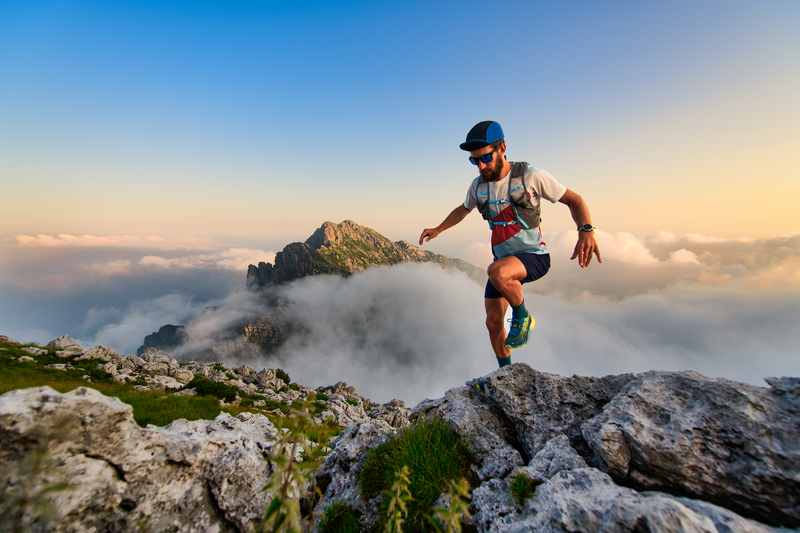
95% of researchers rate our articles as excellent or good
Learn more about the work of our research integrity team to safeguard the quality of each article we publish.
Find out more
REVIEW article
Front. Cardiovasc. Med. , 10 January 2023
Sec. Clinical and Translational Cardiovascular Medicine
Volume 9 - 2022 | https://doi.org/10.3389/fcvm.2022.1041481
This article is part of the Research Topic Therapeutic Ultrasound in Cardiovascular Disease View all 5 articles
Cardiovascular diseases rank the highest incidence and mortality worldwide. As the most common type of cardiovascular disease, myocardial infarction causes high morbidity and mortality. Recent studies have revealed that extracellular vesicles, including exosomes, show great potential as a promising cell-free therapy for the treatment of myocardial infarction. However, low heart-targeting efficiency and short plasma half-life have hampered the clinical translation of extracellular vesicle therapy. Currently, four major types of strategies aiming at enhancing target efficiency have been developed, including modifying EV surface, suppressing non-target absorption, increasing the uptake efficiency of target cells, and utilizing a hydrogel patch. This presented review summarizes the current research aimed at EV heart targeting and discusses the challenges and opportunities in EV therapy, which will be beneficial for the development of effective heart-targeting strategies.
Cardiovascular diseases (CVDs) rank the highest incidence and mortality worldwide (1–4). It is estimated that the number of deaths caused by CVD is 17.3 million every year, and this figure is expected to surpass 23.6 million by 2030 (5). Coronary artery disease, especially myocardial infarction (MI), is the most common type of CVDs and has become a major contributor to high morbidity and mortality (6). Myocardial infarction causes a large amount of myocardium loss, extracellular matrix disorder, and scar formation (7, 8). Continuous loss of numerous cardiomyocytes finally leads to ventricular remodeling and heart failure (9). Although the clinical adoption of drug therapy, thrombolysis, coronary stent placement, and coronary artery bypass grafting has improved the survival rate for patients with MI, these methods fail to effectively reduce the myocardial injury caused by ischemia/reperfusion and restore heart function (10).
Cell-based therapies, such as mesenchymal stem cells (MSCs), have shown great potential for the treatment of MI. However, the clinical application of cell therapies in MI is somehow disappointing: It is reported that compared to traditional treatment, stem cell therapy did not reduce the risk of rehospitalization for heart failure or the combined risk of death, neither resulting in any improvement in heart function (11). In addition, there are some unexpected side effects brought by cell therapies, for example, MSCs may transform into fibroblasts and thus aggravate myocardial fibrosis (12). Moreover, MSCs might trigger severe calcification in the heart (13). Recent studies suggest that cell-mediated paracrine signaling, rather than differentiation, is the main mechanism of cell-based therapy, and extracellular vesicles (EVs) played an important role in this process (14, 15).
Extracellular vesicles are nano- to micron-sized vesicles ranging from 30 to 1,000 nm in diameter, which were secreted by almost all types of cells. The International Society for Extracellular Vesicles (ISEV) has recommended that particles with lipid bilayers that have been released from cells are referred to as EVs (16). Generally, EVs are classified into three subgroups: exosomes, microvesicles, and apoptotic bodies. Due to the overlap in physicochemical properties and lack of definitive molecular markers, it remains challenging to accurately distinguish EV subtypes (17–20). Hence, it is preferable to use the generic term “extracellular vesicles” rather than exosomes. The therapeutic function of EVs can be attributed to their capacity to deliver curative substances to the recipient cells. These substances include non-coding RNAs, mRNAs, proteins, and mitochondria (21–24). The contents of EVs have the potential to trigger a variety of cardioprotective effects, including increasing the survival of cardiomyocytes and endothelial cells, suppressing oxidative stress and inflammatory response, reducing scar formation and left ventricular remodeling, and stimulating post-ischemic angiogenesis (22–24). Compared with conventional cytotherapy, EVs show several advantages as follows: (1) EVs transplantation is cell-free therapy, which is safer than cell transplantation due to that EVs present a low risk of tumorigenicity or trigger an arrhythmogenic response, as well as low immunogenicity and high biocompatibility (25–29); (2) the condition required for EVs storage and transportation is less harsh than cells, and the components of EVs remain stable at −20 to −80°C for a long time without degradation (30–33); (3) in the treatment of MI, MSCs transplanted at the site of myocardial infarction region cannot withstand the ischemic and hypoxic microenvironment and end up mass dying. On the contrary, EVs have no such defect (34); (4) EVs can be loaded with therapeutic cargo and targeted delivery to the lesion zone (26). In terms of drug delivery, viral vectors can transfer gene cargo efficiently and overcome cellular barriers. However, the broad use of viral vectors is unfortunately constrained due to their intrinsic drawbacks, which include tropism, safety issues, production challenges, and a restricted capacity for packaging. Non-viral vectors, such as lipid nanoparticles and liposomes, have been shown to be flexible, are easy to modify, and have high gene packaging capacity. In contrast to natural vectors, artificial vectors are much simpler, so they have difficulties precisely targeting and crossing multiple barriers in vivo (35). EVs can be consider as “nature’s lipid nanoparticles,” as they originate from biological systems (36). Compared with cells and other drug-delivery vehicles, EVs have several advantages because of their natural structure. For example, artificial nanoparticles are easily removed from the bloodstream, whereas natural EVs could avoid this clearance mechanism to a great extent. Furthermore, EVs’ cell-derived membranes granted them minimal toxicity and immunological covertness (37). Lastly, EVs inherit functions of their source cells, as MSC-derived EVs and MSCs are reported to yield similar therapeutic benefits (34, 38). A large number of preclinical experiments have proven that EVs are a promising “medicine” in tackling cardiovascular disease (34, 38–59). Meanwhile, the side effects of EV-based treatments have scarcely been reported. MSCs contribute to tissue repair and regeneration mainly through EV-mediated paracrine, which makes them an ideal source for EV production. Both in vitro and in vivo studies have demonstrated that MSCs are high-yielding sources of EVs, and MSC-derived EVs can improve cardiac functions in ischemia–reperfusion injury (26, 60).
To effectively translate EV-based therapies from bench to clinic, how to improve the short plasma half-life and low targeting efficiency of EVs is pivotal. In most animal experiments, EVs were administered via intramyocardial or intracoronary injection (34, 58, 59). These are undoubtedly efficient methods for EV delivery but are unsuitable for clinical practice, as the processes are invasive, complex, and high-risk practice. In contrast, intravenous delivery is reproducible and technically easier, which make it more preferred due to that patients with MI can poorly tolerate repeat invasive operation. However, this approach is hampered by off-target binding and poor accumulation of EVs in the cardiac tissue. Since it has been revealed that after intravenous administration, EVs are distributed largely to the liver, the lungs, the kidneys, and the spleen (61–65).
It is reported that less than 10% of EVs administrated by intravenous injection are finally absorbed by an uninjured heart (22); therefore, to counteract non-specific delivery, an extra dose of EVs is needed. EVs delivered by a systemic route are barely absorbed by the interstitial myocardium because the myocardial capillary endothelium is about 4 nm in diameter and is continuous with tight junctions, which facilitates the transfer of blood-borne substances but limits the movement of EVs (66, 67).
Owing to EV off-target effects and the low production yield, there is an urgent need to improve the targeted delivery of EVs to cardiomyocytes to accelerate the clinical application of EV therapy. The distribution of EVs can be influenced by many factors, including delivery mode, EVs’ size, injected dose, membrane components of EVs, as well as the type of EV receptor cells and donor cells (68–73). However, the exact mechanisms underlying EV biodistribution and uptake in vivo are still unclear so far. In this review, we summarize the study’s aim to increase the accumulation of EVs in the myocardium and discuss how this field can be elevated.
Most naturally secreted EVs show limited tropism to specific cell types. Thus far, four major types of strategies aimed at enhancing target efficiency have been described, including modifying EV surface to improve heart targeting, suppressing the non-target absorption, increasing the uptake efficiency of target cells, and employing the hydrogel patch (Figure 1). The improvement of effective targeting and delivery methods for EVs will lead to a paradigm shift in the development of more effective, more economical, and less-invasive treatments for many types of cardiovascular diseases.
Figure 1. Strategies for enhancing extracellular vesicle (EV) heart-targeting efficiency. (1) EV surface modification; (2) suppressing EV non-target absorption; (3) increasing the uptake efficiency of target cells; (4) hydrogel patch. This figure contains modified images from Servier Medical Art (https://smart.servier.com) licensed by a Creative Commons Attribution 3.0 Unported License.
Extracellular vesicles are internalized by target cells via membrane fusion, phagocytosis, micropinocytosis, and endocytosis (71). EV binding and uptaking differ between deriving cells and target cells (74). The surface molecules of EVs, such as tetraspanins, integrins, proteoglycans, and lectins, play a crucial role in these processes (17). Since differential protein expression has been documented between normal and ischemic myocardium, (75, 76), the principal approach to confer myocardium-targeting specificity to EVs is through surface modification. EVs engineered to over-express specific surface peptides with ischemia myocardial selectivity have demonstrated improved targeting performance. Through the application of phage display and in vivo biopanning techniques, which are widely used for the identification of cell targeting peptides (CTP), many myocardium homing peptides have been found and verified, and these peptides can target ischemic myocardium (77). The coding sequences of CSTSMLKAC are the most frequently utilized myocardium-targeting peptide (77–82). The examples of EV surface modification for myocardial targeted delivery are shown in Table 1.
Surface modification can be achieved through biological, chemical, or physical methods. For biological modification, by using gene transfer vectors, such as adeno-associated viruses (AAVs) or lentiviruses, to encode the sequences of myocardium-targeting peptide incorporated into the EV-donor cells genome, the host cells are genetically modified to express a myocardial homing peptide. This myocardial homing peptide is then fused with transmembrane proteins that are expressed on the surface of EVs (87). The lysosome-associated membrane glycoprotein 2B (LAMP-2B), which belongs to the lysosomal-associated membrane protein (LAMP) family and is expressed on EV membrane, is the most commonly used membrane protein (82–84, 88, 89). It comprises an N-terminal extramembrane domain and a C-terminal transmembrane domain, and the targeting peptide can be fused with the N-terminal extracellular domain of LAMP-2B to achieve a targeting effect.
By introducing vectors encoding cardiac-targeting peptide (CTP)-Lamp2B into HEK 293 cells, Kim et al. (83) generated CTP-expressing EVs. The in vitro and in vivo delivery efficiencies of the CTP-expressing EVs to the hearts were increased. Compared with blank EVs, a significant increase in targeting ischemic myocardium was observed in the myocardium-targeting peptide-engineered EVs, resulting in remarkable suppression of inflammation and cardiomyocyte apoptosis, enhanced angiogenesis, and reduced infarct size in a mouse MI model. A similar approach was employed by Mentkowski et al. (84), and they engineered cardiosphere-derived cells (CDCs) to express Lamp2b, which was then fused to a cardiomyocyte-specific peptide WLSEAGPVVTVRALRGTGSW. The EVs isolated from those engineered CDCs expressed cardiomyocyte-specific peptide on the surface and resulted in higher cardiac retention.
The surface of EVs can also be modified by a chemical approach after isolation. Compared with the biological modification method, chemical reactions are more rapid, efficient, and simple. Zhu et al. (78) conjugated EVs with an ischemic myocardium-targeted peptide by bio-orthogonal chemistry and found that modified EVs showed specific targeting to the ischemic lesions in the injured heart and exerted a marked cardioprotective function post-MI. Antes et al. (80) described an EV membrane anchoring platform termed “cloaking” to embed targeting moieties on EV surfaces. The cloaking system consists of three components: a DMPE phospholipid membrane anchor, a polyethylene glycol spacer, and a conjugated streptavidin platform molecule, to which any biotinylated molecule can be coupled for EV decoration. Vandergriff et al. (79) and Wang et al. (81) conjugated EVs with cardiac homing peptide through a dioleoylphosphatidylethanolamine N-hydroxysuccinimide (DOPE-NHS) linker and demonstrated enhanced homing efficiency of the injured heart and improved therapeutic outcomes.
Instead of genetically modifying EV donor cells or chemically modifying EVs, the physical approach of modifying the surface of EVs is membrane fusion. Zhang et al. (86) fused monocyte cell membranes with mesenchymal stem cell-derived EVs to generate monocyte mimetic EVs, which was performed by the fusion-extrusion method. These modified EVs were endowed with the inflammatory targeting features of monocytes, and these monocyte mimetic EVs could infiltrate injured myocardium from circulation. This approach exhibited a high targeting efficiency for injured myocardium and improved therapeutic outcomes in cardiac function. By extruding EVs and platelet membranes through 200 nm filters, Hu et al. (85) mixed platelet membranes and MSC-derived EVs to increase their macropinocytosis-mediated cellular internalization, as well as their ability to target the injured tissue. They found that platelet membrane modification of EVs significantly enhanced the cellular uptake of EVs by endothelial cells and cardiomyocytes and reduced the uptake of EVs by the mononuclear phagocyte system in vivo.
Surface peptide engineering conferred EVs with advanced targeting performance. However, the impacts of these methods on EV biological features are unknown. Whether this surface engineering performance will trigger side effects such as immunogenic activities or affect the normal functions of exosome membrane proteins still needs further investigation. Moreover, modification of the EV-deriving cells is time-consuming and exhausting, and these methods may damage EV membrane stability and integrity or lead to a low yield of EVs (90, 91). In addition, surface modification cannot load multiple surface peptides simultaneously (90). Another concern is that peptides displayed on the EV surface are unstable and may degrade. Hung et al. (92) demonstrated that membrane display by fusion with LAMP-2B may cause ligand loss. To protect peptides from degradation, engineered targeted peptide-Lamp2b fusion proteins with a glycosylation motif are experimentally feasible (83, 92).
As exogenous vesicles in circulation, a challenge faced by EVs is the rapid hepatic clearance mediated by macrophages. Previous studies have shown that natural EVs can only be detected in the circulatory system for up to 1–3 h in normal mice after tail vein injection (63, 93, 94). Consequently, there has long been an interest in suppressing the non-specific uptake of EVs, prolonging the retention time of EVs in circulation, and, therefore, improving their chances of being absorbed by the heart.
CD47 is a transmembrane protein that enables cancer cells to evade clearance by macrophages through the initiation of a “don’t eat me” signal. By applying the EVs isolated from CD47 overexpressing MSCs, Wei et al. (95) prolonged the retention of CD47-EVs in the plasma, thus improving EV biodistribution in the heart.
Wan et al. (64) developed a strategy that uses blocking EVs to suppress the endocytosis of EVs by macrophages at first and then deliver therapeutic EVs to the heart. Blocking EVs were prepared by encapsulating EVs with siClathrin, which prevents EVs clathrin-dependent endocytosis by the macrophages in the liver and the spleen. This method significantly decreased the uptake of EVs by macrophages and improved the delivery efficiency of EVs to the heart. However, extra EVs are needed to produce blocking EVs in this method, which may lead to increased costs due to the low yield of EVs.
Suppressing the monocyte/macrophage or reticuloendothelial system to promote EVs absorption by the heart can be termed as “passive targeting,” which means after being injected into the circulation, EVs can avoid non-specific entrapment by the reticuloendothelial system and concentrate in the heart. The key dilemma in this strategy is that if other lesions were present, such as tumors, these lesions may competitively uptake EVs in vivo (96).
Once EVs are distributed to the desired tissues or organs, the increase in cell-specific uptake is another challenge. Targeting EVs to the heart is difficult, partly because the intact endothelial barriers block the entry of EVs, so promoting the uptake efficiency of cardiomyocytes is a practical approach for EV delivery. It has been reported that endocytosis is the major pathway of EV uptake (72, 97). Although other uptake routes also exist (71, 98, 99), including phagocytosis, membrane fusion, and micropinocytosis, the mechanism by which these processes are regulated remains unclear.
Ultrasound-targeted microbubble destruction (UTMD), which means burst gas-filled microbubbles under the assistance of ultrasound, can enlarge the capillary gaps and induce transient pores on cell membranes to promote the endocytosis of EVs (100–102), thus transiently enhancing the uptake of EVs by the target tissue. Particularly, when combined with ultrasound, the delivery of EVs can be controlled and enhanced in cardiomyocytes. Sun et al. proved that UTMD can significantly increase the EVs endocytosis by cardiomyocytes (65, 103). Though UTMD is an efficient and controllable targeting technique, high ultrasound pressures and corresponding microbubble generation may induce adverse effects (103). Therefore, the key to success is to optimize UTMD settings.
Alternatively, another study has used a magnet and pH-based method to improve the cardiomyocyte targeting specificity. Liu et al. (104) constructed pH-responsive magnetic nanoparticles decorated with two types of antibodies, which bind either to CD63 antigens on the surface of EVs or to myosin-light-chain surface markers on injured cardiomyocytes. These nanoparticles captured circulating EVs in vivo and the magnetic field guided nanoparticles accumulating in the heart; then, under the acidic pH of the injured myocardium, the EVs were released. This approach improved cardiac functions by circulating EVs. Concern about this method is that the toxicity of magnetic nanoparticles needs to be further studied; moreover, the magnetic force may be hard to reach the heart in obese patients.
Constant and fast blood flow also prevents EVs from accumulating in the heart. The strategy of encapsulating EVs in hydrogels prolongs EVs retention and uptake in the heart. In addition to in situ delivery, the special strength of hydrogel is the capacity to continuously release EVs. In this context, a variety of biomaterials can be used to produce functional hydrogels that reduce fibrosis, suppress inflammatory reactions, and promote cardiac function recovery (93, 105–109). Examples of EV hydrogel applications in MI treatment are shown in Table 2.
Laponite® is a synthetic smectite clay that is commercially available in pharmaceutics. By mixing Laponite ® with the gelatin solution, Waters et al. (108) designed a nanocomposite hydrogel that can provide prolonged retention of stem cell-derived secretome at the myocardial infarction site. Zhang et al. (106) incorporated dendritic cell-derived EVs with alginate hydrogel and found prolonged retention of EVs in vitro and in vivo, resulting in a significant improvement of cardiac function after MI in mice. Han et al. (105) incorporated cardiac protective peptides and matrix metalloprotease-2 (MMP-2) degradable sequence with a novel injectable self-assembled hydrogel, which was used to encapsulate EVs. Compared with EVs alone, this mixture of hydrogel showed better cardiac function with reduced inflammation, fibrosis, and apoptosis. Liu et al. (93) placed an engineered collagen-based hydrogel patch on the myocardial tissue, and the EVs were capable of slowly releasing at least for 7 days after in vivo implantation. In another study, Chen et al. (107) produced a shear-thinning hydrogel that could steadily release EVs for over 21 days. A notable study in this area comes from Firoozi et al. (109), and they found that adding extra cardioprotective bioactive peptides in hydrogel could not further improve the therapeutic outcome of EVs. However, this observation is worthy of further investigation.
Although hydrogel patches can provide sufficient storage of EVs, huge obstacles to further application still exist because of their strong dependence on intramyocardial injection. To avoid secondary tissue injury by intramyocardial injection, Tang et al. (110) demonstrated an injection-free delivery approach by spraying a mixture of EVs, gelatin methacryloyl precursors, and photoinitiators, followed by visible light irradiation to form a cardiac patch covering the surface of the heart. This approach results in an enhanced retention rate of EVs and improved therapeutic effects. However, as thoracotomy is inevitable in this method, it is an invasive treatment too.
Cardiovascular disease is a leading cause of death worldwide, and novel therapeutic strategies such as EVs demonstrate a promising future. Minimizing the invasive operation and improving the myocardial targeting delivery are the keys to successful clinical translation. Challenges in the efficient delivery of EVs to the heart can be mainly summarized into three aspects: First, naturally derived EVs showed low myocardial targeting efficiency; second, rapid uptake of EVs by non-targeted tissues; third, the compacted structure of myocardial tissue and constant fast blood flow in the heart prevents EVs uptake by the heart. To address these problems, four strategies have been reported so far: (1) modify EV surface to endow them with the property of targeting the heart. (2) Suppress the non-specific uptake of EVs and prolong the retention time of EVs in circulation. (3) Increase the uptake efficiency of cardiomyocytes. (4) Encapsulate EVs into hydrogels. The targeting effects and cardioprotective vary depending on the targeting strategy as well as the source and content of EVs. Therefore, it is challenging to determine which approach is better due to the lack of adequate controls, comparable doses, and dosing frequency. Albeit, each method demonstrated improved EV uptake and retention in the heart and led to improvements in therapeutic outcomes.
In future studies, combining these targeting strategies will be a feasible direction. For example, combining “passive targeting” with surface modification of myocardial homing peptides may dramatically boost the targeting effectiveness. Furthermore, it has been widely reported that exposure to ultrasonic irradiation could trigger a short-time, high-dose release of drug encapsulated in a hydrogel on demand (111–114). Thus, it is rational to assume that combining ultrasound stimulation and hydrogel may control the delivery timing of EVs to the heart and enhance their uptake efficiency. In another study, Wang et al. (115) fabricated a targeting peptide and ultrasound-responsive nanodrug in cancer therapy, and this approach is also worth trying in EV targeting. Finally, it should be noted that the strategies employed for EV heart targeting can also be applied in other fields such as tumor targeting (116) and brain targeting (117).
Extracellular vesicles are regarded as an effective therapeutic modality for the treatment of CVD, although current research outputs remain in their infancy and distant from clinical translation. A better understanding of the internalization and trafficking of EVs in vivo, as well as their biogenesis, heterogeneity, and interaction with receiving cells, is required to maximize their therapeutic effectiveness. Moreover, the targeting effect of EVs has only been confirmed in small laboratory animals, which is partly due to the limited output of EVs, and systematic non-human primate experiments are still required before translation into humans. Despite all these challenges, with the continuous development of targeting delivery strategies, this field demonstrates a promising future.
XY: conceptualization, investigation, and writing—original draft. L-HJ: conceptualization, funding acquisition, resources, supervision, writing—review and editing. Both authors contributed to the article and approved the submitted version.
The authors declare that the research was conducted in the absence of any commercial or financial relationships that could be construed as a potential conflict of interest.
All claims expressed in this article are solely those of the authors and do not necessarily represent those of their affiliated organizations, or those of the publisher, the editors and the reviewers. Any product that may be evaluated in this article, or claim that may be made by its manufacturer, is not guaranteed or endorsed by the publisher.
1. Gasior M, Gierlotka M, Pyka U, Zdrojewski T, Wojtyniak B, Chlebus K, et al. Temporal trends in secondary prevention in myocardial infarction patients discharged with left ventricular systolic dysfunction in Poland. Eur J Prev Cardiol. (2018) 25:960–9. doi: 10.1177/2047487318770830
2. Roth G, Johnson C, Abajobir A, Abd-Allah F, Abera S, Abyu G, et al. Global, regional, and national burden of cardiovascular diseases for 10 causes, 1990 to 2015. J Am Coll Cardiol. (2017) 70:1–25.
3. Roth G, Forouzanfar M, Moran A, Barber R, Nguyen G, Feigin V, et al. Demographic and epidemiologic drivers of global cardiovascular mortality. New Engl J Med. (2015) 372:1333–41. doi: 10.1056/NEJMoa1406656
4. GBD 2017 Disease and Injury Incidence and Prevalence Collaborators. Global, regional, and national incidence, prevalence, and years lived with disability for 354 diseases and injuries for 195 countries and territories, 1990-2017: a systematic analysis for the Global Burden of Disease Study 2017. Lancet (London, England). (2018) 392:1789–858.
5. O’Neill B, Rana S, Bowman V. An integrated approach for vascular health: A call to action. Can J Cardiol. (2015) 31:99–102. doi: 10.1016/j.cjca.2014.10.034
6. Mozaffarian D, Benjamin E, Go A, Arnett D, Circulation M. Heart disease and stroke statistics–2015 update: a report from the American Heart Association. Circulation. (2015) 131:e29–322.
7. Frangogiannis N. Pathophysiology of myocardial infarction. Compr Physiol. (2015) 5:1841–75. doi: 10.1002/cphy.c150006
8. Prabhu S, Frangogiannis N. The biological basis for cardiac repair after myocardial infarction: From inflammation to fibrosis. Circ Res. (2016) 119:91–112. doi: 10.1161/CIRCRESAHA.116.303577
9. Piek A, de Boer R, Silljé H. The fibrosis-cell death axis in heart failure. Heart Fail Rev. (2016) 21:199–211. doi: 10.1007/s10741-016-9536-9
10. Reed G, Rossi J, Cannon C. Acute myocardial infarction. Lancet. (2016) 389:197. doi: 10.1016/S0140-6736(16)30677-8
11. Fisher S, Brunskill S, Doree C, Mathur A, Taggart D, Martin-Rendon E. Stem cell therapy for chronic ischaemic heart disease and congestive heart failure. Cochrane Database Syst Rev. (2014) 12:CD007888. doi: 10.1002/14651858.CD007888.pub2
12. Packer M. The Alchemist’s Nightmare: Might mesenchymal stem cells that are recruited to repair the injured heart be transformed into fibroblasts rather than cardiomyocytes? Circulation. (2018) 137:2068–73. doi: 10.1161/CIRCULATIONAHA.117.032190
13. Yoon Y, Park J, Tkebuchava T, Luedeman C, Losordo D. Unexpected severe calcification after transplantation of bone marrow cells in acute myocardial infarction. Circulation. (2004) 109:3154–7. doi: 10.1161/01.CIR.0000134696.08436.65
14. Lima Correa B, El Harane N, Desgres M, Perotto M, Alayrac P, Guillas C, et al. Extracellular vesicles fail to trigger the generation of new cardiomyocytes in chronically infarcted hearts. Theranostics. (2021) 11:10114–24. doi: 10.7150/thno.62304
15. Wernly B, Mirna M, Rezar R, Prodinger C, Jung C, Podesser B, et al. Regenerative cardiovascular therapies: Stem cells and beyond. Int J Mol Sci. (2019) 20:1420. doi: 10.3390/ijms20061420
16. Théry C, Witwer K, Aikawa E, Alcaraz M, Anderson J, Andriantsitohaina R, et al. Minimal information for studies of extracellular vesicles 2018 (MISEV2018): a position statement of the International Society for Extracellular Vesicles and update of the MISEV2014 guidelines. J Extracell Vesicles. (2018) 7:1535750. doi: 10.1080/20013078.2018.1461450
17. Chuanjiang H, Shu Z, Yan L, Ben W. Exosome theranostics: Biology and translational medicine. Theranostics. (2018) 8:237–55. doi: 10.7150/thno.21945
18. Kuriyama N, Yoshioka Y, Kikuchi S, Okamura A, Azuma N, Ochiya T. Challenges for the development of extracellular vesicle-based nucleic acid medicines. Cancers (Basel). (2021) 13:6137. doi: 10.3390/cancers13236137
19. Buzas E. The roles of extracellular vesicles in the immune system. Nat Rev Immunol. (2022) 4:1–15. doi: 10.1038/s41577-022-00763-8
20. Yang D, Zhang W, Zhang H, Zhang F, Chen L, Ma L, et al. Progress, opportunity, and perspective on exosome isolation - efforts for efficient exosome-based theranostics. Theranostics. (2020) 10:3684–707. doi: 10.7150/thno.41580
21. Valadi H, Ekström K, Bossios A, Sjöstrand M, Lee J, Lötvall J. Exosome-mediated transfer of mRNAs and microRNAs is a novel mechanism of genetic exchange between cells. Nat Cell Biol. (2007) 9:654–9. doi: 10.1038/ncb1596
22. Abreu R, Fernandes H, Martins P, Sahoo S, Emanueli C, Ferreira L. Native and bioengineered extracellular vesicles for cardiovascular therapeutics. Nat Rev Cardiol. (2020) 17:685–97. doi: 10.1038/s41569-020-0389-5
23. Ikeda G, Santoso M, Tada Y, Li A, Vaskova E, Jung J, et al. Mitochondria-Rich extracellular vesicles from autologous stem cell-derived cardiomyocytes restore energetics of ischemic myocardium. J Am Coll Cardiol. (2021) 77:1073–88. doi: 10.1016/j.jacc.2020.12.060
24. Yue Y, Wang C, Benedict C, Huang G, Truongcao M, Roy R, et al. Interleukin-10 deficiency alters endothelial progenitor cell-derived exosome reparative effect on myocardial repair via integrin-linked kinase enrichment. Circ Res. (2020) 126:315–29. doi: 10.1161/CIRCRESAHA.119.315829
25. Gowen A, Shahjin F, Chand S, Odegaard K, Yelamanchili S. Mesenchymal stem cell-derived extracellular vesicles: Challenges in clinical applications. Front Cell Dev Biol. (2020) 8:149. doi: 10.3389/fcell.2020.00149
26. Yeo R, Lai R, Zhang B, Tan S, Yin Y, Teh B, et al. Mesenchymal stem cell: an efficient mass producer of exosomes for drug delivery. Adv Drug Deliv Rev. (2013) 65:336–41. doi: 10.1016/j.addr.2012.07.001
27. Natasha G, Gundogan B, Tan A, Farhatnia Y, Wu W, Rajadas J, et al. Exosomes as immunotheranostic nanoparticles. Clin Ther. (2014) 36:820–9. doi: 10.1016/j.clinthera.2014.04.019
28. Saleh A, Lázaro-Ibáñez E, Forsgard M, Shatnyeva O, Osteikoetxea X, Karlsson F, et al. Extracellular vesicles induce minimal hepatotoxicity and immunogenicity. Nanoscale. (2019) 11:6990–7001. doi: 10.1039/C8NR08720B
29. Zhu X, Badawi M, Pomeroy S, Sutaria D, Xie Z, Baek A, et al. Comprehensive toxicity and immunogenicity studies reveal minimal effects in mice following sustained dosing of extracellular vesicles derived from HEK293T cells. J Extracell Vesicles. (2017) 6:1324730. doi: 10.1080/20013078.2017.1324730
30. Yuana Y, Böing A, Grootemaat A, van der Pol E, Hau C, Cizmar P, et al. Handling and storage of human body fluids for analysis of extracellular vesicles. J Extracell Vesicles. (2015) 4:29260. doi: 10.3402/jev.v4.29260
31. Jin Y, Chen K, Wang Z, Wang Y, Liu J, Lin L, et al. DNA in serum extracellular vesicles is stable under different storage conditions. BMC Cancer. (2016) 16:753. doi: 10.1186/s12885-016-2783-2
32. Ge Q, Zhou Y, Lu J, Bai Y, Xie X, Lu Z. miRNA in plasma exosome is stable under different storage conditions. Molecules. (2014) 19:1568–75. doi: 10.3390/molecules19021568
33. Barreiro K, Dwivedi O, Valkonen S, Groop P, Tuomi T, Holthofer H, et al. Urinary extracellular vesicles: Assessment of pre-analytical variables and development of a quality control with focus on transcriptomic biomarker research. J Extracell Vesicles. (2021) 10:e12158. doi: 10.1002/jev2.12158
34. Bian S, Zhang L, Duan L, Wang X, Min Y, Yu H. Extracellular vesicles derived from human bone marrow mesenchymal stem cells promote angiogenesis in a rat myocardial infarction model. J Mol Med (Berlin, Germany). (2014) 92:387–97. doi: 10.1007/s00109-013-1110-5
35. Jiang X, Zhang T, Gao J. The in vivo fate and targeting engineering of crossover vesicle-based gene delivery system. Adv Drug Deliv Rev. (2022) 187:114324. doi: 10.1016/j.addr.2022.114324
36. Tenchov R, Sasso J, Wang X, Liaw W, Chen C, Zhou Q. Exosomes-Nature’s lipid nanoparticles, a rising star in drug delivery and diagnostics. ACS Nano. (2022) 16:17802–46. doi: 10.1021/acsnano.2c08774
37. Lai R, Yeo R, Tan K, Lim S. Exosomes for drug delivery - a novel application for the mesenchymal stem cell. Biotechnol Adv. (2013) 31:543–51. doi: 10.1016/j.biotechadv.2012.08.008
38. Shao L, Yu Z, Lan B, Wang J, Zhang Z, Zhang L, et al. MiRNA-Sequence indicates that mesenchymal stem cells and exosomes have similar mechanism to enhance cardiac repair. BioMed Res Int. (2017) 2017:4150705. doi: 10.1155/2017/4150705
39. Zhu J, Lu K, Zhang N, Zhao Y, Ma Q, Shen J, et al. Myocardial reparative functions of exosomes from mesenchymal stem cells are enhanced by hypoxia treatment of the cells via transferring microRNA-210 in an nSMase2-dependent way. Artif Cells Nanomed Biotechnol. (2017) 46:1659–70. doi: 10.1080/21691401.2017.1388249
40. Lucio B, Elisabetta C, Vincenzo L, Giuseppina M, Alessandra C, Vanessa B, et al. Cardioprotection by cardiac progenitor cell-secreted exosomes: Role of pregnancy-associated plasma protein-A. Cardiovasc Res. (2018) 114:992–1005. doi: 10.1093/cvr/cvy055
41. Peisen H, Li W, Qing L, Xiaqiu T, Yuejin Y. Atorvastatin enhances the therapeutic efficacy of mesenchymal stem cells derived exosomes in acute myocardial infarction via up-regulating long non-coding RNA H19. Cardiovasc Res. (2019) 116:353–67. doi: 10.1093/cvr/cvz139
42. Zhao J, Li X, Hu J, Chen F, Qiao S, Sun X, et al. Mesenchymal stromal cell-derived exosomes attenuate myocardial ischaemia-reperfusion injury through miR-182-regulated macrophage polarization. Cardiovasc Res. (2019) 115:1205–16. doi: 10.1093/cvr/cvz040
43. Hou Z, Qin X, Hu Y, Zhang X, Li G, Wu J, et al. Longterm exercise-derived exosomal mir-342-5p: A novel exerkine for cardioprotection. Circ Res. (2019) 124:1386–400. doi: 10.1161/CIRCRESAHA.118.314635
44. Khan M, Nickoloff E, Abramova T, Johnson J, Verma SK, Krishnamurthy P, et al. Embryonic stem cell-derived exosomes promote endogenous repair mechanisms and enhance cardiac function following myocardial infarction. Circ Res. (2015) 117:52–64. doi: 10.1161/CIRCRESAHA.117.305990
45. Cambier L, De Couto G, Ibrahim A, Echavez A, Valle J, Liu W, et al. Y RNA fragment in extracellular vesicles confers cardioprotection via modulation of IL expression and secretion. EMBO Mol Med. (2017) 9:337–52. doi: 10.15252/emmm.201606924
46. Yu B, Kim H, Gong M, Wang J, Millard R, Wang Y, et al. Exosomes secreted from GATA-4 overexpressing mesenchymal stem cells serve as a reservoir of anti-apoptotic microRNAs for cardioprotection. Int J Cardiol. (2015) 82:349–60. doi: 10.1016/j.ijcard.2014.12.043
47. Wang X, Zhao Y, Sun L, Shi Y, Li Z, Zhao X, et al. Exosomes derived from human umbilical cord mesenchymal stem cells improve myocardial repair via upregulation of Smad7. Int J Mol Med. (2018) 41:3063–72. doi: 10.3892/ijmm.2018.3496
48. Feng Y, Wei H, Mashhood W, Yu X, Muhammad A, Qin G. Ischemic preconditioning potentiates the protective effect of stem cells through secretion of exosomes by targeting Mecp2 via miR-22. PLoS One. (2014) 9:e88685.
49. Yu B, Min G, Wang Y, Millard R, Zeeshan P, Yang Y, et al. Cardiomyocyte protection by GATA-4 gene engineered mesenchymal stem cells is partially mediated by translocation of miR-221 in Microvesicles. PLoS One. (2013) 8:e73304. doi: 10.1371/journal.pone.0073304
50. Wang X, Gu H, Qin D, Yang L, Huang W, Essandoh K, et al. Exosomal miR-223 contributes to mesenchymal stem cell-elicited cardioprotection in polymicrobial sepsis. Sci Rep. (2015) 5:13721. doi: 10.1038/srep13721
51. Ma T, Chen Y, Chen Y, Meng Q, Sun J, Shao L, et al. MicroRNA-132, delivered by mesenchymal stem cell-derived exosomes, promote angiogenesis in myocardial infarction. Stem Cells Int. (2018) 2018:3290372. doi: 10.1155/2018/3290372
52. Wang K, Jiang Z, Webster K, Chen J, Hu H, Zhou Y, et al. Enhanced cardioprotection by human endometrium mesenchymal stem cells driven by exosomal MicroRNA-21. Stem Cells Transl Med. (2017) 6:209–22. doi: 10.5966/sctm.2015-0386
53. Gao L, Wang L, Wei Y, Krishnamurthy P, Walcott G, Menasché P, et al. Exosomes secreted by hiPSC-derived cardiac cells improve recovery from myocardial infarction in swine. Sci Transl Med. (2020) 12:eaay1318. doi: 10.1126/scitranslmed.aay1318
54. El Harane N, Kervadec A, Bellamy V, Pidial L, Neametalla H, Perier M, et al. Acellular therapeutic approach for heart failure: in vitro production of extracellular vesicles from human cardiovascular progenitors. Eur Heart J. (2018) 39:1835–47. doi: 10.1093/eurheartj/ehy012
55. Phinney D, Pittenger M. Concise Review: MSC-Derived Exosomes for Cell-Free Therapy. Stem Cells (Dayton, Ohio). (2017) 35:851–8. doi: 10.1002/stem.2575
56. Zhao Y, Sun X, Cao W, Ma J, Sun L, Qian H, et al. Exosomes derived from human umbilical cord mesenchymal stem cells relieve acute myocardial ischemic injury. Stem Cells Int. (2015) 2015:761643. doi: 10.1155/2015/761643
57. Gallet R, Dawkins J, Valle J, Simsolo E, de Couto G, Middleton R, et al. Exosomes secreted by cardiosphere-derived cells reduce scarring, attenuate adverse remodelling, and improve function in acute and chronic porcine myocardial infarction. Eur Heart J. (2017) 38:201–11. doi: 10.1093/eurheartj/ehw240
58. Feng Y, Huang W, Wani M, Yu X, Ashraf M. Ischemic preconditioning potentiates the protective effect of stem cells through secretion of exosomes by targeting Mecp2 via miR-22. PLoS One. (2014) 9:e88685.
59. Wei Z, Qiao S, Zhao J, Liu Y, Li Q, Wei Z, et al. miRNA-181a over-expression in mesenchymal stem cell-derived exosomes influenced inflammatory response after myocardial ischemia-reperfusion injury. Life Sci. (2019) 232:116632. doi: 10.1016/j.lfs.2019.116632
60. Peng Y, Zhao J, Peng Z, Xu W, Yu G. Exosomal miR-25-3p from mesenchymal stem cells alleviates myocardial infarction by targeting pro-apoptotic proteins and EZH2. Cell Death Dis. (2020) 11:317. doi: 10.1038/s41419-020-03025-4
61. Kang M, Jordan V, Blenkiron C, Chamley L. Biodistribution of extracellular vesicles following administration into animals: A systematic review. J Extracell Vesicles. (2021) 10:e12085. doi: 10.1002/jev2.12085
62. Gupta D, Liang X, Pavlova S, Wiklander O, Corso G, Zhao Y, et al. Quantification of extracellular vesicles in vitro and in vivo using sensitive bioluminescence imaging. J Extracell Vesicles. (2020) 9:1800222. doi: 10.1080/20013078.2020.1800222
63. Lai C, Mardini O, Ericsson M, Prabhakar S, Maguire C, Chen J, et al. Dynamic biodistribution of extracellular vesicles in vivo using a multimodal imaging reporter. ACS Nano. (2014) 8:483–94. doi: 10.1021/nn404945r
64. Wan Z, Zhao L, Lu F, Gao X, Dong Y, Zhao Y, et al. Mononuclear phagocyte system blockade improves therapeutic exosome delivery to the myocardium. Theranostics. (2020) 10:218–30. doi: 10.7150/thno.38198
65. Sun W, Li Z, Zhou X, Yang G, Yuan L. Efficient exosome delivery in refractory tissues assisted by ultrasound-targeted microbubble destruction. Drug Deliv. (2019) 26:45–50. doi: 10.1080/10717544.2018.1534898
66. Hausner E, Elmore S, Yang X. Overview of the components of cardiac metabolism. Drug Metab Disposit. (2019) 47:673–88. doi: 10.1124/dmd.119.086611
67. Firth J. Endothelial barriers: from hypothetical pores to membrane proteins. J Anatomy. (2002) 200:541–8. doi: 10.1046/j.1469-7580.2002.00059.x
68. Duan P, Tan J, Miao Y, Zhang Q. Potential role of exosomes in the pathophysiology, diagnosis, and treatment of hypoxic diseases. Am J Transl Res. (2019) 11:1184–201.
69. Caponnetto F, Manini I, Skrap M, Palmai-Pallag T, Di Loreto C, Beltrami A, et al. Size-dependent cellular uptake of exosomes. Nanomedicine. (2017) 13:1011–20. doi: 10.1016/j.nano.2016.12.009
70. Wiklander O, Nordin J, O’Loughlin A, Gustafsson Y, Corso G, Mäger I, et al. Extracellular vesicle in vivo biodistribution is determined by cell source, route of administration and targeting. J Extracell Vesicles. (2015) 4:26316. doi: 10.3402/jev.v4.26316
71. Mulcahy L, Pink R, Carter D. Routes and mechanisms of extracellular vesicle uptake. J Extracell Vesicles. (2014) 3:24641. doi: 10.3402/jev.v3.24641
72. Verdera H, Gitz-Francois J, Schiffelers R, Vader P. Cellular uptake of extracellular vesicles is mediated by clathrin-independent endocytosis and macropinocytosis. J Control Release. (2017) 266:100–8. doi: 10.1016/j.jconrel.2017.09.019
73. Hoshino A, Costa-Silva B, Shen T, Rodrigues G, Hashimoto A, Tesic Mark M, et al. Tumour exosome integrins determine organotropic metastasis. Nature. (2015) 527:329–35.
74. Rana S, Yue S, Stadel D, Zöller M. Toward tailored exosomes: the exosomal tetraspanin web contributes to target cell selection. Int J Biochem Cell Biol. (2012) 44:1574–84. doi: 10.1016/j.biocel.2012.06.018
75. De Celle T, Vanrobaeys F, Lijnen P, Blankesteijn W, Heeneman S, Van Beeumen J, et al. Alterations in mouse cardiac proteome after in vivo myocardial infarction: permanent ischaemia versus ischaemia-reperfusion. Exp Physiol. (2005) 90:593–606. doi: 10.1113/expphysiol.2005.030296
76. Kim N, Lee Y, Kim H, Joo H, Youm J, Park W, et al. Potential biomarkers for ischemic heart damage identified in mitochondrial proteins by comparative proteomics. Proteomics. (2006) 6:1237–49. doi: 10.1002/pmic.200500291
77. Kanki S, Jaalouk D, Lee S, Yu A, Gannon J, Lee R. Identification of targeting peptides for ischemic myocardium by in vivo phage display. J Mol Cell Cardiol. (2011) 50:841–8. doi: 10.1016/j.yjmcc.2011.02.003
78. Zhu L, Tian T, Wang J, He J, Chen T, Pan M, et al. Hypoxia-elicited mesenchymal stem cell-derived exosomes facilitates cardiac repair through miR-125b-mediated prevention of cell death in myocardial infarction. Theranostics. (2018) 8:6163–77. doi: 10.7150/thno.28021
79. Vandergriff A, Huang K, Shen D, Hu S, Hensley M, Caranasos T, et al. Targeting regenerative exosomes to myocardial infarction using cardiac homing peptide. Theranostics. (2018) 8:1869–78. doi: 10.7150/thno.20524
80. Antes T, Middleton R, Luther K, Ijichi T, Peck K, Liu W, et al. Targeting extracellular vesicles to injured tissue using membrane cloaking and surface display. J Nanobiotechnol. (2018) 16:61. doi: 10.1186/s12951-018-0388-4
81. Wang H, Maimaitiaili R, Yao J, Xie Y, Qiang S, Hu F, et al. Percutaneous intracoronary delivery of plasma extracellular vesicles protects the myocardium against ischemia-reperfusion injury in canis. Hypertension. (2021) 78:1541–54. doi: 10.1161/HYPERTENSIONAHA.121.17574
82. Wang X, Chen Y, Zhao Z, Meng Q, Yu Y, Sun J, et al. Engineered exosomes with ischemic myocardium-targeting peptide for targeted therapy in myocardial infarction. J Am Heart Assoc. (2018) 7:e008737. doi: 10.1161/JAHA.118.008737
83. Kim H, Yun N, Mun D, Kang J, Lee S, Park H, et al. Cardiac-specific delivery by cardiac tissue-targeting peptide-expressing exosomes. Biochem Biophys Res Commun. (2018) 499:803–8. doi: 10.1016/j.bbrc.2018.03.227
84. Mentkowski K, Lang J. Exosomes engineered to express a cardiomyocyte binding peptide demonstrate improved cardiac retention in vivo. Sci Rep. (2019) 9:10041. doi: 10.1038/s41598-019-46407-1
85. Hu S, Wang X, Li Z, Zhu D, Cores J, Wang Z, et al. Platelet membrane and stem cell exosome hybrid enhances cellular uptake and targeting to heart injury. Nano Today. (2021) 39:101210. doi: 10.1016/j.nantod.2021.101210
86. Zhang N, Song Y, Huang Z, Chen J, Tan H, Yang H, et al. Monocyte mimics improve mesenchymal stem cell-derived extracellular vesicle homing in a mouse MI/RI model. Biomaterials. (2020) 255:120168. doi: 10.1016/j.biomaterials.2020.120168
87. Witwer K, Wolfram J. Extracellular vesicles versus synthetic nanoparticles for drug delivery. Nat Rev Mater. (2021) 6:103–6. doi: 10.1038/s41578-020-00277-6
88. Liu X, Yang X, Sun W, Wu Q, Song Y, Yuan L, et al. Systematic evolution of ligands by exosome enrichment: A proof-of-concept study for exosome-based targeting peptide screening. Adv Biosyst. (2019) 3:e1800275. doi: 10.1002/adbi.201800275
89. Salunkhe S, Dheeraj, Basak M, Chitkara D, Mittal A. Surface functionalization of exosomes for target-specific delivery and in vivo imaging & tracking: Strategies and significance. J Control Release. (2020) 326:599–614. doi: 10.1016/j.jconrel.2020.07.042
90. Lee J, Lee H, Goh U, Kim J, Jeong M, Lee J, et al. Cellular engineering with membrane fusogenic liposomes to produce functionalized extracellular vesicles. ACS Appl Mater Interfaces. (2016) 11:6790–5. doi: 10.1021/acsami.6b01315
91. Armstrong J, Holme M, Stevens M. Re-Engineering extracellular vesicles as smart nanoscale therapeutics. ACS Nano. (2017) 11:69–83. doi: 10.1021/acsnano.6b07607
92. Hung M, Leonard J. Stabilization of exosome-targeting peptides via engineered glycosylation. J Biol Chem. (2015) 290:8166–72. doi: 10.1074/jbc.M114.621383
93. Liu B, Lee B, Nakanishi K, Villasante A, Williamson R, Metz J, et al. Cardiac recovery via extended cell-free delivery of extracellular vesicles secreted by cardiomyocytes derived from induced pluripotent stem cells. Nat Biomed Eng. (2018) 2:293–303. doi: 10.1038/s41551-018-0229-7
94. Romain G, James D, Jackelyn V, Eli S, Geoffrey D, Ryan M, et al. Exosomes secreted by cardiosphere-derived cells reduce scarring, attenuate adverse remodelling, and improve function in acute and chronic porcine myocardial infarction. Eur Heart J. (2016) 38:201–11.
95. Wei Z, Chen Z, Zhao Y, Fan F, Xiong W, Song S, et al. Mononuclear phagocyte system blockade using extracellular vesicles modified with CD47 on membrane surface for myocardial infarction reperfusion injury treatment. Biomaterials. (2021) 275:121000. doi: 10.1016/j.biomaterials.2021.121000
96. Watson D, Bayik D, Srivatsan A, Bergamaschi C, Valentin A, Niu G, et al. Efficient production and enhanced tumor delivery of engineered extracellular vesicles. Biomaterials. (2016) 105:195–205. doi: 10.1016/j.biomaterials.2016.07.003
97. Escrevente C, Keller S, Altevogt P, Costa J. Interaction and uptake of exosomes by ovarian cancer cells. BMC Cancer. (2011) 11:108. doi: 10.1186/1471-2407-11-108
98. Simons M, Raposo G. Exosomes–vesicular carriers for intercellular communication. Curr Opin Cell Biol. (2009) 21:575–81. doi: 10.1016/j.ceb.2009.03.007
99. Feng D, Zhao W, Ye Y, Bai X, Liu R, Chang L, et al. Cellular internalization of exosomes occurs through phagocytosis. Traffic (Copenhagen, Denmark). (2010) 11:675–87. doi: 10.1111/j.1600-0854.2010.01041.x
100. Jin L, Li F, Wang H, Wei F, Qin P, Du L. Ultrasound targeted microbubble destruction stimulates cellular endocytosis in facilitation of adeno-associated virus delivery. Int J Mol Sci. (2013) 14:9737–50. doi: 10.3390/ijms14059737
101. Kooiman K, Vos H, Versluis M, Jong N. Acoustic behavior of microbubbles and implications for drug delivery. Adv Drug Deliv Rev. (2014) 72:28–48. doi: 10.1016/j.addr.2014.03.003
102. Sutton J, Haworth K, Pyne-Geithman G, Holland C. Ultrasound-mediated drug delivery for cardiovascular disease. Exp Opin Drug Deliv. (2013) 10:573–92. doi: 10.1517/17425247.2013.772578
103. Sun W, Zhao P, Zhou Y, Xing C, Zhao L, Li Z, et al. Ultrasound targeted microbubble destruction assisted exosomal delivery of miR-21 protects the heart from chemotherapy associated cardiotoxicity. Biochem Biophys Res Commun. (2020) 532:60–7. doi: 10.1016/j.bbrc.2020.05.044
104. Liu S, Chen X, Bao L, Liu T, Yuan P, Yang X, et al. Treatment of infarcted heart tissue via the capture and local delivery of circulating exosomes through antibody-conjugated magnetic nanoparticles. Nat Biomed Eng. (2020) 4:1063–75. doi: 10.1038/s41551-020-00637-1
105. Han C, Zhou J, Liang C, Liu B, Pan X, Zhang Y, et al. Human umbilical cord mesenchymal stem cell derived exosomes encapsulated in functional peptide hydrogels promote cardiac repair. Biomater Sci. (2019) 7:2920–33. doi: 10.1039/C9BM00101H
106. Zhang Y, Cai Z, Shen Y, Lu Q, Gao W, Zhong X, et al. Hydrogel-load exosomes derived from dendritic cells improve cardiac function via Treg cells and the polarization of macrophages following myocardial infarction. J Nanobiotechnol. (2021) 19:271. doi: 10.1186/s12951-021-01016-x
107. Chen C, Wang L, Zaman S, Gordon J, Arisi M, Venkataraman C, et al. Sustained release of endothelial progenitor cell-derived extracellular vesicles from shear-thinning hydrogels improves angiogenesis and promotes function after myocardial infarction. Cardiovasc Res. (2018) 114:1029–40. doi: 10.1093/cvr/cvy067
108. Waters R, Alam P, Pacelli S, Chakravarti A, Ahmed R, Paul A. Stem cell-inspired secretome-rich injectable hydrogel to repair injured cardiac tissue. Acta Biomater. (2018) 69:95–106. doi: 10.1016/j.actbio.2017.12.025
109. Firoozi S, Pahlavan S, Ghanian M, Rabbani S, Barekat M, Nazari A, et al. Mesenchymal stem cell-derived extracellular vesicles alone or in conjunction with a SDKP-conjugated self-assembling peptide improve a rat model of myocardial infarction. Biochem Biophys Res Commun. (2020) 524:903–9. doi: 10.1016/j.bbrc.2020.02.009
110. Tang J, Cui X, Zhang Z, Xu Y, Guo J, Soliman B, et al. Injection-Free delivery of msc-derived extracellular vesicles for myocardial infarction therapeutics. Adv Healthcare Mater. (2021) 11:e2100312. doi: 10.1002/adhm.202100312
111. Huebsch N, Kearney C, Zhao X, Kim J, Cezar C, Suo Z, et al. Ultrasound-triggered disruption and self-healing of reversibly cross-linked hydrogels for drug delivery and enhanced chemotherapy. Proc Natl Acad Sci USA. (2014) 111:9762–7. doi: 10.1073/pnas.1405469111
112. Moncion A, Lin M, Kripfgans O, Franceschi R, Putnam A, Fabiilli M. Sequential payload release from acoustically-responsive scaffolds using focused ultrasound. Ultrasound Med Biol. (2018) 44:2323–35. doi: 10.1016/j.ultrasmedbio.2018.06.011
113. Lu X, Dong X, Natla S, Kripfgans O, Fowlkes J, Wang X, et al. Parametric study of acoustic droplet vaporization thresholds and payload release from acoustically-responsive scaffolds. Ultrasound Med Biol. (2019) 45:2471–84. doi: 10.1016/j.ultrasmedbio.2019.05.024
114. Wu C, Sun M, Kung Y, Wang Y, Chen S, Shen H, et al. One injection for one-week controlled release: In vitro and in vivo assessment of ultrasound-triggered drug release from injectable thermoresponsive biocompatible hydrogels. Ultrason Sonochem. (2020) 62:104875. doi: 10.1016/j.ultsonch.2019.104875
115. Wang Z, He Q, Zhao W, Luo J, Gao W. Tumor-homing, pH- and ultrasound-responsive polypeptide-doxorubicin nanoconjugates overcome doxorubicin resistance in cancer therapy. J Control Release. (2017) 264:66–75. doi: 10.1016/j.jconrel.2017.08.017
116. Wang J, Zheng Y, Zhao M. Exosome-Based cancer therapy: Implication for targeting cancer stem cells. Front Pharmacol. (2016) 7:533. doi: 10.3389/fphar.2016.00533
Keywords: extracellular vesicles, cardiovascular diseases, target delivery, myocardial infarction, exosome (vesicle)
Citation: Yin X and Jiang L-H (2023) Extracellular vesicles: Targeting the heart. Front. Cardiovasc. Med. 9:1041481. doi: 10.3389/fcvm.2022.1041481
Received: 11 September 2022; Accepted: 16 December 2022;
Published: 10 January 2023.
Edited by:
Tobias Eckle, University of Colorado Anschutz Medical Campus, United StatesReviewed by:
John Pearce Morrow, Columbia University, United StatesCopyright © 2023 Yin and Jiang. This is an open-access article distributed under the terms of the Creative Commons Attribution License (CC BY). The use, distribution or reproduction in other forums is permitted, provided the original author(s) and the copyright owner(s) are credited and that the original publication in this journal is cited, in accordance with accepted academic practice. No use, distribution or reproduction is permitted which does not comply with these terms.
*Correspondence: Li-Hong Jiang, ZG9jdG9ya3lsZUAxMjYuY29t
Disclaimer: All claims expressed in this article are solely those of the authors and do not necessarily represent those of their affiliated organizations, or those of the publisher, the editors and the reviewers. Any product that may be evaluated in this article or claim that may be made by its manufacturer is not guaranteed or endorsed by the publisher.
Research integrity at Frontiers
Learn more about the work of our research integrity team to safeguard the quality of each article we publish.