- 1Department of Cardiovascular, The First Affiliated Hospital of Xi'an Jiaotong University, Xi'an, China
- 2Key Laboratory of Environment and Genes Related to Diseases, Ministry of Education, Xi'an, China
Heart failure (HF) is a complex clinical syndrome of which the incidence is on the rise worldwide. Cardiometabolic disorders are associated with the deterioration of cardiac function and progression of HF. Recently, there has been renewed interest in gut microbiota (GM) and its metabolites in the cardiovascular disease. HF-caused hypoperfusion could increase intestinal permeability, and a “leaky” bowel leads to bacterial translocation and make its metabolites more easily enter the circulation. Considerable evidence shows that the composition of microbiota and amino acids (AAs) has been altered in HF patients, and AAs could serve as a diagnostic and prognostic biomarker in HF. The findings indicate that the gut–amino acid–HF axis may play a key role in the progression of HF. In this paper, we focus on the interrelationship between the AA metabolism and GM alterations during the development of heart failure. We also discuss the potential prognostic and therapeutic value of the gut–amino acid–HF axis in the cortex of HF.
Introduction
Heart failure (HF) is a complex clinical syndrome and the end-stage of various cardiovascular diseases, which affects approximately 2% of adults worldwide (1). Although there have been great advances in the diagnosis and new treatments of HF, the overall prognosis for patients with HF is still not promising, and the hospital readmission and mortality rates of the patients are still high (2, 3). This makes it particularly important to understand and treat HF more precisely. Nowadays, with the advances of multiomic approaches (e.g., genomics, proteomics, transcriptomic, and metabolomics), novel therapeutic targets and strategies have been emerging to better characterize HF mechanistically as well as to investigate cutting-edge therapeutic strategies (4–7).
A growing number of research findings indicate that gut microbiota (GM) is strongly associated with human health and various diseases, including cardiovascular diseases (8–14). Recent researches have shown the regulating function of the gut microbiota and its metabolites in the manifestation and progression of HF (15, 16). A number of GM-associated metabolites, including short-chain fatty acids (SCFAs), bile acids (BAs), trimethylamine N-oxide (TMAO), amino acids (AAs) metabolites, and so on has been proven to take an active part in HF (16–21). These metabolites are the main pathways through which GM interacts with their hosts. Besides, the recent research have shown that the cardiac proteolysis attenuates in HF (22); thus, amino acids and its metabolites may have a strong impact on host through metabolic pathways. For example, the
tryptophan–kynurenine pathway and glycine-glyR α2-MAPK/ERK pathway have been indicated to obtain a cardioprotective effect (23, 24). However, with the emergence of other metabolite exploration in HF, there is basically no review mainly focused on the relationship among GM, AAs, and HF.
In this review, we reviewed the interrelationship between the long-term GM dysbiosis and AA perturbations in the development of HF. Moreover, we summarized the current researches regarding the alterations in the composition of intestinal microbiota in HF patients and the important pathogenic mechanism of AAs.
Gut Microbiota Alterations in Heart Failure
HF-caused hypoperfusion could increase intestinal permeability. The “leaky” bowel leads to bacterial translocation, making its metabolites more easily enter the circulation, such as endotoxin (LPS), the trigger of chronic inflammation (16, 25–28). However, except for the mechanism of a “leaky” gut, it is possible that the progression of HF could be regulated by alterations in the composition of GM and its metabolites (16).
Changes in the composition of GM in HF have been confirmed not only in the clinical study but also in experimental animal studies, which helped elucidate the role that microbiota played in the development of HF (14, 15, 29). Table 1 summarizes the several cohort studies that have demonstrated the GM alterations in HF patients (Table 1) (15, 25, 27, 30–33).
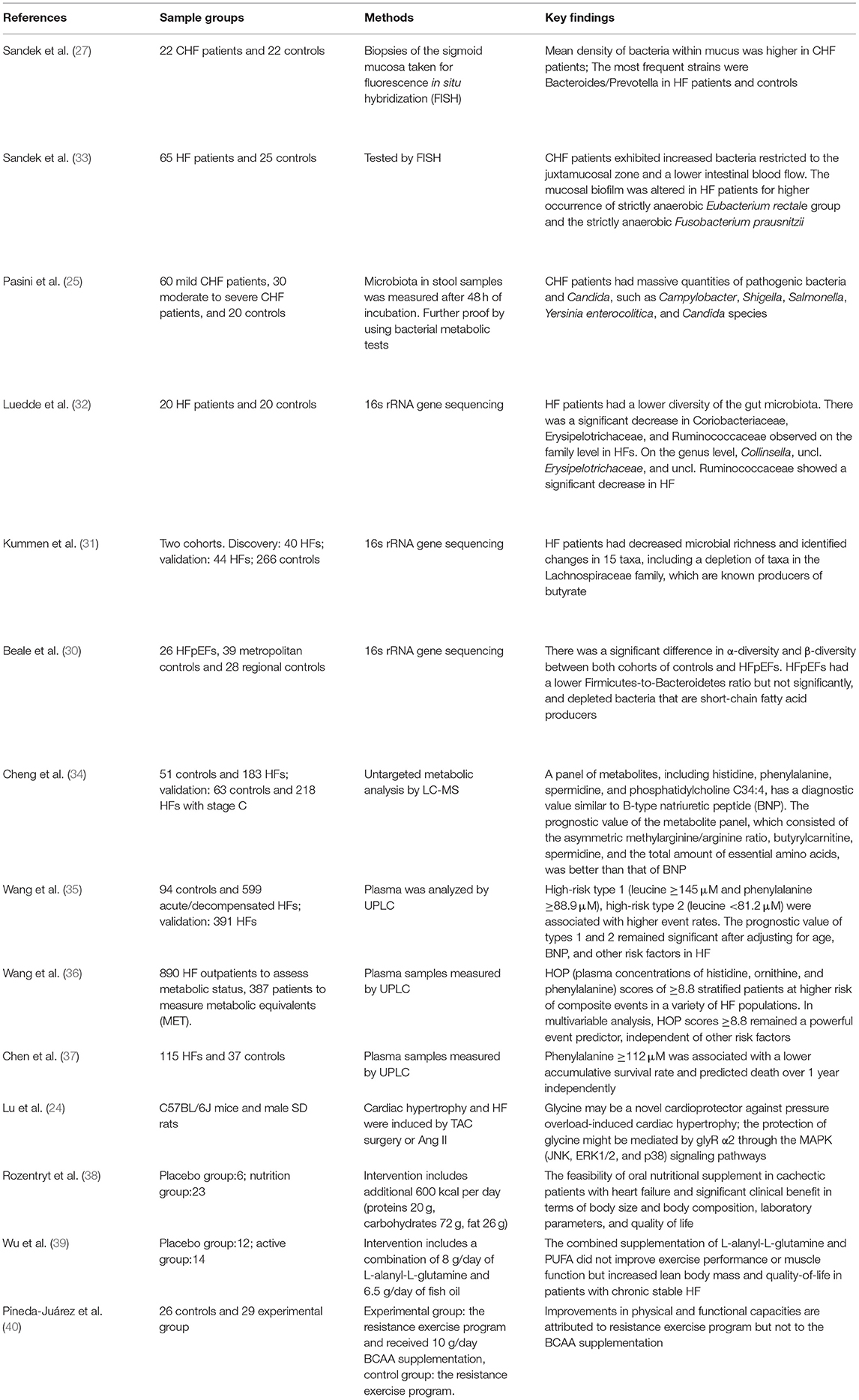
Table 1. Brief summary of studies investigating heart failure (HF) and alterations in microbiota and amino acids.
The study of Sandek et al. might play a fundamental role in the exploration of the relationship between HF and microbiota (27, 30). They found that the mean density of microbiota within mucus was higher in CHF patients, and the mucosal biofilm was altered for a higher occurrence of strictly anaerobic Eubacterium rectale group and the strictly anaerobic Fusobacterium prausnitzii. The first study using 16s rRNA gene sequencing approach was reported by Luedde et al. in 2017 and revealed that HF patients had a decreased diversity of the microbiota in parallel with the downregulation of vital microbiota groups (31). More recently, Kummen et al. identified microbiota changes in 15 taxa, including depletion of taxa in the Lachnospiraceae family, which are known producers of butyrate (32). Another study showed that HFpEFs had a lower Firmicutes-to-Bacteroidetes ratio but not significantly and depleted bacteria that are short-chain fatty acid producers (33). Additionally, there has been evidence that Bacteroides fragilis and Ruminococcus had a positive effect on HF (41).
Nonetheless, current studies have a controversy on the shifting tendency of the specific microbiota and its function in HF (14). Further studies are required to explore the link between HF and microbiota and to investigate the exact effects of microbiota.
Bacterial Metabolism of Amino Acids
As an essential part of nutrients in the diet, AAs could affect the nutrition of the host by interacting with gut microbiota. In addition, AAs also play a vital role in regulating the diversity and abundance of AA-fermenting microbiota for their heterogeneity in turn (42–46).
The catabolism of protein in the diet is inseparable from the GM. GM promotes the proteolysis of protein through host- and bacteria-derived proteases and peptidases, which are used for incorporation of amino acids into structural and respective proteins. Besides, GM facilitates protein fermentation, the productions of which are SCFAs, gases (H2, CO2, CH4, andH2S), nitrogenous metabolites, amines, indoles, and so on. There are several ways of disposal of the AA production: (1) excretion by feces or breath, (2) utilization by microbiota, (3) detoxification by colonic epithelium, and (4) absorption by intestinal epithelium and entering circulation (42).
AA-fermenting microbiota are effectively distinct in the intestine for the preference of AA utilization. Preferred AA substrates of Clostridium genus bacteria are lysine or proline, and the Peptostreptococcaceae genus played a vital role in glutamate or tryptophan utilization (42, 47). Anaerobes ferment aromatic amino acids, including Bacteroides, Lactobacillus, Bifidobacterium, Clostridium, and Peptostreptococcus (43). Moreover, it is shown that Clostridium sporogenes using aromatic amino acids as substrates generated 12 compounds, one of which, indolepropionic acid (IPA), act as a major metabolite and could affect intestinal permeability and immunity (48). Lactobacillus could also use tryptophan to produce indole metabolites offering mucosal protection from inflammation (49).
Moreover, it is noteworthy that several species are the key driver of AA metabolism, including Staphylococcus aureus, Megasphaera elsdenii, Bacteroides spp. et al. (42, 47). In conclusion, AAs and microbiota have a strong interaction with each other, and microbiota contributes to host AA homeostasis.
Dysregulated Amino Acid Metabolism in Heart Failure
As metabolomics is maturing, it is being utilized for early identification of organ function and dysfunction, and exploration of original disease pathways (7, 50). It is becoming clear that circulating metabolite profiles have a strong connection with HF severity and prognosis, which serve as promising novel biomarkers for identifying HF progression. Additionally, circulating amino acid profiles can directly reflect the host nutrition status, including food intake, absorption, tissue synthesis, and breakdown. Recent studies have provided evidence for microbial metabolism of AA in HF and investigated the value of AA for the identification and evaluation of HF.
Branched-chain amino acids (BCAAs), including valine, leucine, and isoleucine, are the major components in most mammals and account for nearly 35% of essential amino acids and 18% of the total amino acids (51, 52). One of the pivotal synthesis pathways of BCAA is in the microbiota, and uptake of BCAA from food is indispensable in humans. Different from not being synthesized in humans, BCAA catabolism not only occurs in the microbiota but also in humans and is mainly absorbed in the gut (52). More attention has been paid to the association between elevated circulating BCAA and HF (53–55). There are considerable evidence convincing the alterations of BCAA during HF, but these studies fail to explain the underlying mechanisms of how BCAA contributes to the progression of HF.
Large-scale trails, to date, have been carried out to demonstrate the relationship between circulating phenylalanine concentrations and HF (34, 35, 37, 56–59). Most of them suggest that increased phenylalanine could provide both diagnostic and prognostic value for HF. Consistent with such changings, there was a paralleling increasing trend in the serum tyrosine levels (35). Phenylalanine and tyrosine participates in the biopterin cycle; accumulation of them is a signal for tetrahydrobiopterin depletion, which often causes a problem with the production of NO and leading to cardiac dysfunction (34, 37). What is more, higher phenylalanine levels were correlated with higher C-reactive protein levels and higher pro-inflammation (37). It is an indication that HF patients with high phenylalanine levels have a more severe inflammation.
Tryptophan is one of the aromatic amino acids, and the kynurenine pathway (KP) is the key mechanism for tryptophan degradation (60–62). It has been shown that kynurenine has a strong relation to the pathophysiology of HF, such as inflammation, apoptosis, and oxidative stress (62). Increasing plasma levels of the kynurenine metabolites and the kynurenine:tryptophan ratio were associated with increased mortality in HF patients (23, 62). Furthermore, tryptophan is a pivotal substance for serotonin (5-HT) synthesis, and more than 90% of serotonin is synthesized from tryptophan in the intestine (61). Prior studies convincingly showed the considerable changes of 5-HT and 5-HT(2A)R in heart failure mice, and 5-HT2B receptor antagonists could inhibit right ventricular fibrosis through reducing collagen deposition (63–65). The newest study conducted by Cristina Razquin et al. examined the serotonin pathway and tryptophan-indole-3-propionic acid pathway of tryptophan metabolism, providing more evidence for understanding the relationship between tryptophan-related metabolites and HF (23).
In the pathophysiology of HF, failing hearts suffer from mitochondrial dysfunction, and their energy supplementation shifts from fatty acids to glucose utilization (34, 66). Histidine, arginine, and glutamine can be transferred into glutamate, offering energy and ornithine for cardiac tissues by participating into the Krebs cycle as alpha-ketoglutarate or the glutamate–ornithine–proline pathway (34). Previous studies further support the implicated mechanism of metabolic pathways in HF pathogenesis. It is almost certain that HF patients have a commonly decreasing trend in histidine, arginine, glutamine, and a reversely increase in ornithine (34). Furthermore, glutamate metabolism is ranked in the top 50 pathways in the enrichment overview for plasma in HF mice and patients (67).
As a nonessential amino acid, glycine has been repeatedly reported to have a hand in anti-inflammatory response, growth, and cytoprotection (68, 69). Recently, its beneficial effect in antihypertrophy and HF has been reported. Glycine antagonized left ventricular (LV) hypertrophy and cardiac fibrosis in HF mice (24). This paved a path for the generation of a novel treatment of HF.
In summary, a considerable number of previous studies confirmed that there commonly exists a disorder of AAs with a different trending in HF. Consistent with that, these results further supported the connection between HF and AAs.
Amino Acid As a Diagnostic and Prognostic Marker for Heart Failure
AAs have been shown to be a potential promising biomarker with significant diagnostic and prognostic values for HF. What we know about the values of AAs is largely based on the clinical studies that evaluate the possibility and stability of AAs as original biomarkers.
The study finished by Cheng et al. is one of the most influential researches to assess the metabolites and has identified a panel of four metabolites (histidine, phenylalanine, phosphatidylcholine diacyl C34:4, spermidine), which provided a similar diagnosis value and a better prognostic value than the conventional biomarker, BNP (34).
A recent study has classified patients at high risk for HF-related events into two groups: high-risk type 1 (leucine ≥145 μM and phenylalanine ≥88.9 μM), high-risk type 2 (leucine <81.2 μM), and other HF patients were placed into the low-risk group (35). Compared with the low-risk type, types of high-risk patients had a lower event-free survival rate, especially type 2 high-risk patients, which were characterized by severe malnutrition. This kind of novel simplified amino acid-based risk stratification offered a prognostic value for HF patients.
Wang et al. have assessed amino acid-based profile including histidine, ornithine, and phenylalanine (HOP score) (36). They have found a strong association between HOP score and cardiac function evaluated by the 6-min walking distance, which can compensate for the limitation of the NYHA Functional Classification System. A HOP score of ≥8.8 was associated with more risk factors for HF events.
In a study conducted by Chen et al., they measured the prognostic mortality value of phenylalanine in HF patients (37). Phenylalanine level has appeared to be positively related to the mortality of HF patients. In contrast to HF patients with phenylalanine <112 μM, patients with phenylalanine >112 μM had higher APACHE II and SOFA scores with higher mortality. Their analysis demonstrated that phenylalanine was an independent predictor of mortality and suggested setting 112 μM as a critical point for identifying different outcomes.
Up to now, there have been considerable clinical researches published to describe the significant value of AAs as diagnostic and prognostic biomarkers in HF. These results indicate that dysregulated AA is not only the result of HF but also a potential indicator for the progression of HF.
Gut–Amino Acid–Heart Failure Axis As a Potential Therapeutic Target for Heart Failure
A burgeoning number of relevant studies have demonstrated that there is a microbiota dysbiosis and amino acid alterations in HF; otherwise, there is a tight connection among gut microbiota, amino acids, and heart failure. What stands out is the promising values of the gut–amino acid–HF axis as a potential therapeutic target for HF.
Probiotics are live microorganisms, and prebiotics are nondigestible food products, which can change the microbiota composition and activity (14). Both of them are reported to be beneficial to the host and be cardioprotective. Lactobacillus rhamnosus GR-1 has been considered as a potential therapy for the attenuation of heart failure, so did Lactobacillus plantarum 299v and Saccharomyces boulardii (70, 71). However, antibiotics regulate the composition of microbiota through inhibiting specific types of negative bacteria (72). Vancomycin could improve the cardiac function in rats; polymyxin B had a similar function (70, 73). At the same time, it also increases the possibility of drug-resistant microbiota (74).
Dietary intervention for delaying the progression of HF mainly follows the guidelines from the American College of Cardiology/American Heart Association (75). The recommended Dietary Approaches to Stop Hypertension (DASH) eating plan has been assessed by several clinical trials and suggested its beneficial role for reducing HF incidence (76–79). It is also reported that several nutritional factors could change intestinal permeability, acting as a potential dietary intervention of HF (80).
Fecal microbiota transplant (FMT) is a budding way of transferring a positive microbiota into the unhealthy receiver. It has been proven to be effective in the treatment of Clostridium difficile infection, inflammatory bowel disease, and metabolic syndrome (81, 82). However, it also brings about a problem of virus transmission (83). Up to now, there are no related trials in HF.
Even though considerable studies have demonstrated that quite a few amino acids are useful for HF outcomes, there are some studies that evaluate how effective the amino acids are for HF treatment. There is one RCT study indicating that high-caloric protein-rich oral supplement has significant clinical benefits in terms of body composition and quality of life in CHF (38). Another RCT shows that supplementation of L-alanyl-L-glutamine and PUFA have the same effects in the CHF patients (39). However, BCAA supplementation has no improvements on physical and functional capacities in patients with HF (40). In one experimental study, combing β-alanine and histidine with exercise could elevate functional capacity and maximum strength in rats with CHF (84). Although it has been proven that glycine could reduce cardiac fibrosis in HF mice, its cardioprotective role remains to be validated in clinical trials.
Conclusions
Overall, current clinical studies have demonstrated the connection among gut microbiota, the circulating amino acids, and HF, the graphic abstract is shown in Figure 1. A number of AAs have been proven to serve as a diagnostic and prognostic biomarker in HF. Besides, several AAs are tested to show the positive effects of improving cardiac function in HF. Nevertheless, these results may not be adequate to elucidate the cause and effect between gut microbiota and HF. Further mechanism studies and clinical trials are needed to evaluate the gut–amino acid–HF axis as a potential therapeutic target for HF and to develop a deeper understanding of this axis in the future.
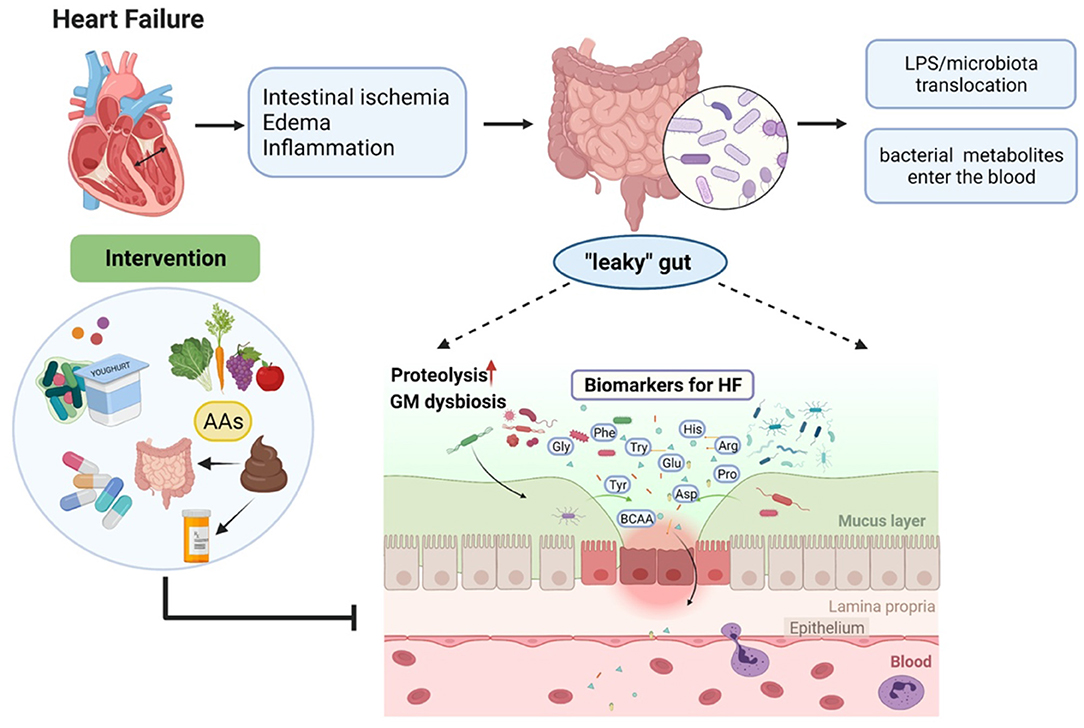
Figure 1. Heart failure (HF)-caused gut microbiota dysbiosis leads to metabolite disorders and therapeutic interventions.
This review has pivotally presented the evidence of the gut–amino acid–HF axis as a whole and contributed in several ways for our understanding of HF. However, these findings and conclusions are limited by the quality and quantity of the relative research. Therefore, it is recommended that further research should be undertaken deeply in this axis.
Author Contributions
GT, ZY, and YW designed and executed the review. GT, MG, XQ, BL, CW, HW, and JS drafted the manuscript, figure, and table. All authors contributed to the article and approved the submitted version.
Funding
This work was supported by the National Natural Science Foundation of China (81800390).
Conflict of Interest
The authors declare that the research was conducted in the absence of any commercial or financial relationships that could be construed as a potential conflict of interest.
Publisher's Note
All claims expressed in this article are solely those of the authors and do not necessarily represent those of their affiliated organizations, or those of the publisher, the editors and the reviewers. Any product that may be evaluated in this article, or claim that may be made by its manufacturer, is not guaranteed or endorsed by the publisher.
Acknowledgments
The figure was created with BioRender software, Biorender.com.
Abbreviations
HF, heart failure; GM, gut microbiota; SCFA, short-chain fatty acid; BA, bile acid; TMAO, trimethylamine N-oxide; AA, amino acid; IPA, indolepropionic acid; BCAA, branched-chain amino acid; KP, kynurenine pathway; 5-HT, serotonin; LV: left ventricular, ; DASH: Dietary Approaches to Stop Hypertension, ; FMT, fecal microbiota transplant.
References
1. Benjamin EJ, Virani SS, Callaway CW, Chamberlain AM, Chang AR, Cheng S, et al. Heart Disease and Stroke Statistics-2018 Update: A Report From the American Heart Association. Circulation. (2018) 137:e67–e492. doi: 10.1161/cir.0000000000000558
2. Targher G, Dauriz M, Laroche C, Temporelli PL, Hassanein M, Seferovic PM, et al. In-hospital and 1-year mortality associated with diabetes in patients with acute heart failure: results from the ESC-HFA Heart Failure Long-Term Registry. Eur J Heart Fail. (2017) 19:54–65. doi: 10.1002/ejhf.679
3. Chioncel O, Mebazaa A, Harjola VP, Coats AJ, Piepoli MF, Crespo-Leiro MG, et al. Clinical phenotypes and outcome of patients hospitalized for acute heart failure: the ESC Heart Failure Long-Term Registry. Eur J Heart Fail. (2017) 19:1242–54. doi: 10.1002/ejhf.890
4. Ibrahim NE, Januzzi JL Jr. Established and emerging roles of biomarkers in heart failure. Circ Res. (2018) 123:614–29. doi: 10.1161/circresaha.118.312706
5. Pasipoularides A. Implementing genome-driven personalized cardiology in clinical practice. J Mol Cell Cardiol. (2018) 115:142–57. doi: 10.1016/j.yjmcc.2018.01.008
6. Sarhene M, Wang Y, Wei J, Huang Y, Li M, Li L, et al. Biomarkers in heart failure: the past, current and future. Heart Fail Rev. (2019) 24:867–903. doi: 10.1007/s10741-019-09807-z
7. Bayes-Genis A, Liu PP, Lanfear DE, de Boer RA, Gonzalez A, Thum T, et al. Omics phenotyping in heart failure: the next frontier. European Heart Journal. (2020) 41:3477. doi: 10.1093/eurheartj/ehaa270
8. Fu J, Bonder MJ, Cenit MC, Tigchelaar EF, Maatman A, Dekens JA, et al. The gut microbiome contributes to a substantial proportion of the variation in blood lipids. Circ Res. (2015) 117:817–24. doi: 10.1161/circresaha.115.306807
9. Jie Z, Xia H, Zhong SL, Feng Q, Li S, Liang S, et al. The gut microbiome in atherosclerotic cardiovascular disease. Nat Commun. (2017) 8:845. doi: 10.1038/s41467-017-00900-1
10. Menni C, Lin C, Cecelja M, Mangino M, Matey-Hernandez ML, Keehn L, et al. Gut microbial diversity is associated with lower arterial stiffness in women. Eur Heart J. (2018) 39:2390–7. doi: 10.1093/eurheartj/ehy226
11. Zhernakova DV, Le TH, Kurilshikov A, Atanasovska B, Bonder MJ, Sanna S, et al. Individual variations in cardiovascular-disease-related protein levels are driven by genetics and gut microbiome. Nat Genet. (2018) 50:1524–32. doi: 10.1038/s41588-018-0224-7
12. Kurilshikov A, van den Munckhof ICL, Chen L, Bonder MJ, Schraa K, Rutten JHW, et al. Gut microbial associations to plasma metabolites linked to cardiovascular phenotypes and risk. Circ Res. (2019) 124:1808–20. doi: 10.1161/circresaha.118.314642
13. Witkowski M, Weeks TL, Hazen SL. Gut microbiota and cardiovascular disease. Circ Res. (2020) 127:553–70. doi: 10.1161/circresaha.120.316242
14. Tang WHW Li DY, Hazen SL. Dietary metabolism, the gut microbiome, and heart failure. Nat Rev Cardiol. (2019) 16:137–54. doi: 10.1038/s41569-018-0108-7
15. Branchereau M, Burcelin R, Heymes C. The gut microbiome and heart failure: A better gut for a better heart. Rev Endocr Metab Disord. (2019) 20:407–14. doi: 10.1007/s11154-019-09519-7
16. Mamic P, Chaikijurajai T, Tang WHW. Gut microbiome - A potential mediator of pathogenesis in heart failure and its comorbidities: State-of-the-art review. J Mol Cell Cardiol. (2021) 152:105–17. doi: 10.1016/j.yjmcc.2020.12.001
17. Li L, Zhong SJ, Hu SY, Cheng B, Qiu H, Hu ZX. Changes of gut microbiome composition and metabolites associated with hypertensive heart failure rats. BMC Microbiol. (2021) 21:141. doi: 10.1186/s12866-021-02202-5
18. Li W, Huang A, Zhu H, Liu X, Huang X, Huang Y, et al. Gut microbiota-derived trimethylamine N-oxide is associated with poor prognosis in patients with heart failure. Med J Aust. (2020) 213:374–9. doi: 10.5694/mja2.50781
19. Drapala A, Szudzik M, Chabowski D, Mogilnicka I, Jaworska K, Kraszewska K, et al. Heart Failure Disturbs Gut-Blood Barrier and Increases Plasma Trimethylamine, a Toxic Bacterial Metabolite. Int J Mol Sci. (2020) 21. doi: 10.3390/ijms21176161
20. Kitai T, Tang WHW. Gut microbiota in cardiovascular disease and heart failure. Clin Sci (Lond). (2018) 132:85–91. doi: 10.1042/cs20171090
21. Mayerhofer CCK, Ueland T, Broch K, Vincent RP, Cross GF, Dahl CP, et al. Increased secondary/primary bile acid ratio in chronic heart failure. J Card Fail. (2017) 23:666–71. doi: 10.1016/j.cardfail.2017.06.007
22. Murashige D, Jang C, Neinast M, Edwards JJ, Cowan A, Hyman MC, et al. Comprehensive quantification of fuel use by the failing and nonfailing human heart. Science. (2020) 370:364–8. doi: 10.1126/science.abc8861
23. Razquin C, Ruiz-Canela M, Toledo E, Hernández-Alonso P, Clish CB, Guasch-Ferré M, et al. Metabolomics of the tryptophan-kynurenine degradation pathway and risk of atrial fibrillation and heart failure: potential modification effect of Mediterranean diet. Am J Clin Nutr. (2021). doi: 10.1093/ajcn/nqab238. [Epub ahead of print].
24. Lu Y, Zhu X, Li J, Fang R, Wang Z, Zhang J, et al. Glycine prevents pressure overload induced cardiac hypertrophy mediated by glycine receptor. Biochem Pharmacol. (2017) 123:40–51. doi: 10.1016/j.bcp.2016.11.008
25. Pasini E, Aquilani R, Testa C, Baiardi P, Angioletti S, Boschi F, et al. Pathogenic gut flora in patients with chronic heart failure. JACC Heart Fail. (2016) 4:220–7. doi: 10.1016/j.jchf.2015.10.009
26. Arutyunov GP, Kostyukevich OI, Serov RA, Rylova NV, Bylova NA. Collagen accumulation and dysfunctional mucosal barrier of the small intestine in patients with chronic heart failure. Int J Cardiol. (2008) 125:240–5. doi: 10.1016/j.ijcard.2007.11.103
27. Sandek A, Bauditz J, Swidsinski A, Buhner S, Weber-Eibel J, von Haehling S, et al. Altered intestinal function in patients with chronic heart failure. J Am Coll Cardiol. (2007) 50:1561–9. doi: 10.1016/j.jacc.2007.07.016
28. Krack A, Sharma R, Figulla HR, Anker SD. The importance of the gastrointestinal system in the pathogenesis of heart failure. Eur Heart J. (2005) 26:2368–74. doi: 10.1093/eurheartj/ehi389
29. Gutiérrez-Calabrés E, Ortega-Hernández A, Modrego J, Gómez-Gordo R, Caro-Vadillo A, Rodríguez-Bobada C, et al. Gut microbiota profile identifies transition from compensated cardiac hypertrophy to heart failure in hypertensive rats. Hypertension. (2020) 76:1545–54. doi: 10.1161/hypertensionaha.120.15123
30. Sandek A, Swidsinski A, Schroedl W, Watson A, Valentova M, Herrmann R, et al. Intestinal blood flow in patients with chronic heart failure: a link with bacterial growth, gastrointestinal symptoms, and cachexia. J Am Coll Cardiol. (2014) 64:1092–102. doi: 10.1016/j.jacc.2014.06.1179
31. Luedde M, Winkler T, Heinsen FA, Rühlemann MC, Spehlmann ME, Bajrovic A, et al. Heart failure is associated with depletion of core intestinal microbiota. ESC Heart Fail. (2017) 4:282–90. doi: 10.1002/ehf2.12155
32. Kummen M, Mayerhofer CCK, Vestad B, Broch K, Awoyemi A, Storm-Larsen C, et al. Gut microbiota signature in heart failure defined from profiling of 2 independent cohorts. J Am Coll Cardiol. (2018) 71:1184–6. doi: 10.1016/j.jacc.2017.12.057
33. Beale AL, O'Donnell JA, Nakai ME, Nanayakkara S, Vizi D, Carter K, et al. The gut microbiome of heart failure with preserved ejection fraction. J Am Heart Assoc. (2021) 10:e020654. doi: 10.1161/jaha.120.020654
34. Cheng ML, Wang CH, Shiao MS, Liu MH, Huang YY, Huang CY, et al. Metabolic disturbances identified in plasma are associated with outcomes in patients with heart failure: diagnostic and prognostic value of metabolomics. J Am Coll Cardiol. (2015) 65:1509–20. doi: 10.1016/j.jacc.2015.02.018
35. Wang CH, Cheng ML, Liu MH. Simplified plasma essential amino acid-based profiling provides metabolic information and prognostic value additive to traditional risk factors in heart failure. Amino Acids. (2018) 50:1739–48. doi: 10.1007/s00726-018-2649-9
36. Wang CH, Cheng ML, Liu MH, Fu TC. Amino acid-based metabolic profile provides functional assessment and prognostic value for heart failure outpatients. Dis Markers. (2019) 2019:8632726. doi: 10.1155/2019/8632726
37. Chen WS, Wang CH, Cheng CW, Liu MH, Chu CM, Wu HP, et al. Elevated plasma phenylalanine predicts mortality in critical patients with heart failure. ESC Heart Fail. (2020) 7:2884–93. doi: 10.1002/ehf2.12896
38. Rozentryt P, von Haehling S, Lainscak M, Nowak JU, Kalantar-Zadeh K, Polonski L, et al. The effects of a high-caloric protein-rich oral nutritional supplement in patients with chronic heart failure and cachexia on quality of life, body composition, and inflammation markers: a randomized, double-blind pilot study. J Cachexia Sarcopenia Muscle. (2010) 1:35–42. doi: 10.1007/s13539-010-0008-0
39. Wu C, Kato TS Ji R, Zizola C, Brunjes DL, Deng Y, et al. Supplementation of l-Alanyl-l-glutamine and fish oil improves body composition and quality of life in patients with chronic heart failure. Circ Heart Fail. (2015) 8:1077–87. doi: 10.1161/circheartfailure.115.002073
40. Pineda-Juárez JA, Sánchez-Ortiz NA, Castillo-Martínez L, Orea-Tejeda A, Cervantes-Gaytán R, Keirns-Davis C, et al. Changes in body composition in heart failure patients after a resistance exercise program and branched chain amino acid supplementation. Clin Nutr. (2016) 35:41–7. doi: 10.1016/j.clnu.2015.02.004
41. Zhou W, Cheng Y, Zhu P, Nasser MI, Zhang X, Zhao M. Implication of gut microbiota in cardiovascular diseases. Oxid Med Cell Longev. (2020) 2020:5394096. doi: 10.1155/2020/5394096
42. Lin R, Liu W, Piao M, Zhu H A. review of the relationship between the gut microbiota and amino acid metabolism. Amino Acids. (2017) 49:2083–90. doi: 10.1007/s00726-017-2493-3
43. Davila AM, Blachier F, Gotteland M, Andriamihaja M, Benetti PH, Sanz Y, et al. Intestinal luminal nitrogen metabolism: role of the gut microbiota and consequences for the host. Pharmacol Res. (2013) 68:95–107. doi: 10.1016/j.phrs.2012.11.005
44. Dai ZL, Zhang J, Wu G, Zhu WY. Utilization of amino acids by bacteria from the pig small intestine. Amino Acids. (2010) 39:1201–15. doi: 10.1007/s00726-010-0556-9
45. Bergen WG, Wu G. Intestinal nitrogen recycling and utilization in health and disease. J Nutr. (2009) 139:821–5. doi: 10.3945/jn.109.104497
46. Macfarlane GT, Cummings JH, Allison C. Protein degradation by human intestinal bacteria. J Gen Microbiol. (1986) 132:1647–56. doi: 10.1099/00221287-132-6-1647
47. Dai ZL, Wu G, Zhu WY. Amino acid metabolism in intestinal bacteria: links between gut ecology and host health. Front Biosci (Landmark Ed). (2011) 16:1768–86. doi: 10.2741/3820
48. Dodd D, Spitzer MH, Van Treuren W, Merrill BD, Hryckowian AJ, Higginbottom SK, et al. A gut bacterial pathway metabolizes aromatic amino acids into nine circulating metabolites. Nature. (2017) 551:648–52. doi: 10.1038/nature24661
49. Zelante T, Iannitti RG, Cunha C, De Luca A, Giovannini G, Pieraccini G, et al. Tryptophan catabolites from microbiota engage aryl hydrocarbon receptor and balance mucosal reactivity via interleukin-22. Immunity. (2013) 39:372–85. doi: 10.1016/j.immuni.2013.08.003
50. Ussher JR, Elmariah S, Gerszten RE, Dyck JR. The emerging role of metabolomics in the diagnosis and prognosis of cardiovascular disease. J Am Coll Cardiol. (2016) 68:2850–70. doi: 10.1016/j.jacc.2016.09.972
51. Moura A, Savageau MA, Alves R. Relative amino acid composition signatures of organisms and environments. PLoS ONE. (2013) 8:e77319. doi: 10.1371/journal.pone.0077319
52. Neinast M, Murashige D, Arany Z. Branched chain amino acids. Annu Rev Physiol. (2019) 81:139–64. doi: 10.1146/annurev-physiol-020518-114455
53. Sun H, Olson KC, Gao C, Prosdocimo DA, Zhou M, Wang Z, et al. Catabolic defect of branched-chain amino acids promotes heart failure. Circulation. (2016) 133:2038–49. doi: 10.1161/circulationaha.115.020226
54. Wang W, Zhang F, Xia Y, Zhao S, Yan W, Wang H, et al. Defective branched chain amino acid catabolism contributes to cardiac dysfunction and remodeling following myocardial infarction. Am J Physiol Heart Circ Physiol. (2016) 311:H1160–h1169. doi: 10.1152/ajpheart.00114.2016
55. Li T, Zhang Z, Kolwicz SC Jr, Abell L, Roe ND, Kim M, et al. Defective branched-chain amino acid catabolism disrupts glucose metabolism and sensitizes the heart to ischemia-reperfusion injury. Cell Metab. (2017) 25:374–85. doi: 10.1016/j.cmet.2016.11.005
56. Alexander D, Lombardi R, Rodriguez G, Mitchell MM, Marian AJ. Metabolomic distinction and insights into the pathogenesis of human primary dilated cardiomyopathy. Eur J Clin Invest. (2011) 41:527–38. doi: 10.1111/j.1365-2362.2010.02441.x
57. Tenori L, Hu X, Pantaleo P, Alterini B, Castelli G, Olivotto I, et al. Metabolomic fingerprint of heart failure in humans: a nuclear magnetic resonance spectroscopy analysis. Int J Cardiol. (2013) 168:e113–5. doi: 10.1016/j.ijcard.2013.08.042
58. Hunter WG, Kelly JP, McGarrah RW III, Kraus WE, Shah SH. Metabolic dysfunction in heart failure: diagnostic, prognostic, and pathophysiologic insights from metabolomic profiling. Curr Heart Fail Rep. (2016) 13:119–31. doi: 10.1007/s11897-016-0289-5
59. Wang CH, Cheng ML, Liu MH. Amino acid-based metabolic panel provides robust prognostic value additive to b-natriuretic peptide and traditional risk factors in heart failure. Dis Markers. (2018) 2018:3784589. doi: 10.1155/2018/3784589
60. Konishi M, Ebner N, Springer J, Schefold JC, Doehner W, Dschietzig TB, et al. Impact of plasma kynurenine level on functional capacity and outcome in heart failure - results from studies investigating co-morbidities aggravating heart failure (SICA-HF). Circ J. (2016) 81:52–61. doi: 10.1253/circj.CJ-16-0791
61. Kasahara K, Rey FE. The emerging role of gut microbial metabolism on cardiovascular disease. Curr Opin Microbiol. (2019) 50:64–70. doi: 10.1016/j.mib.2019.09.007
62. Lund A, Nordrehaug JE, Slettom G, Solvang SH, Pedersen EK, Midttun Ø, et al. Plasma kynurenines and prognosis in patients with heart failure. PLoS ONE. (2020) 15:e0227365. doi: 10.1371/journal.pone.0227365
63. Janssen W, Schymura Y, Novoyatleva T, Kojonazarov B, Boehm M, Wietelmann A, et al. 5-HT2B receptor antagonists inhibit fibrosis and protect from RV heart failure. Biomed Res Int. (2015) 2015:438403. doi: 10.1155/2015/438403
64. Liu MY, Zhang LJ. [Role of Ginkgo biloba extract in regulating 5-hydroxytrytamine and its receptor in heart failure mice]. Zhonghua Yi Xue Za Zhi. (2018) 98:2024–9. doi: 10.3760/cma.j.issn.0376-2491.2018.25.012
65. Snider JC, Riley LA, Mallory NT, Bersi MR, Umbarkar P, Gautam R, et al. Targeting 5-HT(2B) receptor signaling prevents border zone expansion and improves microstructural remodeling after myocardial infarction. Circulation. (2021) 143:1317–30. doi: 10.1161/circulationaha.120.051517
66. Drake KJ, Sidorov VY, McGuinness OP, Wasserman DH, Wikswo JP. Amino acids as metabolic substrates during cardiac ischemia. Exp Biol Med (Maywood). (2012) 237:1369–78. doi: 10.1258/ebm.2012.012025
67. Zhang X, Liu H, Gao J, Zhu M, Wang Y, Jiang C, et al. Metabolic disorder in the progression of heart failure. Sci China Life Sci. (2019) 62:1153–67. doi: 10.1007/s11427-019-9548-9
68. Gundersen RY, Vaagenes P, Breivik T, Fonnum F, Opstad PK. Glycine–an important neurotransmitter and cytoprotective agent. Acta Anaesthesiol Scand. (2005) 49:1108–16. doi: 10.1111/j.1399-6576.2005.00786.x
69. Van den Eynden J, Ali SS, Horwood N, Carmans S, Brône B, Hellings N, et al. Glycine and glycine receptor signalling in non-neuronal cells. Front Mol Neurosci. (2009) 2:9. doi: 10.3389/neuro.02.009.2009
70. Lam V, Su J, Koprowski S, Hsu A, Tweddell JS, Rafiee P, et al. Intestinal microbiota determine severity of myocardial infarction in rats. FASEB J. (2012) 26:1727–35. doi: 10.1096/fj.11-197921
71. Gan XT, Ettinger G, Huang CX, Burton JP, Haist JV, Rajapurohitam V, et al. Probiotic administration attenuates myocardial hypertrophy and heart failure after myocardial infarction in the rat. Circ Heart Fail. (2014) 7:491–9. doi: 10.1161/circheartfailure.113.000978
72. Zhang Y, Wang Y, Ke B, Du J, TMAO. how gut microbiota contributes to heart failure. Transl Res. (2021) 228:109–25. doi: 10.1016/j.trsl.2020.08.007
73. Conraads VM, Jorens PG, De Clerck LS, Van Saene HK, Ieven MM, Bosmans JM, et al. Selective intestinal decontamination in advanced chronic heart failure: a pilot trial. Eur J Heart Fail. (2004) 6:483–91. doi: 10.1016/j.ejheart.2003.12.004
74. Tang WH, Wang Z, Levison BS, Koeth RA, Britt EB, Fu X, et al. Intestinal microbial metabolism of phosphatidylcholine and cardiovascular risk. N Engl J Med. (2013) 368:1575–84. doi: 10.1056/NEJMoa1109400
75. Yancy CW, Jessup M, Bozkurt B, Butler J, Casey DE Jr, Drazner MH, et al. 2013 ACCF/AHA guideline for the management of heart failure: executive summary: a report of the American College of Cardiology Foundation/American Heart Association Task Force on practice guidelines. Circulation. (2013) 128:1810–52. doi: 10.1161/CIR.0b013e31829e8807
76. Levitan EB, Wolk A, Mittleman MA. Consistency with the DASH diet and incidence of heart failure. Arch Intern Med. (2009) 169:851–7. doi: 10.1001/archinternmed.2009.56
77. Salehi-Abargouei A, Maghsoudi Z, Shirani F, Azadbakht L. Effects of Dietary Approaches to Stop Hypertension (DASH)-style diet on fatal or nonfatal cardiovascular diseases–incidence: a systematic review and meta-analysis on observational prospective studies. Nutrition. (2013) 29:611–8. doi: 10.1016/j.nut.2012.12.018
78. Abu-Sawwa R, Dunbar SB, Quyyumi AA, Sattler ELP. Nutrition intervention in heart failure: should consumption of the DASH eating pattern be recommended to improve outcomes? Heart Fail Rev. (2019) 24:565–73. doi: 10.1007/s10741-019-09781-6
79. Kerley CP. Dietary patterns and components to prevent and treat heart failure: a comprehensive review of human studies. Nutr Res Rev. (2019) 32:1–27. doi: 10.1017/s0954422418000148
80. Aaron L, Christian S, Torsten M. Feed your microbiome and your heart: The gut-heart axis. Front Biosci (Landmark Ed). (2021) 26:468–77. doi: 10.2741/4902
81. Vrieze A, Van Nood E, Holleman F, Salojärvi J, Kootte RS, Bartelsman JF, et al. Transfer of intestinal microbiota from lean donors increases insulin sensitivity in individuals with metabolic syndrome. Gastroenterology. (2012) 143:913–6.e7. doi: 10.1053/j.gastro.2012.06.031
82. Zellmer C, De Wolfe TJ, Van Hoof S, Blakney R, Safdar N. Patient perspectives on fecal microbiota transplantation for clostridium difficile infection. Infect Dis Ther. (2016) 5:155–64. doi: 10.1007/s40121-016-0106-1
83. Chehoud C, Dryga A, Hwang Y, Nagy-Szakal D, Hollister EB, Luna RA, et al. Transfer of viral communities between human individuals during fecal microbiota transplantation. MBio. (2016) 7:e00322. doi: 10.1128/mBio.00322-16
Keywords: heart failure, gut microbiota, amino acids, metabolism, cardiometabolic disorders
Citation: Tuerhongjiang G, Guo M, Qiao X, Lou B, Wang C, Wu H, Wu Y, Yuan Z and She J (2021) Interplay Between Gut Microbiota and Amino Acid Metabolism in Heart Failure. Front. Cardiovasc. Med. 8:752241. doi: 10.3389/fcvm.2021.752241
Received: 02 August 2021; Accepted: 07 September 2021;
Published: 21 October 2021.
Edited by:
Chen Liu, The First Affiliated Hospital of Sun Yat-sen University, ChinaReviewed by:
Xiaolei Yang, Dalian Medical University, ChinaPaulo M. Dourado, University of São Paulo, Brazil
Copyright © 2021 Tuerhongjiang, Guo, Qiao, Lou, Wang, Wu, Wu, Yuan and She. This is an open-access article distributed under the terms of the Creative Commons Attribution License (CC BY). The use, distribution or reproduction in other forums is permitted, provided the original author(s) and the copyright owner(s) are credited and that the original publication in this journal is cited, in accordance with accepted academic practice. No use, distribution or reproduction is permitted which does not comply with these terms.
*Correspondence: Jianqing She, amlhbnFpbmdzaGVAeGp0dS5lZHUuY24=; Zuyi Yuan, enV5aXl1YW5AbWFpbC54anR1LmVkdS5jbg==; Yue Wu, eXVlLnd1QHhqdHUuZWR1LmNu