- 1Department of Cardiovascular Surgery, Xiangya Hospital, Central South University, Changsha, China
- 2National Clinical Research Center for Geriatric Disorders, Xiangya Hospital, Central South University, Changsha, China
Background: Pathological tissue remodeling such as fibrosis is developed in various cardiac diseases. As one of cardiac activated-myofibroblast protein markers, CKAP4 may be involved in this process and the mechanisms have not been explored.
Methods: We assumed that CKAP4 held a role in the regulation of cardiac fibrotic remodeling as an RNA-binding protein. Using improved RNA immunoprecipitation and sequencing (iRIP-seq), we sought to analyze the RNAs bound by CKAP4 in normal atrial muscle (IP1 group) and remodeling fibrotic atrial muscle (IP2 group) from patients with cardiac valvular disease. Quantitative PCR and Western blotting were applied to identify CKAP4 mRNA and protein expression levels in human right atrium samples.
Results: iRIP-seq was successfully performed, CKAP4-bound RNAs were characterized. By statistically analyzing the distribution of binding peaks in various regions on the reference human genome, we found that the reads of IP samples were mainly distributed in the intergenic and intron regions implying that CKAP4 is more inclined to combine non-coding RNAs. There were 913 overlapping binding peaks between the IP1 and IP2 groups. The top five binding motifs were obtained by HOMER, in which GGGAU was the binding sequence that appeared simultaneously in both IP groups. Binding peak-related gene cluster enrichment analysis demonstrated these genes were mainly involved in biological processes such as signal transduction, protein phosphorylation, axonal guidance, and cell connection. The signal pathways ranking most varied in the IP2 group compared to the IP1 group were relating to mitotic cell cycle, protein ubiquitination and nerve growth factor receptors. More impressively, peak analysis revealed the lncRNA-binding features of CKAP4 in both IP groups. Furthermore, qPCR verified CKAP4 differentially bound lncRNAs including LINC00504, FLJ22447, RP11-326N17.2, and HELLPAR in remodeling myocardial tissues when compared with normal myocardial tissues. Finally, the expression of CKAP4 is down-regulated in human remodeling fibrotic atrium.
Conclusions: We reveal certain RNA-binding features of CKAP4 suggesting a relevant role as an unconventional RNA-binding protein in cardiac remodeling process. Deeper structural and functional analysis will be helpful to enrich the regulatory network of cardiac remodeling and to identify potential therapeutic targets.
Introduction
Maintenance of normal cardiac function requires fine-tune regulation of myocardial homeostasis that enables the heart to self-adjust under physiological stress and volume load to produce physiological hypertrophic responses and compensatory growth, thus playing a key role in the cardiac development and stress response (1–3). Acquisition of cardiac homeostasis needs normal specific cellular-molecular mechanisms and coordinated intercellular interactions which could be interfered by persistent and harmful external environmental stimuli such as overload, myocardial ischemia, leading to pathological tissue remodeling deviating from homeostasis and causing various cardiac diseases such as ischemic cardiomyopathy, hypertrophic cardiomyopathy, atrial fibrillation and other arrhythmias (4–7). Heart failure caused by these diseases further promotes abnormal myocardial remodeling, which in turn exacerbates heart failure to form a vicious circle (8). Excessive fibrosis, degeneration and loss of cardiomyocytes have been involved in this pathological process (9–11). Although the potentially complex molecular regulatory networks have been extensively studied, our knowledge of pathological cardiac remodeling is still limited (12–14).
Classical RNA-binding proteins (RBPs) have been discovered to participate the regulation of genetic posttranscriptional expression and been essential regulatory molecules to maintain normal cell function and RNA stability (15, 16). These proteins are also implicated in the development of multiple cardiovascular diseases (17, 18). Recently, by high-throughput translation group sequencing, many RBPs associated with myocardial fibrosis have been excavated (19). They have been validated to target transcriptional sequences, control protein expression levels, and to promote cardiac fibroblasts activation into myofibroblasts, which is an important mechanism dominating the progression of dilated cardiomyopathy and heart failure (18, 19). Furthermore, recent efforts have identified a series of non-classical RBPs, expanding our understanding of this category proteins (20, 21). Without classical RNA-binding domains, these RBPs not only bind mRNA, but also interact with non-coding RNA, mediating important biological process such as stress response and energy metabolism (20, 21).
Previous studies have asserted that cytoskeleton-associated protein 4 (CKAP4, CLIMP-63) is a multifunctional trans-membrane protein as a receptor for several ligands (APF, SPA, tPA, DKK-1) (22–25). As an initially discovered functional protein on the endoplasmic reticulum, CKAP4 acts to govern the morphology and quantity of endoplasmic reticulum membrane and mediate the cross-linking of endoplasmic reticulum and microtubules (26, 27). Interestingly, CKAP4 can also form complexes with protein Dicer and be involved in the processing of microRNA precursors (28). In addition, CKAP4 have potential interaction with DNA sequences. For example, CKAP4 enhances the expression of connective tissue growth factor (CTGF/CCN2) gene through binding to its promoter (29). Recent research has demonstrated that CKAP4 as one of cardiac activated-myofibroblast protein markers may be involved in cardiac fibrosis (30).
Here, we assumed that CKAP4 held a role in the regulation of cardiac function and remodeling as an RNA-binding protein. Our study has confirmed decreased expression of CKAP4 in the enlarged atrial myocardium with pathological remodeling. By improved RNA immunoprecipitation and sequencing technology (iRIP-seq), we have revealed certain RNA-binding features of CKAP4 suggesting a relevant role as an unconventional RNA-binding protein in cardiac remodeling process.
Materials and Methods
Tissue Preparation
Atrial tissues were obtained from patients with cardiac valvular disease during valvular operations and were rapidly frozen in liquid nitrogen and stored at −80°C for protein extraction and RNA isolation. Atrial tissues of normal size from patients without atrial fibrillation were classified as normal group, and those of enlarged size from patients with atrial fibrillation were classified as remodeling group. The study was approved by the Ethics Committee of Xiangya Hospital, Central South University.
Immunoprecipitation
Myocardial tissues were irradiated once for 400 mJ/cm2, grinded in liquid nitrogen and lysed in ice-cold lysis buffer (1×PBS, 0.5% sodium deoxycholate, 0.1% SDS, 0.5% NP40) with RNase inhibitor (2313,Takara bio, Shiga, Japan) and a protease inhibitor (329-98-6, Solarbio, Beijing, China) on ice for 5 min. RQ I DNase (M610A, Promega, Madison, WI, USA) was added in lysis solution and incubated at 37 °C for 15 min followed by MNase (EN0181, Thermo Fisher Scientific, Waltham, MA, USA) added and incubated at 37°C for 10 min. The mixture was then vibrated vigorously and centrifuged at 13,000 x g at 4°C for 20 min to remove cell debris. The supernatant was incubated with DynaBeads protein A/G (26162, Thermo Fisher Scientific) conjugated with anti-CKAP4 antibody (16686-1-AP, Proteintech, Chicago, IL, USA) or normal IgG at 4°C overnight. Then the beads were washed with Low-salt Wash buffer, High-salt Wash buffer and 1X PNK Buffer, respectively. Following the beads were resuspended in Elution Buffer (50 mM Tris-Cl (PH = 8.0), 10 mM EDTA (PH = 8.0), 1%SDS) and divided into two parts, 4/5 for RNA isolation from CKAP4-RNA complexes and 1/5 for the western blotting assay for CKAP4.
Western Blot
The sample from immunoprecipitation was resuspended with 40 ul Elution Buffer, incubated at 70°C for 20 min at 1,400 rpm, and then centrifuged at 13,200 x g for a short time. The supernatant was transferred to a new EP tube on a magnetic separator. Next, complexes were eluted in boiling water for 10 min with 1X SDS sample buffer and separated on 10% SDS-PAGE. With TBST buffer (20 mM Tris-buffered saline and 0.1% Tween-20) containing 5% non-fat milk powder for 1 h at room temperature, the Membrane was incubated with primary antibody: CKAP4 antibody (1:1,000, 16686-1-AP, Proteintech), GAPDH antibody (1:2000, CUSABIO, Wuhan, China) and then with HRP-conjugated secondary antibody. Bound secondary antibody (anti-mouse or anti-rabbit 1:10,000) (Abcam, Cambridge, MA, USA) was detected using the enhanced chemiluminescence (ECL) reagent (170506, Bio-Rad, Hercules, CA, USA).
RNA Extraction and RT-qPCR
The CKAP4-bound RNAs were isolated from the immunoprecipitation sample using TRIzol (15596, Invitrogen, Carlsbad, CA, USA). cDNA libraries were prepared with One-Step gDNA Removal and cDNA Synthesis Mix (AT311-03, Transgen Biotech, Beijing, China). qPCR was prepared with HieffTM qPCR SYBR® Green Master Mix (11201, YEASEN, Shanghai, China) and detected on Q6 real-time PCR detection system. The information of primers used for RT-qPCR is presented in Supplemental Material.
For assessing the expression of CKAP4 in human myocardial tissues, we used housekeeping gene GAPDH (glyceraldehyde-3-phosphate dehydrogenase) as a control gene. cDNA synthesis was conducted by standard procedures for following real-time quantification PCR, which was performed with the HieffTM qPCR SYBR Green Master Mix (Low Rox Plus) (11202, YEASEN, Shanghai, China). The results were processed using 2-ΔΔCT method.
iRIP-Seq Library Preparation and Sequencing
The CKAP4-bound RNAs from the immunoprecipitation sample were isolated using TRIzol (Invitrogen). cDNA libraries were prepared with the KAPA RNA Hyper Prep Kit (KK8541, KAPA Biosystems, Boston, USA) according the manufacturer's procedure, followed by high-throughput sequencing on an Illumina Xten platform for 150 bp paired-end sequencing.
Data Analysis
Paired-end sequencing reads were aligned onto the human genome, only uniquely mapped reads were used for the following analysis. “ABLIRC” strategy was applied to identify the binding regions of CKAP4 on genome (31). Reads with at least 1 bp overlap were clustered as peaks. For each gene, computational simulation was performed to randomly generate reads with the same number and lengths as reads in peaks. The outputting reads were further mapped to the same gene to generate random max peak height from overlapping reads. The whole process was repeated for 500 times. All the observed peaks with heights higher than those of random max peaks (p < 0.05) were selected. The IP and input samples were analyzed by the simulation independently, and the IP peaks that have overlap with input peaks were removed. The target genes of IP were finally determined by the peaks and the binding motifs of CKAP4 were called by HOMER software.
Functional Enrichment Analysis
To sort out functional categories of peak-associated genes (target genes), Gene Ontology (GO) terms and KEGG pathways were identified using KOBAS 2.0 server (32). Hypergeometric test and Benjamini-Hochberg FDR controlling procedure were applied to define the enrichment of each term.
Availability of Data and Materials
The raw data are available under GEO Series accession number GSE161695.
Results
iRIP-Seq of CKAP4 in Myocardial Tissues
Using improved RNA immunoprecipitation and sequencing (iRIP-seq, improved RIP-seq), we sought to analyze the RNAs bound by CKAP4 in normal atrial muscle (IP1 group) and remodeling fibrotic atrial muscle (IP2 group) from patients with cardiac valvular disease. Quantitative PCR proved that CKAP4 was differentially expressed in two groups, and the expression was significantly down-regulated in the remodeling fibrosis group (Figure 1A). Western blotting further confirmed the difference of CKAP4 protein expression level between the two groups, and also showed the binding specificity of CKAP4 antibody and the effectiveness of immunoprecipitation technology (Figure 1B). Correlation analysis and hierarchical clustering analysis between samples suggested that the IP group and the input group had significant differences in the CKAP4-bound RNA and their respective characteristics (Figure 1C). By statistically analyzing the distribution of binding peaks in various regions on the reference human genome, we found that the reads of IP samples on the reference genome were mainly distributed in the intergenic and intron regions, and were enriched relative to the input group, which implies that CKAP4 is more inclined to combine non-coding RNAs (Figures 1D,E). The Venn diagram showed that there are 913 overlapping binding peaks between the IP1 and IP2 groups (Figure 1F). We used HOMER (Hypergeometric Optimization of Motif EnRichment) to perform motif analysis on the binding peaks obtained by the ABLIRC analysis method (31) to obtain the top five binding motifs in the IP samples, where GGGAU was the binding sequence that appeared simultaneously in both groups, suggesting that this sequence may be CKAP4 Preferred binding sequences (Figure 1G).
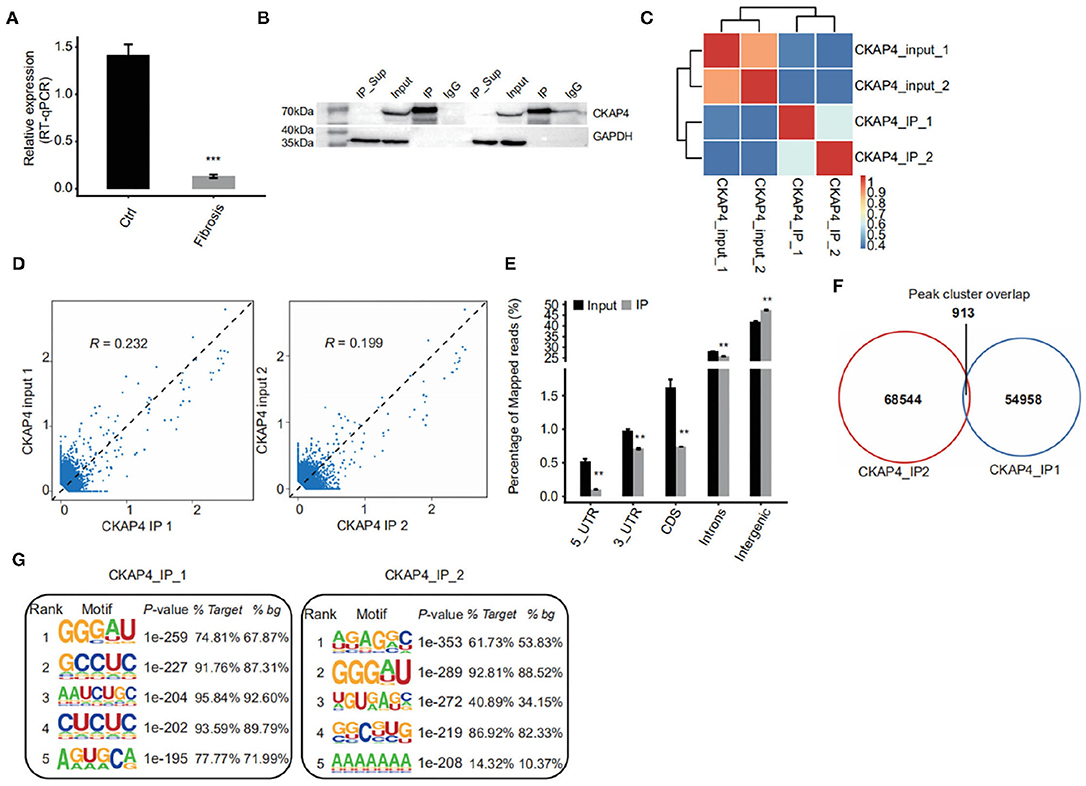
Figure 1. iRIP-seq of CKAP4 in myocardial tissues. (A) CAP4 RNA level was quantified by qRT-PCR. Error bars represent mean ± SEM. ***p < 0.001. (B) CKAP4 protein detection by western blot. (C) Heat map shows the hierarchically clustered Pearson correlation matrix resulted from comparing the transcript expression values for input and CKAP4 IP samples. (D) Scatter plot of reads abundance across reference genome in paired samples. (E) Reads distribution across reference genome. Error bars represent mean ± SEM. **p < 0.01. (F) Venn diagram of peaks identified from the IP1 and IP2 samples. (G) Top 5 overrepresented CKAP4-binding motifs identified by Homer from the iRIP-seq data.
Binding Peak-Related Gene Cluster Enrichment Analysis
Using Gene Ontology, we demonstrated the top 10 GO terms with their corrected p-values (Figures 2A–C). Cellular component categories showed that CKAP4-bound genes were mainly existed in cytoplasm, nucleus, cytoskeleton and cell membrane (Figure 2A). These genes were mainly involved in biological processes such as signal transduction, protein phosphorylation, axonal guidance, and cell connection (Figure 2C). The signal pathways ranking most varied in the remodeling myocardium group (IP2) compared to the normal atrial muscle group (IP1) were relating to mitotic cell cycle, protein ubiquitination and nerve growth factor receptors (Figure 2C). The results of KEGG pathway enrichment analysis were illustrated in Figure 2D in which focal adhesion, axonal guidance, cGMP-PKG signaling pathway were common in both groups.
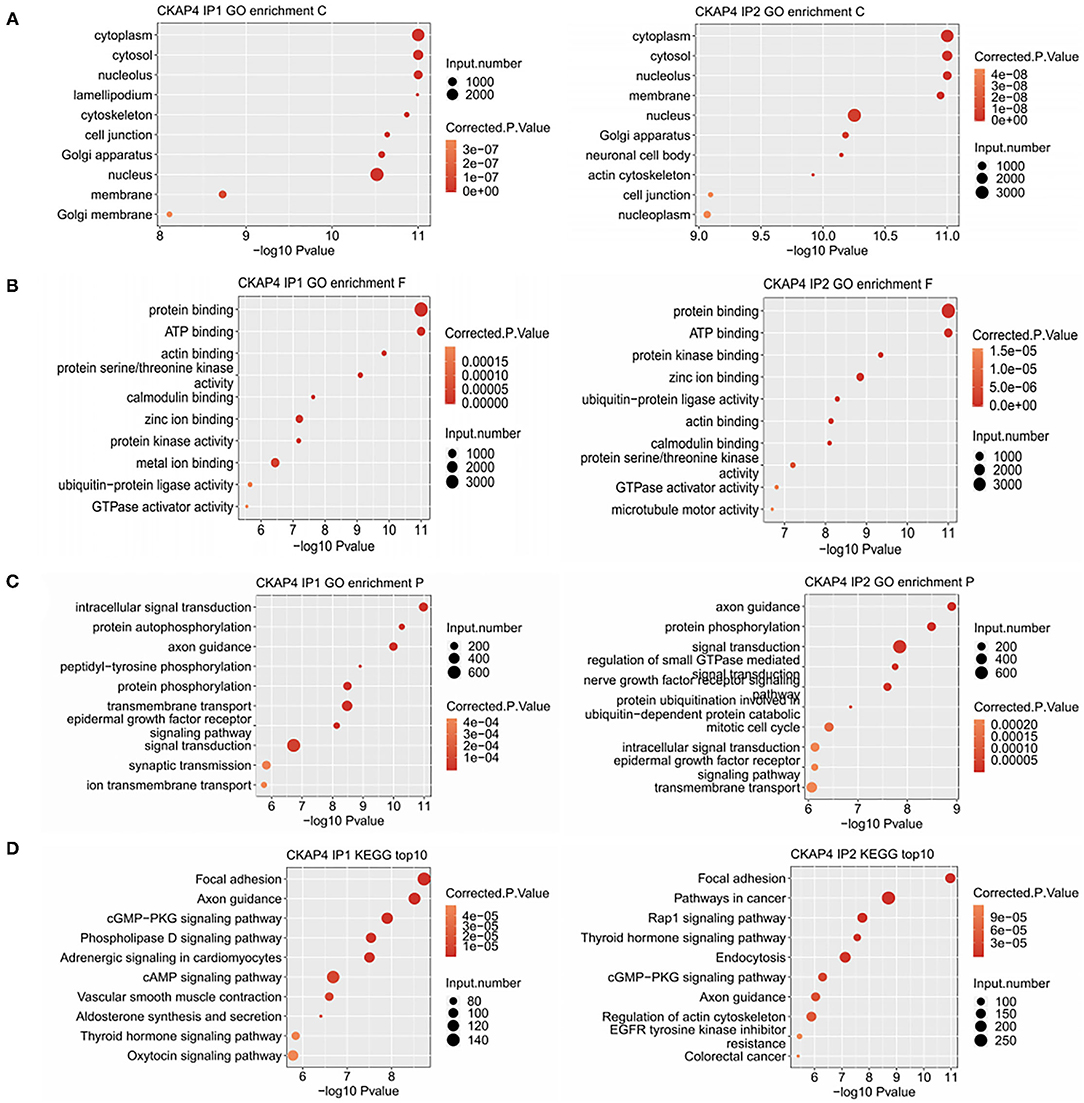
Figure 2. Binding peak-related gene cluster enrichment analysis. Bubble plot showed the top 10 enriched GO cellular component (A), molecular function (B), biological process (C) and KEGG (D) categories for the CKAP4-bound genes.
Characteristics of CKAP4 Binding to Non-coding RNAs
In our results, more fascinating is the ability of CKAP4 interacting with non-coding RNAs, especially the long non-coding RNAs (lncRNAs) (Figure 3A). HOMER motif analysis revealed the top 10 binding-motifs on lncRNAs tended to enrich base G, A, C (Figure 3B). Through gene annotation and correlation analysis of binding-peaks reads, we found that at least four lncRNAs including LINC00504, FLJ22447, RP11-326N17.2 and HELLPAR were CKAP4 potential binding lncRNAs in myocardial tissues (Figure 3C).
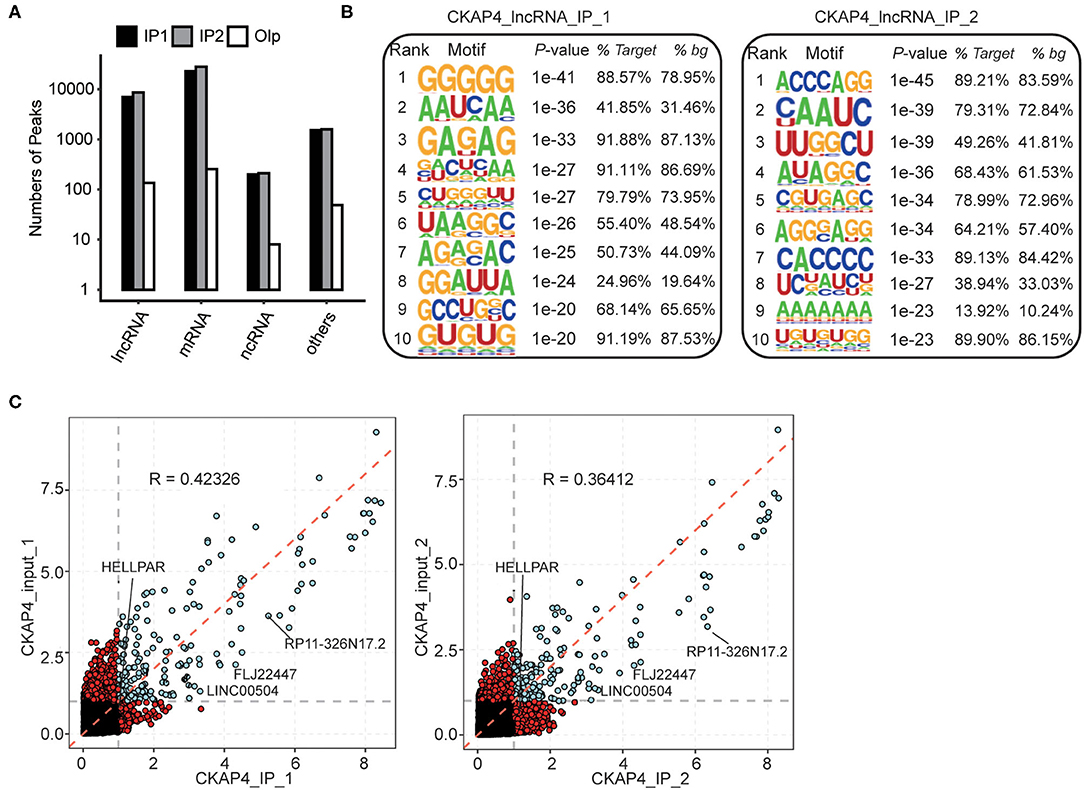
Figure 3. Peak analysis revealed the lncRNA-binding features of CKAP4. (A) Bar plot showed the peak numbers of different RNA types. (B) Motif analysis showed the top 10 preferred bound motifs of lncRNAs by HOMER software. (C) Scatter plot showed the gene RPKM (Reads per kilo base of a gene per million reads) in paired samples. Red points represented the specifically expressed genes in IP or input samples.
CKAP4 Preferentially Binds to lncRNAs in Remodeling Myocardial Tissues
We further delved into the binding sites of CKAP4 with four lncRNAs including LINC00504, FLJ22447, RP11-326N17.2 and HELLPAR. The localization of these sites on genome is detailed in Figures 4A–D. Some sites overlap in the normal and remodeling myocardial groups and are considered to be more credible binding sites. Finally, we used quantitative PCR to verify the binding reliability of CKAP4 with these lncRNAs. The results suggest that CKAP4 differentially bind lncRNAs in remodeling myocardial tissues when compared with the control group (Figures 4A–D, right), indicating that the interaction between CKAP4 and lncRNAs may be related to myocardial remodeling such as fibrosis.
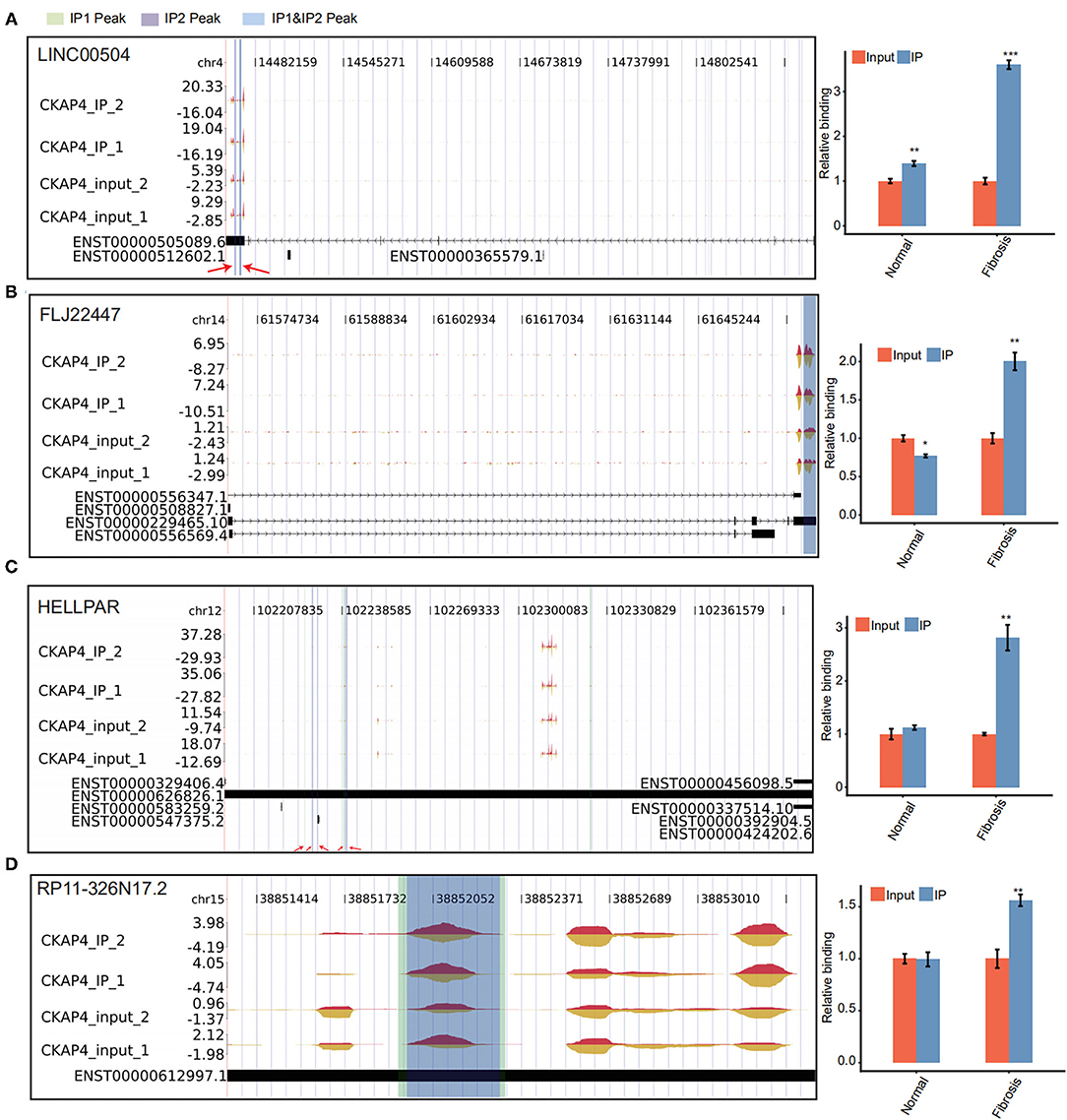
Figure 4. CKAP4 preferentially binds to lncRNAs in remodeling myocardial tissues. (A–D) IGV-sashimi plot showed CKAP4 binding sites across lncRNAs, including LINC00504 (A), FLJ22447 (B), HELLPAR (C) and RP11-326N17.2 (D). RIP-qPCR validated the binding reliability of CKAP4 with these lncRNAs (right). The asterisk (*) indicates *p < 0.05, **p < 0.01, ***p < 0.001.
CKAP4 Expression Is Down-Regulated in Remodeling Fibrotic Myocardium
we selected right atrial specimens from patients with heart valve disease for quantitative PCR detection (Figure 5A) and Western blotting (Figure 5B) to identify CKAP4 mRNA and protein expression levels. We found that the mRNA and protein expression of CKAP4 in remodeling myocardial samples from significantly enlarged right atrium decreased relative to normal-size right atrial specimens.
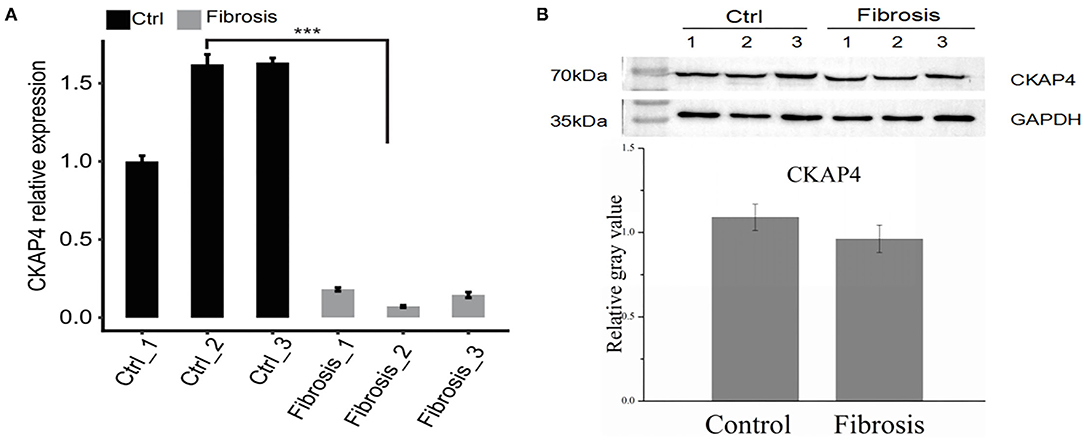
Figure 5. CKAP4 expression is down-regulated in remodeling fibrotic myocardium. Quantitative PCR (A) and Western blotting (B) results indicated the mRNA and protein expression of CKAP4 in remodeling fibrotic myocardial samples (n = 3) decreased significantly relative to control samples (n = 3). ***p < 0.001.
Discussion
Although previous studies have shown that CKAP4 is a multifunctional protein (33), few reports of its ability to bind to RNAs were published. A recent study demonstrates that lncRNA SENCER in vascular cells can bind to CKAP4 non-classical RBD (RNA binding domain) to influence its interaction with membrane protein CDH5(VE-cadherin), thereby stabilizing the latter and maintaining the integrity of endothelial cell adhesion junctions (34). Knockout SENCER enhances protein-protein interactions between CKAP4 and CDH5 which facilitates CDH5 internalization, therefore impairs the adhesion function of endothelial cells (34). Interestingly, in remodeling cardiac tissues, we have found four binding peaks of CKAP4 with CDH5 transcripts (data not shown), but not in normal myocardial tissues. This suggests that during cardiac remodeling, CKAP4 may affect adhesion function and permeability of endothelial cells by regulating location and function of CDH5. In addition, our research reveals that CKAP4 has a broader unknown RNA-binding potential. We believe its interactions with mRNAs and lncRNAs can not only regulate the RNA network, but also in turn may affect its own expression level, cell localization and complex functions.
Using single-cell sequencing techniques, Monika M.Gladka et al. have confirmed that CKAP4 is a protein marker for activated myofibroblasts in ischemic injury myocardium (30). Inhibition of CKAP4 expression increases the expression of TGF-β-stimulated fibroblast activation-related genes implying that CKAP4 functions to weaken the expression of these genes (30). However, another study has reported that CKAP4 Promotes activation of atrial fibroblasts (35). The seemingly contradictory outcomes of these two studies are both originated from in vitro cell model. Our results indicate that CKAP4 expression is downregulated in the enlarged myocardium caused by human valvular diseases, which may be associated with myocardial fibrosis. We speculate that the expression level of CKAP4 in tissue is different from that at cellular level. Also CKAP4 may present different expression levels in tissues at different stages of the development of fibroblast transformation into myofibroblasts in which CKAP4 may be up-regulated in the very early stage of fibrosis and down-regulated in the later stage. In any case its specific role in the process of myocardial fibrosis needs further study.
Based on cluster analysis of CKAP4 binding peak-related genes, the localization of these genes is mainly in the cytoplasm, cytoskeleton, membrane bodies, and also in the nucleus. This is consistent with the function of CKAP4 as a transmembrane and framework-related protein. As one of the most abundant proteins in the flat cystic membrane around the endoplasmic reticulum, CKAP4 has higher expression levels in highly active secretory cells (27). It often interacts with other proteins and ribosomes to form polyribosomes on coarse endoplasmic reticulum membranes (27). All these studies indicate that CKAP4 is involved in the synthesis, transport and secretion of secretory proteins and membrane proteins. Furthermore, as one of the endoplasmic reticulum mRNA-related proteins and mRNA receptors, CKAP4 mediates mRNA to be anchored to the endoplasmic reticulum (36). The potential mechanism so far is not clear in detail, although supposed to be independent of ribosomes and translation process (36). We speculate that CKAP4 may directly bind to the mRNA of some secreted proteins and membrane proteins to localize them on the endoplasmic reticulum.
According to our results, the most important function of CKAP4 targeted genes is the protein-binding function, which is the most common role of secretory and membrane proteins. Through further functional and pathway analysis of binding targets, we have found that CKAP4 is associated with signal transduction, protein phosphorylation, axonal orientation, and cellular junction. Axon orientation involves the regulation of skeletal proteins such as actin and microtubules (37, 38). As one of the cell junctions, Focal adhesion is closely related to integrins (39). As an RNA-binding protein, How CKAP4 could participate in the regulatory network of cytoskeleton proteins and integrins, and how it affects myocardial remodeling requires more in-depth investigation.
Among targeted coding genes, zinc finger protein ZNF771 is most pronounced one with the most number of reads in the CKAP4-specific binding peaks in the remodeling myocardium group (IP2) (data not shown), and the function of this gene is unknown until now. Based on enrichment analysis of mitotic cycle-related genes of CKAP4 targets, MNAT1, ALMS1, HDAC1 have been dug out (data not shown). These genes have been found to be participated in the events of heart failure, cardiomyopathy and cardiac fibrosis in previous researches (40–45).
Our primary exploration suggests that CKAP4 is a non-classical RNA-binding protein. In addition to mRNA, lncRNAs are also its preferred binding targets. Our results confirm lncRNA FLJ22447 is an enriched binding target of CKAP4 in remodeling myocardium. There is evidence that lncRNA FLJ22447 expression is upregulated in tumor-associated stromal fibroblasts of oral squamous cell carcinoma, stabilizing IL-33 by inhibiting the autophagic lysosomal pathway, thereby promoting tumor cell proliferation (46). IL-33 is considered as a myocardial protective factor, which can inhibit cardiomyocyte apoptosis, reduce myocardial fibrosis and inflammatory response, and improve survival and function of ischemic myocardium (47, 48). Whether interaction between CKAP4 and lncRNA FLJ22447 could regulate myocardial remodeling by governing IL-33 expression is worth exploring, which is our next research program. Some other lncRNAs including HELLPAR, RP11-326N17.2 and LINC00504 are potential preferred binding targets of CKAP4, while their function is currently unknown.
Our study was limited to intrinsic defects in iRIP-RNA sequencing techniques and small quantities of tissue samples. Lack of cellular and animal experiments limited current research mainly to bio-information analysis. More samples and multi-level investigation are beneficial to obtain more reliable and concrete results.
Conclusion
In brief, CKAP4 is an impressive multifaceted protein. Previous studies have unraveled the protein-protein or protein-DNA interactions that CKAP4 is involved in, our study reveals that CKAP4 may be involved in a variety of biological processes through non-classical protein-RNA interactions in cardiac tissues. Deeper structural and functional analysis will be helpful to enrich the regulatory network of cardiac remodeling and to identify potential therapeutic targets.
Data Availability Statement
The datasets presented in this study can be found in online repositories. The names of the repository/repositories and accession number(s) can be found in the article/Supplementary Material.
Ethics Statement
The studies involving human participants were reviewed and approved by the Ethics Committee of Xiangya Hospital, Central South University. The patients/participants provided their written informed consent to participate in this study. Written informed consent was obtained from the individual(s) for the publication of any potentially identifiable images or data included in this article.
Author Contributions
HZ: collection of samples, experiments, data analysis, manuscript writing, and final approval of manuscript. YZ and CZ: experiments. ZX: concept and design, data analysis and interpretation, manuscript writing, and final approval of manuscript. All authors contributed to the article and approved the submitted version.
Conflict of Interest
The authors declare that the research was conducted in the absence of any commercial or financial relationships that could be construed as a potential conflict of interest.
Publisher's Note
All claims expressed in this article are solely those of the authors and do not necessarily represent those of their affiliated organizations, or those of the publisher, the editors and the reviewers. Any product that may be evaluated in this article, or claim that may be made by its manufacturer, is not guaranteed or endorsed by the publisher.
Supplementary Material
The Supplementary Material for this article can be found online at: https://www.frontiersin.org/articles/10.3389/fcvm.2021.773573/full#supplementary-material
Abbreviations
CKAP4, Cytoskeleton-associated protein 4; CLIMP-63, The microtubule-binding 63-kDa cytoskeleton-linking membrane protein; CTGF/CCN2, Connective tissue growth factor; iRIP-seq, Improved RNA immunoprecipitation and sequencing; lncRNAs, Long non-coding RNAs; RBPs, RNA-binding proteins; MNAT1, CDK-activating kinase assembly factor MAT1; ALMS1, Alstrom syndrome protein 1; HDAC1, Histone deacetylase 1; APF, Anti-proliferative factor; SPA, Surfactant protein A; tPA, Tissue plasminogen activator; DKK-1, Dickkopf-1; TGF-β, Transforming growth factor-β; CDH5, Cadherin 5.
References
1. Shave RE, Lieberman DE, Drane AL, Brown MG, Batterham AM, Worthington S, et al. Selection of endurance capabilities and the trade-off between pressure and volume in the evolution of the human heart. Proc Natl Acad Sci U S A. (2019) 116:19905–10. doi: 10.1073/pnas.1906902116
2. Arbab-Zadeh A, Perhonen M, Howden E, Peshock RM, Zhang R, Adams-Huet B, et al. Cardiac remodeling in response to 1 year of intensive endurance training. Circulation. (2014) 130:2152–61. doi: 10.1161/CIRCULATIONAHA.114.010775
3. Shimizu I, Minamino T. Physiological and pathological cardiac hypertrophy. J Mol Cell Cardiol. (2016) 97:245–62. doi: 10.1016/j.yjmcc.2016.06.001
4. Tucker NR, Chaffin M, Fleming SJ, Hall AW, Parsons VA, Bedi KJ, et al. Transcriptional and cellular diversity of the human heart. Circulation. (2020). doi: 10.1101/2020.01.06.896076
5. Skelly DA, Squiers GT, McLellan MA, Bolisetty MT, Robson P, Rosenthal NA, et al. Single-cell transcriptional profiling reveals cellular diversity and intercommunication in the mouse heart. Cell Rep. (2018) 22:600–10. doi: 10.1016/j.celrep.2017.12.072
6. Sciarretta S, Maejima Y, Zablocki D, Sadoshima J. The Role of Autophagy in the Heart. Annu Rev Physiol. (2018) 80:1–26. doi: 10.1146/annurev-physiol-021317-121427
7. Nakamura M, Sadoshima J. Mechanisms of physiological and pathological cardiac hypertrophy. Nat Rev Cardiol. (2018) 15:387–407. doi: 10.1038/s41569-018-0007-y
8. Kemp CD, Conte JV. The pathophysiology of heart failure. Cardiovasc Pathol. (2012) 21:365–71. doi: 10.1016/j.carpath.2011.11.007
9. Gonzalez A, Schelbert EB, Diez J, Butler J. Myocardial interstitial fibrosis in heart failure: biological and translational perspectives. J Am Coll Cardiol. (2018) 71:1696–706. doi: 10.1016/j.jacc.2018.02.021
10. Takemura G, Miyata S, Kawase Y, Okada H, Maruyama R, Fujiwara H. Autophagic degeneration and death of cardiomyocytes in heart failure. Autophagy. (2006) 2:212–4. doi: 10.4161/auto.2608
11. Gasparovic H, Cikes M, Kopjar T, Hlupic L, Velagic V, Milicic D, et al. Atrial apoptosis and fibrosis adversely affect atrial conduit, reservoir and contractile functions. Interact Cardiovasc Thorac Surg. (2014) 19:223–30. doi: 10.1093/icvts/ivu095
12. Cohn JN, Ferrari R, Sharpe N. Cardiac remodeling–concepts and clinical implications: a consensus paper from an international forum on cardiac remodeling. Behalf of an International Forum on Cardiac Remodeling. J Am Coll Cardiol. (2000) 35:569–82. doi: 10.1016/S0735-1097(99)00630-0
13. Schirone L, Forte M, Palmerio S, Yee D, Nocella C, Angelini F, et al. A Review of the molecular mechanisms underlying the development and progression of cardiac remodeling. Oxid Med Cell Longev. (2017) 2017:3920195. doi: 10.1155/2017/3920195
14. Hein S, Arnon E, Kostin S, Schonburg M, Elsasser A, Polyakova V, et al. Progression from compensated hypertrophy to failure in the pressure-overloaded human heart: structural deterioration and compensatory mechanisms. Circulation. (2003) 107:984–91. doi: 10.1161/01.CIR.0000051865.66123.B7
15. Van Nostrand EL, Freese P, Pratt GA, Wang X, Wei X, Xiao R, et al. A large-scale binding and functional map of human RNA-binding proteins. Nature. (2020) 583:711–9. doi: 10.1038/s41586-020-2077-3
16. Gerstberger S, Hafner M, Tuschl T. A census of human RNA-binding proteins. Nat Rev Genet. (2014) 15:829–45. doi: 10.1038/nrg3813
17. Chu M, Novak SM, Cover C, Wang AA, Chinyere IR, Juneman EB, et al. Increased cardiac arrhythmogenesis associated with gap junction remodeling with upregulation of RNA-binding protein FXR1. Circulation. (2018) 137:605–18. doi: 10.1161/CIRCULATIONAHA.117.028976
18. de Bruin RG, Rabelink TJ, van Zonneveld AJ, van der Veer EP. Emerging roles for RNA-binding proteins as effectors and regulators of cardiovascular disease. Eur Heart J. (2017) 38:1380–8. doi: 10.1093/eurheartj/ehw567
19. Chothani S, Schafer S, Adami E, Viswanathan S, Widjaja AA, Langley SR, et al. Widespread translational control of fibrosis in the human heart by RNA-binding proteins. Circulation. (2019) 140:937–51. doi: 10.1161/CIRCULATIONAHA.119.039596
20. Hentze MW, Castello A, Schwarzl T, Preiss T. A brave new world of RNA-binding proteins. Nat Rev Mol Cell Biol. (2018) 19:327–41. doi: 10.1038/nrm.2017.130
21. Albihlal WS, Gerber AP. Unconventional RNA-binding proteins: an uncharted zone in RNA biology. FEBS Lett. (2018) 592:2917–31. doi: 10.1002/1873-3468.13161
22. Conrads TP, Tocci GM, Hood BL, Zhang CO, Guo L, Koch KR, et al. CKAP4/p63 is a receptor for the frizzled-8 protein-related antiproliferative factor from interstitial cystitis patients. J Biol Chem. (2006) 281:37836–43. doi: 10.1074/jbc.M604581200
23. Gupta N, Manevich Y, Kazi AS, Tao JQ, Fisher AB, Bates SR. Identification and characterization of p63 (CKAP4/ERGIC-63/CLIMP-63), a surfactant protein A binding protein, on type II pneumocytes. Am J Physiol Lung Cell Mol Physiol. (2006) 291:L436–46. doi: 10.1152/ajplung.00415.2005
24. Razzaq TM, Bass R, Vines DJ, Werner F, Whawell SA, Ellis V. Functional regulation of tissue plasminogen activator on the surface of vascular smooth muscle cells by the type-II transmembrane protein p63 (CKAP4). J Biol Chem. (2003) 278:42679–85. doi: 10.1074/jbc.M305695200
25. Kimura H, Fumoto K, Shojima K, Nojima S, Osugi Y, Tomihara H, et al. CKAP4 is a Dickkopf1 receptor and is involved in tumor progression. J Clin Invest. (2016) 126:2689–705. doi: 10.1172/JCI84658
26. Vedrenne C, Klopfenstein DR, Hauri HP. Phosphorylation controls CLIMP-63-mediated anchoring of the endoplasmic reticulum to microtubules. Mol Biol Cell. (2005) 16:1928–37. doi: 10.1091/mbc.e04-07-0554
27. Shibata Y, Shemesh T, Prinz WA, Palazzo AF, Kozlov MM, Rapoport TA. Mechanisms determining the morphology of the peripheral ER. Cell. (2010) 143:774–88. doi: 10.1016/j.cell.2010.11.007
28. Pepin G, Perron MP, Provost P. Regulation of human Dicer by the resident ER membrane protein CLIMP-63. Nucleic Acids Res. (2012) 40:11603–17. doi: 10.1093/nar/gks903
29. Matika CA, Wasilewski M, Arnott JA, Planey SL. Antiproliferative factor regulates connective tissue growth factor (CTGF/CCN2) expression in T24 bladder carcinoma cells. Mol Biol Cell. (2012) 23:1976–85. doi: 10.1091/mbc.e11-08-0714
30. Gladka MM, Molenaar B, de Ruiter H, van der Elst S, Tsui H, Versteeg D, et al. Single-cell sequencing of the healthy and diseased heart reveals cytoskeleton-associated protein 4 as a new modulator of fibroblasts activation. Circulation. (2018) 138:166–80. doi: 10.1161/CIRCULATIONAHA.117.030742
31. Xia H, Chen D, Wu Q, Wu G, Zhou Y, Zhang Y, et al. CELF1 preferentially binds to exon-intron boundary and regulates alternative splicing in HeLa cells. Biochim Biophys Acta Gene Regul Mech. (2017) 1860:911–21. doi: 10.1016/j.bbagrm.2017.07.004
32. Xie C, Mao X, Huang J, Ding Y, Wu J, Dong S, et al. KOBAS 2. 0: a web server for annotation and identification of enriched pathways and diseases. Nucleic Acids Res. (2011) 39:W316–22. doi: 10.1093/nar/gkr483
33. Sandoz PA, van der Goot FG. How many lives does CLIMP-63 have? Biochem Soc Trans. (2015) 43:222–8. doi: 10.1042/BST20140272
34. Lyu Q, Xu S, Lyu Y, Choi M, Christie CK, Slivano OJ, et al. SENCR stabilizes vascular endothelial cell adherens junctions through interaction with CKAP4. Proc Natl Acad Sci U S A. (2019) 116:546–55. doi: 10.1073/pnas.1810729116
35. Tan H, Chen Z, Chen F, Xu W, Liu X. CKAP4 participates in tryptase-induced phenotypic conversion in atrial fibroblasts through PAR2/p38/JNK pathway. Am J Transl Res. (2021) 13:2270–82.
36. Cui XA, Zhang H, Palazzo AF. p180 promotes the ribosome-independent localization of a subset of mRNA to the endoplasmic reticulum. PLoS Biol. (2012) 10:e1001336. doi: 10.1371/journal.pbio.1001336
37. Georges PC, Hadzimichalis NM, Sweet ES, Firestein BL. The yin-yang of dendrite morphology: unity of actin and microtubules. Mol Neurobiol. (2008) 38:270–84. doi: 10.1007/s12035-008-8046-8
38. Quinn CC, Wadsworth WG. Axon guidance: asymmetric signaling orients polarized outgrowth. Trends Cell Biol. (2008) 18:597–603. doi: 10.1016/j.tcb.2008.09.005
39. Burridge K. Focal adhesions: a personal perspective on a half century of progress. FEBS J. (2017) 284:3355–61. doi: 10.1111/febs.14195
40. Sano M, Izumi Y, Helenius K, Asakura M, Rossi DJ, Xie M, et al. Menage-a-trois 1 is critical for the transcriptional function of PPARgamma coactivator 1. Cell Metab. (2007) 5:129–42. doi: 10.1016/j.cmet.2007.01.003
41. Louw JJ, Corveleyn A, Jia Y, Iqbal S, Boshoff D, Gewillig M, et al. Homozygous loss-of-function mutation in ALMS1 causes the lethal disorder mitogenic cardiomyopathy in two siblings. Eur J Med Genet. (2014) 57:532–5. doi: 10.1016/j.ejmg.2014.06.004
42. Ichihara S, Yamamoto K, Asano H, Nakatochi M, Sukegawa M, Ichihara G, et al. Identification of a glutamic acid repeat polymorphism of ALMS1 as a novel genetic risk marker for early-onset myocardial infarction by genome-wide linkage analysis. Circ Cardiovasc Genet. (2013) 6:569–78. doi: 10.1161/CIRCGENETICS.111.000027
43. Zulato E, Favaretto F, Veronese C, Campanaro S, Marshall JD, Romano S, et al. ALMS1-deficient fibroblasts over-express extra-cellular matrix components, display cell cycle delay and are resistant to apoptosis. PLoS ONE. (2011) 6:e19081. doi: 10.1371/journal.pone.0019081
44. Williams SM, Golden-Mason L, Ferguson BS, Schuetze KB, Cavasin MA, Demos-Davies K, et al. Class I HDACs regulate angiotensin II-dependent cardiac fibrosis via fibroblasts and circulating fibrocytes. J Mol Cell Cardiol. (2014) 67:112–25. doi: 10.1016/j.yjmcc.2013.12.013
45. Schuetze KB, McKinsey TA, Long CS. Targeting cardiac fibroblasts to treat fibrosis of the heart: focus on HDACs. J Mol Cell Cardiol. (2014) 70:100–7. doi: 10.1016/j.yjmcc.2014.02.015
46. Ding L, Ren J, Zhang D, Li Y, Huang X, Hu Q, et al. A novel stromal lncRNA signature reprograms fibroblasts to promote the growth of oral squamous cell carcinoma via LncRNA-CAF/interleukin-33. Carcinogenesis. (2018) 39:397–406. doi: 10.1093/carcin/bgy006
47. Lax A, Sanchez-Mas J, Asensio-Lopez MC, Fernandez-Del PM, Caballero L, Garrido IP, et al. Mineralocorticoid receptor antagonists modulate galectin-3 and interleukin-33/ST2 signaling in left ventricular systolic dysfunction after acute myocardial infarction. JACC Heart Fail. (2015) 3:50–8. doi: 10.1016/j.jchf.2014.07.015
Keywords: cardiac fibrosis, cardiac remodeling, iRIP-seq, CKAP4, lncRNA
Citation: Zhu H, Zhang Y, Zhang C and Xie Z (2021) RNA-Binding Profiles of CKAP4 as an RNA-Binding Protein in Myocardial Tissues. Front. Cardiovasc. Med. 8:773573. doi: 10.3389/fcvm.2021.773573
Received: 10 September 2021; Accepted: 29 November 2021;
Published: 23 December 2021.
Edited by:
Lina A. Shehadeh, University of Miami, United StatesReviewed by:
Jose Francisco Islas, Autonomous University of Nuevo León, MexicoYasuhiro Maejima, Tokyo Medical and Dental University, Japan
Copyright © 2021 Zhu, Zhang, Zhang and Xie. This is an open-access article distributed under the terms of the Creative Commons Attribution License (CC BY). The use, distribution or reproduction in other forums is permitted, provided the original author(s) and the copyright owner(s) are credited and that the original publication in this journal is cited, in accordance with accepted academic practice. No use, distribution or reproduction is permitted which does not comply with these terms.
*Correspondence: Zhongshang Xie, eHpzNDEwNiYjeDAwMDQwO2NzdS5lZHUuY24=