflet architecture and microstructure were rather well-preserved while the autograft walls exhibited pronounced degeneration. The excellent freedom from reoperation for the subcoronary and inclusion techniques, with some patients surviving 44 years after surgery, support the notion that the pulmonary leaflets may adapt better than the wall (17, 18, 20).
One might argue that animal models are not clinically relevant as they often use young, healthy animals and short implantation times compared to the development of clinically relevant autograft dilatation. Nevertheless, they provide the opportunity to study the early adaptation mechanisms in seemingly well remodeled autografts. Table 2 shows an overview of all animal models relevant to the Ross procedure and their main findings. In an ovine model of a main pulmonary artery interposition graft in the descending aorta, pulmonary architecture was well-preserved in some areas while vascular atrophy was observed in others. A proportion of explanted tissue samples exhibited aorta-like mechanical behavior during biaxial tensile testing, indicating the pulmonary artery's ability to remodel (84, 118, 119). In a similar animal model using resorbable external support, Nappi et al. suggest that a “neovessel” developed with an increase in elastic wall components (120). This finding is in stark contrast to findings by Schoof et al., who noted preservation of the typical pulmonary arterial microstructure in pigs (26). While new elastin can be produced during adulthood, it is uncertain to what extent these new fibers can contribute to mechanical adaptation, and to what extent the data by Nappi et al. can be extrapolated to humans (27).
Table 2. Overview of animal models relevant to the Ross procedure with main findings (22, 26, 84, 118–124).
In a porcine model of the freestanding Ross procedure, Schoof et al. reported a revascularized wall lacking degenerative features after 10 months. Furthermore, enlarged and rearranged SMCs were seen alongside adventitial fibrosis (26, 121). In the longest experimental evaluation to date, Tudorache et al. observed preservation of typical tri-layered leaflet histology with normal cellular distribution at 22 ± 2.7 months in lambs after the freestanding Ross procedure. Unfortunately, they did not investigate the dilated autograft wall or ECM remodeling of the valve (122). Our group recently developed an ovine model of the Ross procedure performed as a freestanding root replacement (123). Preliminary histological evaluation is consistent with previous clinical and experimental explant studies (Figure 6). Future work will include a comprehensive evaluation of autograft mechanobiology with analysis of hemodynamic parameters, imaging, histology, gene expression response and mechanical testing of explanted tissues.
Figure 6. Representative longitudinal sections through the sinus and leaflets of the pulmonary artery and aorta of a sheep weighing 60 kg. Also shown are the pulmonary autograft and homograft at 6 months post-operatively in a sheep who underwent the Ross procedure (weighing 43 kg at operation). Neo-vascularization in the base of the pulmonary autograft leaflet (white arrowhead) and added collagen on the adventitial side of the sinus wall (black arrowhead). The arterial wall and leaflet of the pulmonary homograft are thin and acellular. Elastica Von Gieson staining. Adapted with permission from Van Hoof et al. (123).
Insight into the underlying mechanisms can be gained by correlating established principles of arterial mechanobiology with changes observed in the pulmonary autograft. In physiological conditions, SMCs and adventitial fibroblasts are stress-shielded mainly by elastic fibers. Upon completion of the freestanding Ross procedure, the autograft wall dilates, resulting in a 5- to 10-fold greater circumferential wall stress (126). As the elastic fibers become stretched and the mechanical load is transmitted to previously underrecruited collagen fibers, the artery behaves stiffer (28). This disruption of baseline stress values prompts a response to restore mechanical homeostasis. Via cell-ECM and cell-cell connections, cells sense the stress acting on the ECM. Changes in the mechanical environment are translated into intracellular signals via specific mechanotransduction pathways, inducing the production of ECM proteins, adhesion molecules and matrix metalloproteinases (MMPs) (70). Figure 7 provides an overview of known and potentially involved mechanisms of ECM remodeling.
Figure 7. Overview of established and potentially involved mechanisms of pulmonary autograft wall remodeling in the Ross procedure. The + indicates an adaptive response, — indicates maladaptive remodeling. IEL, internal elastic lamina; SMC, vascular smooth muscle cell; ECM, extracellular matrix; MMP, matrix metalloproteinase; TGFβ, transforming growth factor β; (M)FBR, fibroblast/myofibroblast.
Fibroblasts located mainly in the adventitia are among the first to be activated by overdistension (127). These fibroblasts proliferate, and via the release of growth factors, induce the differentiation of SMCs from the contractile to the synthetic phenotype. Fibroblasts differentiate into myofibroblasts by the influence of transforming growth factor β (TGF-β) and mechanical stresses (69, 128). Collagen is deposited at the newly imposed mechanical load while existing fibers are removed by MMPs or modulated, for example by changing collagen cross-linking patterns (27). Increased adventitial collagen is observed in all autograft samples acquired at reoperation and in all animal studies, suggesting a common mode of remodeling in both failed and well-adapted autografts. This form of ECM remodeling is a crucial part of arterial homeostasis in arterial aging, pulmonary arterial hypertension and aneurysm development (27, 129, 130). Elevated myofibroblast, MMP1, MMP13 and TGF-β levels are seen in clinical autograft explants at over 10 years post-operatively (24, 25, 30, 109). This persistent ECM remodeling likely indicates the inability to restore the homeostatic state.
As regeneration of elastic fibers is limited, certainly in comparison with collagen, they are susceptible to mechanical fatigue by repetitive overdistension. Currently, the threshold pressure or diameter which causes acute elastic damage is unknown. In an ex vivo inflation set-up, the main pulmonary artery became susceptible to damage upon acute exposure to a transmural pressure beyond 60 mmHg, with pressures above 100 mmHg likely to induce collagen damage as well (131). Because this study by Wang et al. employed previously frozen porcine pulmonary arteries, it is uncertain if these data can be extrapolated to the Ross procedure in humans. Previous studies of dilated, explanted pulmonary autografts show fragmented elastin fibers and an increase in synthetic-type smooth muscle cells. As the stress in SMCs is typically concentrated at focal adhesions, connecting the cytoskeleton to surrounding elastic fibers, it seems likely that damage to the elastic-contractile units occurs (68). Besides representing a change in mechanical properties, elastic fiber fragments have a signaling function by inducing SMC proliferation and a phenotype switch, and will undergo proteolytic degradation by MMPs and cathepsins (28, 132).
Immediately after the Ross procedure, autograft leaflets are exposed to elevated shear, compressive and tensile stresses. Furthermore, aortic root dilatation may contribute to cusp stretching. It is likely that collagen production plays an important role in valve remodeling in response to increased stress, as it does in the wall, with an important role for VICs in the cusps (133). Previous histological studies suggest successful leaflet adaptation to the systemic circulation, with increased cusp mass mainly due to the apposition of an extra layer of fibrous tissue on the ventricular cusp side (20, 24, 25, 109). While this suggests improved mechanical properties, there are no data to support this. The absence of the degenerative features which are seen in dilated autograft walls could indicate a greater ability of the leaflet to adapt to the systemic circulation. By preventing excessive deformation and providing reciprocal support, good leaflet coaptation in the early phase after the Ross procedure likely protects the cusps from acute ECM damage, thereby permitting adaptation. Endothelial to mesenchymal transition may also play a role in maintaining leaflet integrity (1).
Endothelial hyperplasia is consistently observed in wall samples explanted late after the Ross procedure (20, 25). This feature, commonly seen in aortic aneurysms, suggests remodeling in response to elevated shear or intramural stress (134). Furthermore, endothelial cells of explanted autograft valves express Ephrin B2. While this may indicate a stable systemic VEC phenotype, it cannot be excluded that these cells migrated from the native aorta or endocardium (24).
Among all aortic valve substitutes, blood flow patterns are closest to normal after the Ross operation (7, 41). In BAV disease, abnormal flow patterns with elevated wall shear stress associate with aneurysm progression and focal elastin degeneration (135). The changes in flow and shear stress that occur in the pulmonary autograft, upon transposition from pulmonary to aortic position, have not been quantified. Therefore, the role of abnormal flow patterns, pre-existent or related to neo-aortic regurgitation which develops later, on autograft remodeling remains unknown.
As their wall is thicker than 0.5 mm, the external layers of the pulmonary sinuses are supplied by vasa vasorum (136). Disruption thereof during autograft harvesting may lead to damage or impair remodeling. Both in a porcine model of the Ross procedure and in clinical explant studies, a well-revascularized arterial wall with normal to slightly increased presence of vasa vasorum in the tunica media and adventitia was seen (20, 25, 26). The magnitude of this contribution and the role of neovascularization in mechanobiological adaptation after the Ross procedure are unknown. The autograft leaflets are likely less affected by ischemia as they are supplied by diffusion from both the aortic and ventricular side.
Goffin et al. observed a disappearance of dendritic cells in an explanted autograft of a 14-year old patient who died of unrelated causes (113). While these accessory immune cells play a role in regulating early ECM remodeling, the significance of this finding is uncertain (127). In vitro, repetitive overstretching of valvular endothelial cells leads to an upregulation of inflammatory pathways (74). However, there is no evidence for relevant inflammatory activity or calcification in samples explanted several years after the Ross procedure.
A recent study by Chiarini et al. suggests a unique maladaptive process in the autograft which differs from that of aortic aneurysms (30). They compared the proteomic signature of the tunica media of dilated autografts, acquired 8–16 years post-operatively, against that for normal PA, aorta and aortic aneurysm samples. An upregulation of paxillin, a key component of focal adhesions, was observed. As the integrin-containing focal adhesions mechanically link the ECM and actin cytoskeleton, their finding may represent abnormal cytoskeleton remodeling and impaired mechanotransduction (70). Vimentin, a component of the SMC cytoskeleton, was also upregulated, confirming the activation of pathways involving synthetic SMCs. A downregulation of MAGP1 was seen, suggesting impaired elastic fiber buildup. They also found evidence of a disturbed JAG1-Notch1 signaling, potentially impeding tissue remodeling by limiting cell-cell interactions. Unfortunately, it is unknown if the observed proteomic changes represent a cause or consequence of maladaptation and repetitive overdistension.
In the general population, pulmonary artery aneurysm is a rare entity, reported only 8 times in 109,571 autopsies and mainly associated with pulmonary arterial hypertension in the setting of congenital heart disease (130, 137). There are yet several molecular pathways implied in thoracic aortic aneurysms which are also involved in the development of pulmonary arterial hypertension and pulmonary artery aneurysm. Analogous with BAV disease, a combination of hemodynamics and underlying molecular pathways likely impair mechanoregulation in both the aorta and pulmonary artery (130). With regards to collagen integrity, deficiencies in the following proteins are relevant: biglycan (an ECM component regulating collagen formation) and collagen 3 α1 chain (implied in Ehlers-Danlos Syndrome type IV). For elastic fibers: fibrillin-1 (elastic fiber core glycoprotein with signaling function, implicated in MFS) and fibulin 4 (regulation of elastic fiber assembly). Reduced expression of lysyl oxidase, a collagen and elastin cross-linking enzyme, also leads to mural degeneration. For the actin cytoskeleton, proteins like smooth muscle α-actin and filamin A are relevant. With regards to mechanoregulation, TGF-β receptors (implicated in Loeys-Dietz syndrome) and Notch1 (impaired signaling seen in dilated autografts) are implicated (130). These interesting associations provide insight into possible underlying mechanisms of pulmonary autograft maladaptation. Finally, the role of mechanosensitive ion channels should be explored (21).
Undoubtedly, blood pressure is one of the main determinants of remodeling. As early remodeling is crucial to avoid initiating a vicious cycle of pathological remodeling, strict blood pressure control (systolic pressure <110 mmHg) is advocated by many experts starting immediately post-operatively and continued for 6–12 months (19). While there is currently no direct evidence for an effect on dilatation, reoperation rate or histological outcome, aggressive blood pressure control appears justified as there are many indirect arguments that antihypertensive medication can improve autograft remodeling. In ascending aortic aneurysms, hypertension is associated with aneurysm growth rate as well as dissection (138). While the decrease in wall stress is non-linearly related to blood pressure lowering, antihypertensive treatment can achieve a marked reduction in wall stress, even in patients with mildly elevated blood pressure. Therefore, even in patients with normal or slightly elevated blood pressure, antihypertensive treatment can achieve a marked reduction in wall stress. In the Ross procedure, this could make the difference needed to allow autograft adaptation.
The underlying idea is to gradually expose the autograft to aortic pressures. There is anecdotal evidence that preoperative pulmonary arterial hypertension pre-conditions the autograft by promoting ECM organization (65). In animal models of pulmonary hypertension, thickness of the tunica media is increased (29). This concept is further supported by numerical simulations of saphenous vein remodeling when used as arterial bypass graft, whereby gradual loading improves remodeling (139).
In both MFS and after the Ross procedure, a repetitive cycle of overdistension occurs in an arterial wall at risk for pathological remodeling (140). Aortic stiffness is inherently increased in MFS. Similarly, the pulmonary autograft wall behaves stiffer than the aorta at systemic pressures, at least before remodeling (84). By lowering blood pressure and the force and velocity of ventricular contraction, beta-blockers reduce aortic wall stress, thereby lowering the risk of aortic dissection in patients with MFS and potentially enabling the wall to heal. Angiotensin-converting enzyme inhibitors and angiotensin II receptor blockers reduce blood pressure and aortic stiffness (105). In murine models of MFS, prenatal initiation of angiotensin II receptor blockers has the potential to prevent pathological remodeling and aortic dilatation. As this effect is related to hemodynamic and vasomotor changes as well as the interference with TGF-β signaling, it may be of interest to the Ross procedure (141).
The technical considerations of currently used strategies to prevent autograft dilatation were recently reviewed by Chauvette et al. (23). While it is uncertain at this point what the best strategy is, external support should be biocompatible in terms of geometry, compliance and tissue reaction. The primary goal of external support is to stabilize root dimensions; this will inevitably reduce distensibility, and therefore circumferential wall stress and strain. Repetitive strain is crucial to arterial homeostasis, just as mechanical loading is essential for the maintenance of bone density and skeletal muscle mass (142). In a landmark study, Courtman et al. banded the infrarenal abdominal aorta in rabbits, thereby reducing local strain. At 6 weeks, they observed apoptotic loss of 30% of SMCs and a 45% reduction of medial area. No endothelial cell loss was seen, nor was neo-intimalization impaired in animals undergoing banding and endothelial balloon denudation (143). In an experiment involving iliac artery wrapping in baboons, a tighter wrap induced more ECM loss and SMC atrophy (144). These banding studies indicate that excessive stress-shielding, so that cells experience low tensile stress, is undesirable. This is analogous to applying a tight cast to a fractured leg without allowing movement of the limb, resulting in muscle and bone atrophy (142). Furthermore, damage induced by compression between the wrap and pulsatile blood flow cannot be excluded. Numerical simulations of prosthetic external support of the descending aorta indicate the potential of external support to ameliorate the arterial homeostatic response to elevated pressure, and also point to stiffness of the support material as a crucial determinant of remodeling (145). It seems that the autograft needs to feel just the right amount of stress in order to heal after being devascularized during the operation, and subsequently remodel.
Any type of prosthetic external support induces a tissue reaction, influenced by the material, presence of a coating and porosity. Early cellular inflammation soon shifts to a foreign body giant cell reaction, characterized by macrophages and giant cells attempting to encapsulate the implanted material (146). For external support, porosity is one of the most important parameters. A porous material will permit the ingrowth of a fibrotic neo-adventitia and blood vessels, thereby enabling the transport of oxygen and nutrients through the material (147, 148). Furthermore, the graft will become anchored to the arterial wall, forming a composite. A non-biodegradable low-porosity material, on the other hand, will prevent tissue ingrowth and elicit the formation of a thick sheath of fibrosis, potentially further increasing arterial stiffness (148, 149). As the inflammatory reaction induced by the graft is situated in the peri-adventitia, its contribution to vascular remodeling is unknown.
In the autologous inclusion technique (Figure 1.3), the autograft is sutured into the aortic annulus and then included within the native aortic wall, which is reduced or enlarged to achieve the desired dimension (18). From an anatomical and biomechanical point of view, this technique optimally preserves functional aortic root integrity and compliance. The native aorta naturally augments the autograft's structural properties to withstand systemic pressures, though without a detailed analysis of changes in wall stress from homeostatic values. While this technique is technically challenging, an exceptionally low incidence of significant root dilatation and reoperation can be achieved (18). To our knowledge, there are no histological reports of autografts explanted after the inclusion technique.
The autograft can be included within a segment of Dacron vascular tube or Valsalva graft before implantation into the aortic annulus (Figure 8A), stabilizing neo-aortic dimensions up to 5 years post-operatively (34–36). While this may seem a straightforward and reproducible technique, it risks distorting the autograft and preventing it from settling into its natural post-operative shape. As a microporous and sealed graft allows limited tissue ingrowth, seroma formation, erosion and graft migration have been a great concern for conventional aortic wrapping of aneurysms using the same material (151, 152). Because the rigid, woven material impairs pulsatility, there are also concerns about elevated leaflet stress and abnormal flow patterns, as evidenced by 4D MRI studies after valve-sparing root replacement (79). Finally, vascular atrophy seems inevitable with this stiff material due to excessive stress-shielding (148). To date, these complications have not been reported for the supported Ross procedure.
Figure 8. (A) Pulmonary autograft wrapped with a cylinder of microporous Dacron graft. (B) Personalized external aortic root support implant fashioned from porous, soft mesh. Figure adapted with permission from Carrel et al. (34) and Treasure et al. (150).
In Personalized External Aortic Root Support (PEARS), a soft, custom-made mesh is used to stabilize a moderately dilated aortic root with at most mild AR, primarily in patients with MFS (153). The porous support (Figure 8B) becomes well-incorporated into a neo-adventitia and stabilizes root dimensions (148, 150, 154–156). Subsequently, the risk of dissection is mitigated by avoiding repetitive overdistension. Pepper et al. had the opportunity to histologically examine the aorta of a patient with MFS who underwent PEARS and later died of unrelated causes. The supported region of his aorta showed a normal tunica media whereas the unsupported aortic arch showed features of Marfan syndrome, suggesting that the external support allowed the aorta to heal (156).
This flexible, porous material that conforms to aortic anatomy holds great promise for the Ross procedure and has currently been applied in nearly 50 patients (157). In conventional aortic PEARS, the support is modeled using the patient's pre-operative aortic CT scan. The challenge in the Ross procedure has been to predict external support morphology based on pre-operative imaging, as the pulmonary autograft changes shape and dilates immediately upon exposure to aortic pressures. In an ovine model of a pulmonary artery interposition graft in the descending aorta, the mesh material used in PEARS successfully stabilized dimensions at 2 months after allowing some initial dilatation. All supported samples showed thinning of the media with SMC atrophy (119). While this atrophy may be an inevitable consequence of stress-shielding, it indicates that improvements to the material's mechanical properties are possible. Furthermore, a major difference with conventional aortic PEARS is that the pulmonary autograft is devascularized during the operation, and external support may influence healing and the development of vasa vasorum.
If protection during the early phase, when the risk of dilatation is greatest, will promote appropriate pulmonary autograft remodeling, external support might not be required permanently. One could envision a composite external support, partially consisting of resorbable material, gradually resorbing and allowing some initial dilatation while the degree of support decreases. A similar strategy is used to promote in vivo neovessel development using biodegradable tissue engineering scaffolds (158).
The clinical use of resorbable support was already described in 1993 by Moritz et al., who used a VICRYL® mesh composed of polyglactin 910. Unfortunately, data on the effect on dilatation is not available (159). Nappi et al. designed a composite support consisting of a resorbable polydioxanone mesh interwoven with fibers of expanded polytetrafluoroethylene, the latter allowing gradual expansion but serving as permanent support. They used this material as support for a pulmonary artery interposition graft in the descending aorta of 10 growing sheep. At 6 months, dilatation was effectively prevented and the material was well-incorporated without marked inflammatory changes (22). Unfortunately, their report lacks reliable measurements of wall thickness, protein fractions, elastin integrity and mechanical testing to confirm remodeling. While composite resorbable support holds great promise to prevent neo-aortic root dilatation, like in the Ross procedure, its clinical implementation is currently not justified.
The available evidence indicates that the pulmonary autograft can become a permanent solution for a wide range of patients. Several fundamental questions remain to be answered before this can become reality (Table 3). Besides the universally observed collagen remodeling by myofibroblasts and synthetic type SMCs, it is unknown which mechanoregulation pathways enable some autografts to withstand systemic conditions for several decades. Candidate mechanisms include changes in collagen cross-linking or an increase in cell-ECM connections.
Table 3. Remaining fundamental questions and unmet clinical needs regarding pulmonary autograft remodeling after the Ross procedure.
A greater understanding of the relation between patient characteristics and autograft remodeling may identify biomarkers or cardiac imaging features related to autograft maladaptation. This will enable us to define in which patients the autograft's innate remodeling ability will likely suffice to withstand systemic conditions, and in whom additional measures are needed to guarantee adaptation. A pre-operative in vivo assessment of the pulmonary autograft using dynamic imaging studies may indicate whether a patient is a good candidate for the Ross procedure. Serial post-operative imaging studies evaluating changes in stiffness may identify patients at risk for excessive dilatation or reoperation (85, 114, 115). As in aortic aneurysms, localized changes in shear stress may signal underlying elastin degradation (135). In patients with congestive heart failure, excessive myocardial collagen cross-linking indicates undue cardiac stiffness and associates with adverse clinical outcome. This collagen cross-linking can be quantified non-invasively on blood samples or based on the urinary proteome (160). Furthermore, diastolic left ventricular function can be predicted based on the urinary proteome in individuals without heart failure (161). Similarly, serial biochemical studies in patients before and after the Ross procedure might identify biomarkers to guide patient selection or confirm the presence of maladaptation in the post-operative setting.
In vitro culture of a pulmonary artery in a bioreactor may provide valuable insights into the timeline of early remodeling and the role of shear stress, pre-stretch at implantation and acute hypertension. Large animal models also hold great promise to evaluate whether current strategies to prevent dilatation—external support and antihypertensive treatment—promote remodeling, either by modifying the mechanical environment or by interfering with molecular pathways (123). To assess whether the autograft remains viable—capable of healing and regulating its mechanical properties—active mechanical testing of freshly explanted leaflets and wall would be required (162). A rat model with heterotopic implantation of the pulmonary root of a syngeneic donor animal into the abdominal aorta would allow a serial evaluation of adaptation in the first post-operative months at a lower cost.
By providing data on microstructure, mechanical properties, geometry, hemodynamics and the underlying pathways, experimental models can enable numerical simulation of autograft remodeling (163, 164). Subsequently, the ideal conditions for remodeling, or conversely, the risk of dissection, could be identified in so-called in silico trials (165). Until now, available computational studies have mainly confirmed important in vivo observations, such as the importance of STJ dimensions on wall and leaflet stress (126, 163, 166, 167). It has proven very challenging to mathematically simulate the complex torsional deformation of the aortic root which results in spiraling blood flow and flow vortices behind leaflets (41).
Notwithstanding its clinical use since 2004, several questions about prosthetic external support of the autograft sinuses should be resolved (36). First, concerns about the deleterious hemodynamic effects on the left ventricle and autograft leaflets should be addressed by echocardiographic and 4D flow MRI studies. Second, once placed in the aortic position, optimal autograft geometry must be clearly defined. Quantitative comparison of pre-operative and post-operative imaging in patients undergoing the Ross procedure might yield a predictive algorithm for ideal autograft geometry and subsequent external support configuration.
The mechanical properties of external support should be determined by how much stress-shielding is needed in each phase of remodeling. To this end, the timeline of changes in mechanical properties should be evaluated in clinical imaging studies and in an animal model. Finally, polymer materials are required which truly augment the autograft. Even the best currently used polymer materials, including resorbable ones, are relatively stiff and inelastic (119). Therefore, the mechanical behavior will be dominated by the external support, potentially leading to excessive stress-shielding and deleterious hemodynamic effects. Polyglycerol sebacate (PGS) is a promising new resorbable elastomer which has been used as tissue-engineered vascular grafts to serve as scaffold for cellularization (168). More futuristic options include a bioengineered matrix of collagen and elastin, containing growth factors, or even seeded with stem cells, immediately optimizing mechanical properties while promoting autograft incorporation into the aortic root (169).
Long-term clinical success of the Ross procedure relies on a well-functioning, living valve integrated into an aortic root having a normal hemodynamic profile. From this point of view, the subcoronary and autologous inclusion technique may be superior to the freestanding root technique, yet the latter is applicable over a wider spectrum of cardiac anatomy and is more surgically reproducible. All evidence indicates that the autograft valve is suited to withstand the systemic circulation and remodel, given that it is implanted symmetrically with stable annular and STJ dimensions over time. Therefore, one of the key surgical principles of the Ross procedure is to ensure that the proximal autograft is constrained within the native aortic annulus. The autograft sinuses, on the other hand, sit unrestrained after a freestanding Ross procedure and are at risk of dilating and subsequently causing the valve to fail. The currently available evidence indicates that the pulmonary autograft wall is not capable of truly achieving mechanical homeostasis and remodeling into an aortic phenotype. Perhaps the aortic and pulmonary root have diverged too far apart after arising from a common embryological origin.
Protecting the autograft during the early adaptation phase is crucial to avoid initiating a sequence of pathological remodeling. Therefore, strict blood pressure control during the first 6–12 post-operative months is justified to reduce wall and leaflet stress. Adequate patient selection is critical and the surgical technique should be tailored individually, aiming to minimize the amount of wall tissue exposed to aortic pressures. While external autograft support may stabilize root dimensions, its efficacy should be measured by a reduction in reinterventions, without negatively affecting valve or left ventricular function.
Remodeling of the ECM with mainly the production of additional collagen is a common feature in both autograft walls and leaflets. A distinct feature of autograft leaflets is the apposition of an extra layer of tissue on the ventricular side, resulting in increased leaflet thickness. Future studies should pinpoint the remodeling processes in well-remodeled and externally supported autografts. Several molecular pathways are proposed. To this end, animal models or bioreactor studies should include a comprehensive mechanobiological assessment at different time-points consisting of microstructural evaluation, transcriptional and proteomic characterization, mechanical testing of tissue samples and dynamic imaging studies with complete hemodynamic profile.
Numerical simulations of tissue growth and remodeling may aid in distilling the ideal conditions for autograft adaptation. Subsequently, a patient-specific strategy for autograft protection and external support could be determined, and the indications for the Ross procedure might be expanded. Widespread clinical implementation of the PEARS concept for the Ross procedure is greatly anticipated because of the many advantages over microporous vascular grafts. Future innovations to external support may include the use of resorbable materials or bio-engineered scaffolds to augment the autograft's mechanical properties and guide remodeling.
This manuscript is the result of 3 years of research into the mechanobiology of the Ross procedure in the context of the PhD program of LV, performed under supervision of FR, PV, NF, and EJ. LV wrote the first draft and edited the figures and tables. Subsequent revisions were written based on multidisciplinary discussions involving LV, PV, EJ, JH, SJ, NF, and FR, including written feedback. All authors approved the final version.
KU Leuven Research Project (C2 Project: C24/16/026 24ZKD1128-00-W01) + LV is the holder of a predoctoral grant Strategic basic research (SB 1S70220N) from the Research Foundation Flanders (FWO).
The authors declare that the research was conducted in the absence of any commercial or financial relationships that could be construed as a potential conflict of interest.
All claims expressed in this article are solely those of the authors and do not necessarily represent those of their affiliated organizations, or those of the publisher, the editors and the reviewers. Any product that may be evaluated in this article, or claim that may be made by its manufacturer, is not guaranteed or endorsed by the publisher.
The authors would like to thank Piet Claus for the knowledge he shared on cardiovascular imaging and dynamics which contributed to the realization of this manuscript.
1. Chester AH, El-Hamamsy I, Butcher JT, Latif N, Bertazzo S, Yacoub MH. The living aortic valve: From molecules to function. Glob Cardiol Sci Pract. (2014) 2014:11. doi: 10.5339/gcsp.2014.11
3. Spray TL. Technique of pulmonary autograft aortic valve replacement in children (the Ross procedure). Semin Thorac Cardiovasc Surg Pediatr Card Surg Annu. (1998) 1:165–78. doi: 10.1016/S1092-9126(98)70022-5
4. Sievers HH. Ross procedure H.H. HSR Proc Intensive Care Cardiovasc Anesth. (2012) 4:119–23. Available online at: https://citeseerx.ist.psu.edu/viewdoc/download?doi=10.1.1.680.9781&rep=rep1&type=pdf
5. Ross DN. Replacement of aortic and mitral valves with a pulmonary autograft. Lancet. (1967) 290:956–8. doi: 10.1016/S0140-6736(67)90794-5
6. Torii R, El-Hamamsy I, Donya M, Babu-Narayan SV, Ibrahim M, Kilner PJ, et al. Integrated morphologic and functional assessment of the aortic root after different tissue valve root replacement procedures. J Thorac Cardiovasc Surg. (2012) 143:1422–8.e2. doi: 10.1016/j.jtcvs.2011.12.034
7. Bissell MM, Loudon M, Hess AT, Stoll V, Orchard E, Neubauer S, et al. Differential flow improvements after valve replacements in bicuspid aortic valve disease: A cardiovascular magnetic resonance assessment. J Cardiovasc Magn Reson. (2018) 20:1–10. doi: 10.1186/s12968-018-0431-5
8. Nötzold A, Hüppe M, Schmidtke C, Blömer P, Uhlig T, Sievers HH. Quality of life in aortic valve replacement: pulmonary autografts versus mechanical prostheses. J Am Coll Cardiol. (2001) 37:1963–6. doi: 10.1016/S0735-1097(01)01267-0
9. Buratto E, Shi WY, Wynne R, Poh CL, Larobina M, Keefe MO, et al. Improved survival after the ross procedure compared with mechanical aortic valve replacement. J Am Coll Cardiol. (2018) 71:1337–44. doi: 10.1016/j.jacc.2018.01.048
10. Sharabiani MTA, Dorobantu DM, Mahani AS, Turner M, Peter Tometzki AJ, Angelini GD, et al. Aortic valve replacement and the ross operation in children and young adults. J Am Coll Cardiol. (2016) 67:2858–70. doi: 10.1016/j.jacc.2016.04.021
11. McClure GR, Belley-Cote EP, Um K, Gupta S, Bouhout I, Lortie H, et al. The ross procedure versus other aortic valve replacement: a systematic review and meta-analysis. Eur J Cardio-thoracic Surg. (2018) 59:462–70. doi: 10.1093/ejcts/ezy247
12. Etnel JRG, Grashuis P, Huygens SA, Pekbay B, Papageorgiou G, Helbing WA, et al. The ross procedure: a systematic review, meta-analysis, and microsimulation. Circ Cardiovasc Qual Outcomes. (2018) 11:e004748. doi: 10.1161/CIRCOUTCOMES.118.004748
13. Sievers HH, Stierle U, Charitos EI, Takkenberg JJM, Hörer J, Lange R, et al. A multicentre evaluation of the autograft procedure for young patients undergoing aortic valve replacement: update on the German Ross Registry. Eur J Cardio-thoracic Surg. (2016) 49:212–8. doi: 10.1093/ejcts/ezv001
14. Reece TB, Welke KF, O'Brien S, Grau-Sepulveda MV, Grover FL, Gammie JS. Rethinking the ross procedure in adults. Ann Thorac Surg. (2014) 97:175–81. doi: 10.1016/j.athoracsur.2013.07.036
15. Stulak JM, Burkhart HM, Sundt TM, Connolly HM, Suri RM, Schaff H V, et al. Spectrum and outcome of reoperations after the ross procedure. Circulation. (2010) 122:1153–8. doi: 10.1161/CIRCULATIONAHA.109.897538
16. Takkenberg JJM, van Herwerden LA, Galema TW, Bekkers JA, Kleyburg-Linkers VA, Eijkemans MJ, et al. Serial echocardiographic assessment of neo-aortic regurgitation and root dimensions after the modified Ross procedure. J Hear Valve Dis. (2006) 15:100–6.
17. Sievers HH, Stierle U, Petersen M, Klotz S, Richardt D, Diwoky M, et al. Valve performance classification in 630 subcoronary Ross patients over 22 years. J Thorac Cardiovasc Surg. (2018) 156:79–86. doi: 10.1016/j.jtcvs.2018.03.015
18. Skillington PD, Mokhles MM, Takkenberg JJM, Larobina M, O'Keefe M, Wynne R, et al. The Ross procedure using autologous support of the pulmonary autograft: Techniques and late results. J Thorac Cardiovasc Surg. (2015) 149:S46–52. doi: 10.1016/j.jtcvs.2014.08.068
19. Mazine A, El-Hamamsy I, Verma S, Peterson MD, Bonow RO, Yacoub MH, et al. Ross procedure in adults for cardiologists and cardiac surgeons. J Am Coll Cardiol. (2018) 72:2761–77. doi: 10.1016/j.jacc.2018.08.2200
20. Yacoub MH, Tsang V, Sarathchandra P, Jensen H, Hughes S, Latif N. Long-term adaptive versus maladaptive remodelling of the pulmonary autograft after the Ross operation. Eur J Cardiothorac Surg. (2020) 57:977–85. doi: 10.1093/ejcts/ezaa019
21. Al-Shammari H, Latif N, Sarathchandra P, McCormack A, Rog-Zielinska EA, Raja S, et al. Expression and function of mechanosensitive ion channels in human valve interstitial cells. PLoS ONE. (2020) 15:e0240532. doi: 10.1371/journal.pone.0240532
22. Nappi F, Spadaccio C, Fraldi M, Montagnani S, Fouret P, Chachques JC, et al. A composite semiresorbable armoured scaffold stabilizes pulmonary autograft after the Ross operation: Mr Ross's dream fulfilled. J Thorac Cardiovasc Surg. (2016) 151:155–64. doi: 10.1016/j.jtcvs.2015.09.084
23. Chauvette V, Chamberland MÈ, El-Hamamsy I. A review of pulmonary autograft external support in the Ross procedure. Expert Rev Med Devices. (2019) 16:981–8. doi: 10.1080/17434440.2019.1685380
24. Rabkin-Aikawa E, Aikawa M, Farber M, Kratz JR, Garcia-Cardena G, Kouchoukos NT, et al. Clinical pulmonary autograft valves: Pathologic evidence of adaptive remodeling in the aortic site. J Thorac Cardiovasc Surg. (2004) 128:552–61. doi: 10.1016/j.jtcvs.2004.04.016
25. Schoof PH, Takkenberg JJM, van Suylen RJ, Zondervan PE, Hazekamp MG, Dion RAE, et al. Degeneration of the pulmonary autograft: An explant study. J Thorac Cardiovasc Surg. (2006) 132:1426–32. doi: 10.1016/j.jtcvs.2006.07.035
26. Schoof PH. Gittenberger-de Groot AC, De Heer E, Bruijn JA, Hazekamp MG, Huysmans HA. Remodeling of the porcine pulmonary autograft wall in the aortic position. J Thorac Cardiovasc Surg. (2000) 120:55–65. doi: 10.1067/mtc.2000.106970
27. Humphrey JD, Dufresne ER, Schwartz MA. Mechanotransduction and extracellular matrix homeostasis. Nat Rev Mol Cell Biol. (2014) 15:802–12. doi: 10.1038/nrm3896
28. Thompson RW, Geraghty PJ, Lee JK. Abdominal aortic aneurysms: Basic mechanisms and clinical implications. Curr Probl Surg. (2002) 39:110–230. doi: 10.1067/msg.2002.121421
29. Zuckerman BD, Orton EC, Stenmark KR, Trapp JA, Murphy JR, Coffeen PR, et al. Alteration of the pulsatile load in the high-altitude calf model of pulmonary hypertension. J Appl Physiol. (1991) 70:859–68. doi: 10.1152/jappl.1991.70.2.859
30. Chiarini A, Dal Prà I, Faggian G, Armato U, Luciani GB. Maladaptive remodeling of pulmonary artery root autografts after Ross procedure: a proteomic study. J Thorac Cardiovasc Surg. (2020) 159:621–32.e3. doi: 10.1016/j.jtcvs.2019.07.083
32. David TE, David C, Woo A, Manlhiot C. The Ross procedure: outcomes at 20 years. J Thorac Cardiovasc Surg. (2014) 147:85–94. doi: 10.1016/j.jtcvs.2013.08.007
33. de Kerchove L, Rubay J, Pasquet A, Poncelet A, Ovaert C, Pirotte M, et al. Ross operation in the adult: long-term outcomes after root replacement and inclusion techniques. Ann Thorac Surg. (2009) 87:95–102. doi: 10.1016/j.athoracsur.2008.09.031
34. Carrel T, Schwerzmann M, Eckstein F, Aymard T, Kadner A. Preliminary results following reinforcement of the pulmonary autograft to prevent dilatation after the Ross procedure. J Thorac Cardiovasc Surg. (2008) 136:472–5. doi: 10.1016/j.jtcvs.2008.02.004
35. Juthier F, Banfi C, Vincentelli A, Ennezat PV, Le Tourneau T, Pinçon C, et al. Modified Ross operation with reinforcement of the pulmonary autograft: Six-year results. J Thorac Cardiovasc Surg. (2010) 139:1420–3. doi: 10.1016/j.jtcvs.2010.01.032
36. Slater M, Shen I, Welke K, Komanapalli C, Ungerleider R. Modification to the Ross procedure to prevent autograft dilatation. Pediatr Card Surg Annu. (2005) 8:181–4. doi: 10.1053/j.pcsu.2005.01.022
37. Bouhout I, Ghoneim A, Tousch M, Stevens LM, Semplonius T, Tarabzoni M, et al. Impact of a tailored surgical approach on autograft root dimensions in patients undergoing the Ross procedure for aortic regurgitation. Eur J Cardio-thoracic Surg. (2019) 56:959–67. doi: 10.1093/ejcts/ezz105
38. Martin E, Mohammadi S, Jacques F, Kalavrouziotis D, Voisine P, Doyle D, et al. Clinical outcomes following the Ross procedure in adults: A 25-year longitudinal study. J Am Coll Cardiol. (2017) 70:1890–9. doi: 10.1016/j.jacc.2017.08.030
39. Boodhwani M, de Kerchove L, Glineur D, Poncelet A, Rubay J, Astarci P, et al. Repair-oriented classification of aortic insufficiency: Impact on surgical techniques and clinical outcomes. J Thorac Cardiovasc Surg. (2009) 137:286–94. doi: 10.1016/j.jtcvs.2008.08.054
40. Nagy ZL, Fisher J, Walker PG, Watterson KG. The in vitro hydrodynamic characteristics of the porcine pulmonary valve and root with regard to the Ross procedure. J Thorac Cardiovasc Surg. (2000) 120:284–9. doi: 10.1067/mtc.2000.107473
41. Torii R, Xu XY, El-Hamamsy I, Mohiaddin R, Yacoub MH. Computational biomechanics of the aortic root. Aswan Hear Cent Sci Pract Ser. (2011) 2011:16. doi: 10.5339/ahcsps.2011.16
42. Hokken RB, Bogers AJJC, Taams MA, Schiks-Berghourt MB, Van Herwerden LA, Roelandt JRTC, et al. Does the pulmonary autograft in the aortic position in adults increase in diameter? An echocardiographic study. J Thorac Cardiovasc Surg. (1997) 113:667–74. doi: 10.1016/S0022-5223(97)70223-X
43. Bellhouse BJ, Bellhouse F, Abbott JA, Talbot L. Mechanism of valvular incompetence in aortic sinus dilatation. Cardiovasc Res. (1973) 7:490–4. doi: 10.1093/cvr/7.4.490
44. Kim DH, Handschumacher MD, Levine RA, Joo Sun B, Jang JY, Yang DH, et al. Aortic valve adaptation to aortic root dilatation insights into the mechanism of functional aortic regurgitation from 3-dimensional cardiac computed tomography. Circ Cardiovasc Imaging. (2014) 7:828–35. doi: 10.1161/CIRCIMAGING.113.001976
45. Matalanis G, Perera NK. Aortic valve sparing root surgery for Marfan syndrome. Ann Cardiothorac Surg. (2017) 6:682–91. doi: 10.21037/acs.2017.11.05
46. Frigiola A, Ranucci M, Carlucci C, Giamberti A, Abella R, Donato M Di, et al. The Ross procedure in adults: long-term follow-up and echocardiographic changes leading to pulmonary autograft reoperation. Ann Thorac Surg. (2008) 86:482–90. doi: 10.1016/j.athoracsur.2008.04.001
47. Charitos EI, Takkenberg JJM, Hanke T, Gorski A, Botha C, Franke U, et al. Reoperations on the pulmonary autograft and pulmonary homograft after the Ross procedure: an update on the German Dutch Ross Registry. J Thorac Cardiovasc Surg. (2012) 144:813–23. doi: 10.1016/j.jtcvs.2012.07.005
48. David TE, Ouzounian M, David CM, Lafreniere-Roula M, Manlhiot C. Late results of the Ross procedure. J Thorac Cardiovasc Surg. (2019) 157:201–8. doi: 10.1016/j.jtcvs.2018.06.037
49. Kincaid EH, Maloney JD, Lavender SW, Kon ND. Dissection in a pulmonary autograft. Ann Thorac Surg. (2004) 77:707–8. doi: 10.1016/S0003-4975(03)01402-4
50. Luciani GB, Favaro A, Viscardi F, Bertolini P, Mazzucco A. Valve-sparing root replacement for pulmonary autograft dissection late after the Ross operation. J Thorac Cardiovasc Surg. (2004) 128:753–6. doi: 10.1016/j.jtcvs.2004.03.031
51. Klieverik LMA, Takkenberg JJM, Elbers BCJ, Oei FBS, van Herwerden LA, Bogers AJJC. Dissection of a dilated autograft root. J Thorac Cardiovasc Surg. (2007) 133:817–8. doi: 10.1016/j.jtcvs.2006.11.006
52. Venkataraman R, Vaidyanathan KR, Sankar MN, Cherian KM. Late dissection of pulmonary autograft treated by valve-sparing aortic root replacement. J Card Surg. (2009) 24:443–5. doi: 10.1111/j.1540-8191.2008.00764.x
53. Rabkin DG, Reid BB, Doty JR. Acute on chronic pulmonary autograft dissection. Interact Cardiovasc Thorac Surg. (2015) 20:563–4. doi: 10.1093/icvts/ivu427
54. Myers MR, Magruder JT, Crawford TC, Grimm JC, Halushka MK, Baumgartner WA, et al. Surgical repair of aortic dissection 16 years post-Ross procedure. J Surg Case Reports. (2016) 4:1–3. doi: 10.1093/jscr/rjw059
55. Richey S, Fiore AC, Huddleston CB. Type A aortic dissection after the ross procedure. Ann Thorac Surg. (2018) 106:e105–6. doi: 10.1016/j.athoracsur.2018.02.042
56. Peeters G, Arrigoni SC, Schoof P, Accord RE, Mariani MA. Acute type A dissection 18 years after a Ross operation: the old prejudice. Ann Thorac Surg. (2019) 107:e255–7. doi: 10.1016/j.athoracsur.2018.08.075
57. Siudalska H, Kuśmierczyk M, Rózański J, Petryka-Mazurkiewicz J, Kumor M, Michałowska AM, et al. Aortic dissection after the Ross procedure. Kardiol Pol. (2021) 79:702–3. doi: 10.33963/KP.15957
58. Goor DA, Dische R, Lillehei CW. The conotruncus. I Its normal inversion and conus absorption. Circulation. (1972) 46:634–6. doi: 10.1161/01.CIR.46.3.635
59. Leung DYM, Glagov S, Mathews MB. Elastin and collagen accumulation in rabbit ascending aorta and pulmonary trunk during postnatal growth. Correlation of cellular synthetic response with medial tension. Circ Res. (1977) 41:316–23. doi: 10.1161/01.RES.41.3.316
60. Ho SY. Structure and anatomy of the aortic root. Eur J Echocardiogr. (2009) 10:3–10. doi: 10.1093/ejechocard/jen243
61. Hokken RB, Bartelings MM, Bogers AJJC, Gittonberger-De Groot AC. Morphology of the pulmonary and aortic roots with regard to the pulmonary autograft procedure. J Thorac Cardiovasc Surg. (1997) 113:453–61. doi: 10.1016/S0022-5223(97)70357-X
62. Tamás E, Nylander E. Echocardiographic description of the anatomic relations within the normal aortic root. J Heart Valve Dis. (2007) 16:240–6.
63. Kunzelman KS, Grande KJ, David TE, Cochran RP, Verrier ED. Aortic root and valve relationships: impact on surgical repair. J Thorac Cardiovasc Surg. (1994) 107:162–70. doi: 10.1016/S0022-5223(94)70465-1
64. Capps SB, Elkins RC, Fronk DM. Body surface area as a predictor of aortic and pulmonary valve diameter. J Thorac Cardiovasc Surg. (2000) 119:975–82. doi: 10.1016/S0022-5223(00)70092-4
65. Carr-White GS, Afoke A, Birks EJ, Hughes S, O'Halloran A, Glennen S, et al. Aortic root characteristics of human pulmonary autografts. Circulation. (2000) 102:III15–I21. doi: 10.1161/01.CIR.102.suppl_3.III-15
66. Azadani AN, Chitsaz S, Matthews PB, Jaussaud N, Leung J, Wisneski A, et al. Biomechanical comparison of human pulmonary and aortic roots. Eur J Cardio-thoracic Surg. (2012) 41:1111–6. doi: 10.1093/ejcts/ezr163
67. Dionne PO, Wener E, Emmott A, Cartier R, Mongrain R, Leask R, et al. The Ross procedure: biomechanical properties of the pulmonary artery according to aortic valve phenotype. Interact Cardiovasc Thorac Surg. (2016) 23:371–6. doi: 10.1093/icvts/ivw148
68. Karimi A, Milewicz DM. Structure of the elastin-contractile units in the thoracic aorta and how genes that cause thoracic aortic aneurysms and dissections disrupt this structure. Can J Cardiol. (2016) 32:26–34. doi: 10.1016/j.cjca.2015.11.004
69. Tomasek JJ, Gabbiani G, Hinz B, Chaponnier C, Brown RA. Myofibroblasts and mechano: regulation of connective tissue remodelling. Nat Rev Mol Cell Biol. (2002) 3:349–63. doi: 10.1038/nrm809
70. Humphrey JD, Schwartz MA, Tellides G, Milewicz DM. Role of mechanotransduction in vascular biology. Circ Res. (2015) 116:1448–61. doi: 10.1161/CIRCRESAHA.114.304936
71. Frid MG, Dempsey EC, Durmowicz AG, Stenmark KR. Smooth muscle cell heterogeneity in pulmonary and systemic vessels: importance in vascular disease. Arterioscler Thromb Vasc Biol. (1997) 17:1203–9. doi: 10.1161/01.ATV.17.7.1203
72. El-Hamamsy I, Chester AH, Yacoub MH. Cellular regulation of the structure and function of aortic valves. J Adv Res. (2010) 1:5–12. doi: 10.1016/j.jare.2010.02.007
73. Marron K, Yacoub MH, Polak JM, Sheppard MN, Fagan D, Whitehead BF, et al. Innervation of human atrioventricular and arterial valves. Circulation. (1996) 94:368–75. doi: 10.1161/01.CIR.94.3.368
74. Metzler SA, Pregonero CA, Butcher JT, Burgess SC, Warnock JN. Cyclic strain regulates pro-inflammatory protein expression in porcine aortic valve endothelial cells. J Heart Valve Dis. (2008) 17:571–8.
75. Rutkovskiy A, Malashicheva A, Sullivan G, Bogdanova M, Kostareva A, Stensløkken KO, et al. Valve interstitial cells: the key to understanding the pathophysiology of heart valve calcification. J Am Heart Assoc. (2017) 6:1–23. doi: 10.1161/JAHA.117.006339
76. Yang X, Fullerton DA, Su X, Ao L, Cleveland JC, Meng X. Pro-osteogenic phenotype of human aortic valve interstitial cells is associated with higher levels of toll-like receptors 2 and 4 and enhanced expression of bone morphogenetic protein 2. J Am Coll Cardiol. (2009) 53:491–500. doi: 10.1016/j.jacc.2008.09.052
77. Boron WF, Boulpaep EL. Medical Physiology: A Cellular and Molecular Approach. Philadelphia, PA: Saunders/Elsevier (2009).
78. Barker AJ, Roldán-Alzate A, Entezari P, Shah SJ, Chesler NC, Wieben O, et al. Four-dimensional flow assessment of pulmonary artery flow and wall shear stress in adult pulmonary arterial hypertension: results from two institutions. Magn Reson Med. (2015) 73:1904–13. doi: 10.1002/mrm.25326
79. Oechtering TH, Frydrychowicz A, Sievers HH. Malrotated sinus vortices in straight graft valve-sparing aortic root treatment: a matter of concern? J Thorac Cardiovasc Surg. (2017) 154:794–7. doi: 10.1016/j.jtcvs.2017.02.024
80. Gardin JM, Burn CS, Childs WJ, Henry WL. Evaluation of blood flow velocity in the ascending aorta and main pulmonary artery of normal subjects by Doppler echocardiography. Am Heart J. (1984) 107:310–9. doi: 10.1016/0002-8703(84)90380-6
81. Beller CJ, Labrosse MR, Thubrikar MJ, Robicsek F. Role of aortic root motion in the pathogenesis of aortic dissection. Circulation. (2004) 109:763–9. doi: 10.1161/01.CIR.0000112569.27151.F7
82. Lansac E, Lim HS, Shomura Y, Lim KH, Goetz W, Rice NT, et al. Aortic and pulmonary root: are their dynamics similar? Eur J Cardio-thoracic Surg. (2002) 21:268–75. doi: 10.1016/S1010-7940(01)01132-0
83. Bia D, Armentano RL, Grignola JC, Craiem D, Zócalo YA, Ginés FF, et al. The vascular smooth muscle of great arteries: local control site of arterial buffering function? Rev Esp Cardiol. (2003) 56:1202–9. doi: 10.1016/S0300-8932(03)77039-0
84. Vastmans J, Fehervary H, Verbrugghe P, Verbelen T, Vanderveken E, Vander Sloten J, et al. Biomechanical evaluation of a personalized external aortic root support applied in the Ross procedure. J Mech Behav Biomed Mater. (2018) 78:164–74. doi: 10.1016/j.jmbbm.2017.11.018
85. Lenoir M, Emmott A, Bouhout I, Poirier N, Tousch M, El-Hamamsy I, et al. Autograft remodeling after the Ross procedure by cardiovascular magnetic resonance imaging: aortic stenosis versus insufficiency. J Thorac Cardiovasc Surg. (2022) 163:578–87.e1. doi: 10.1016/j.jtcvs.2020.03.185
86. Hasan A, Ragaert K, Swieszkowski W, Selimović Š, Paul A, Camci-Unal G, et al. Biomechanical properties of native and tissue engineered heart valve constructs. J Biomech. (2014) 47:1949–63. doi: 10.1016/j.jbiomech.2013.09.023
87. Stradins P, Lacis R, Ozolanta I, Purina B, Ose V, Feldmane L, et al. Comparison of biomechanical and structural properties between human aortic and pulmonary valve. Eur J Cardio-thoracic Surg. (2004) 26:634–9. doi: 10.1016/j.ejcts.2004.05.043
88. Leeson-Dietrich J, Boughner D, Vesely I. Porcine pulmonary and aortic valves: a comparison of their tensile viscoelastic properties at physiological strain rates. J Heart Valve Dis. (1995) 4:88–94.
89. Soares ALF, van Geemen D, van den Bogaerdt AJ, Oomens CWJ, Bouten CVC, Baaijens FPT. Mechanics of the pulmonary valve in the aortic position. J Mech Behav Biomed Mater. (2014) 29:557–67. doi: 10.1016/j.jmbbm.2013.07.009
90. Christie GW, Barratt-Boyes BG. Mechanical properties of porcine pulmonary valve leaflets: how do they differ from aortic leaflets? Ann Thorac Surg. (1995) 60(Suppl. 2):S195–9. doi: 10.1016/0003-4975(95)00279-T
91. Mazine A, Ghoneim A, El-Hamamsy I. The Ross procedure: how I teach it. Ann Thorac Surg. (2018) 105:1294–8. doi: 10.1016/j.athoracsur.2018.01.048
92. Iung B, Baron G, Butchart EG, Delahaye F, Gohlke-Bärwolf C, Levang OW, et al. A prospective survey of patients with valvular heart disease in Europe: The Euro Heart Survey on valvular heart disease. Eur Heart J. (2003) 24:1231–43. doi: 10.1016/S0195-668X(03)00201-X
93. Robicsek F, Thubrikar MJ, Cook JW, Fowler B. The congenitally bicuspid aortic valve: How does it function? Why does it fail? Ann Thorac Surg. (2004) 77:177–85. doi: 10.1016/S0003-4975(03)01249-9
94. Schmid FX, Bielenberg K, Holmer S, Lehle K, Djavidani B, Prasser C, et al. Structural and biomolecular changes in aorta and pulmonary trunk of patients with aortic aneurysm and valve disease: Implications for the Ross procedure. Eur J Cardio-thoracic Surg. (2004) 25:748–53. doi: 10.1016/j.ejcts.2004.02.028
95. Michelena HI, Prakash SK, Della CA, Bissell MM, Anavekar N, Mathieu P, et al. Bicuspid aortic valve identifying knowledge gaps and rising to the challenge from the international bicuspid aortic valve consortium (BAVCON). Circulation. (2014) 129:2691–704. doi: 10.1161/CIRCULATIONAHA.113.007851
96. Richardson R, Eley L, Donald-Wilson C, Davis J, Curley N, Alqahtani A, et al. Development and maturation of the fibrous components of the arterial roots in the mouse heart. J Anat. (2018) 232:554–67. doi: 10.1111/joa.12713
97. Verma S, Siu SC. Aortic dilatation in patients with bicuspid aortic valve. N Engl J Med. (2014) 370:1920–9. doi: 10.1056/NEJMra1207059
98. Vistarini N, Gebhard C, Desjardins G, El-Hamamsy I. Successful repair of a bicuspid pulmonary autograft valve causing early insufficiency after a Ross procedure. Ann Thorac Surg. (2016) 101:e99–101. doi: 10.1016/j.athoracsur.2015.06.119
99. Kajingu Enciso S, Elens M, Rubay J. Congenital bicuspid pulmonary valve. An uncommon impede for Ross procedure. Acta Chir Belg. (2017) 117:115–7. doi: 10.1080/00015458.2016.1220121
100. Jashari R, Van Hoeck B, Goffin Y, Vanderkelen A. The incidence of congenital bicuspid or bileaflet and quadricuspid or quadrileaflet arterial valves in 3,861 donor hearts in the European Homograft Bank. J Heart Valve Dis. (2009) 18:337–44.
101. De Sa M, Moshkovitz Y, Butany J, David TE, Robicsek F, Gardner TJ, et al. Histologic abnormalities of the ascending aorta and pulmonary trunk in patients with bicuspid aortic valve disease: clinical relevance to the Ross procedure. J Thorac Cardiovasc Surg. (1999) 118:588–96. doi: 10.1016/S0022-5223(99)70002-4
102. Luciani GB, Barozzi L, Tomezzoli A, Casali G, Mazzucco A. Bicuspid aortic valve disease and pulmonary autograft root dilatation after the Ross procedure: a clinicopathologic study. J Thorac Cardiovasc Surg. (2001) 122:74–9. doi: 10.1067/mtc.2001.114638
103. Celik M, Yuksel UC, Yalcinkaya E, Gokoglan Y, Yildirim E, Bugan B, et al. Elasticity properties of pulmonary artery in patients with bicuspid aortic valve. Echocardiography. (2014) 31:759–64. doi: 10.1111/echo.12455
104. Kutty S, Kaul S, Danford CJ, Danford DA. Main pulmonary artery dilation in association with congenital bicuspid aortic valve in the absence of pulmonary valve abnormality. Heart. (2010) 96:1756–61. doi: 10.1136/hrt.2010.199109
105. Cañadas V, Vilacosta I, Bruna I, Fuster V. Marfan syndrome. Part 1: pathophysiology and diagnosis. Nat Rev Cardiol. (2010) 7:256–65. doi: 10.1038/nrcardio.2010.30
106. Chiu P, Irons M, van de Rijn M, Liang DH, Miller DC. Giant pulmonary artery aneurysm in a patient with Marfan syndrome and pulmonary hypertension. Circulation. (2016) 133:1218–21. doi: 10.1161/CIRCULATIONAHA.115.020537
107. Sheikhzadeh S, De Backer J, Gorgan NR, Rybczynski M, Hillebrand M, Schüler H, et al. The main pulmonary artery in adults: a controlled multicenter study with assessment of echocardiographic reference values, and the frequency of dilatation and aneurysm in Marfan syndrome Orphanet. J Rare Dis. (2014) 9:203. doi: 10.1186/s13023-014-0203-8
108. Yacoub MH. The Ross operation - an evolutionary tale. Asian Cardiovasc Thorac Ann. (2006) 14:1–2. doi: 10.1177/021849230601400101
109. Mookhoek A, de Heer E, Bogers AJJC, Takkenberg JJM, Schoof PH. Pulmonary autograft valve explants show typical degeneration. J Thorac Cardiovasc Surg. (2010) 139:1416–9. doi: 10.1016/j.jtcvs.2010.01.020
110. Ishizaka T, Devaney EJ, Ramsburgh SR, Suzuki T, Ohye RG, Bove EL. Valve sparing aortic root replacement for dilatation of the pulmonary autograft and aortic regurgitation after the Ross procedure. Ann Thorac Surg. (2003) 75:1518–22. doi: 10.1016/S0003-4975(02)04904-4
111. Takkenberg JJM, Zondervan PE, Van Herwerden LA. Progressive pulmonary autograft root dilatation and failure after ross procedure. Ann Thorac Surg. (1999) 67:551–4. doi: 10.1016/S0003-4975(98)01274-0
112. Sundt TM, Moon MR, Xu H, Stelzer P. Reoperation for dilatation of the pulmonary autograft after the ross procedure. J Thorac Cardiovasc Surg. (2002) 122:1249–52. doi: 10.1067/mtc.2001.116320
113. Goffin YA, Narine KR, Alexander JP, Van Goethem J, Daenen WJ. Histopathologic comparison of a pulmonary autograft and pulmonary homograft in a patient 17 months after a Ross procedure: an autopsy study. J Heart Valve Dis. (1998) 7:327–30.
114. Ando Y, Ochiai Y, Tokunaga S, Hisahara M, Baba H, Miyagi C, et al. Size and stiffness of the pulmonary autograft after the Ross procedure in children. Pediatr Cardiol. (2019) 40:776–83. doi: 10.1007/s00246-019-02064-9
115. Xuan Y, Alonso E, Emmott A, Wang Z, Kumar S, Mongeon F-P, et al. Wall stresses of early remodeled pulmonary autografts. J Thorac Cardiovasc Surg. (2021). doi: 10.1016/j.jtcvs.2021.08.058. [Epub ahead of print].
116. Mookhoek A, Krishnan K, Chitsaz S, Kuang H, Ge L, Schoof PH, et al. Biomechanics of failed pulmonary autografts compared with normal pulmonary roots. Ann Thorac Surg. (2016) 102:1996–2002. doi: 10.1016/j.athoracsur.2016.05.010
117. Mookhoek A, Krishnan K, Chitsaz S, Kuang H, Ge L, Schoof PH, et al. Biomechanics of failed pulmonary autografts compared to native aortic roots. Ann Thorac Surg. (2017) 103:1482–8. doi: 10.1016/j.athoracsur.2016.08.061
118. Vanderveken E, Vastmans J, Verbelen T, Verbrugghe P, Famaey N, Verbeken E, et al. Reinforcing the pulmonary artery autograft in the aortic position with a textile mesh: a histological evaluation. Interact Cardiovasc Thorac Surg. (2018) 27:566–73. doi: 10.1093/icvts/ivy134
119. Vanderveken E, Vastmans J, Claus P, Verbeken E, Fehervary H, Van Hoof L, et al. Mechano-biological adaptation of the pulmonary artery exposed to systemic conditions. Sci Rep. (2020) 10:1–12. doi: 10.1038/s41598-020-59554-7
120. Nappi F, Spadaccio C, Fouret P, Hammoudi N, Chachques JC, Chello M, et al. An experimental model of the Ross operation: development of resorbable reinforcements for pulmonary autografts. J Thorac Cardiovasc Surg. (2015) 149:1134–42. doi: 10.1016/j.jtcvs.2014.12.056
121. Schoof PH, Hazekamp MG, Van Wermeskerken GK, De Heer E, Bruijn JA, Gittenberger-De Groot AC, et al. Disproportionate enlargement of the pulmonary autograft in the aortic position in the growing pig. J Thorac Cardiovasc Surg. (1998) 115:1264–72. doi: 10.1016/S0022-5223(98)70208-9
122. Tudorache I, Theodoridis K, Baraki H, Sarikouch S, Bara C, Meyer T, et al. Decellularized aortic allografts versus pulmonary autografts for aortic valve replacement in the growing sheep model: haemodynamic and morphological results at 20 months after implantation. Eur J Cardio-thoracic Surg. (2016) 49:1228–38. doi: 10.1093/ejcts/ezv362
123. Van Hoof L, Claus P, Jones EAV, Meuris B, Famaey N, Verbrugghe P, et al. Back to the root: a large animal model of the Ross procedure. Ann Cardiothorac Surg. (2021) 10:444–53. doi: 10.21037/acs-2020-rp-21
124. Lower RR, Stofer RC, Shumway NE. Autotransplantation of the pulmonic valve into the aorta. J Thorac Cardiovasc Surg. (1960) 39:680–7. doi: 10.1016/S0022-5223(20)31814-6
125. Pillsbury RC, Shumway NE. Replacement of the aortic valve with the autologous pulmonic valve. Surg Forum. (1966) 17:176–7.
126. Wisneski AD, Matthews PB, Azadani AN, Chitsaz S, Lui N, Takabe K, et al. Finite element modeling of the human pulmonary autograft: a quantitative analysis of wall stress after the Ross procedure. J Am Coll Surg. (2012) 215:S46. doi: 10.1016/j.jamcollsurg.2012.06.137
127. Stenmark KR, Nozik-Grayck E, Gerasimovskaya E, Anwar A, Li M, Riddle S, et al. The adventitia: Essential role in pulmonary vascular remodeling. Compr Physiol. (2011) 1:141–61. doi: 10.1002/cphy.c090017
128. Rensen SSM, Doevendans PAFM, Van Eys GJJM. Regulation and characteristics of vascular smooth muscle cell phenotypic diversity. Netherlands Hear J. (2007) 15:100–8. doi: 10.1007/BF03085963
129. Van Varik BJ, Rennenberg RJMW, Chris P, Kroon AA, De Leeuw PW, Schurgers LJ. Mechanisms of arterial remodeling: lessons from genetic diseases. Front Genet. (2012) 3:1–10. doi: 10.3389/fgene.2012.00290
130. Nuche J, Palomino-Doza J, Ynsaurriaga FA, Delgado JF, Ibáñez B, Oliver E, et al. Potential molecular pathways related to pulmonary artery aneurysm development: Lessons to learn from the aorta. Int J Mol Sci. (2020) 21:509. doi: 10.3390/ijms21072509
131. Wang Y, Gharahi H, Grobbel MR, Rao A, Roccabianca S, Baek S. Potential damage in pulmonary arterial hypertension: an experimental study of pressure-induced damage of pulmonary artery. J Biomed Mater Res. (2021) 109:579–89. doi: 10.1002/jbm.a.37042
132. Patel A, Fine B, Sandig M, Mequanint K. Elastin biosynthesis: The missing link in tissue-engineered blood vessels. Cardiovasc Res. (2006) 71:40–9. doi: 10.1016/j.cardiores.2006.02.021
133. Ku CH, Johnson PH, Batten P, Sarathchandra P, Chambers RC, Taylor PM, et al. Collagen synthesis by mesenchymal stem cells and aortic valve interstitial cells in response to mechanical stretch. Cardiovasc Res. (2006) 71:548–56. doi: 10.1016/j.cardiores.2006.03.022
134. Tang PCY, Coady MA, Lovoulos C, Dardik A, Aslan M, Elefteriades JA, et al. Hyperplastic cellular remodeling of the media in ascending thoracic aortic aneurysms. Circulation. (2005) 112:1098–105. doi: 10.1161/CIRCULATIONAHA.104.511717
135. Guzzardi DG, Barker AJ, Van Ooij P, Malaisrie SC, Puthumana JJ, Belke DD, et al. Valve-related hemodynamics mediate human bicuspid aortopathy: insights from wall shear stress mapping. J Am Coll Cardiol. (2015) 66:892–900. doi: 10.1016/j.jacc.2015.06.1310
136. Wolinsky H, Glagov S. Nature of species differences in the medial distribution of aortic vasa vasorum in mammals. Circ Res. (1967) 20:409–21. doi: 10.1161/01.RES.20.4.409
137. Bartter T, Irwin RS, Nash G. Aneurysms of the pulmonary arteries. Chest. (1988) 94:1065–75. doi: 10.1378/chest.94.5.1065
138. Tong J, Rabkin S. The relationship between hypertension and thoracic aortic aneurysm of degenerative or atherosclerotic origin: a systematic review. Austin Hypertens. (2016) 1:1004.
139. Ramachandra AB, Humphrey JD, Marsden AL. Gradual loading ameliorates maladaptation in computational simulations of vein graft growth and remodelling. J R Soc Interface. (2017) 14:e0995. doi: 10.1098/rsif.2016.0995
140. Pyeritz RE. The Marfan syndrome. Annu Rev Med. (2000) 51:481–510. doi: 10.1146/annurev.med.51.1.481
141. Habashi JP, Judge DP, Holm TM, Cohn RD, Loeys BL, Cooper TK, et al. Losartan, an AT1 antagonist, prevents aortic aneurysm in a mouse model of Marfan syndrome. Science. (2006) 312:117–21. doi: 10.1126/science.1124287
142. Ceroni D, Martin X, Delhumeau C, Rizzoli R, Kaelin A, Farpour-Lambert N. Effects of cast-mediated immobilization on bone mineral mass at various sites in adolescents with lower-extremity fracture. J Bone Jt Surg A. (2012) 94:208–16. doi: 10.2106/JBJS.K.00420
143. Courtman DW, Cho A, Langille L, Wilson GJ. Eliminating arterial pulsatile strain by external banding induces medial but not neointimal atrophy and apoptosis in the rabbit. Am J Pathol. (1998) 153:1723–9. doi: 10.1016/S0002-9440(10)65687-8
144. Min SK, Kenagy RD, Jeanette JP, Clowes AW. Effects of external wrapping and increased blood flow on atrophy of the baboon iliac artery. J Vasc Surg. (2008) 47:1039–47. doi: 10.1016/j.jvs.2007.12.043
145. Ramachandra AB, Latorre M, Szafron JM, Marsden AL, Humphrey JD. Vascular adaptation in the presence of external support - a modeling study. J Mech Behav Biomed Mater. (2020) 110:103943. doi: 10.1016/j.jmbbm.2020.103943
146. Schürmann K, Vorwerk D, Bücker A, Neuerburg J, Klosterhalfen B, Müller G, et al. Perigraft inflammation due to Dacron-covered stent-grafts in sheep iliac arteries: correlation of MR imaging and histopathologic findings. Cardiovasc Radiol. (1997) 204:757–63. doi: 10.1148/radiology.204.3.9280255
147. Orenstein SB, Saberski ER, Kreutzer DL, Novitsky YW. Comparative analysis of histopathologic effects of synthetic meshes based on material, weight, and pore size in mice. J Surg Res. (2012) 176:423–9. doi: 10.1016/j.jss.2011.09.031
148. Van Hoof L, Verbrugghe P, Verbeken E, Treasure T, Famaey N, Meuris B, et al. Support of the aortic wall: a histological study in sheep comparing a macroporous mesh with low-porosity vascular graft of the same polyethylene terephthalate material. Interact Cardiovasc Thorac Surg. (2017) 25:89–95. doi: 10.1093/icvts/ivx009
149. White RA. The effect of porosity and biomaterial on the healing and long-term mechanical properties of vascular prostheses. Trans Am Soc Artif Intern Organs. (1988) 34:95–100. doi: 10.1097/00002480-198804000-00004
150. Treasure T, Petrou M, Rosendahl U, Austin C, Rega F, Pirk J, et al. Personalized external aortic root support: a review of the current status. Eur J Cardio-Thoracic Surg. (2016) 50:400–4. doi: 10.1093/ejcts/ezw078
151. Treasure T. A “ compare and contrast” exercise: wrapping versus personalised external aortic root support (PEARS). J Cardiothorac Surg. (2016) 11:10–1. doi: 10.1186/s13019-016-0499-7
152. Plonek T, Dumanski A, Nowicki R, Kustrzycki W. Computed tomography angiography of aorta subjected to external wrapping. J Cardiothorac Surg. (2016) 11:1–5. doi: 10.1186/s13019-016-0487-y
153. Van Hoof L, Rega F, Golesworthy T, Verbrugghe P, Austin C, Takkenberg JJM, et al. Personalised external aortic root support for elective treatment of aortic root dilation in 200 patients. Heart. (2021) 107:1790–5. doi: 10.1136/heartjnl-2021-319300
154. Verbrugghe P, Verbeken E, Pepper J, Treasure T, Meyns B, Meuris B, et al. External aortic root support: a histological and mechanical study in sheep. Interact Cardiovasc Thorac Surg. (2013) 17:334–9. doi: 10.1093/icvts/ivt165
155. Izgi C, Newsome S, Alpendurada F, Nyktari E, Boutsikou M, Pepper J, et al. External aortic root support to prevent aortic dilatation in patients with Marfan syndrome. J Am Coll Cardiol. (2018) 72:1095–105. doi: 10.1016/j.jacc.2018.06.053
156. Pepper J, Goddard M, Mohiaddin R, Treasure T. Histology of a Marfan aorta 45 years after personalized external aortic root support. Eur J Cardio-Thoracic Surg. (2015) 48:502–5. doi: 10.1093/ejcts/ezu415
157. Golesworthy T. ExoVasc® Personalised External Aortic Root Support Project Status – 6 August 2021. (2021).
158. Drews JD, Pepper VK, Best CA, Szafron JM, Cheatham JP, Yates AR, et al. Spontaneous reversal of stenosis in tissue-engineered vascular grafts. Sci Transl Med. (2020) 12:1–14. doi: 10.1126/scitranslmed.aax6919
159. Mortiz A, Domanig E, Marx M, Moidl R, Simon P, Laufer G, et al. Pulmonary autograft valve replacement in the dilated and asymmetric aortic root. Eur J Cardio-Thoracic Surg. (1993) 7:405–8. doi: 10.1016/1010-7940(93)90003-T
160. López B, Ravassa S, González A, Zubillaga E, Bonavila C, Bergés M, et al. Myocardial collagen cross-linking is associated with heart failure hospitalization in patients with hypertensive heart failure. J Am Coll Cardiol. (2016) 67:251–60. doi: 10.1016/j.jacc.2015.10.063
161. Zhang ZY, Ravassa S, Yang WY, Petit T, Pejchinovski M, Zürbig P, et al. Diastolic left ventricular function in relation to urinary and serum collagen biomarkers in a general population. PLoS ONE. (2016) 11:1–17. doi: 10.1371/journal.pone.0167582
162. El-Hamamsy I, Balachandran K, Yacoub MH, Stevens LM, Sarathchandra P, Taylor PM, et al. Endothelium-dependent regulation of the mechanical properties of aortic valve cusps. J Am Coll Cardiol. (2009) 53:1448–55. doi: 10.1016/j.jacc.2008.11.056
163. Famaey N, Vastmans J, Fehervary H, Maes L, Vanderveken E, Rega F, et al. Numerical simulation of arterial remodeling in pulmonary autografts. Z Angew Math Mech. (2018) 98:2239–57. doi: 10.1002/zamm.201700351
164. Vastmans J, Maes L, Peirlinck M, Vanderveken E, Rega F, Kuhl E, et al. Growth and remodeling in the pulmonary autograft: computational evaluation using kinematic growth models and constrained mixture theory. Int J Numer Method Biomed Eng. (2021) e3545:1–24. doi: 10.1002/cnm.3545
165. Morrison TM, Pathmanathan P, Adwan M, Margerrison E. Advancing regulatory science with computational modeling for medical devices at the FDA's office of science and engineering laboratories. Front Med. (2018) 5:1–11. doi: 10.3389/fmed.2018.00241
166. Qiao A, Pan Y, Dong N. Modeling study of aortic root for ross procedure: a structural finite element analysis. J Heart Valve Dis. (2014) 23:683–7.
167. Alonso E, Xuan Y, Emmott A, Wang Z, Kumar S, Mongeon F-P, et al. Alonso 2020 wall stress after Ross operation. Circulation. (2020) 142:A16230. doi: 10.1161/circ.142.suppl_3.16230
168. Wu W, Allen RA, Wang Y. Fast-degrading elastomer enables rapid remodeling of a cell-free synthetic graft into a neoartery. Nat Med. (2012) 18:1148–53. doi: 10.1038/nm.2821
Keywords: Ross procedure, pulmonary autograft, mechanobiology, remodeling, extracellular matrix, external support
Citation: Van Hoof L, Verbrugghe P, Jones EAV, Humphrey JD, Janssens S, Famaey N and Rega F (2022) Understanding Pulmonary Autograft Remodeling After the Ross Procedure: Stick to the Facts. Front. Cardiovasc. Med. 9:829120. doi: 10.3389/fcvm.2022.829120
Received: 04 December 2021; Accepted: 17 January 2022;
Published: 09 February 2022.
Edited by:
Anthal Smits, Eindhoven University of Technology, NetherlandsReviewed by:
Paul Human, University of Cape Town, South AfricaCopyright © 2022 Van Hoof, Verbrugghe, Jones, Humphrey, Janssens, Famaey and Rega. This is an open-access article distributed under the terms of the Creative Commons Attribution License (CC BY). The use, distribution or reproduction in other forums is permitted, provided the original author(s) and the copyright owner(s) are credited and that the original publication in this journal is cited, in accordance with accepted academic practice. No use, distribution or reproduction is permitted which does not comply with these terms.
*Correspondence: Lucas Van Hoof, bHVjYXMudmFuaG9vZkBrdWxldXZlbi5iZQ==; bHVjYXN2YW5ob29mMUBnbWFpbC5jb20=
Disclaimer: All claims expressed in this article are solely those of the authors and do not necessarily represent those of their affiliated organizations, or those of the publisher, the editors and the reviewers. Any product that may be evaluated in this article or claim that may be made by its manufacturer is not guaranteed or endorsed by the publisher.
Research integrity at Frontiers
Learn more about the work of our research integrity team to safeguard the quality of each article we publish.