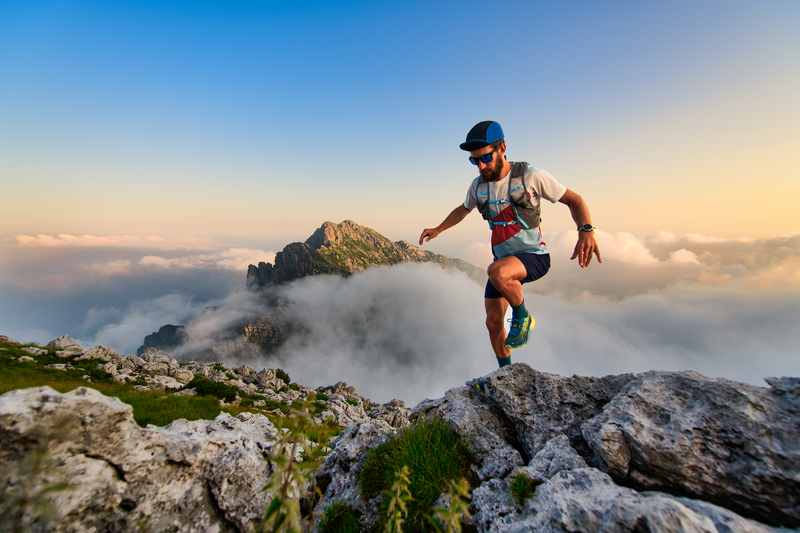
95% of researchers rate our articles as excellent or good
Learn more about the work of our research integrity team to safeguard the quality of each article we publish.
Find out more
ORIGINAL RESEARCH article
Front. Cardiovasc. Med. , 24 October 2022
Sec. Cardiovascular Genetics and Systems Medicine
Volume 9 - 2022 | https://doi.org/10.3389/fcvm.2022.1012615
Background: The causal direction and magnitude of the associations between telomere length (TL) and cardiovascular diseases (CVDs) remain uncertain due to susceptibility of reverse causation and confounding. This study aimed to investigate the associations between TL and CVDs using Mendelian randomization (MR).
Materials and methods: In this two-sample MR study, we identified 154 independent TL-associated genetic variants from a genome-wide association study (GWAS) consisting of 472,174 individuals (aged 40–69) in the UK Biobank. Summary level data of CVDs were obtained from different GWASs datasets. Methods of inverse variance weighted (IVW), Mendelian Randomization-Egger (MR-Egger), Mendelian Randomization robust adjusted profile score (MR-RAPS), maximum likelihood estimation, weighted mode, penalized weighted mode methods, and Mendelian randomization pleiotropy residual sum and outlier test (MR-PRESSO) were conducted to investigate the associations between TL and CVDs.
Results: Our findings indicated that longer TL was significantly associated with decreased risk of coronary atherosclerosis [odds ratio (OR), 0.85; 95% confidence interval (CI), 0.75–0.95; P = 4.36E-03], myocardial infarction (OR, 0.72; 95% CI, 0.63–0.83; P = 2.31E-06), ischemic heart disease (OR, 0.87; 95% CI, 0.78–0.97; P = 1.01E-02), stroke (OR, 0.87; 95% CI, 0.79–0.95; P = 1.60E-03), but an increased risk of hypertension (OR, 1.12; 95% CI, 1.02–1.23; P = 2.00E-02). However, there was no significant association between TL and heart failure (OR, 0.94; 95% CI, 0.87–1.01; P = 1.10E-01), atrial fibrillation (OR, 1.01; 95% CI, 0.93–1.11; P = 7.50E-01), or cardiac death (OR, 0.95; 95% CI, 0.82–1.10; P = 4.80E-01). Both raw and outlier corrected estimates from MR-PRESSO were consistent with those of IVW results. The sensitivity analyses showed no evidence of pleiotropy (MR-Egger intercept, P > 0.05), while Cochran’s Q test and MR-Egger suggested different degrees of heterogeneity.
Conclusion: Our MR study suggested that longer telomeres were associated with decreased risk of several CVDs, including coronary atherosclerosis, myocardial infarction, ischemic heart disease, and stroke, as well as an increased risk of hypertension. Future studies are still warranted to validate the results and investigate the mechanisms underlying these associations.
Telomeres are DNA-protein complexes composed of deoxyribonucleic acid repeats and are located at the termini of linear chromosomes. Telomeres protect the genes from damage and are thus important for chromosomal stability and cellular integrity (1). Telomere length (TL) has long been regarded as a reliable biomarker for cellular aging due to its progressive shortening at each cell cycle division (2–4). At a population level, TL is often measured in blood leukocytes, which has been suggested highly correlated with TL across different tissues (5). Previous studies have shown that TL is largely genetically determined (6, 7). The shortening of telomeres can induce DNA damage and provoke apoptosis and cell senescence, which may contribute to many aging-associated diseases (8, 9). Epidemiological studies have shown that telomere shortening is related to an increased risk of diabetes (10), cancer (11), and non-vascular, non-neoplastic causes of mortality (12).
Cardiovascular diseases (CVDs) are defined as disorders of the heart and blood vessels that include hypertension, coronary heart disease, myocardial infarction, ischemic heart disease, atrial fibrillation, heart failure, and stroke (13). With the aging of the global population, the socioeconomic burden brought about by CVDs continues to increase every year. Globally, CVDs are continuing to be the leading cause of death in 2019, causing an estimated 17.9 million deaths each year (13). Telomeres have been suggested to play an important role in the development and prognosis of CVDs, which has drawn considerable research interest in recent years (14–17). A multicenter, community-based cohort study from the UK Biobank showed that reduced leukocyte TL was associated with increased cardiovascular mortality (18). Meanwhile, a meta-analysis involving 43,725 individuals indicated a negative correlation between TL and coronary heart disease, but no significant association with cerebrovascular disease was found (19). However, a recent study suggested that TL was not associated with cardiovascular mortality (20). Therefore, it still remains unclear whether TL is causally involved in the development of CVDs, as the results have been varied and inconsistent across observational studies mainly due to confounding, and reverse causation. A clear understanding of the relationship between TL and the risk of CVDs is essential to the prevention and treatment of CVDs among the elderly population.
Mendelian randomization (MR) is a robust method of establishing a causal association between an exposure and an outcome using genetic variants related to the exposure of interest (21). These genetic variants are randomly inherited from parents and are thus not affected by confounders of the exposure-outcome association. Compared to traditional epidemiological approaches, MR is better at establishing causal relationships between the exposure and outcome variables with much less confounding and biases and has gained increasing popularity in research. In recent years, MR study has been widely used to assess causality in epidemiologic settings. In the present study, we conducted the largest two-sample MR study to assess the possible associations between TL and the risk of CVDs using the latest genome-wide association study (GWAS) summary data.
This is a two-sample MR study design based on the data from different large-scale GWAS datasets. The study design overview of the MR analysis is presented in Figure 1. The potential genetic variants selected to estimate the effects must comply with three key assumptions (22): (1) the genetic variants should be associated with TL (P < 5 × 10–8); (2) the genetic variants must not be associated with the outcome and confounding factors of the exposure-outcome association; (3) the genetic variants must be associated with CVDs only through TL. All contributing studies have received ethical approval from their respective medical ethical committees and obtained informed consent from all study participants.
Figure 1. Assumptions of the Mendelian randomization (MR) analysis for TL and the risk of CVDs. The MR study assumes that genetic variants are associated with only TL and not with confounders or alternative causal pathways, that is, the IVs affect the risk of CVDs only directly through TL. TL, telomere length; CVDs, cardiovascular diseases; IVs, instrument variables.
Genetic variants associated with TL were retrieved from a hitherto largest GWAS (Dataset ID: ieu-b-4879) including 472,174 individuals (aged 40–69) in the UK Biobank, with a similar proportion of males (45.8%) and females (54.2%) (23). The self-reported ethnicity was predominantly White European (94.3% White, 1.9% Asian, 1.5% Black, 0.3% Chinese, 0.6% Mixed, and 0.9% other ethnicities) (23). Age, sex, and ethnic group were adjusted in this study. In the UK Biobank cohort, DNA was extracted from the peripheral blood leukocytes, and TL was measured as the T/S ratio using the quantitative polymerase chain reaction methodology (24). We selected only independent (R2 < 0.001) and genome-wide significant (P < 5 × 10–8) single nucleotide polymorphisms (SNPs) from this GWAS as the instrumental variables (IVs) for TL, which resulted in 154 SNPs (Supplementary Table 1). The strength of each genetic instrument was measured using F-statistics (F = R2/(1-R2) × [(N-K-1)/K], where R2 is the proportion of the exposure explained by the genetic instrument, N is the sample size, and K is the number of SNPs) (25). The PhenoScanner database V2 was used to assess and remove those SNPs that are associated with other phenotypes, including the potential confounders and mediators (i.e., diabetes, hyperlipidemia, hypertension, smoking, and body mass index) (26, 27). We also removed SNPs for palindromic or incompatible alleles when harmonizing TL and outcomes. In the present study, 130, 128, 128, 128, 119, 116, 130, and 138 SNPs were eventually obtained as the IVs for TL to assess the associations between TL and hypertension, myocardial infarction, coronary atherosclerosis, ischemic heart disease, heart failure, stroke, atrial fibrillation, and cardiac death, respectively.
Summary-level data for CVDs were obtained from recently published GWAS datasets. The GWAS summary data of hypertension (55,917 cases and 162,837 controls), coronary atherosclerosis (23,363 cases and 187,840 controls), ischemic heart disease (31,640 cases and 187,152 controls), myocardial infarction (11,622 cases and 187,840 controls), and cardiac death (7,563 cases and 211,229 controls) were from the FinnGen consortium. The heart failure GWAS dataset (47,309 cases and 930,014 controls) was from the Heart Failure Molecular Epidemiology for Therapeutic Targets (HERMES) consortium. The atrial fibrillation (60,620 cases and 970,216 controls) data was from a GWAS meta-analysis of The Nord-Trøndelag Health Study (HUNT), the Michigan Genomics Initiative (MGI), deCODE, UK Biobank, DiscovEHR, and the AFGen Consortium (28). The GWAS data of stroke (40,585 cases and 406,111 controls) was also from different consortiums (MEGASTROKE, etc.) (29). The participants included in this study were mostly of European ancestry. All datasets included in the current study are summarized in Table 1.
The IVW method was used as the principal MR analytic approach to assess the associations between TL and the risk of CVDs. All statistical analyses were performed using R software (version 4.1.2). Analyses were performed using TwoSampleMR R package (30), which included inverse variance weighted (IVW), Mendelian Randomization-Egger (MR-Egger), and weighted mode. A P-value less than 0.05 (two-sided) was considered statistically significant. We used the IVW (Q) and MR-Egger methods to test for heterogeneity, the MR-Egger regression (MR-Egger intercept test) to evaluate the horizontal pleiotropy (statistical significance was set at a level of P < 0.05), and the leave-one-out analysis to exclude the possible influence of individual SNPs on the overall results. For sensitivity analyses, P < 0.05 in Cochran’s Q test suggested significant heterogeneity. The MR-IVW fixed-effects model was adopted for SNPs without heterogeneity (P > 0.05 in Cochran’s Q test), while the MR-IVW random-effects model was used for heterogeneous SNPs. We further performed the robust adjusted profile score (MR-RAPS), maximum likelihood estimation, and penalized weighted median (PWM) method to assess the effects of TL on the risk of CVDs (31–33). Additionally, we used the Mendelian randomization pleiotropy residual sum and outlier (MR-PRESSO) test to identify and remove the horizontal pleiotropic outliers (34).
In our MR study, 154 independent leukocyte TL-associated SNPs were obtained as IVs (Supplementary Table 1). The F-statistics of all these genetic variants were above the threshold of 10 (range: 29.86–1628.82), indicating that our IVs were strongly predictive of TL. The SNPs that were associated with confounding factors and SNPs for palindromic or incompatible alleles were removed.
Six different methods of MR analyses, i.e., the IVW method, MR-RAPS, MR-Egger, PWM, weighted mode, and maximum likelihood, were implemented. The IVW method was used as the principal MR analytic approach. Using the IVW method, we found that genetically increased TL was significantly associated with lower odds of coronary atherosclerosis [IVW: odds ratio (OR), 0.85; 95% confidence interval (CI), 0.75–0.95; P = 4.36E-03], myocardial infarction (IVW: OR, 0.72; 95% CI, 0.63–0.83; P = 2.31E-06), ischemic heart disease (IVW: OR, 0.87; 95% CI, 0.78–0.97; P = 1.01E-02), and stroke (IVW: OR, 0.87; 95% CI, 0.79–0.95; P = 1.60E-03), but higher odds of hypertension (IVW: OR, 1.12; 95% CI, 1.02–1.23; P = 2.00E-02). However, we did not find enough evidence for the relationships between TL and heart failure (IVW: OR, 0.94; 95% CI, 0.87–1.01; P = 1.10E-01), atrial fibrillation (IVW: OR, 1.01; 95% CI, 0.93–1.11; P = 7.50E-01), and cardiac death (IVW: OR, 0.95; 95% CI, 0.82–1.10; P = 4.80E-01). The two-sample MR estimates for the associations between TL and the risk of CVDs were presented in Figure 2. The scatter plots for the MR TL-to-CVDs association were presented in Figures 3, 4.
Figure 2. Associations of genetically predicted telomere length (TL) and the risk of cardiovascular diseases (CVDs). SNPs, single nucleotide polymorphisms; IVW, inverse variance weighted (random-effects model); IVW*, inverse variance weighted (fixed-effects model); MR-RAPS, Mendelian randomization robust adjusted profile score; MR-Egger, Mendelian randomization-Egger; PWM, penalized weighted median; OR, odds ratio; CI, confidence interval.
Figure 3. Scatter plots for Mendelian randomization (MR) analyses of the correlation between telomere length (TL) and cardiovascular diseases (CVDs). (A) TL-hypertension; (B) TL-myocardial infarction; (C) TL-coronary atherosclerosis; (D) TL-ischemic heart disease. The slope of each line corresponds to the estimated association effect between TL and the risk of CVDs of different MR methods.
Figure 4. Scatter plots for Mendelian randomization (MR) analyses of the correlation between telomere length (TL) and cardiovascular diseases (CVDs). (A) TL-heart failure; (B) TL-atrial fibrillation; (C) TL-stroke; (D) TL-cardiac death. The slope of each line corresponds to the estimated association effect between TL and the risk of CVDs of different MR methods.
The MR-PRESSO test identified one outlier SNP for ischemic heart disease (rs429358, Rssobs = 5.08E-03, P < 0.128), five outlier SNPs for atrial fibrillation (rs12369950, Rssobs = 2.05E-03, P < 0.13; rs2306646, Rssobs = 8,89E-04, P < 0.13; rs4743037, Rssobs = 1.06E-03, P < 0.13; rs6584579, Rssobs = 1.11E-03, P < 0.13; rs6751209, Rssobs = 7.48E-04, P < 0.13), and one outlier SNP for stroke (rs6584579, Rssobs = 1.69E-03, P < 0.116). No outlier SNPs were identified for hypertension, myocardial infarction, coronary atherosclerosis, heart failure, and cardiac death. Both raw and outlier corrected estimates from MR-PRESSO were consistent with the IVW results (Table 2). After removing the outlier SNPs, the IVW analysis showed basically the same results (Supplementary Table 2).
Table 2. The Mendelian randomization pleiotropy residual sum and outlier (MR-PRESSO) tests of the relationship between telomere length (TL) and cardiovascular diseases (CVDs).
The IVW, MR-Egger, and MR-Egger intercept tests were performed as sensitivity analyses to detect the potential heterogeneity and horizontal pleiotropy (Table 3). According to the sensitivity analyses, the intercept test for MR-egger suggested no pleiotropy; however, Cochran’s Q test and MR-Egger test suggested different degrees of heterogeneity (Table 3). The leave-one-out sensitivity analysis was presented in Supplementary Figures 1–8, which validated the stability of the results.
Table 3. Heterogeneity and horizontal pleiotropy of the associations between telomere length (TL) and cardiovascular diseases (CVDs).
In our study, we found that TL was associated with the risk of certain kinds of CVDs. To be specific, longer TL predicted a lower risk of coronary atherosclerosis, myocardial infarction, ischemic heart disease, and stroke, but a higher risk of hypertension. However, we did not find enough evidence to support the associations of TL with heart failure, atrial fibrillation, and cardiac death.
The findings that longer TL was associated with decreased risk of coronary atherosclerosis, ischemic heart disease, myocardial infarction, and stroke were consistent with previous studies. For instance, a recent meta-analysis based on 18 studies (involving 14,491 individuals) showed that the TL in patients with coronary artery disease was significantly shorter than that in the controls (standard mean difference = –0.45; 95% CI, –0.65–0.25) (35). A meta-analysis involving 32 studies (44,610 participants) reported that the shortest TL was related to a higher risk of myocardial infarction (risk ratio, 1.39; 95% CI, 1.16–1.67) (36). A cohort study with 29 years of follow-up reported that the lowest TL was modestly related to an increased risk of ischemic heart disease (hazard ratio, 1.55; 95% CI, 1.02–2.35). A meta-analysis based on 11 studies (25,340 individuals) indicated a significant association between shortened TL and stroke (OR: 1.5; 95% CI, 1.13–2.00) (37). All these findings supported the protective roles of increased TL in certain CVDs and suggest that special attention should be paid to those with shortened TL and certain measures to be taken to prevent the occurrence of CVDs in the future.
Of note, we found that longer TL was related to an increased risk of hypertension, which was opposite to the findings regarding coronary atherosclerosis, ischemic heart disease, myocardial infarction, and stroke. Previous studies have reached inconsistent conclusions on the correlation between TL and hypertension. For instance, some previous studies suggested that there was no association between TL and blood pressure (38–40). A previous meta-analysis of 10 studies indicated a significant negative correlation between TL and hypertension (41), which was also observed in recent studies (42–45). However, it has also been reported that longer TL is associated with higher blood pressure (46, 47). Meanwhile, several studies have found a non-linear correlation between TL and blood pressure (48–50). These findings suggest inconclusive and contradictory associations between TL length and hypertension, which warrant further research. Additionally, although our MR study did not find evidence to support the associations between TL and heart failure or cardiac death, the ORs for these associations were both less than 1.0, suggesting that TL might be a potential risk factor for heart failure and cardiac death. Previous studies have shown inconsistent results in the associations of TL with heart failure, atrial fibrillation, and cardiac death (14, 51–57), indicating the need for further research.
The mechanism of the associations between TL and CVDs remains elusive. Previous studies have reported that accelerated telomere shortening was associated with oxidative stress and chronic inflammation, both were critical factors that contribute to CVDs (58). Telomere shortening might also induce endothelial cell senescence and thereby promote human atherogenesis (59, 60). In addition, accelerated telomere shorting has been reported to be related to bone marrow-derived endothelial injury, which was important for the re-endothelialization of damaged vessels (61). It has also been suggested that TL was related to the development of type 2 diabetes, a common risk factor for CVDs (62). Cellular senescence in arteries induced by telomere dysfunction might contribute to the pathogenesis of hypertension (39). Besides, multiple social, environmental, and psychological factors could also affect these relationships. For example, it has been found that lack of physical activity, smoking, drinking, and high body mass index was negatively associated with TL, while regular exercise and a high-fiber diet were positively associated with the length of telomeres (63).
Several strengths of this MR study should be noted. First, we excluded the genetic variants that were related to potential confounders commonly found in epidemiological studies and selected only the SNPs strongly associated with TL. Second, the large sample size of our MR analysis enhanced our statistical power and provided reliable evidence of associations. Besides, we used the MR-PRESSO test to identify and remove variants that were horizontal pleiotropic outliers. Finally, we performed several sensitivity analyses, such as IVW, MR-Egger, and leave-one-out analysis, to confirm the robustness of these findings. Despite the strengths, there are some limitations to this study. First, our summary-level MR analysis assumed a linear relationship between TL and CVDs, which might not be the fact. Second, selection bias, such as the potential “healthy volunteer” bias and low recruitment rate, and bias from sample overlap could not be completely avoided. Third, different degrees of heterogeneity were observed in the MR-Egger and IVW (Q) methods, suggesting that our findings might be affected by pleiotropy. Furthermore, the participants in this study were mostly of European ancestry, which minimized the population stratification bias but limited the generalizability of our findings to other populations.
Our MR study provided strong evidence of the relationships between TL shortening and increased risk of coronary atherosclerosis, myocardial infarction, ischemic heart disease, and stroke, as well as decreased risk of hypertension. We did not find the casual associations of TL with heart failure, atrial fibrillation, and cardiac death. Future studies are still warranted to validate the results and investigate the mechanisms underlying these associations.
The original contributions presented in this study are included in the article/Supplementary material, further inquiries can be directed to the corresponding author.
YD and QL designed the study and analyzed and interpreted the data. YD drafted the manuscript. FZ, GL, JHL, JLL, and LL provided feedback on earlier drafts of the manuscript. DC reviewed and edited the manuscript. All authors contributed to the article and approved the submitted version.
The authors declare that the research was conducted in the absence of any commercial or financial relationships that could be construed as a potential conflict of interest.
All claims expressed in this article are solely those of the authors and do not necessarily represent those of their affiliated organizations, or those of the publisher, the editors and the reviewers. Any product that may be evaluated in this article, or claim that may be made by its manufacturer, is not guaranteed or endorsed by the publisher.
The Supplementary Material for this article can be found online at: https://www.frontiersin.org/articles/10.3389/fcvm.2022.1012615/full#supplementary-material
1. Blackburn EH. Switching and signaling at the telomere. Cell. (2001) 106:661–73. doi: 10.1016/S0092-8674(01)00492-5
2. Blasco MA. Telomere length, stem cells and aging. Nat Chem Biol. (2007) 3:640–9. doi: 10.1038/nchembio.2007.38
3. Fasching CL. Telomere length measurement as a clinical biomarker of aging and disease. Crit Rev Clin Lab Sci. (2018) 55:443–65. doi: 10.1080/10408363.2018.1504274
4. Srinivas N, Rachakonda S, Kumar R. Telomeres and telomere length: a general overview. Cancers (Basel). (2020) 12:558. doi: 10.3390/cancers12030558
5. Demanelis K, Jasmine F, Chen LS, Chernoff M, Tong L, Delgado D, et al. Determinants of telomere length across human tissues. Science. (2020) 369:eaaz6876. doi: 10.1126/science.aaz6876
6. Andrew T, Aviv A, Falchi M, Surdulescu GL, Gardner JP, Lu X, et al. Mapping genetic loci that determine leukocyte telomere length in a large sample of unselected female sibling pairs. Am J Hum Genet. (2006) 78:480–6. doi: 10.1086/500052
7. Jeanclos E, Schork NJ, Kyvik KO, Kimura M, Skurnick JH, Aviv A. Telomere length inversely correlates with pulse pressure and is highly familial. Hypertension. (2000) 36:195–200. doi: 10.1161/01.hyp.36.2.195
8. Armanios M, Blackburn EH. The telomere syndromes. Nat Rev Genet. (2012) 13:693–704. doi: 10.1038/nrg3246
9. Sanford SL, Welfer GA, Freudenthal BD, Opresko PL. Mechanisms of telomerase inhibition by oxidized and therapeutic Dntps. Nat Commun. (2020) 11:5288. doi: 10.1038/s41467-020-19115-y
10. Zee RY, Castonguay AJ, Barton NS, Germer S, Martin M. Mean leukocyte telomere length shortening and Type 2 diabetes mellitus: a case-control study. Transl Res. (2010) 155:166–9. doi: 10.1016/j.trsl.2009.09.012
11. Rode L, Nordestgaard BG, Bojesen SE. Long telomeres and cancer risk among 95 568 individuals from the general population. Int J Epidemiol. (2016) 45:1634–43. doi: 10.1093/ije/dyw179
12. Rode L, Nordestgaard BG, Bojesen SE. Peripheral blood leukocyte telomere length and mortality among 64,637 individuals from the general population. J Natl Cancer Inst. (2015) 107:djv074. doi: 10.1093/jnci/djv074
13. World Health Organization [WHO] Cardiovascular Diseases (CVDs). (2021). Available online at: https://wwwwhoint/en/news-room/fact-sheets/detail/cardiovascular-diseases-(cvds) (accessed June 11, 2021).
14. Zheng Y, Zhang N, Wang Y, Wang F, Li G, Tse G, et al. Association between leucocyte telomere length and the risk of atrial fibrillation: an updated systematic review and meta-analysis. Ageing Res Rev. (2022) 81:101707. doi: 10.1016/j.arr.2022.101707
15. Zimnitskaya OV, Petrova MM, Lareva NV, Cherniaeva MS, Al-Zamil M, Ivanova AE, et al. Leukocyte telomere length as a molecular biomarker of coronary heart disease. Genes (Basel). (2022) 13:1234. doi: 10.3390/genes13071234
16. Niu Z, Wen X, Buka SL, Wang M, Tian L, Loucks EB, et al. Associations of telomere length at birth with predicted atherosclerotic lesions and cardiovascular disease risk factors in midlife: a 40-year longitudinal study. Atherosclerosis. (2021) 333:67–74. doi: 10.1016/j.atherosclerosis.2021.08.013
17. Zhang A, Fan L, Bao X, Xu Z, Yin Z, Zhuo Y, et al. Leukocyte telomere length shortening implies plaque instability and major adverse cardiovascular events in patients with angiographically intermediate lesions. Front Cardiovasc Med. (2021) 8:812363. doi: 10.3389/fcvm.2021.812363
18. Schneider CV, Schneider KM, Teumer A, Rudolph KL, Hartmann D, Rader DJ, et al. Association of telomere length with risk of disease and mortality. JAMA Intern Med. (2022) 182:291–300. doi: 10.1001/jamainternmed.2021.7804
19. Haycock PC, Heydon EE, Kaptoge S, Butterworth AS, Thompson A, Willeit P. Leucocyte telomere length and risk of cardiovascular disease: systematic review and meta-analysis. BMJ. (2014) 349:g4227. doi: 10.1136/bmj.g4227
20. Huang YQ, Lo K, Feng YQ, Zhang B. The association of mean telomere length with all-cause, cerebrovascular and cardiovascular mortality. Biosci Rep. (2019) 39:BSR20192306. doi: 10.1042/BSR20192306
21. Richmond RC, Davey Smith G. Mendelian randomization: concepts and scope. Cold Spring Harb Perspect Med. (2022) 12:a040501. doi: 10.1101/cshperspect.a040501
22. Davies NM, Holmes MV, Davey Smith G. Reading mendelian randomisation studies: a guide, glossary, and checklist for clinicians. BMJ. (2018) 362:k601. doi: 10.1136/bmj.k601
23. Codd V, Denniff M, Swinfield C, Warner S, Papakonstantinou M, Sheth S, et al. A major population resource of 474,074 participants in Uk biobank to investigate determinants and biomedical consequences of leukocyte telomere length. MedRxiv [Preprint]. (2021): doi: 10.1101/2021.03.18.21253457
24. Welsh S, Peakman T, Sheard S, Almond R. Comparison of DNA quantification methodology used in the DNA extraction protocol for the UK biobank cohort. BMC Genomics. (2017) 18:26. doi: 10.1186/s12864-016-3391-x
25. Pierce BL, Ahsan H, Vanderweele TJ. Power and instrument strength requirements for mendelian randomization studies using multiple genetic variants. Int J Epidemiol. (2011) 40:740–52. doi: 10.1093/ije/dyq151
26. Kamat MA, Blackshaw JA, Young R, Surendran P, Burgess S, Danesh J, et al. Phenoscanner V2: an expanded tool for searching human genotype-phenotype associations. Bioinformatics. (2019) 35:4851–3. doi: 10.1093/bioinformatics/btz469
27. Staley JR, Blackshaw J, Kamat MA, Ellis S, Surendran P, Sun BB, et al. Phenoscanner: a database of human genotype-phenotype associations. Bioinformatics. (2016) 32:3207–9. doi: 10.1093/bioinformatics/btw373
28. Nielsen JB, Thorolfsdottir RB, Fritsche LG, Zhou W, Skov MW, Graham SE, et al. Biobank-driven genomic discovery yields new insight into atrial fibrillation biology. Nat Genet. (2018) 50:1234–9. doi: 10.1038/s41588-018-0171-3
29. Malik R, Chauhan G, Traylor M, Sargurupremraj M, Okada Y, Mishra A, et al. Multiancestry genome-wide association study of 520,000 subjects identifies 32 loci associated with stroke and stroke subtypes. Nat Genet. (2018) 50:524–37. doi: 10.1038/s41588-018-0058-3
30. Hemani G, Zheng J, Elsworth B, Wade KH, Haberland V, Baird D, et al. The Mr-base platform supports systematic causal inference across the human phenome. Elife. (2018) 7:e34408. doi: 10.7554/eLife.34408
31. Zhao Q, Wang J, Hemani G, Bowden J, Small DS. Statistical inference in two-sample summary-data mendelian randomization using robust adjusted profile score. Ann Stat. (2020) 48:1742–69, 28.
32. Bowden J, Davey Smith G, Haycock PC, Burgess S. Consistent estimation in mendelian randomization with some invalid instruments using a weighted median estimator. Genet Epidemiol. (2016) 40:304–14. doi: 10.1002/gepi.21965
33. Burgess S, Scott RA, Timpson NJ, Davey Smith G, Thompson SG, EPIC- InterAct Consortium. Using published data in mendelian randomization: a blueprint for efficient identification of causal risk factors. Eur J Epidemiol. (2015) 30:543–52. doi: 10.1007/s10654-015-0011-z
34. Verbanck M, Chen CY, Neale B, Do R. Detection of widespread horizontal pleiotropy in causal relationships inferred from mendelian randomization between complex traits and diseases. Nat Genet. (2018) 50:693–8. doi: 10.1038/s41588-018-0099-7
35. Xu X, Hu H, Lin Y, Huang F, Ji H, Li Y, et al. Differences in leukocyte telomere length between coronary heart disease and normal population: a multipopulation meta-analysis. Biomed Res Int. (2019) 2019:5046867. doi: 10.1155/2019/5046867
36. Emami M, Agbaedeng TA, Thomas G, Middeldorp ME, Thiyagarajah A, Wong CX, et al. Accelerated biological aging secondary to cardiometabolic risk factors is a predictor of cardiovascular mortality: a systematic review and meta-analysis. Can J Cardiol. (2022) 38:365–75. doi: 10.1016/j.cjca.2021.10.012
37. Jin X, Pan B, Dang X, Wu H, Xu D. Relationship between short telomere length and stroke: a meta-analysis. Medicine (Baltimore). (2018) 97:e12489. doi: 10.1097/MD.0000000000012489
38. Koriath M, Muller C, Pfeiffer N, Nickels S, Beutel M, Schmidtmann I, et al. Relative telomere length and cardiovascular risk factors. Biomolecules. (2019) 9:192. doi: 10.3390/biom9050192
39. Morgan RG, Ives SJ, Walker AE, Cawthon RM, Andtbacka RH, Noyes D, et al. Role of arterial telomere dysfunction in hypertension: relative contributions of telomere shortening and telomere uncapping. J Hypertens. (2014) 32:1293–9. doi: 10.1097/HJH.0000000000000157
40. Peng H, Mete M, Desale S, Fretts AM, Cole SA, Best LG, et al. Leukocyte telomere length and ideal cardiovascular health in American Indians: The strong heart family study. Eur J Epidemiol. (2017) 32:67–75. doi: 10.1007/s10654-016-0199-6
41. Tellechea ML, Pirola CJ. The impact of hypertension on leukocyte telomere length: A systematic review and meta-analysis of human studies. J Hum Hypertens. (2017) 31:99–105. doi: 10.1038/jhh.2016.45
42. Zgheib NK, Sleiman F, Nasreddine L, Nasrallah M, Nakhoul N, Isma’eel H, et al. Short telomere length is associated with aging, central obesity, poor sleep and hypertension in lebanese individuals. Aging Dis. (2018) 9:77–89. doi: 10.14336/AD.2017.0310
43. Yu SN, Chen SQ, Fan GQ, Pan WZ, Jia J, Wang Q, et al. Relative telomere length in peripheral blood cells and hypertension risk among mine workers: A case-control study in Chinese coal miners. Biomed Res Int. (2020) 2020:5681096. doi: 10.1155/2020/5681096
44. Cheng G, Wang L, Dai M, Wei F, Xu D. Shorter leukocyte telomere length coupled with lower expression of telomerase genes in patients with essential hypertension. Int J Med Sci. (2020) 17:2180–6. doi: 10.7150/ijms.48456
45. Gao YY, Guo JY, Zhang Z, Han ZC, Lei LJ, Sun CM, et al. [Relationship of telomere length, mitochondrial DNA copy number of peripheral blood with hypertension in coal miners]. Zhonghua Liu Xing Bing Xue Za Zhi. (2020) 41:727–32. doi: 10.3760/cma.j.cn112338-20190930-00714
46. Rosero-Bixby L, Rehkopf DH, Dow WH, Lin J, Epel ES, Azofeifa J, et al. Correlates of longitudinal leukocyte telomere length in the costa rican longevity study of healthy aging (CRELES): on the importance of DNA collection and storage procedures. PLoS One. (2019) 14:e0223766. doi: 10.1371/journal.pone.0223766
47. Bekaert S, De Meyer T, Rietzschel ER, De Buyzere ML, De Bacquer D, Langlois M, et al. Telomere length and cardiovascular risk factors in a middle-aged population free of overt cardiovascular disease. Aging Cell. (2007) 6:639–47. doi: 10.1111/j.1474-9726.2007.00321.x
48. Revesz D, Milaneschi Y, Verhoeven JE, Penninx BW. Telomere length as a marker of cellular aging is associated with prevalence and progression of metabolic syndrome. J Clin Endocrinol Metab. (2014) 99:4607–15. doi: 10.1210/jc.2014-1851
49. Rehkopf DH, Needham BL, Lin J, Blackburn EH, Zota AR, Wojcicki JM, et al. Leukocyte telomere length in relation to 17 biomarkers of cardiovascular disease risk: a cross-sectional study of US adults. PLoS Med. (2016) 13:e1002188. doi: 10.1371/journal.pmed.1002188
50. Huang YQ, Liu L, Lo K, Huang JY, Zhang B, Feng YQ. The relationship between mean telomere length and blood pressure: results from the national health and nutrition examination surveys. Ann Transl Med. (2020) 8:535. doi: 10.21037/atm.2020.03.205
51. Mwasongwe S, Gao Y, Griswold M, Wilson JG, Aviv A, Reiner AP, et al. Leukocyte telomere length and cardiovascular disease in African Americans: the Jackson heart study. Atherosclerosis. (2017) 266:41–7. doi: 10.1016/j.atherosclerosis.2017.09.016
52. Yeap BB, Hui J, Knuiman MW, Flicker L, Divitini ML, Arscott GM, et al. U-shaped relationship of leukocyte telomere length with all-cause and cancer-related mortality in older men. J Gerontol A Biol Sci Med Sci. (2021) 76:164–71. doi: 10.1093/gerona/glaa190
53. Arbeev KG, Verhulst S, Steenstrup T, Kark JD, Bagley O, Kooperberg C, et al. Association of leukocyte telomere length with mortality among adult participants in 3 longitudinal studies. JAMA Netw Open. (2020) 3:e200023. doi: 10.1001/jamanetworkopen.2020.0023
54. Wang Q, Zhan Y, Pedersen NL, Fang F, Hagg S. Telomere length and all-cause mortality: a meta-analysis. Ageing Res Rev. (2018) 48:11–20. doi: 10.1016/j.arr.2018.09.002
55. Zhang N, Fan C, Gong M, Liang X, Zhang W, Li G, et al. Leucocyte telomere length and paroxysmal atrial fibrillation: a prospective cohort study and systematic review with meta-analysis. J Clin Lab Anal. (2018) 32:e22599. doi: 10.1002/jcla.22599
56. Romaine SPR, Denniff M, Codd V, Nath M, Koekemoer A, Anker SD, et al. Telomere length is independently associated with all-cause mortality in chronic heart failure. Heart. (2022) 108:124–9. doi: 10.1136/heartjnl-2020-318654
57. Wang Q, Liu Z, Dong Y, Yang X, Chen M, Gao Y. Leukocyte telomere length predicts progression from paroxysmal to persistent atrial fibrillation in the long term after catheter ablation. Front Cardiovasc Med. (2021) 8:813390. doi: 10.3389/fcvm.2021.813390
58. Barnes RP, Fouquerel E, Opresko PL. The impact of oxidative DNA damage and stress on telomere homeostasis. Mech Ageing Dev. (2019) 177:37–45. doi: 10.1016/j.mad.2018.03.013
59. Minamino T, Miyauchi H, Yoshida T, Ishida Y, Yoshida H, Komuro I. Endothelial cell senescence in human atherosclerosis: role of telomere in endothelial dysfunction. Circulation. (2002) 105:1541–4. doi: 10.1161/01.cir.0000013836.85741.17
60. Matthews C, Gorenne I, Scott S, Figg N, Kirkpatrick P, Ritchie A, et al. Vascular smooth muscle cells undergo telomere-based senescence in human atherosclerosis: effects of telomerase and oxidative stress. Circ Res. (2006) 99:156–64. doi: 10.1161/01.RES.0000233315.38086.bc
61. Gruber HJ, Semeraro MD, Renner W, Herrmann M. Telomeres and age-related diseases. Biomedicines. (2021) 9:1335. doi: 10.3390/biomedicines9101335
62. Zhao J, Miao K, Wang H, Ding H, Wang DW. Association between telomere length and Type 2 diabetes mellitus: a meta-analysis. PLoS One. (2013) 8:e79993. doi: 10.1371/journal.pone.0079993
Keywords: telomere length, cardiovascular diseases, genetic variants, Mendelian randomization, causal association
Citation: Deng Y, Li Q, Zhou F, Li G, Liu J, Lv J, Li L and Chang D (2022) Telomere length and the risk of cardiovascular diseases: A Mendelian randomization study. Front. Cardiovasc. Med. 9:1012615. doi: 10.3389/fcvm.2022.1012615
Received: 05 August 2022; Accepted: 26 September 2022;
Published: 24 October 2022.
Edited by:
Margarita Alexandrovna Sazonova, Russian Academy of Medical Sciences, RussiaReviewed by:
Nazareno Paolocci, Johns Hopkins University, United StatesCopyright © 2022 Deng, Li, Zhou, Li, Liu, Lv, Li and Chang. This is an open-access article distributed under the terms of the Creative Commons Attribution License (CC BY). The use, distribution or reproduction in other forums is permitted, provided the original author(s) and the copyright owner(s) are credited and that the original publication in this journal is cited, in accordance with accepted academic practice. No use, distribution or reproduction is permitted which does not comply with these terms.
*Correspondence: Dong Chang, Y2RpaGVhcnRAMTYzLmNvbQ==
Disclaimer: All claims expressed in this article are solely those of the authors and do not necessarily represent those of their affiliated organizations, or those of the publisher, the editors and the reviewers. Any product that may be evaluated in this article or claim that may be made by its manufacturer is not guaranteed or endorsed by the publisher.
Research integrity at Frontiers
Learn more about the work of our research integrity team to safeguard the quality of each article we publish.