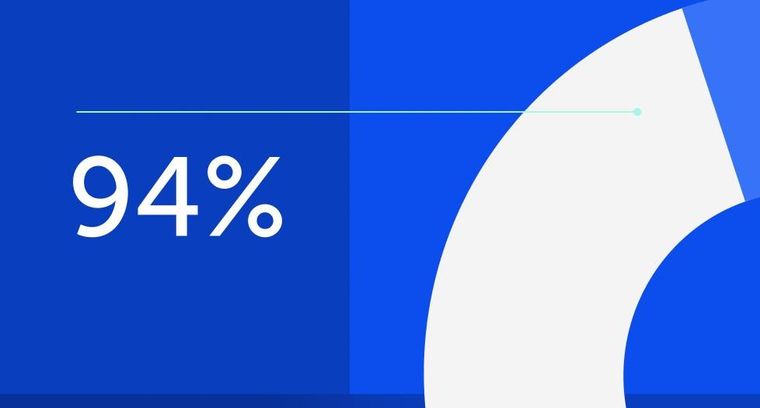
94% of researchers rate our articles as excellent or good
Learn more about the work of our research integrity team to safeguard the quality of each article we publish.
Find out more
MINI REVIEW article
Front. Cardiovasc. Med., 15 February 2022
Sec. Atherosclerosis and Vascular Medicine
Volume 9 - 2022 | https://doi.org/10.3389/fcvm.2022.793072
This article is part of the Research TopicCerebrovasculature in Health and DiseasesView all 13 articles
Intracranial aneurysms (IA) are often asymptomatic and have a prevalence of 3 to 5% in the adult population. The risk of IA rupture is low, however when it occurs half of the patients dies from subarachnoid hemorrhage (SAH). To avoid this fatal evolution, the main treatment is an invasive surgical procedure, which is considered to be at high risk of rupture. This risk score of IA rupture is evaluated mainly according to its size and location. Therefore, angiography and anatomic imaging of the intracranial aneurysm are crucial for its diagnosis. Moreover, it has become obvious in recent years that several other factors are implied in this complication, such as the blood flow complexity or inflammation. These recent findings lead to the development of new IA imaging tools such as vessel wall imaging, 4D-MRI, or molecular MRI to visualize inflammation at the site of IA in human and animal models. In this review, we will summarize IA imaging techniques used for the patients and those currently in development.
Intracranial aneurysms (IA) are pathological focal dilatations of intracranial arteries mainly located at bifurcations of the circle of Willis. IAs are found approximatively in 3.2% of the adult population and are being detected mostly incidentally. Unruptured IAs are commonly asymptomatic but their rupture has severe consequences. Indeed, IA rupture leads to aneurysmal subarachnoid hemorrhage (SAH) which affects 6 in 100,000 persons per year and leads to death for 27–44% of patients (1, 2). Even if the majority of IAs do not evolve toward their rupture, 1 in 200 to 400 will (3). Therefore, there is a need to identify those IAs at risk of rupture in order to treat them and decrease this risk.
In the past few decades, several pathophysiological processes leading to IA rupture were identified as irregular IA shape, an altered hemodynamic stress within the IA and vessel wall inflammation (4). Those findings led to the development of a variety of new imaging tools which provide a better characterization of IAs and enable clinicians to identify those at risk of rupture.
This review will summarize the classical methods of imaging aneurysms and the latest development in the field. It should be noted that this review intend to provide a comprehensive overview of the imaging modalities and discuss their relevance in the field of aneurysmal pathology.
Imaging is a crucial diagnostic tool for the aneurysm's detection and characterization. Indeed, IA imaging can provide detailed information such as its location, size, morphology and geometry, determining the therapeutic strategy (surgical intervention or conservative management) (3). In routine clinical practice, IA are detected and imaged based on their morphology.
Digital subtraction angiography (DSA), a fluoroscopy technique using iodine contrast, is used to produce images of intracranial blood vessels without surrounding tissues as they are removed by digital subtraction (5). Thanks to its high spatial resolution, specificity and sensitivity, DSA is the gold standard for IA imaging and can determine its morphological characteristics such as its size, neck diameter and delineation (3, 6, 7). The development of 3D rotational angiography (3DRA) improved the spatial resolution of DSA, as 3D reconstruction helps to avoid imaging errors related to the superposition of vascular structures, allowing the visualization of small IAs (<3mm) (8). However DSA remains invasive and rare complications exist due to the use of intra-arterial devices and iodine-containing contrast agents during the catheter angiography [neurological: 0.1–1%; severe allergic reaction: 0.05–0.1%] (9).
Several non-invasive imaging techniques have been developed such as computational tomography angiography (CTA). CTA specificity and sensitivity are nearly as good as DSA [sensitivity for IA > 3 mm: 93.3–97.2%; specificity: 87.8–100%] (3, 6, 7). However, CTA is a poor choice for detection of small IAs localized near the skull bone as ionizing ray are almost equally absorbed by calcium and iodinated contrast agents [sensitivity = 61%] (10). Thus, a match mask bone elimination (MMBE) technique has been developed, removing non-specific signal induced by bones, but it requires a longer exposure to ionizing ray and is sensitive to patient movement (11). The advent of dual energy CTA (DE-CTA) subsequently improved material differentiation thereby reducing artifacts created by bony structures without the drawback of the MMBE method (12, 13).
Unlike CTA and DSA, magnetic resonance angiography (MRA) is performed without X-rays. MRA sequences, such as time-of-flight MRA (TOF-MRA) or non-enhanced magnetization-prepared rapid acquisition gradient echo (MPRAGE), do not require contrast agents and is thus considered the least invasive method to date. Non-contrast enhanced MRA gained interest in the last decade due to the well-known health risk of iodinated agents (14). TOF-MRA at 1.5 and 3 Tesla (T) are the most common MRA performed to visualize IAs with a greater sensitivity and accuracy for 3T [Sensitivity: 1.5T = 53.6% vs. 3T = 76.6%; accuracy: 1.5T = 84% vs. 3T = 91.9%] (15, 16). This MRA method relies on the magnetic properties of circulating blood (17). Although this allows for the elimination of contrast agents, some artifacts can be observed especially when the blood flow is turbulent or low, which constitute a limiting factor as those flow disturbances are common in large or coiled aneurysms (17, 18). To alleviate this issue, gadolinium-enhanced MRA (GE-MRA) can be performed as it is flow-independent (18–20). Both TOF- and GE-MRA have 95% sensitivity compared to DSA (6). Recently, 7T MRA has been evaluated in the study of IA. 7T MRI remains infrequent but studies agree on its high potential for the detection of IAs as well as their anatomical description and is a great tool for IA follow-up (21–23). The combination of 7T 3D-TOF and MPRAGE has been demonstrated to delineate unruptured IAs as well as DSA (22). Finally, intracranial black blood vessel imaging (MR-IBBVI), a new MRA sequence based on blood signal suppression, has been compared to TOF-MRA and DSA. Its sensitivity and specificity is higher than TOF-MRA regardless of aneurysm size [Sensitivity: MR-IBBVI = 94.5% vs. TOF-MRA = 62.7%; specificity: MR-IBBVI = 94.5% vs. TOF MRA = 92%; both compared with DSA] (24).
All these IA morphology imaging, with their benefits and disadvantages, summarized in Table 1, have a millimeter spatial resolution which is sufficient for IA detection and morphological characterization and the risk of rupture. However, vessel wall remodeling, which is a main feature of IAs evolving toward rupture, can not be observed with classical imaging mentioned above and there is currently no imaging technique to visualize the elastic lamina disruption or the thinning of the media. Optical coherence tomography (OCT), which is already widely used in ophthalmology, is being optimized for intracranial usage. OCT is based on the differential reflective properties of tissues to near infra-red light. A catheter is introduced in the targeted vessel and 2D cross-sectional images are acquired with a high resolution (1 to 15 μm) (31, 33). It has already been demonstrated that OCT allows the visualization of layers disruption in IA as the delimitations between intima and media layers are no longer visible compared to healthy vessel wall (31, 34). Moreover, the good position of intrasaccular devices can be monitored through OCT in real-time during the surgical procedure (35, 36). The development of such imaging would significantly complement the existing IA rupture risk stratification tools based on IA morphology enabled by current imaging.
All the above-mentioned imaging procedures are performed to assess the morphologic characteristics of IAs, evaluating its rupture risk. However, these parameters seem to be insufficient to accurately predict this evolution toward rupture (37). Indeed, it has been described that hemodynamics stressors are a major cause of IA formation, growth and rupture (38, 39). The main hemodynamic parameters studied are the wall shear stress (WSS defined as the frictional force tangent to vessel wall induced by blood flow), the oscillatory shear index (OSI defined as the direction and intensity flow changes during a cardiac cycle), relative residence time (RRT express the distribution of blood flow over time at the aneurysm wall) and flow patterns (40). Nowadays, a high WSS is commonly accepted to be involved in the formation of IAs, but its role in rupture is less certain as a high or a low WSS can both lead to a destructive remodeling of the aneurysm wall. Indeed, a high WSS is believed to be at the origin of a mural cell-mediated pathological response whereas a low WSS is the source of an inflammatory cell-mediated pathological response (39, 41). However, IA rupture is associated with a higher OSI, a prolonged RTT and complex flow patterns and yet, these hemodynamics parameters are not accessible via the current clinical imaging methods described in morphological imaging section of this review (40–43).
Computational fluid dynamics (CFD), widely used to study hemodynamic parameters, is performed on high-resolution 3D data sets (44). CFD uses static characteristics of IAs (size, location, aspect ratio, size ratio) to calculate WSS, OSI, flow velocity and RRT (39, 45). Thus, CFD is highly influenced by the choice of imaging modality, albeit no imaging modality, so far, has been described as the gold standard to CFD calculations (46, 47). Although CFD is an effective method to calculate hemodynamic parameters and has led to a better understanding of IAs, varying degrees of errors are observed due to some limitations (e.g., considers blood as a Newtonian fluid, arteries as rigid, no standardized protocol) and should be overcome in order to provide important information to clinicians (45, 46, 48).
3DRA is considered the gold standard for the detection and definition of static aneurysm characteristics, however, there are no such clear-cut opinions for dynamic features. In clinical practice, a combination of 2D and 3DRA are used to assess cerebrovascular blood flow. 2D-DSA gives flux information during the contrast-agent passage and 3DRA provides static anatomic information (49, 50). This has led to the development of 4D-DSA, also named time-resolved 3DRA, combining 2D-DSA and 3DRA. This method uses the 3D images obtained with conventional 3DRA and retains the temporal information of these acquisitions, allowing visualization of the influx and efflux of the contrast agent at any angle (51). Vanrossomme et al. reviewed several studies which successfully detected and quantified IA wall deformation between different frames with high spatial and temporal resolutions [35–165 ms and 0.2 mm] (52). Concerning hemodynamics, 4D-DSA applications have mostly been studied in arteriovenous malformations (50) and only one study assessed qualitatively the capacity of this imaging to detect IA flow pattern [excellent visualization in 27.7% of IA and fair visualization in 72.3% of IA] (53). Additionally, Lang et al. demonstrated that 4D-DSA is as reliable as 3DRA for CFD analysis as there is no significant differences in the flow velocity or WSS calculated (54). Moreover, with its high spatial resolution [voxel volume = 0.008 mm3] (51) 4D-DSA allows the same anatomic characterization of IAs than the gold standard 3DRA (54). Thus, 4D-DSA still needs to be improved to achieve a direct quantification of blood hemodynamics but its spatial resolution could allow, in addition to a morphological characterization of IAs, a robust CFD analysis.
Conventionally, to visualize blood flow with magnetic resonance imaging (MRI), a phase-contrast method is performed to access unidirectional flow in a 2D space (55). This 2D phase-contrast MRI has evolved to 3D time-resolved phase-contrast MRI, also called 4D-MRI. This flow imaging quantifies direct blood flow velocity in 3D, allowing flow pattern modeling and quantification of WSS, OSI and vorticity (55–58). In 2020, two complete state-of-the-art reviews on the 4D-MRI's ability to study IA hemodynamics have been published (56, 58). To summarize, 4D-MRI, mostly compared to CFD, reliably depicts intra-aneurysmal flow pattern in different IA morphologies. However, this 4D-imaging still has great limitations, in particular in terms of spatial and temporal resolution which has consequences on the calculation of hemodynamic parameters (depending on magnetic field and acquisition protocol, 4D-MRI = ranging from 0.43 × 0.43 × 0.43 to 1 × 1 × 1.6 mm3 voxels vs. CFD = 0.1-mm voxels) (56, 58). For instance, the WSS values had a lower magnitude when derived from 4D-MRI even if the localization of these WSS are similar (59). Another limit to the use of 4D-MRI in clinic is the long time of acquisition (depending on magnetic field and acquisition protocol, 5–30 min) (58). To overcome this limitation, an accelerated high spatiotemporal resolution 4D-7T-MRI have been proposed, providing accurate quantitative flow values with a 10 min acquisition (vs. 20 min) (60). Moreover, 4D-MRI has also been validated in vitro by comparing its hemodynamic measurements to those obtain by particle image velocimetry (PIV) (61). PIV is an optical imaging method which tracks particle displacement throughout a fluid field illuminated by a laser (62). As an increasingly popular in vitro tool to analyze fluid dynamics and validate medical flux imaging modality, PIV has been used to assess flow pattern in patient IA models with ultra-high spatial and temporal resolution [4Mpixel, 100 images/sec] (63).
Compared to classical CTA, with a longer acquisition time or several acquisitions over a given period, 4D-CTA records the influx and efflux of the contrast product and morphological changes of IA within a cardiac cycle when the acquisition is ECG-gated (64). 4D-CTA is mostly used in the evaluation of hemorrhagic/ischemic stroke and vascular malformations and has been proposed to replace the gold standard 3D-DSA for follow-up imaging since it produces accurate IA geometrics and reliable CFD results when compared to 3DRA (65).
Aside from these classical hemodynamic parameters, the notion of aneurysmal pulsatility arose. Aneurysm pulsation is an important dynamic parameter of IA since increased wall motion is assumed to be linked to a reduced stability of the aneurysm wall and, consequently, to the rupture (52, 66). This pulsation, composed of the global pulsation of the aneurysm and the movement of focal parts (blebs), must be differentiated from the physiological cerebrovascular movement during the cardiac cycle. As those pulsations are quick and of low magnitude, the development of an accurate imaging modality is a real challenge (52, 66). A study performed on 7T MRI quantifying volume pulsation showed insufficient accuracy due to multiple imaging artifacts (67). The most used imaging technique to study aneurysmal pulsation is 4D-CTA (52, 66). This imaging achieves a spatial resolution going up to the same order as the studied IA movements [high-resolution CT scans = 0.25 mm; standard scan = 0.6–0.8 mm] (52). Also, its ability to measure aneurysm pulsation in IAs larger than 5 mm in vivo have been reported (68). These above-mentioned imaging techniques have been summarized in Table 2.
Over the past decades, a growing amount of evidence seems to involve vessel wall inflammation in the pathogenesis of IA (73). Indeed, several histological studies demonstrated that inflammatory cells (mainly T-cells and macrophages) infiltration and complement activation are associated with IA rupture (74, 75). In line with this observation, vessel wall inflammation detection could help to identify IA at high risk of rupture. In order to develop new tools to visualize inflammation in vivo, non-invasive inflammation imaging has been developed over the past few years.
As macrophage infiltration is a typical feature observed in aneurysmal vessel wall, macrophage imaging emerged thanks to the development of ferumoxytol. Ferumoxytol is a superparamagnetic form of iron oxide, engulfed by macrophages and detectable using MRI. Therefore, MRI after ferumoxytol infusion can reflect macrophage activity and associated inflammation within aneurysmal vessel wall. A first histological study analyzed unruptured aneurysm tissues from patients displaying ferumoxytol-induced hyposignal (72h after ferumoxytol infusion) and observed both macrophage infiltration and iron particle uptake by IA vessel wall (69). Intriguingly, they observed a noticeably different level of ferumoxytol uptake among patients, some considered with “early uptake” (visible 24h after infusion) or “late uptake” (visible 72h after infusion) (76). The authors showed that IA with “early uptake” had a similar level of macrophage infiltration compared to ruptured IA, and it was significantly higher in “early uptake” IAs vs. “late uptake” IAs. Finally, all of the “early uptake” IAs managed conservatively evolved to rupture within 6 months while no “late uptake” IAs did. Thus, this study by Hasan et al. suggested that ferumoxytol-MRI could identify unstable IAs at high risk of rupture within 6 months. However, since iron is abundant in red blood cells, subtraction of pre- and post- ferumoxytol infusion images is required to detect ferumoxytol engulfed by macrophages. These pre- and post- infusion images performed independently make this analysis technically difficult and time-consuming therefore, a simpler diagnostic method is desirable (70, 77).
Vessel wall imaging (VWI) has recently emerged as a promising diagnostic tool to image intracranial vessel wall inflammation through MRI. This technique, also known as black blood MRI, provides only signals from the vessel wall thanks to the suppression of both blood and cerebrospinal fluid signal (CSF). The acquisition of VWI demands high resolution, therefore a 3T or higher magnet strength is required. Briefly, VWI generally consist in T1-weighted pre- and post- contrast sequences along with blood and CSF suppression obtained with a 3D turbo spin-echo sequence with variable flip angle refocusing pulses (71). VWI sequence names differ among MRI constructors: VISTA (volume isotropic turbo spin-echo acquisition; Phillips healthcare, Eindhoven, Netherlands), SPACE (sampling perfection with application-optimized contrasts by using different flip angle evolutions; Siemens Healthinners, Erlangen, Germany) or CUBE (GE Healthcare, Milwaukee, WI, USA) (78).
Thanks to blood signal suppression, VWI has been used to study aneurysm wall structure, thickness and wall enhancement. Aneurysm wall enhancement (AWE) is mainly qualitatively assessed and can be classified as focal or circumferential. Radio-histological correlation studies revealed that focal AWE (FAWE) is associated with fresh intraluminal thrombus at the rupture site (79). This finding can provide useful information for the surgical treatment of ruptured IA before treating the patient by microsurgical clipping or endovascular coiling. FAWE can also be observed in unruptured IA and colocalized with hemodynamic factors in favor to a higher rupture risk (80). Moreover, FAWE is observed in areas of morphological changes in the IA vessel wall, supporting the hypothesis that FAWE could be a marker of instability (80). On the other hand, circumferential AWE (CAWE) is thought to be due to wall thickening with abundant inflammatory cell infiltration and neovascularization (78, 81, 82).
In cases of subarachnoid hemorrhage and multiple aneurysms, several criteria are used to determine which one underwent rupture (i.e. hemorrhage localization, IA size, location, shape, aspect ratio). As vessel wall inflammation is a risk factor of IA rupture, AWE is nearly always observed in ruptured IAs (83). Along with this observation, some studies performed on patients presenting multiple IAs, demonstrated that VWI can identify the ruptured IA which is characterized by a thick vessel wall enhancement (84, 85). Thus, VWI can be a useful diagnostic tool in identifying ruptured IA and its site of rupture (79).
With regard to unruptured IA, current research is deciphering the clinical interpretation of AWE. It has been proposed that AWE could be a biomarker of vessel wall inflammation in unstable IAs prone to evolve toward rupture (86). Indeed, some studies performed on unruptured IAs demonstrated a correlation between AWE and common risk factors of IA rupture such as a larger size (≥7 mm), an irregular shape, a high aspect ratio (depth/neck width) and its localization in the anterior cerebral, posterior communicating and posterior cerebral arteries (87–91). A correlation between AWE intensity and the severity of PHASES and ELAPSS scores has also been demonstrated (92).
Finally, a meta-analysis performed on 6 studies analyzed VWI and aneurysm instability. The authors concluded that unstable aneurysms (defined as ruptured, symptomatic, or growing on serial imaging) had statistically higher odds to display AWE. There was still a significant correlation between AWE and IA instability after the removal of ruptured aneurysms (93). Moreover, these meta-analyses highlighted that the absence of wall enhancement on VWI is strongly associated with IA stability (negative predictive value: 96.2%). Very recently, another meta-analysis added 6 more studies, including a longitudinal prospective study, and confirmed these positive and negative predictive value (94, 95). Therefore, VWI and AWE could be a useful risk stratification tools in assessing IAs stability.
Despite potential clinical applications of VWI, it is important to highlight potential limitations of this new diagnostic tool. The meta-analysis demonstrated a moderate specificity (62.7%) and positive predictive value (55.8%) of AWE in identifying unstable aneurysms, meaning that a part of IAs with AWE on VWI are considered to be stable (93). Moreover, flow artifacts within the sac, contrast extravasation and stagnant flow could mimic AWE, leading to false-positive signals (96, 97). In addition, there is no consensus on the definition of AWE as some studies included both FAWE and CAWE whereas others only studied CAWE. Most studies qualitatively assessed AWE inducing a lack of reproducibility, therefore quantitative AWE measurement should be considered (91). Finally, there is a heterogeneity concerning the definition of unstable IA qualified as growth, morphology changes, symptomatic and/or rupture (97). The different inflammation imaging methods have been summarized in Table 2.
Understanding of IA pathophysiology has been largely enabled by the use of small animal models (rat, mouse, rabbit) in which induced IA can mimic aspects of the human pathology. As such, induced IA are smaller than those found in humans thus, the above-mentioned imaging techniques are not widely used to analyze IA's dynamic and static characteristics (98). In fact, only a few studies use them other than as IA detection tools, for instance to detect macrophage infiltration or to perform CFD analysis on 3DRA images (77, 99). To the best of our knowledge, only 2 studies managed to follow aneurysmal remodeling in mice over 3 months using really high-field MRI (9,4T) (100, 101). Regarding IA's hemodynamics, Doppler ultrasound imaging can be used to measure blood flow velocity in rabbit models of internal carotid aneurysm (99, 102, 103).
As IA pathophysiology becomes better understood, new factors contributing to IA progression and rupture are discovered, such as altered hemodynamic parameters or inflammation within IA vessel wall. Novel imaging technique must be developed to visualize these important characteristics and provide essential information's to clinicians for a better IA management. One can speculate that the combination of different imaging techniques that rely on morphological, hemodynamic and inflammatory markers will allow clinicians to accurately assess the risk of aneurysm rupture and adopt the best care strategy.
HL and CM did the literature research and wrote the first draft of the review. YB, HL, and CM contributed to the idea of the manuscript. YB provided critical feedback. All authors reviewed the manuscript and approved the submitted version.
This work was funded by a predoctoral fellowship from the Doctoral School Hématologie – Oncogenèse – Biothérapies ED 561 (to CM) and by the European Research Council grant 759880 (to YB).
The authors declare that the research was conducted in the absence of any commercial or financial relationships that could be construed as a potential conflict of interest.
All claims expressed in this article are solely those of the authors and do not necessarily represent those of their affiliated organizations, or those of the publisher, the editors and the reviewers. Any product that may be evaluated in this article, or claim that may be made by its manufacturer, is not guaranteed or endorsed by the publisher.
We would like to thank Dr. David S. Paul for editing this manuscript.
AWE, Aneurysm wall enhancement; CAWE, Circumferential aneurysm wall enhancement; CFD, Computational fluid dynamics; CSF, Cerebrospinal fluid signal; CTA, Computational tomography angiography; DE-CTA, Dual energy computational tomography angiography; DSA, Digital subtraction angiography; FAWE, Focal aneurysm wall enhancement; GE-MRA, Gadolinium-enhanced magnetic resonance angiography; IA, Intracranial aneurysm; MMBE, Match mask bone elimination; MPRAGE, Magnetization-prepared rapid acquisition gradient echo; MRA, Magnetic resonance angiography; MRI, Magnetic resonance imaging; MR-IBBVI, Magnetic resonance- intracranial black blood vessel imaging; OCT, Optical coherence tomography; OSI, Oscillatory shear index; PIV, Particule image velocimetry; RRT, Relative residence time; SAH, Subarachnoid hemorrhage; T, Tesla; TOF-MRA, Time-of-flight magnetic resonance angiography; VWI, Vessel wall imaging; WSS, Wall shear stress; 3DRA, 3-dimensional rotational angiography.
1. Etminan N, Chang H-S, Hackenberg K, de Rooij NK, Vergouwen MDI, Rinkel GJE, et al. Worldwide incidence of aneurysmal subarachnoid hemorrhage according to region, time period, blood pressure, and smoking prevalence in the population: a systematic review and meta-analysis. JAMA Neurol. (2019) 76:588–97. doi: 10.1001/jamaneurol.2019.0006
2. Nieuwkamp DJ, Setz LE, Algra A, Linn FH, de Rooij NK, Rinkel GJ. Changes in case fatality of aneurysmal subarachnoid haemorrhage over time, according to age, sex, and region: a meta-analysis. Lancet Neurol. (2009) 8:635–42. doi: 10.1016/S1474-4422(09)70126-7
3. Thompson BG, Brown RD, Amin-Hanjani S, Broderick JP, Cockroft KM, Connolly ES, et al. Guidelines for the management of patients with unruptured intracranial aneurysms: a guideline for healthcare professionals from the American heart association/American stroke association. Stroke. (2015) 46:2368–400. doi: 10.1161/STR.0000000000000070
4. Texakalidis P, Sweid A, Mouchtouris N, Peterson EC, Sioka C, Rangel-Castilla L, et al. Aneurysm formation, growth, and rupture: the biology and physics of cerebral aneurysms. World Neurosurg. (2019) 130:277–84. doi: 10.1016/j.wneu.2019.07.093
5. Harrington D, Boxt L, Murray P. Digital subtraction angiography: overview of technical principles. Am J Roentgenol. (1982) 139:781–6. doi: 10.2214/ajr.139.4.781
6. Turan N, Heider RA, Roy AK, Miller BA, Mullins ME, Barrow DL, et al. Current perspectives in imaging modalities for the assessment of unruptured intracranial aneurysms: a comparative analysis and review. World Neurosurg. (2018) 113:280–92. doi: 10.1016/j.wneu.2018.01.054
7. Howard BM, Hu R, Barrow JW, Barrow DL. Comprehensive review of imaging of intracranial aneurysms and angiographically negative subarachnoid hemorrhage. Neurosurg Focus. (2019) 47:E20. doi: 10.3171/2019.9.FOCUS19653
8. Wong SC, Nawawi O, Ramli N, Abd Kadir KA. Benefits of 3D rotational DSA compared with 2D DSA in the evaluation of intracranial aneurysm. Acad Radiol. (2012) 19:701–7. doi: 10.1016/j.acra.2012.02.012
9. Alakbarzade V, Pereira AC. Cerebral catheter angiography and its complications. Pract Neurol. (2018) 18:393–8. doi: 10.1136/practneurol-2018-001986
10. White PM, Wardlaw JM, Easton V. Can noninvasive imaging accurately depict intracranial aneurysms? A systematic review. Radiology. (2000) 217:361–70. doi: 10.1148/radiology.217.2.r00nv06361
11. Venema HW, Hulsmans FJ, den Heeten GJ. CT angiography of the circle of Willis and intracranial internal carotid arteries: maximum intensity projection with matched mask bone elimination-feasibility study. Radiology. (2001) 218:893–8. doi: 10.1148/radiology.218.3.r01mr30893
12. Postma AA, Hofman PAM, Stadler AAR, van Oostenbrugge RJ, Tijssen MPM, Wildberger JE. Dual-energy CT of the brain and intracranial vessels. Am J Roentgenol. (2012) 199:S26–33. doi: 10.2214/AJR.12.9115
13. Zhang L-J, Wu S-Y, Niu J-B, Zhang Z-L, Wang HZ, Zhao Y-E, et al. Dual-Energy CT angiography in the evaluation of intracranial aneurysms: image quality, radiation dose, and comparison with 3D rotational digital subtraction angiography. Am J Roentgenol. (2010) 194:23–30. doi: 10.2214/AJR.08.2290
14. Edelman RR, Koktzoglou I. Non-contrast MR angiography: an update. J Magn Reson Imaging. (2019) 49:355–73. doi: 10.1002/jmri.26288
15. Gibbs GF, Huston J, Bernstein MA, Riederer SJ. Brown RD. Improved image quality of intracranial aneurysms: 30-T versus 15-T time-of-flight MR angiography. AJNR Am J Neuroradiol. (2004) 25:84–7.
16. Kwak Y, Son W, Kim Y-S, Park J, Kang D-H. Discrepancy between MRA and DSA in identifying the shape of small intracranial aneurysms. J Neurosurg. (2020) 134:1887–93. doi: 10.3171/2020.4.JNS20128
17. Yanamadala V, Sheth SA, Walcott BP, Buchbinder BR, Buckley D, Ogilvy CS. Non-contrast 3D time-of-flight magnetic resonance angiography for visualization of intracranial aneurysms in patients with absolute contraindications to CT or MRI contrast. J Clin Neurosci. (2013) 20:1122–6. doi: 10.1016/j.jocn.2012.12.005
18. Cirillo M, Scomazzoni F, Cirillo L, Cadioli M, Simionato F, Iadanza A, et al. Comparison of 3D TOF-MRA and 3D CE-MRA at 3T for imaging of intracranial aneurysms. Eur J Radiol. (2013) 82:e853–9. doi: 10.1016/j.ejrad.2013.08.052
19. Levent A, Yuce I, Eren S, Ozyigit O, Kantarci M. Contrast-enhanced and time-of-flight MR angiographic assessment of endovascular coiled intracranial aneurysms at 1.5 T. Interv Neuroradiol. (2014) 20:686–92. doi: 10.15274/INR-2014-10064
20. Nael K, Villablanca JP, Saleh R, Pope W, Nael A, Laub G, et al. Contrast-enhanced MR angiography at 3T in the evaluation of intracranial aneurysms: a comparison with time-of-flight MR angiography. AJNR Am J Neuroradiol. (2006) 27:2118–21. doi: 10.1097/01.rli.0000242835.00032.f5
21. Wrede KH, Dammann P, Mönninghoff C, Johst S, Maderwald S, Sandalcioglu IE., et al. Non-enhanced MR imaging of cerebral aneurysms: 7 tesla versus 15 tesla. PLoS ONE. (2014) 9:e84562. doi: 10.1371/journal.pone.0084562
22. Wrede KH, Matsushige T, Goericke SL, Chen B, Umutlu L, Quick HH, et al. Non-enhanced magnetic resonance imaging of unruptured intracranial aneurysms at 7 tesla: comparison with digital subtraction angiography. Eur Radiol. (2017) 27:354–64. doi: 10.1007/s00330-016-4323-5
23. Stamm AC, Wright CL, Knopp MV, Schmalbrock P. Heverhagen JT. Phase contrast and time-of-flight magnetic resonance angiography of the intracerebral arteries at 15, 3 and 7 T. Magn Reson Imaging. (2013) 31:545–9. doi: 10.1016/j.mri.2012.10.023
24. Songsaeng D, Sakarunchai I, Mongkolnaowarat S, Harmontree S, Pornpunyawut P, Suwanbundit A, et al. Detection and measurement of intracranial aneurysm compared between magnetic resonance intracranial black blood vessel imaging and gold standard cerebral digital subtraction angiography. J Neurosci Rural Pract. (2020) 11:545–51. doi: 10.1055/s-0040-1714042
25. Anxionnat R, Bracard S, Ducrocq X, Trousset Y, Launay L, Kerrien E, et al. Intracranial aneurysms: clinical value of 3D digital subtraction angiography in the therapeutic decision and endovascular treatment. Radiology. (2001) 218:799–808. doi: 10.1148/radiology.218.3.r01mr09799
26. Foley WD, Karcaaltincaba M. Computed tomography angiography: principles and clinical applications. J Comput Assist Tomogr. (2003) 27:S23–30. doi: 10.1097/00004728-200305001-00006
27. Lell MM, Anders K, Uder M, Klotz E, Ditt H, Vega-Higuera F, et al. New techniques in CT angiography. RadioGraphics. (2006) 26:S45–62. doi: 10.1148/rg.26si065508
28. Fleischmann D, Chin AS, Molvin L, Wang J, Hallett R. Computed tomography angiography. Radiol Clin North Am. (2016) 54:1–12. doi: 10.1016/j.rcl.2015.09.002
29. Saloner D. The AAPM/RSNA physics tutorial for residents. An introduction to MR angiography. RadioGraphics. (1995) 15:453–65. doi: 10.1148/radiographics.15.2.7761648
30. Maki JH, Chenevert TL, Prince MR. Contrast-enhanced MR angiography. Abdom Imaging. (1998) 23:469–84. doi: 10.1007/s002619900384
31. Hoffmann T, Glaßer S, Boese A, Brandstädter K, Kalinski T, Beuing O, et al. Experimental investigation of intravascular OCT for imaging of intracranial aneurysms. Int J CARS. (2016) 11:231–41. doi: 10.1007/s11548-015-1275-1
32. Popescu DP, Choo-Smith L-P, Flueraru C, Mao Y, Chang S, Disano J, et al. Optical coherence tomography: fundamental principles, instrumental designs and biomedical applications. Biophys Rev. (2011) 3:155. doi: 10.1007/s12551-011-0054-7
33. Fujimoto JG, Pitris C, Boppart SA, Brezinski ME. Optical coherence tomography: an emerging technology for biomedical imaging and optical biopsy. Neoplasia. (2000) 2:9–25. doi: 10.1038/sj.neo.7900071
34. Liu Y, Zheng Y, An Q, Song Y, Leng B. Optical coherence tomography for intracranial aneurysms: a new method for assessing the aneurysm structure. World Neurosurg. (2019) 123:e194–201. doi: 10.1016/j.wneu.2018.11.123
35. King RM, Marosfoi M, Caroff J, Ughi GJ, Groth DM, Gounis MJ, et al. High frequency optical coherence tomography assessment of homogenous neck coverage by intrasaccular devices predicts successful aneurysm occlusion. J Neurointerv Surg. (2019) 11:1150–4. doi: 10.1136/neurintsurg-2019-014843
36. Vardar Z, King RM, Kraitem A, Langan ET, Peterson LM, Duncan BH, et al. High-resolution image-guided WEB aneurysm embolization by high-frequency optical coherence tomography. J Neurointerv Surg. (2021) 13:669–73. doi: 10.1136/neurintsurg-2020-016447
37. Xiang J, Natarajan SK, Tremmel M, Ma D, Mocco J, Hopkins LN, et al. Hemodynamic-morphologic discriminants for intracranial aneurysm rupture. Stroke. (2011) 42:144–52. doi: 10.1161/STROKEAHA.110.592923
38. Cebral J, Ollikainen E, Chung BJ, Mut F, Sippola V, Jahromi BR, et al. Flow conditions in the intracranial aneurysm lumen are associated with inflammation and degenerative changes of the aneurysm wall. AJNR Am J Neuroradiol. (2017) 38:119–26. doi: 10.3174/ajnr.A4951
39. Soldozy S, Norat P, Elsarrag M, Chatrath A, Costello JS, Sokolowski JD, et al. The biophysical role of hemodynamics in the pathogenesis of cerebral aneurysm formation and rupture. Neurosurg Focus. (2019) 47:E11. doi: 10.3171/2019.4.FOCUS19232
40. Sheikh MAA, Shuib AS, Mohyi MHH. A review of hemodynamic parameters in cerebral aneurysm. Interdiscipl Neurosurg. (2020) 22:100716. doi: 10.1016/j.inat.2020.100716
41. Sforza DM, Putman CM, Cebral JR. Hemodynamics of cerebral aneurysms. Annu Rev Fluid Mech. (2009) 41:91–107. doi: 10.1146/annurev.fluid.40.111406.102126
42. Jiang P, Liu Q, Wu J, Chen X, Li M, Li Z, et al. A novel scoring system for rupture risk stratification of intracranial aneurysms: a hemodynamic and morphological study. Front Neurosci. (2018) 12:596. doi: 10.3389/fnins.2018.00596
43. Byrne G, Mut F, Cebral J. Quantifying the large-scale hemodynamics of intracranial aneurysms. AJNR Am J Neuroradiol. (2014) 35:333–8. doi: 10.3174/ajnr.A3678
44. Murayama Y, Fujimura S, Suzuki T, Takao H. Computational fluid dynamics as a risk assessment tool for aneurysm rupture. Neurosurg Focus. (2019) 47:E12. doi: 10.3171/2019.4.FOCUS19189
45. Wu T, Zhu Q. Advancement in the haemodynamic study of intracranial aneurysms by computational fluid dynamics. Brain Hemorrhages. (2021) 2:71–5. doi: 10.1016/j.hest.2020.12.002
46. Berg P, Saalfeld S, Voß S, Beuing O, Janiga G. A review on the reliability of hemodynamic modeling in intracranial aneurysms: why computational fluid dynamics alone cannot solve the equation. Neurosurg Focus. (2019) 47:E15. doi: 10.3171/2019.4.FOCUS19181
47. Ren Y, Chen G-Z, Liu Z, Cai Y, Lu G-M, Li Z-Y. Reproducibility of image-based computational models of intracranial aneurysm: a comparison between 3D rotational angiography, CT angiography and MR angiography. Biomed Eng Online. (2016) 15:50. doi: 10.1186/s12938-016-0163-4
48. Saqr KM, Rashad S, Tupin S, Niizuma K, Hassan T, Tominaga T, et al. What does computational fluid dynamics tell us about intracranial aneurysms? A meta-analysis and critical review. J Cereb Blood Flow Metab. (2020) 40:1021–39. doi: 10.1177/0271678X19854640
49. Castillo M. Digital subtraction angiography (DSA): basic principles. In: Ramalho JN, Castillo M (eds), Vascular Imaging of the Central Nervous System. Oxford, UK: John Wiley & Sons, Ltd., (2013). p. 207–220. doi: 10.1002/9781118434550.ch14
50. Ruedinger KL, Schafer S, Speidel MA, Strother CM. 4D-DSA: development and current neurovascular applications. AJNR Am J Neuroradiol. (2021) 42:214–20. doi: 10.3174/ajnr.A6860
51. Davis B, Royalty K, Kowarschik M, Rohkohl C, Oberstar E, Aagaard-Kienitz B, et al. 4D digital subtraction angiography: implementation and demonstration of feasibility. Am J Neuroradiol. (2013) 34:1914–21. doi: 10.3174/ajnr.A3529
52. Vanrossomme AE, Eker OF, Thiran J-P, Courbebaisse GP, Zouaoui Boudjeltia K. Intracranial aneurysms: wall motion analysis for prediction of rupture. AJNR Am J Neuroradiol. (2015) 36:1796–802. doi: 10.3174/ajnr.A4310
53. Kato N, Yuki I, Hataoka S, Dahmani C, Otani K, Abe Y, et al. 4D digital subtraction angiography for the temporal flow visualization of intracranial aneurysms and vascular malformations. J Stroke Cerebrov Dis. (2020) 29:105327. doi: 10.1016/j.jstrokecerebrovasdis.2020.105327
54. Lang S, Hoelter P, Birkhold AI, Schmidt M, Endres J, Strother C, et al. Quantitative and qualitative comparison of 4D-DSA with 3D-DSA using computational fluid dynamics simulations in cerebral aneurysms. AJNR Am J Neuroradiol. (2019) 40:1505–10. doi: 10.3174/ajnr.A6172
55. Markl M, Frydrychowicz A, Kozerke S, Hope M, Wieben O. 4D flow MRI. J Magn Reson Imaging. (2012) 36:1015–36. doi: 10.1002/jmri.23632
56. Castle-Kirszbaum M, Maingard J, Lim RP, Barras CD, Kok HK, Chandra RV, et al. Four-dimensional magnetic resonance imaging assessment of intracranial aneurysms: a state-of-the-art review. Neurosurgery. (2020) 87:453–65. doi: 10.1093/neuros/nyaa021
57. Turski P, Scarano A, Hartman E, Clark Z, Schubert T, Rivera L, et al. Neurovascular 4DFlow MRI (Phase Contrast MRA): emerging clinical applications. Neurovasc Imaging. (2016) 2:8. doi: 10.1186/s40809-016-0019-0
58. Youn SW, Lee J. From 2D to 4D phase-contrast MRI in the neurovascular system: will it be a quantum jump or a fancy decoration? J Magn Reson Imaging. (2020) 55:347–72. doi: 10.1002/jmri.27430
59. Szajer J, Ho-Shon K. A comparison of 4D flow MRI-derived wall shear stress with computational fluid dynamics methods for intracranial aneurysms and carotid bifurcations - a review. Magn Reson Imaging. (2018) 48:62–9. doi: 10.1016/j.mri.2017.12.005
60. Gottwald LM, Töger J, Bloch KM, Peper ES, Coolen BF, Strijkers GJ, et al. High spatiotemporal resolution 4D flow MRI of intracranial aneurysms at 7T in 10 minutes. Am J Neuroradiol. (2020) 41:1201–8. doi: 10.3174/ajnr.A6603
61. Medero R, Ruedinger K, Rutkowski D, Johnson K, Roldán-Alzate A. In vitro assessment of flow variability in an intracranial aneurysm model using 4D flow MRI and tomographic PIV. Ann Biomed Eng. (2020) 48:2484–93. doi: 10.1007/s10439-020-02543-8
62. Ruedinger KL, Medero R, Roldán-Alzate A. Fabrication of low-cost patient-specific vascular models for particle image velocimetry. Cardiovasc Eng Technol. (2019) 10:500–7. doi: 10.1007/s13239-019-00417-2
63. Medero R, Hoffman C, Roldán-Alzate A. Comparison of 4D flow MRI and particle image velocimetry using an in vitro carotid bifurcation model. Ann Biomed Eng. (2018) 46:2112–22. doi: 10.1007/s10439-018-02109-9
64. Kortman HGJ, Smit EJ, Oei MTH, Manniesing R, Prokop M, Meijer FJA. 4D-CTA in neurovascular disease: a review. AJNR Am J Neuroradiol. (2015) 36:1026–33. doi: 10.3174/ajnr.A4162
65. Cancelliere NM, Najafi M, Brina O, Bouillot P, Vargas MI, Lovblad K-O, et al. 4D-CT angiography versus 3D-rotational angiography as the imaging modality for computational fluid dynamics of cerebral aneurysms. J Neurointerv Surg. (2020) 12:626–30. doi: 10.1136/neurintsurg-2019-015389
66. Stam LB, Aquarius R, de Jong GA, Slump CH, Meijer FJA, Boogaarts HD, et al. review on imaging techniques and quantitative measurements for dynamic imaging of cerebral aneurysm pulsations. Sci Rep. (2021) 11:2175. doi: 10.1038/s41598-021-81753-z
67. Kleinloog R, Zwanenburg JJM, Schermers B, Krikken E, Ruigrok YM, Luijten PR, et al. Quantification of intracranial aneurysm volume pulsation with 7T MRI. AJNR Am J Neuroradiol. (2018) 39:713–9. doi: 10.3174/ajnr.A5546
68. Gu Y, Zhang Y, Luo M, Zhang H, Liu X, Miao C. Risk factors for asymptomatic intracranial small aneurysm rupture determined by electrocardiographic-gated 4D computed tomographic (CT) angiography. Med Sci Monit. (2020) 26:e921835-1–e921835-8. doi: 10.12659/MSM.921835
69. Hasan DM, Mahaney KB, Magnotta VA, Kung DK, Lawton MT, Hashimoto T, et al. Macrophage imaging within human cerebral aneurysms wall using ferumoxytol-enhanced MRI: a pilot study. ATVB. (2012) 32:1032–8. doi: 10.1161/ATVBAHA.111.239871
70. Shimizu K, Kushamae M, Aoki T. Macrophage imaging of intracranial aneurysms. Neurol Med Chir(Tokyo). (2019) 59:257–63. doi: 10.2176/nmc.st.2019-0034
71. Leao DJ, Agarwal A, Mohan S, Bathla G. Intracranial vessel wall imaging: applications, interpretation, and pitfalls. Clin Radiol. (2020) 75:730–9. doi: 10.1016/j.crad.2020.02.006
72. Mandell DM, Mossa-Basha M, Qiao Y, Hess CP, Hui F, Matouk C, et al. Intracranial vessel wall MRI: principles and expert consensus recommendations of the American society of neuroradiology. AJNR Am J Neuroradiol. (2017) 38:218–29. doi: 10.3174/ajnr.A4893
73. Chalouhi N, Ali MS, Jabbour PM, Tjoumakaris SI, Gonzalez LF, Rosenwasser RH, et al. Biology of intracranial aneurysms: role of inflammation. J Cereb Blood Flow Metab. (2012) 32:1659–76. doi: 10.1038/jcbfm.2012.84
74. Tulamo R, Frösen J, Junnikkala S, Paetau A, Pitkäniemi J, Kangasniemi M, et al. Complement activation associates with saccular cerebral artery aneurysm wall degeneration and rupture. Neurosurgery. (2006) 59:1069–76. doi: 10.1227/01.NEU.0000245598.84698.26
75. Frösen J, Piippo A, Paetau A, Kangasniemi M, Niemelä M, Hernesniemi J, et al. Remodeling of saccular cerebral artery aneurysm wall is associated with rupture: histological analysis of 24 unruptured and 42 ruptured cases. Stroke. (2004) 35:2287–93. doi: 10.1161/01.STR.0000140636.30204.da
76. Hasan D, Chalouhi N, Jabbour P, Dumont AS, Kung DK, Magnotta VA, et al. Early change in ferumoxytol-enhanced magnetic resonance imaging signal suggests unstable human cerebral aneurysm: a pilot study. Stroke. (2012) 43:3258–65. doi: 10.1161/STROKEAHA.112.673400
77. Aoki T, Saito M, Koseki H, Tsuji K, Tsuji A, Murata K, et al. Macrophage imaging of cerebral aneurysms with ferumoxytol: an exploratory study in an animal model and in patients. J Stroke Cerebrovasc Dis. (2017) 26:2055–64. doi: 10.1016/j.jstrokecerebrovasdis.2016.10.026
78. Matsushige T, Shimonaga K, Mizoue T, Hosogai M, Hashimoto Y, Takahashi H, et al. Lessons from vessel wall imaging of intracranial aneurysms: new era of aneurysm evaluation beyond morphology. Neurol Med Chir. (2019) 59:407–14. doi: 10.2176/nmc.ra.2019-0103
79. Matsushige T, Shimonaga K, Mizoue T, Hosogai M, Hashimoto Y, Kaneko M, et al. Focal aneurysm wall enhancement on magnetic resonance imaging indicates intraluminal thrombus and the rupture point. World Neurosurg. (2019) 127:e578–84. doi: 10.1016/j.wneu.2019.03.209
80. Larsen N, Flüh C, Saalfeld S, Voß S, Hille G, Trick D, et al. Multimodal validation of focal enhancement in intracranial aneurysms as a surrogate marker for aneurysm instability. Neuroradiology. (2020) 62:1627–35. doi: 10.1007/s00234-020-02498-6
81. Zhong W, Su W, Li T, Tan X, Chen C, Wang Q, et al. Aneurysm wall enhancement in unruptured intracranial aneurysms: a histopathological evaluation. J Am Heart Assoc. (2021) 10:e018633. doi: 10.1161/JAHA.120.018633
82. Shimonaga K, Matsushige T, Ishii D, Sakamoto S, Hosogai M, Kawasumi T, et al. Clinicopathological insights from vessel wall imaging of unruptured intracranial aneurysms. Stroke. (2018) 49:2516–9. doi: 10.1161/STROKEAHA.118.021819
83. Wang X, Zhu C, Leng Y, Degnan AJ, Lu J. Intracranial aneurysm wall enhancement associated with aneurysm rupture: a systematic review and meta-analysis. Acad Radiol. (2019) 26:664–73. doi: 10.1016/j.acra.2018.05.005
84. Matouk CC, Mandell DM, Günel M, Bulsara KR, Malhotra A, Hebert R, et al. Vessel wall magnetic resonance imaging identifies the site of rupture in patients with multiple intracranial aneurysms: proof of principle. Neurosurgery. (2013) 72:492–6. doi: 10.1227/NEU.0b013e31827d1012
85. Kondo R, Yamaki T, Mouri W, Sato S, Saito S, Nagahata M, et al. Magnetic resonance vessel wall imaging reveals rupture site in subarachnoid hemorrhage with multiple cerebral aneurysms. No Shinkei Geka. (2014) 42:1147–50.
86. Hu P, Yang Q, Wang D-D, Guan S-C, Zhang H-Q. Wall enhancement on high-resolution magnetic resonance imaging may predict an unsteady state of an intracranial saccular aneurysm. Neuroradiology. (2016) 58:979–85. doi: 10.1007/s00234-016-1729-3
87. Liu P, Qi H, Liu A, Lv X, Jiang Y, Zhao X, et al. Relationship between aneurysm wall enhancement and conventional risk factors in patients with unruptured intracranial aneurysms: a black-blood MRI study. Interv Neuroradiol. (2016) 22:501–5. doi: 10.1177/1591019916653252
88. Lv N, Karmonik C, Chen S, Wang X, Fang Y, Huang Q, et al. Wall enhancement, hemodynamics, and morphology in unruptured intracranial aneurysms with high rupture risk. Transl Stroke Res. (2020) 11:882–9. doi: 10.1007/s12975-020-00782-4
89. Lv N, Karmonik C, Chen S, Wang X, Fang Y, Huang Q, et al. Relationship between aneurysm wall enhancement in vessel wall magnetic resonance imaging and rupture risk of unruptured intracranial aneurysms. Neurosurgery. (2019) 84:E385–91. doi: 10.1093/neuros/nyy310
90. Wang G-X, Li W, Lei S, Ge X-D, Yin J-B, Zhang D. Relationships between aneurysmal wall enhancement and conventional risk factors in patients with intracranial aneurysm: a high-resolution MRI study. J Neuroradiol. (2019) 46:25–8. doi: 10.1016/j.neurad.2018.09.007
91. Zhang Y, Fu Q, Wang Y, Cheng J, Ren C, Guan S, et al. Qualitative and quantitative wall enhancement analyses in unruptured aneurysms are associated with an increased risk of aneurysm instability. Front Neurosci. (2020) 14:580205. doi: 10.3389/fnins.2020.580205
92. Bae H, Suh S-I, Yoon WK, Roh H, Kim C, Kwon T-H. Correlation of aneurysmal wall enhancement of unruptured intracranial aneurysms on high-resolution vessel-wall imaging with clinical indices and surgical findings. Neurosurgery. (2021) 89:420–7. doi: 10.1093/neuros/nyab178
93. Texakalidis P, Hilditch CA, Lehman V, Lanzino G, Pereira VM, Brinjikji W. Vessel wall imaging of intracranial aneurysms: systematic review and meta-analysis. World Neurosurg. (2018) 117:453–8.e1. doi: 10.1016/j.wneu.2018.06.008
94. Vergouwen MDI, Backes D, van der Schaaf IC, Hendrikse J, Kleinloog R, Algra A, et al. Gadolinium enhancement of the aneurysm wall in unruptured intracranial aneurysms is associated with an increased risk of aneurysm instability: a follow-up study. AJNR Am J Neuroradiol. (2019) 40:1112–6. doi: 10.3174/ajnr.A6105
95. Molenberg R, Aalbers MW, Appelman APA, Uyttenboogaart M, Dijk JMC. Intracranial aneurysm wall enhancement as an indicator of instability: a systematic review and meta-analysis. Eur J Neurol. (2021) 28:3837–48. doi: 10.1111/ene.15046
96. Kalsoum E, Chabernaud Negrier A, Tuilier T, Benaïssa A, Blanc R, Gallas S, et al. Blood flow mimicking aneurysmal wall enhancement: a diagnostic pitfall of vessel wall MRI using the postcontrast 3D turbo spin-echo MR imaging sequence. AJNR Am J Neuroradiol. (2018) 39:1065–7. doi: 10.3174/ajnr.A5616
97. Samaniego EA, Roa JA, Hasan D. Vessel wall imaging in intracranial aneurysms. J NeuroIntervent Surg. (2019) 11:1105–12. doi: 10.1136/neurintsurg-2019-014938
98. Tutino VM, Rajabzadeh-Oghaz H, Veeturi SS, Poppenberg KE, Waqas M, Mandelbaum M, et al. Endogenous animal models of intracranial aneurysm development: a review. Neurosurg Rev. (2021) 44:2545–70. doi: 10.1007/s10143-021-01481-w
99. Zeng Z, Kallmes DF, Durka MJ, Ding Y, Lewis D, Kadirvel R, et al. Hemodynamics and anatomy of elastase-induced rabbit aneurysm models: similarity to human cerebral aneurysms? Am J Neuroradiol. (2011) 32:595–601. doi: 10.3174/ajnr.A2324
100. Tutino VM, Rajabzadeh-Oghaz H, Chandra AR, Gutierrez LC, Schweser F, Preda M., et al. 94T magnetic resonance imaging of the mouse circle of willis enables serial characterization of flow-induced vascular remodeling by computational fluid dynamics. Curr Neurovasc Res. (2018) 15:312–25. doi: 10.2174/1567202616666181127165943
101. Rajabzadeh-Oghaz H, Chandra AR, Gutierrez LC, Schweser F, Ionita C, Siddiqui A, et al. High-resolution MRI of the mouse cerebral vasculature to study hemodynamic-induced vascular remodeling. Proc SPIE. (2019) 10953:1–8. doi: 10.1117/12.2511772
102. Lyu Y, Luo J, Zhang Y, Wang C, Li A, Zhou Y, et al. An effective and simple way to establish elastase-induced middle carotid artery fusiform aneurysms in rabbits. Biomed Res Int. (2020) 2020:6707012. doi: 10.1155/2020/6707012
Keywords: intracranial aneurysm, vessel wall imaging, imaging technique, hemodynamic imaging, inflammation imaging
Citation: Maupu C, Lebas H and Boulaftali Y (2022) Imaging Modalities for Intracranial Aneurysm: More Than Meets the Eye. Front. Cardiovasc. Med. 9:793072. doi: 10.3389/fcvm.2022.793072
Received: 11 October 2021; Accepted: 17 January 2022;
Published: 15 February 2022.
Edited by:
Margreet R. De Vries, Leiden University Medical Center, NetherlandsReviewed by:
Philipp Berg, Otto von Guericke University Magdeburg, GermanyCopyright © 2022 Maupu, Lebas and Boulaftali. This is an open-access article distributed under the terms of the Creative Commons Attribution License (CC BY). The use, distribution or reproduction in other forums is permitted, provided the original author(s) and the copyright owner(s) are credited and that the original publication in this journal is cited, in accordance with accepted academic practice. No use, distribution or reproduction is permitted which does not comply with these terms.
*Correspondence: Héloïse Lebas, aGVsb2lzZS5sZWJhc0BpbnNlcm0uZnI=
Disclaimer: All claims expressed in this article are solely those of the authors and do not necessarily represent those of their affiliated organizations, or those of the publisher, the editors and the reviewers. Any product that may be evaluated in this article or claim that may be made by its manufacturer is not guaranteed or endorsed by the publisher.
Research integrity at Frontiers
Learn more about the work of our research integrity team to safeguard the quality of each article we publish.