- 1Department of Radiology, Guangdong Provincial People's Hospital, Guangdong Academy of Medical Sciences, Guangzhou, China
- 2College of Medicine, Shantou University, Shantou, China
Coronary computed tomography angiography (CCTA) is a comprehensive, non-invasive and cost-effective imaging assessment approach, which can provide the ability to identify the characteristics and morphology of high-risk atherosclerotic plaques associated with acute coronary syndrome (ACS). The development of CCTA and latest advances in emerging technologies, such as computational fluid dynamics (CFD), have made it possible not only to identify the morphological characteristics of high-risk plaques non-invasively, but also to assess the hemodynamic parameters, the environment surrounding coronaries and so on, which may help to predict the risk of ACS. In this review, we present how CCTA was used to characterize the composition and morphology of high-risk plaques prone to ACS and the current role of CCTA, including emerging CCTA technologies, advanced analysis, and characterization techniques in prognosticating the occurrence of ACS.
Introduction
Acute coronary syndrome (ACS) may be the first manifestation of coronary artery disease (CAD), mainly caused by the rupture or erosion of unstable plaques (1–4), which is the leading cause of death for most of the world's population. Therefore, it has been a driving force to identify these high-risk plaques prone to rupture which may lead to ACS. Substantial study efforts have confirmed that virtual histology intravascular ultrasound (IVUS) or optical coherence tomography (OCT) can be valuable (5).
However, these invasive diagnostic approaches with low positive predictive value and unclear cost-effectiveness have not been widely used in clinical practice (6). Coronary computed tomography angiography (CCTA), as a comprehensive non-invasive imaging assessment approach, which allows for the quantification and characterization of coronary atherosclerosis, can effectively evaluate the condition of all coronary arteries and the branches in the whole-heart, and has great clinical application value in identifying adverse plaque characteristics (7, 8). The high accuracy and high efficiency of CCTA are well-confirmed in previous studies, as well as its higher diagnostic performance compared with invasive reference standards (7, 9, 10). CCTA has been used to identify the characteristics and morphology of high-risk atherosclerotic plaques associated with ACS in previous studies, including positive remodeling, low attenuation, spotty calcification, and the napkin-ring sign (8, 10, 11). The development of CCTA and latest advances in emerging technologies, such as computational fluid dynamics (CFD), have made it possible to simultaneously perform the comprehensive evaluation of anatomical severity degree, lesion geometry, plaque characteristics, quantification of hemodynamic parameters and detection of vascular inflammation, which may help to identify the high-risk plaques and predict the risk of ACS (12).
In this review, we delineate the current understanding of the pathology of the atherosclerotic plaques associated with ACS and corresponding manifestations on CCTA in clinical practice. The application of CCTA in characterizing the composition and morphology of high-risk plaques prone to ACS and prognosticating the occurrence of ACS are further described. Finally, the progress in emerging CCTA technologies, advanced analysis and characterization techniques are also reviewed. The new techniques can provide the comprehensive assessment of high-risk plaques and surrounding environment, and may provide personalized risk assessment of future ACS events and further guide clinical decision-making.
From High-Risk Plaque Histopathology to CCTA
The detection and characterization of plaque by CCTA is based on histopathology, therefore, and therefore it is vital to grasp the histopathological characteristics and evolution process of high-risk atherosclerotic plaque.
Atherosclerosis, most often observed at the branch points and low shear stress areas of blood vessels, is a multifactorial systemic disease and has a chronic and progressive process (13). About two-thirds of ACS results from the rupture of atherosclerotic plaque (14, 15). The early manifestations of atherosclerosis are non-atherosclerotic intimal lesions, which include intimal thickening and xanthoma. Subsequently, starting from pathological intimal thickening, they further develop into increasingly vulnerable and rupture-prone lesions, and progress into fibroatheroma or even thin cap fibroatheroma, which is considered to be the precursor of plaque rupture (16). Moreover, the development of lesions before rupture can be explained by the histopathological nature of unstable lesions, including intraplaque hemorrhage, neo-vascularization, plaque healing, and recurrent rupture (17, 18). Compared with plaque erosion and stable CAD, intraplaque hemorrhage is the most common finding in plaque rupture, which includes numerous foam cells (lipid-laden macrophage), cholesterol clefts, and an expanding necrotic core, contributing to the vulnerability of plaque (19).
The characteristics of high-risk plaques are related to the vulnerability of plaques (18). Based on the histopathologic composition of vulnerable plaques, including thin cap fibroatheroma, macrophage infiltration, and necrotic core (20, 21), the corresponding typical manifestations of high-risk plaques in CCTA are listed as follows: positive remodeling, low attenuation, spotty calcification, and napkin-ring sign (22–24) (Figure 1).
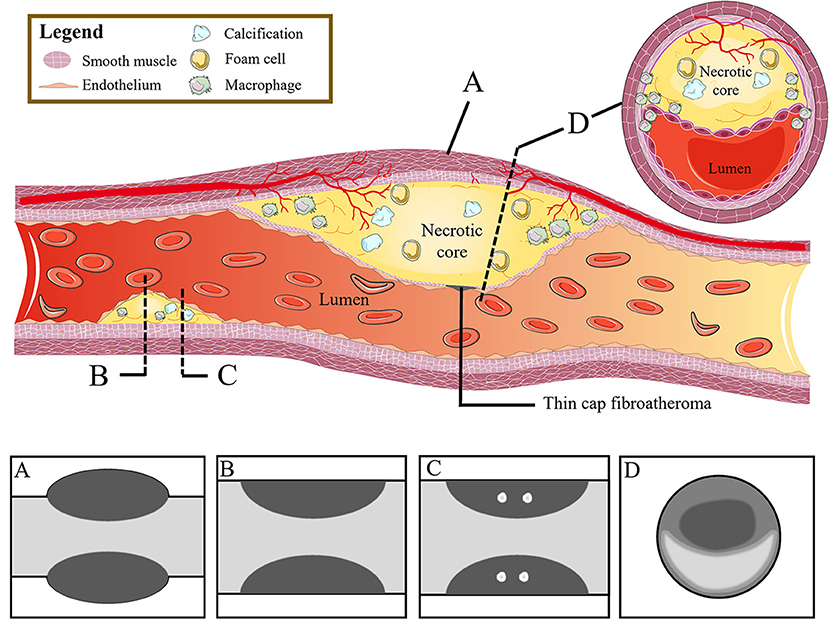
Figure 1. From high-risk plaque histopathology to CCTA. The figure shows the histopathologic components of vulnerable plaques (colored illustrations) and the corresponding typical CT features of high-risk plaques: (A) positive remodeling, (B) low attenuation, (C) spotty calcification, and (D) napkin-ring sign. CCTA, coronary computed tomography angiography.
From High-Risk Plaque CCTA Features to Acute Coronary Syndrome
CCTA Assessment of High-Risk Plaque Features
The percentage of stenosis is important information on CCTA. When coronary heart disease cannot be ruled out clinically, the percentage of stenosis on CCTA can help to rule out patients in stable condition and with low possibility of coronary heart disease (25–27). However, the main cause of ACS is plaque rupture and erosion rather than fixed stenosis. Given that plaque instability and plaque progression are important factors leading to subsequent acute coronary events, identifying high-risk plaque characteristics is necessary (17, 25, 28–31). As a comprehensive non-invasive imaging assessment approach, CCTA has been used to identify the morphological characteristics of high-risk plaques prone to rupture that may lead to ACS (32–35).
Positive Remodeling
Clinically, coronary artery remodeling refers to be the compensatory changes of cross-sectional area and structure of coronary artery in the progression of coronary atherosclerosis. In pathological findings, the lumen of some coronary arteries was found to be increased during atherogenesis in autopsy (36). For in vivo detection of coronary artery, IVUS examination confirmed that the cross-sectional area of the vessel at the atherosclerotic site was significantly larger than that at the proximal reference segment, then the concept of positive remodeling was proposed which refers to the compensatory increase of vessel wall when atherosclerotic plaque volume increases continuously, thus maintaining the effective area in the lumen (37). While on CT, the outer vessel wall dimension could be measured. The remodeling index (RI) is calculated by dividing the vessel cross-sectional area/diameter of the largest stenosis (or maximum vessel area/diameter) by the average cross-sectional area/diameter of the proximal and distal reference segments (7, 38, 39) (Figure 2Aa). At present, positive remodeling is generally defined as RI ≥ 1.1 in CCTA (8, 40, 41), while some researchers prefer other cut-off point (42, 43). In addition, automatic software makes it easier to quantify the RI (44).
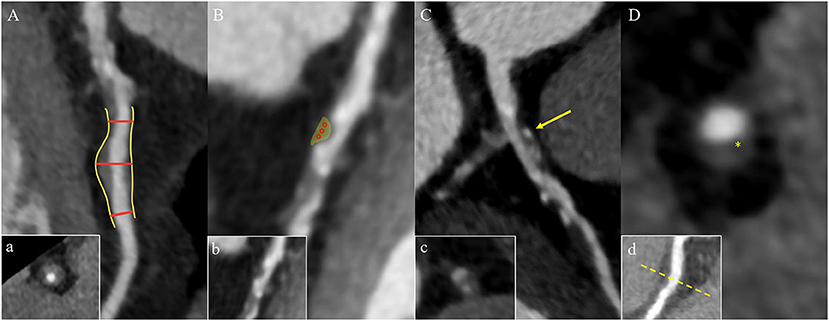
Figure 2. High-risk plaque characteristics on CCTA. (A) Positive remodeling of a non-calcified plaque in the proximal left anterior descending coronary artery. The two short red lines indicate the vessel diameters of the proximal and distal of the plaque (both 1.0 mm), and the long red line indicates the maximum vessel diameter in the middle of the plaque (1.6 mm). The remodeling index is 1.6. Picture a is the cross section of picture (A). (B) A low-attenuation plaque (yellow area) in the mid segment of the left anterior descending coronary artery with a mean CT attenuation value of 21 HU in the three regions of interest (red circles). Picture b is the original picture (B). (C) Spotty calcification of a partially calcified plaque surrounded by non-calcified components in the proximal left anterior descending coronary artery with a diameter <3 mm in all directions (yellow arrow). Picture c is the cross section of picture (C). (D) A napkin-ring sign plaque in the proximal right coronary artery. The yellow star shows the central area of the plaque with a low HU close to the lumen, which is surrounded by the peripheral edge of higher CT attenuation. Picture d is the multiplanar reconstructed image of picture (D). CCTA, coronary computed tomography angiography; HU, Hounsfield units.
Low Attenuation
The composition of plaques can be reflected by CT attenuation value, with the highest CT attenuation value for calcification, followed by fibrous tissue, and the lowest CT value for lipid. Low-attenuation plaques refer to those with the lowest CT attenuation value and the most easily ruptured lipid composition (a lipid-rich necrotic core), which is defined as mean attenuation <30 Hounsfield units (HU) of at least three regions of interest (ROIs) in general (39, 45) (Figure 2Bb). However, the CT attenuation value of lipid plaques overlaps with that of fibrous plaques, so it is difficult to distinguish the plaques only by CT attenuation value alone. In addition, the CT value of plaques is affected by many factors, such as contrast agent, plaque volume, slice thickness, tube voltage, and so on. Therefore, the current research mainly relies on special procedures to identify which plaque is low-attenuation (7, 45).
Spotty Calcification
Spotty calcification is the initial state of calcification. Since calcification is one of the consequences of local inflammation, spotty calcification may indicate active local inflammation. Mechanical stimulation on fibrous cap caused by spotty calcification and local inflammation may lead to the plaque with spotty calcification easy to rupture, thereby accelerating disease progression (46–48). Therefore, spotty calcification is considered to be one of the characteristics of high-risk plaques. In CCTA, spotty calcification is generally manifested as calcification in plaques with a density of more than 130 HU and a diameter of <3 mm surrounded by non-calcified components (42, 49, 50) (Figure 2Cc). However, only calcification more than 0.5 mm in diameter is visible on CT, so nearly two-thirds of the calcifications cannot be recognized on CT (51, 52).
Napkin-Ring Sign
The napkin ring sign is a qualitative plaque feature that can be defined by the presence of two features on the cross section of non-calcified plaques: the low-attenuation central area obviously contacting with the coronary artery lumen and the annular high attenuation plaque tissue surrounding the central area (7, 34) (Figure 2Dd). Histologically, the low-density area corresponds to the large necrotic nucleus, while the “annular” outer area is associated with fibrous tissue. The necrotic core area in plaque with the napkin-ring sign may be more than twice that without napkin ring sign (1.10 vs. 0.46 mm2) (53) corresponding to some studies' indications that a necrotic core area >1 mm2 when plaque is prone to rupture (14). The density of the ring is greater than that of the inner core but <130 HU in CT scans. Currently, the napkin-ring sign is considered to be a special CT feature of plaque with a large necrotic core, and it is a reliable marker of plaque instability (7, 14).
High-Risk Plaque Features on CCTA in Prediction of Acute Coronary Syndrome
Although the risk of plaque instability increases with the degree of coronary stenosis, most of the culprit lesions found in ACS are considered non-obstructive before rupture. Previous studies indicated that the characteristics of high-risk plaques identified by CCTA have a prognostic value independent of stenosis and atherosclerotic burden (41) (see Table 1).
In 2007, Motoyama et al. found that compared with SA lesions, the frequency of positive remodeling, non-calcified plaques or low-attenuation plaques (<30 HU) and spotty calcification on CCTA was higher in ACS culprit lesions, and these three characteristics could independently predict the occurrence of ACS. Moreover, among the three plaque features, positive remodeling has the highest accuracy in ACS prediction (22). In addition, an increase in the RI of culprit lesions in ACS patients compared with stable plaques in ACS and stable angina (SA) patients was also observed in other cross-sectional studies (11, 43, 54, 55).
In a subsequent study, Ozaki et al. compared the characteristics of lesions with intact fibrous caps and ruptured fibrous cap in ACS patients. The results revealed 88% of ruptured plaques had low-attenuation characteristics (P = 0.01) (56). These results were consistent with previous studies (43, 54, 55). Recently, a post-mortem multiple comparison analysis based on the SCOT-HEART study which included 1,769 patients with median follow up of 4.7 years identified the predictive effect of low-attenuation plaques on future cardiovascular events. They found that 4% was the cut-off value of low-attenuation plaque load as well as the strongest predictor of myocardial infarction. The predictive effect was independent of cardiovascular risk factors, calcification score and percentage of stenosis (45).
In a number of cross-sectional studies, investigators found that spotty calcification was associated with culprit lesions in ACS (49–52, 55). Compared with ACS, large calcification (≥3 mm) was more common in SA (22, 43). However, the predictive value of spotty calcification for plaque rupture is still controversial due to the huge heterogeneity in results (57). In addition, the definition and pathophysiological role of spotty calcification were questioned in studies using other imaging technologies such as OCT (58).
On CCTA, another manifestation of high-risk plaques is napkin ring sign. In a prospective study, Otsuka et al. recruited 895 patients and found that the hazard ratio (HR) of ACS in patients with the napkin ring sign was 5.55 [95% confidence interval (CI), 2.0–14.70; P < 0.001], which indicated napkin ring sign was an independent predictor of ACS (34). In a long-term follow-up study using ACS as one of the primary points, a strong prognostic implication of napkin-ring sign was also demonstrated by Feuchtner et al. (59).
Combining the characteristics of high-risk plaques could better predict the risk of ACS in the future (10, 42). The result of another study, which included 1,059 patients with follow-up for 27 ± 10 months also showed that PR or low-attenuation plaques, especially in combination, was independent predictors of future ACS events (49). Subsequently, this result was confirmed in a follow-up study (8). Furthermore, in the post-hoc analysis of the SCOT-HEART study, the adverse plaque was defined as a plaque with one or more of the features above observed. The results revealed that patients with adverse plaques had three times higher risk of myocardial infarction than patients without adverse plaques, and the prediction effect was stronger when combined with the presence of stenosis (23).
Although there remain some controversies regarding the definition of high-risk plaques on CCTA and the predictive efficacy of different high-risk plaque characteristics in future ACS events to date (5, 8, 25, 41, 60–63), the correlation between the existence of high-risk plaques and the occurrence of future ACS events is generally obtained.
Emerging CCTA Technologies and Acute Coronary Syndrome
The emerging technologies have promoted the clinical application of CCTA. Right now, CCTA can not only identify the morphological characteristics of high-risk plaques non-invasively, but also enable the intracoronary and extracoronary imaging, so as to realize the comprehensive assessment of high-risk plaques and surrounding environment, which may provide personalized risk assessment of future ACS events and further guide clinical decision-making (12).
Computational Fluid Dynamics and Acute Coronary Syndrome
Plaque rupture happens when the stress inside the plaque exceeds its strength (64), while rupture of plaques is a complex biomechanical process, which is affected by the plaque structure and composition, external forces, and hemodynamic factors. Even if the plaque manifests the same vulnerable characteristics, the risk of rupture is different due to the heterogeneity of hemodynamic press exerting on the plaque (13, 65). Therefore, in addition to the assessment of plaque characteristics, accurate evaluation of plaque hemodynamic parameters is also vital for the identification of high-risk plaques and the prediction of ACS risk.
Fractional flow reserve (FFR) is the ratio of the pressure at the distal end of the stenosis to the pressure at the proximal end of the normal vessel in a state of maximum hyperemic condition. In recent years, FFR derived from CCTA (FFRCT) has used advanced fluid dynamics analysis method which combines the advantages of CCTA and traditional FFR (Figure 3). It is an image post-processing technology that applies hemodynamics to CCTA examination, which uses conventional standardized CCTA image data to evaluate the hemodynamic differences of coronary artery stenosis (66). Assuming that blood is an incompressible Newtonian fluid with constant density and viscosity, the flow and pressure in the coronary model volume can be calculated by the Navier-Stokes equations (12, 58). Three prospective clinical trials (67–69) all have verified that FFRCT can evaluate coronary artery stenosis from both anatomy and function. In addition, it has avoided the invasive operations of traditional FFR and the complications such as coronary artery tearing, bleeding, arrhythmia, myocardial infarction, and so on (70). This “one-stop” technology can fundamentally avoid unnecessary coronary angiography and revascularization. Moreover, it can provide more information for clinical practice and has the potential to replace other traditional methods recommended in clinical guidelines. As a long-term gatekeeper to guide revascularization, FFRCT is a new hot spot in clinical research (69, 71, 72).
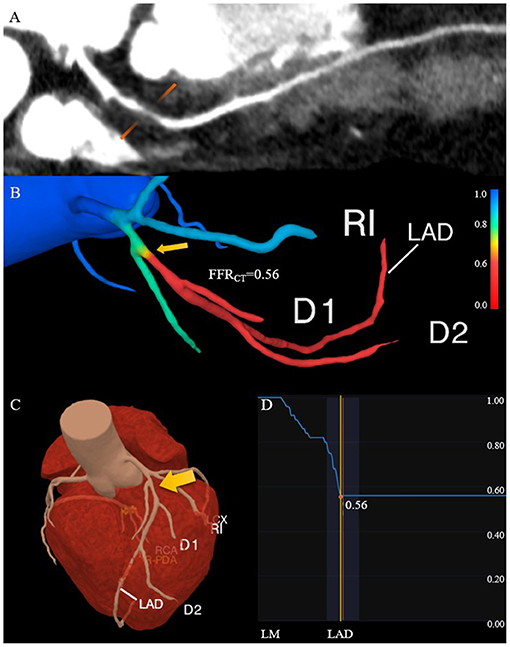
Figure 3. Comprehensive assessment of the characteristics of high-risk plaque with CCTA. (A) The lesion in the proximal of the left anterior descending coronary artery has positive remodeling and low attenuation. (B) The FFRCT map derived from computational fluid dynamics shows a value of 0.56 distal to the stenosis, which indicates lesion ischemia. The transition from blue to red along the left anterior descending coronary artery indicates the decreasing trend of the FFRCT. (C) The 3D model diagram of the coronary artery, and the yellow arrow indicates the location of the high-risk plaque. (D) The curve of the FFRCT value from the proximal to distal of the left anterior descending branch. CCTA, coronary computed tomography angiography; FFRCT, fractional flow reserve derived from CCTA.
FFRCT, change in FFRCT across the lesion (ΔFFRCT), wall shear stress (WSS) and axial plaque stress are important hemodynamic parameters, which has been reported to be associated with the occurrence of adverse clinical events (13, 65, 66, 73–75). Lee et al. had demonstrated the role of these non-invasive hemodynamic parameters in identifying high-risk plaques leading to ACS by comparing adverse plaque characteristics and non-invasive hemodynamics parameters between culprit and non-culprit lesions (76). The adverse hemodynamics were defined as FFRCT ≤ 0.80, ΔFFRCT ≥ 0.06, WSS ≥ 154.7 dyn/cm2 or axial play stress ≥ 1606.6 dyn/cm2. The results showed that the FFRCT of culprit lesions was lower compared with non-culprit lesions, which was consistent with the findings of Ferencik et al. (77). While ΔFFRCT, WSS, and axial plaque stress are higher in culprit lesions, and ΔFFRCT has the highest incremental value. Moreover, Park et al. proved that ΔFFRCT has a powerful value in ACS risk prediction (78), indicating that lesion-specific hemodynamic parameters have greater influence on the occurrence of plaque rupture and ACS than that in vascular level. The value of WSS in identifying high-risk plaques has also been confirmed (79–81).
Compared with plaques with high-risk anatomical characteristics or high-risk hemodynamic characteristics, plaques with both characteristics have a significantly higher risk of progressing to ACS (HR: 3.22; 95%CI: 1.86 to 5.55; P < 0.001; and HR: 11.75; 95%CI: 2.85–48.51; P = 0.001, respectively) (76). These studies are very valuable because they identified adverse hemodynamic characteristics before the onset of ACS. On the basis of anatomic severity and adverse plaque characteristics, the non-invasive evaluation of hemodynamic parameters was added, which improved the C-index and reclassification ability in identifying the high-risk plaques that led to ACS. In the future, the evaluation of non-invasive hemodynamic parameters may improve the identification of plaques prone to rupture as well as the prediction efficiency of high-risk plaques for ACS, and help to make the risk stratification. Further studies need to explore and determine the optimal hemodynamic parameters or combination of different patients and lesion subgroups.
Pericoronary Fat and Acute Coronary Syndrome
Vascular inflammation can promote the progression of coronary atherosclerosis and vulnerable plaque rupture, leading to the occurrence of ACS (64). Epicardial pericoronary adipose tissue (PCAT) is a special type of adipose tissue. It interacts with the adjacent vascular wall, regulates the cardiovascular biological function in a paracrine manner, and changes its phenotype in response to signals from vascular walls (82–86). Antonopoulos et al. (84) studied the gene expression, histology and CT imaging of adipose tissue samples collected during cardiac surgery, and considered that the CT density of adipose tissue (usually defined as −190 to −30 HU) reflects the balance of lipid and water phase, which is a marker of adipocyte size and lipid content. Inflammatory signals released by inflamed blood vessels are directly spread to PCAT, which can induce local lipolysis and inhibit fat formation, and also increase microvascular permeability, thus promoting perivascular edema. With the decrease of lipid content and morphology in adipocytes of PCAT, the lipid phase in adipose tissue decreases and the water phase increases, resulting in different gradients of adipocytes around the coronary artery. A further study confirmed that PCAT CT attenuation measured by CCTA can detect vascular inflammation confirmed by biopsy, and the fat attenuation index (FAI) was proposed (84). Pericoronary FAI was used to track and quantify the composition changes of PCAT by evaluating the spatial changes of peripheral fat attenuation by CCTA, which was the average density of adipose tissue in the target area (84) (Figure 4), reflecting inflammatory burden of target coronary segment (A higher pericoronary FAI was associated with a higher inflammatory burden).
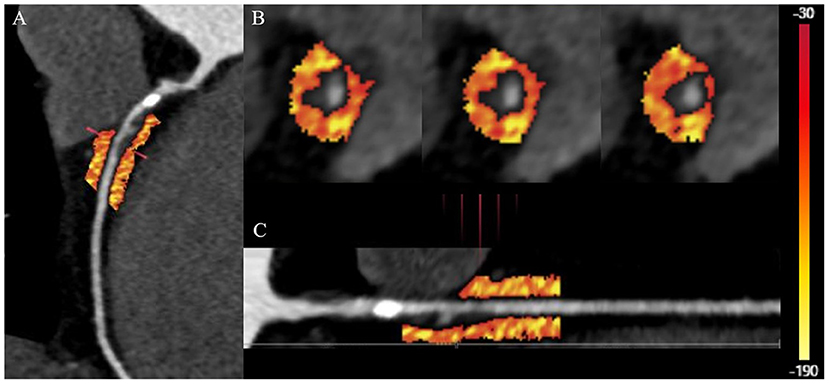
Figure 4. Quantification of PCAT CT attenuation of low-attenuation plaques in the proximal-mid right coronary artery. (A) The multiplanar reconstructed image of PCAT measured with the range from −190 to −30 HU. (B) Cross-section images of PCAT measure. (C) Straightened image of PCAT measure. PCAT, pericoronary adipose tissue; HU, Hounsfield units.
Inflammation of adipose tissue around the plaque will directly affect the formation and stability of coronary plaque. Therefore, PCAT attenuation measured by CCTA is a promising indicator for identifying high-risk plaques. In patients with ACS, cases of PCAT stranding have been reported around culprit lesions (87). Goeller et al. retrospectively recruited 19 patients with ACS and 16 patients with stable CAD (88). They found that culprit lesions were associated with increased FAI around the lesions. The frequency of pericoronary FAI ≥-68.2 HU of culprit lesions in ACS is higher, which can be used as a potential cut-off value to distinguish culprit lesions and non-culprit lesions. Therefore, combining the characteristics of high-risk plaques with the FAI around the plaques can be more reliable in identifying the high-risk plaques leading to ACS. Goeller et al. further studied the correlation between pericoronary FAI and coronary plaque progression (89). They found that the increased non-calcified plaque burden was related to the increased FAI, on the contrary, the decreased non-calcified plaque burden was related to the decreased FAI, and FAI ≥ −75 HU around the proximal end of right coronary artery (RCA) was an independent predictor of increased load of non-calcified plaques and total plaques. Therefore, PCAT is helpful to identify patients with high risk of plaque progression.
Although FAI can detect the changes of PCAT composition caused by coronary artery inflammation, it can only measure the average density of the ROI and cannot fully reflect the fine structural characteristics of the ROI. Inflammation, fibrosis, and angiogenesis are three main causes of adipose tissue dysfunction (90). Advanced imaging omics analysis can supplement the deficiency of FAI, revealing the structural changes of PCAT that cannot be recognized by the naked eye, so as to carry out more personalized assessment, and finally provide new biological insights into the pathogenesis of the disease. Recently, some researchers have verified the feasibility of PCAT image transcriptomics (91). They collected adipose tissue biopsies from 167 patients undergoing CCTA and cardiac surgery. In addition, they used image transcriptomics to correlate the gene expression of inflammation, fibrosis, and microvascular remodeling with the image omics features extracted from CT images. They proposed a new machine learning derived biomarker-fat radiomic profile (FRP), which was trained and validated to discover the PCAT imaging features related to inflammation, fibrosis, and microvascular remodeling associated with an increased cardiovascular risk. The FRP turned out to be affected by a series of factors such as scanning conditions, and the analysis was time-consuming (91). At present, a new application is being developed to automatically detect the coronary artery and pericardium, and may realize pericoronary space segmentation and feature extraction, so as to calculate FRP and FAI, and simultaneously correct the technical and anatomical information. The calculation time can be reduced to 5 min. In conclusion, PCAT imaging analysis is a new field, and its application in assessing the risk of ACS needs to be explored.
Limitations and Future Directions of CCTA in Acute Coronary Syndrome
Modern CCTA assessment provides a series of advantages by combining the coronary anatomy, plaque morphology, atherosclerotic plaque load and coronary blood flow, which allows for the evaluation of morphological and functional characteristics of high-risk plaques, and the individual risk of ACS.
In addition to CCTA, positron emission tomography (PET), magnetic resonance coronary angiography (MRCA), IVUS, OCT, and other imaging modalities are also effective methods to assess coronary atherosclerotic plaques (92–98). Notably, CCTA has irreplaceable advantages over other examinations. Firstly, CCTA can quickly provide powerful diagnostic information. Secondly, compared to coronary angiography, IVUS, and OCT, CCTA is a relatively non-invasive method with low requirements for practitioners and high universality of clinical application. In addition, CCTA has a higher spatial resolution in identifying high-risk plaque characteristics and more stable image quality than MRCA and PET. With the development of advanced hardware technology, such as photon count detectors and novel contrast agents, potential applications of multi-parameter techniques may make the plaque characterization more precise. Moreover, in previous studies, CCTA has been confirmed as a cost-effective imaging strategy (99–103).
However, there still remain certain drawbacks in CCTA. Compared with IVUS and OCT (92–98), CCTA has relatively lower spatial resolution, which hindered the detection of microscopic structure in histology (9, 104), such as the fibrous cap thickness or plaque rupture. Further CCTA studies are needed to investigate pathophysiology of rapid progression of high-risk coronary plaques leading to ACS, which will offer clinical utility on the management of patients with CAD. Recent development of intravascular imaging enables to evaluate biomechanical plaque rupture stress in vivo, offering prognostic implication of patients at higher risk of ACS (105, 106). The combination of intravascular imaging and CCTA may help development a novel methodology for plaque assessment of the probability of coronary plaque rupture. In addition, the beam hardening and related halo caused by the high attenuation structure may affect the image quality (107–109), causing reduced accuracy when identifying heavily calcified lesions. Moreover, the temporal resolution of CCTA can be affected by heart cycle as well as respiration, and it is not uncommon to find the motion artifacts in images of CCTA. Another limitation is the potential increased radiation exposure (110). While recent development of CCTA enables to reduce radiation doses by increasing the use of low-end potential scans, high-pitch scan protocols, and iterative image reconstruction. The true cardiac-capable photon-count detector will be likely available soon (111–113). The emergence of wide-detector technology, dual source X-ray and high-pitch acquisition platforms has made it possible for end-diastolic and end-systolic acquisition in a single heartbeat and with sub-millisievert dose (114–116).
Although the presence of high-risk plaque features has been widely recognized in the prediction of clinical ACS, its positive predictive value for ACS is still limited (60). In previous studies, there was a relatively long-time span between CCTA and ACS events, so the changes in drug treatment during this period have considerable heterogeneity. In addition, the clinical significance of these findings may be uncertain in the case of patients having received the effective medication. Therefore, these high-risk plaque characteristics should be further investigated in cohort studies and prospective ACS prevention trials. In addition, multicenter randomized controlled trials are needed to determine whether drug treatment and/or intervention based on high-risk plaque CCTA characteristics can improve the clinical outcomes of patients.
Recently, some technological innovations in image acquisition, post-processing and other imaging biomarkers have become more and more important, which may affect the implementation, interpretation and clinical application of CCTA. To achieve high image quality should continue to be emphasized in the future, so as to accurately apply new methods such as functional assessment and plaque quantification to CCTA imaging (117). Moreover, artificial intelligence and machine learning methods may get more attention (118–120). Currently, emerging technologies such as CCTA-based identification of high-risk plaque features and FAI have not been verified in randomized controlled trials. Therefore, in the future, further studies are needed to prove the clinical benefits of CCTA application. Furthermore, with the development of hardware and advanced analysis tools, and true clinical and cost-effectiveness data, it will continue to popularize in clinical practice (121).
Conclusion
Current findings support more attention and careful management of patients with high-risk atherosclerotic lesions, and monitoring plaque progression for individualized treatment. CCTA has an important significance in research and daily clinical practice, which allows for not only predicting the occurrence of ACS by analyzing the characteristics of high-risk plaques but also improving the predictive value in future ACS through emerging technologies such as computational fluid dynamics and evaluation of pericoronary radiation group characteristics (such as pericoronary FAI). Furthermore, combining multi-dimensional methods can comprehensively evaluate the anatomy and biology of the coronary artery, and further integrate CCTA into clinical practice. However, the identification of high-risk plaque characteristics based on CCTA and emerging technologies such as FAI have not been verified in randomized controlled trials with definite results, which requires verification of the clinical benefits of CCTA applications in the future.
Author Contributions
GL substantially planned, wrote, and revised the manuscript and made illustrates and the table. WY polished and revised the manuscript. JO and XL contributed to the collection of CT images. ZT and TL contributed to linguistic revision of the manuscript. HL substantially contributed to instruction of the writing and revision of the manuscript. All authors read and approved the final manuscript.
Funding
The work was supported by the Key R&D Program of Guangdong Province of China (No. 2018B030339001 to HL), the National Natural Science Foundation of China (No. 81974262 to HL), Guangdong Basic and Applied Basic Research Foundation (No. 2020A1515010650 to HL), and Guangdong Nurses Association Research Foundation (No. gdshsxh2021b077 to XL).
Conflict of Interest
The authors declare that the research was conducted in the absence of any commercial or financial relationships that could be construed as a potential conflict of interest.
Publisher's Note
All claims expressed in this article are solely those of the authors and do not necessarily represent those of their affiliated organizations, or those of the publisher, the editors and the reviewers. Any product that may be evaluated in this article, or claim that may be made by its manufacturer, is not guaranteed or endorsed by the publisher.
Acknowledgments
We thank Fei Peng from Beijing Tiantan Hospital, Yuelong Yang, Xiaoyu Wei, Xiaobing Zhou from Guangdong Provincial People's Hospital for giving instructions and sharing experience to the manuscript. We thank all participants who were recruited in this work.
References
1. Virmani R, Kolodgie FD, Burke AP, Finn AV, Gold HK, Tulenko TN, et al. Atherosclerotic plaque progression and vulnerability to rupture: angiogenesis as a source of intraplaque hemorrhage. Arterioscler Thromb Vasc Biol. (2005) 25:2054–61. doi: 10.1161/01.ATV.0000178991.71605.18
2. Virmani R, Kolodgie FD, Burke AP, Farb A, Schwartz SM. Lessons from sudden coronary death: a comprehensive morphological classification scheme for atherosclerotic lesions. Arterioscler Thromb Vasc Biol. (2000) 20:1262–75. doi: 10.1161/01.atv.20.5.1262
3. Virani SS, Alonso A, Aparicio HJ, Benjamin EJ, Bittencourt MS, Callaway CW, et al. Heart disease and stroke statistics-2021 update: a report from the American Heart Association. Circulation. (2021) 143:e254–743. doi: 10.1161/CIR.0000000000000950
4. Fishbein MC, Siegel RJ. How big are coronary atherosclerotic plaques that rupture? Circulation. (1996) 94:2662–6. doi: 10.1161/01.cir.94.10.2662
5. Stone GW, Maehara A, Lansky AJ, De Bruyne B, Cristea E, Mintz GS, et al. PROSPECT Investigators. A prospective natural-history study of coronary atherosclerosis. N Engl J Med. (2011) 364:226–35. doi: 10.1056/NEJMoa1002358
6. Koskinas KC, Ughi GJ, Windecker S, Tearney GJ, Raber L. Intracoronary imaging of coronary atherosclerosis: validation for diagnosis, prognosis and treatment. Eur Heart J. (2016) 37:524–35a–c. doi: 10.1093/eurheartj/ehv642
7. Maurovich-Horvat P, Ferencik M, Voros S, Merkely B, Hoffmann U. Comprehensive plaque assessment by coronary CT angiography. Nat Rev Cardiol. (2014) 11:390–402. doi: 10.1038/nrcardio.2014.60
8. Motoyama S, Ito H, Sarai M, Kondo T, Kawai H, Nagahara Y, et al. Plaque characterization by coronary computed tomography angiography and the likelihood of acute coronary events in mid-term follow-up. J Am Coll Cardiol. (2015) 66:337–46. doi: 10.1016/j.jacc.2015.05.069
9. Budoff MJ, Dowe D, Jollis JG, Gitter M, Sutherland J, Halamert E, et al. Diagnostic performance of 64-multidetector row coronary computed tomographic angiography for evaluation of coronary artery stenosis in individuals without known coronary artery disease: results from the prospective multicenter ACCURACY (Assessment by Coronary Computed Tomographic Angiography of Individuals Undergoing Invasive Coronary Angiography) trial. J Am Coll Cardiol. (2008) 52:1724–32. doi: 10.1016/j.jacc.2008.07.031
10. Puchner SB, Liu T, Mayrhofer T, Truong QA, Lee H, Fleg JL, et al. High-risk plaque detected on coronary CT angiography predicts acute coronary syndromes independent of significant stenosis in acute chest pain: results from the ROMICAT-II trial. J Am Coll Cardiol. (2014) 64:684–92. doi: 10.1016/j.jacc.2014.05.039
11. Hoffmann U, Moselewski F, Nieman K, Jang IK, Ferencik M, Rahman AM, et al. Noninvasive assessment of plaque morphology and composition in culprit and stable lesions in acute coronary syndrome and stable lesions in stable angina by multidetector computed tomography. J Am Coll Cardiol. (2006) 47:1655–62. doi: 10.1016/j.jacc.2006.01.041
12. Taylor CA, Fonte TA, Min JK. Computational fluid dynamics applied to cardiac computed tomography for noninvasive quantification of fractional flow reserve: scientific basis. J Am Coll Cardiol. (2013) 61:2233–41. doi: 10.1016/j.jacc.2012.11.083
13. Kwak BR, Back M, Bochaton-Piallat ML, Caligiuri G, Daemen MJ, Davies PF, et al. Biomechanical factors in atherosclerosis: mechanisms and clinical implications. Eur Heart J. (2014) 35:3013–20, 3020a–d. doi: 10.1093/eurheartj/ehu353
14. Virmani R, Burke AP, Farb A, Kolodgie FD. Pathology of the vulnerable plaque. J Am Coll Cardiol. (2006) 47:C13–8. doi: 10.1016/j.jacc.2005.10.065
15. Otsuka F, Yasuda S, Noguchi T, Ishibashi-Ueda H. Pathology of coronary atherosclerosis and thrombosis. Cardiovasc Diagn Ther. (2016) 6:396–408. doi: 10.21037/cdt.2016.06.01
16. Yahagi K, Kolodgie FD, Otsuka F, Finn AV, Davis HR, Joner M, et al. Pathophysiology of native coronary, vein graft, and in-stent atherosclerosis. Nat Rev Cardiol. (2016) 13:79–98. doi: 10.1038/nrcardio.2015.164
17. Ahmadi A, Leipsic J, Blankstein R, Taylor C, Hecht H, Stone GW, et al. Do plaques rapidly progress prior to myocardial infarction? The interplay between plaque vulnerability and progression. Circ Res. (2015) 117:99–104. doi: 10.1161/CIRCRESAHA.117.305637
18. Ahmadi A, Argulian E, Leipsic J, Newby DE, Narula J. From subclinical atherosclerosis to plaque progression and acute coronary events: jacc state-of-the-art review. J Am Coll Cardiol. (2019) 74:1608–17. doi: 10.1016/j.jacc.2019.08.012
19. Kolodgie FD, Gold HK, Burke AP, Fowler DR, Kruth HS, Weber DK, et al. Intraplaque hemorrhage and progression of coronary atheroma. N Engl J Med. (2003) 349:2316–25. doi: 10.1056/NEJMoa035655
20. Kolodgie FD, Burke AP, Farb A, Gold HK, Yuan J, Narula J, et al. The thin-cap fibroatheroma: a type of vulnerable plaque: the major precursor lesion to acute coronary syndromes. Curr Opin Cardiol. (2001) 16:285–92. doi: 10.1097/00001573-200109000-00006
21. Narula J, Finn AV, Demaria AN. Picking plaques that pop. J Am Coll Cardiol. (2005) 45:1970–3. doi: 10.1016/j.jacc.2005.03.034
22. Motoyama S, Kondo T, Sarai M, Sugiura A, Harigaya H, Sato T, et al. Multislice computed tomographic characteristics of coronary lesions in acute coronary syndromes. J Am Coll Cardiol. (2007) 50:319–26. doi: 10.1016/j.jacc.2007.03.044
23. Williams MC, Moss AJ, Dweck M, Adamson PD, Alam S, Hunter A, et al. Coronary artery plaque characteristics associated with adverse outcomes in the SCOT-HEART study. J Am Coll Cardiol. (2019) 73:291–301. doi: 10.1016/j.jacc.2018.10.066
24. Joshi NV, Vesey AT, Williams MC, Shah AS, Calvert PA, Craighead FH, et al. 18F-fluoride positron emission tomography for identification of ruptured and high-risk coronary atherosclerotic plaques: a prospective clinical trial. Lancet. (2014) 383:705–13. doi: 10.1016/S0140-6736(13)61754-7
25. Arbab-Zadeh A, Fuster V. From detecting the vulnerable plaque to managing the vulnerable patient: JACC state-of-the-art review. J Am Coll Cardiol. (2019) 74:1582–93. doi: 10.1016/j.jacc.2019.07.062
26. Hulten E, Pickett C, Bittencourt MS, Villines TC, Petrillo S, Di Carli MF, et al. Outcomes after coronary computed tomography angiography in the emergency department: a systematic review and meta-analysis of randomized, controlled trials. J Am Coll Cardiol. (2013) 61:880–92. doi: 10.1016/j.jacc.2012.11.061
27. Goldstein JA, Chinnaiyan KM, Abidov A, Achenbach S, Berman DS, Hayes SW, et al. The CT-STAT (Coronary Computed Tomographic Angiography for Systematic Triage of Acute Chest Pain Patients to Treatment) trial. J Am Coll Cardiol. (2011) 58:1414–22. doi: 10.1016/j.jacc.2011.03.068
28. Zaman T, Agarwal S, Anabtawi AG, Patel NS, Ellis SG, Tuzcu EM, et al. Angiographic lesion severity and subsequent myocardial infarction. Am J Cardiol. (2012) 110:167–72. doi: 10.1016/j.amjcard.2012.03.008
29. Ojio S, Takatsu H, Tanaka T, Ueno K, Yokoya K, Matsubara T, et al. Considerable time from the onset of plaque rupture and/or thrombi until the onset of acute myocardial infarction in humans: coronary angiographic findings within 1 week before the onset of infarction. Circulation. (2000) 102:2063–9. doi: 10.1161/01.cir.102.17.2063
30. Yokoya K, Takatsu H, Suzuki T, Hosokawa H, Ojio S, Matsubara T, et al. Process of progression of coronary artery lesions from mild or moderate stenosis to moderate or severe stenosis: a study based on four serial coronary arteriograms per year. Circulation. (1999) 100:903–9. doi: 10.1161/01.cir.100.9.903
31. Fracassi F, Crea F, Sugiyama T, Yamamoto E, Uemura S, Vergallo R, et al. Healed culprit plaques in patients with acute coronary syndromes. J Am Coll Cardiol. (2019) 73:2253–63. doi: 10.1016/j.jacc.2018.10.093
32. Kolossvary M, Szilveszter B, Merkely B, Maurovich-Horvat P. Plaque imaging with CT-a comprehensive review on coronary CT angiography based risk assessment. Cardiovasc Diagn Ther. (2017) 7:489–506. doi: 10.21037/cdt.2016.11.06
33. Conte E, Annoni A, Pontone G, Mushtaq S, Guglielmo M, Baggiano A, et al. Evaluation of coronary plaque characteristics with coronary computed tomography angiography in patients with non-obstructive coronary artery disease: a long-term follow-up study. Eur Heart J Cardiovasc Imaging. (2017) 18:1170–8. doi: 10.1093/ehjci/jew200
34. Otsuka K, Fukuda S, Tanaka A, Nakanishi K, Taguchi H, Yoshikawa J, et al. Napkin-ring sign on coronary CT angiography for the prediction of acute coronary syndrome. JACC Cardiovasc Imaging. (2013) 6:448–57. doi: 10.1016/j.jcmg.2012.09.016
35. Kroner ES, van Velzen JE, Boogers MJ, Siebelink HM, Schalij MJ, Kroft LJ, et al. Positive remodeling on coronary computed tomography as a marker for plaque vulnerability on virtual histology intravascular ultrasound. Am J Cardiol. (2011) 107:1725–9. doi: 10.1016/j.amjcard.2011.02.337
36. Glagov S, Weisenberg E, Zarins CK, Stankunavicius R, Kolettis GJ. Compensatory enlargement of human atherosclerotic coronary arteries. N Engl J Med. (1987) 316:1371–5. doi: 10.1056/NEJM198705283162204
37. Nakamura M, Nishikawa H, Mukai S, Setsuda M, Nakajima K, Tamada H, et al. Impact of coronary artery remodeling on clinical presentation of coronary artery disease: an intravascular ultrasound study. J Am Coll Cardiol. (2001) 37:63–9. doi: 10.1016/s0735-1097(00)01097-4
38. Varnava AM, Mills PG, Davies MJ. Relationship between coronary artery remodeling and plaque vulnerability. Circulation. (2002) 105:939–43. doi: 10.1161/hc0802.104327
39. Oikonomou EK, West HW, Antoniades C. Cardiac computed tomography: assessment of coronary inflammation and other plaque features. Arterioscler Thromb Vasc Biol. (2019) 39:2207–19. doi: 10.1161/ATVBAHA.119.312899
40. Achenbach S, Ropers D, Hoffmann U, MacNeill B, Baum U, Pohle K, et al. Assessment of coronary remodeling in stenotic and nonstenotic coronary atherosclerotic lesions by multidetector spiral computed tomography. J Am Coll Cardiol. (2004) 43:842–7. doi: 10.1016/j.jacc.2003.09.053
41. Chang HJ, Lin FY, Lee SE, Andreini D, Bax J, Cademartiri F, et al. Coronary atherosclerotic precursors of acute coronary syndromes. J Am Coll Cardiol. (2018) 71:2511–22. doi: 10.1016/j.jacc.2018.02.079
42. Ferencik M, Schlett CL, Ghoshhajra BB, Kriegel MF, Joshi SB, Maurovich-Horvat P, et al. A computed tomography-based coronary lesion score to predict acute coronary syndrome among patients with acute chest pain and significant coronary stenosis on coronary computed tomographic angiogram. Am J Cardiol. (2012) 110:183–9. doi: 10.1016/j.amjcard.2012.02.066
43. Kim SY, Kim KS, Seung MJ, Chung JW, Kim JH, Mun SH, et al. The culprit lesion score on multi-detector computed tomography can detect vulnerable coronary artery plaque. Int J Cardiovasc Imaging. (2010) 26:245–52. doi: 10.1007/s10554-010-9712-2
44. Boogers MJ, Broersen A, van Velzen JE, de Graaf FR, El-Naggar HM, Kitslaar PH, et al. Automated quantification of coronary plaque with computed tomography: comparison with intravascular ultrasound using a dedicated registration algorithm for fusion-based quantification. Eur Heart J. (2012) 33:1007–16. doi: 10.1093/eurheartj/ehr465
45. Williams MC, Kwiecinski J, Doris M, McElhinney P, D'Souza MS, Cadet S, et al. Low-attenuation noncalcified plaque on coronary computed tomography angiography predicts myocardial infarction: results from the multicenter SCOT-HEART trial (Scottish Computed Tomography of the HEART). Circulation. (2020) 141:1452–62. doi: 10.1161/CIRCULATIONAHA.119.044720
46. Mori H, Torii S, Kutyna M, Sakamoto A, Finn AV, Virmani R. Coronary artery calcification and its progression: what does it really mean? JACC Cardiovasc Imaging. (2018) 11:127–42. doi: 10.1016/j.jcmg.2017.10.012
47. van Velzen JE, de Graaf FR, de Graaf MA, Schuijf JD, Kroft LJ, de Roos A, et al. Comprehensive assessment of spotty calcifications on computed tomography angiography: comparison to plaque characteristics on intravascular ultrasound with radiofrequency backscatter analysis. J Nucl Cardiol. (2011) 18:893–903. doi: 10.1007/s12350-011-9428-2
48. Vancheri F, Longo G, Vancheri S, Danial JSH, Henein MY. Coronary artery microcalcification: imaging and clinical implications. Diagnostics. (2019) 9:125. doi: 10.3390/diagnostics9040125
49. Motoyama S, Sarai M, Harigaya H, Anno H, Inoue K, Hara T, et al. Computed tomographic angiography characteristics of atherosclerotic plaques subsequently resulting in acute coronary syndrome. J Am Coll Cardiol. (2009) 54:49–57. doi: 10.1016/j.jacc.2009.02.068
50. Benedek T, Gyongyosi M, Benedek I. Multislice computed tomographic coronary angiography for quantitative assessment of culprit lesions in acute coronary syndromes. Can J Cardiol. (2013) 29:364–71. doi: 10.1016/j.cjca.2012.11.004
51. Saremi F, Achenbach S. Coronary plaque characterization using CT. Am J Roentgenol. (2015) 204:W249–60. doi: 10.2214/AJR.14.13760
52. Burke AP, Weber DK, Kolodgie FD, Farb A, Taylor AJ, Virmani R. Pathophysiology of calcium deposition in coronary arteries. Herz. (2001) 26:239–44. doi: 10.1007/pl00002026
53. Seifarth H, Schlett CL, Nakano M, Otsuka F, Karolyi M, Liew G, et al. Histopathological correlates of the napkin-ring sign plaque in coronary CT angiography. Atherosclerosis. (2012) 224:90–6. doi: 10.1016/j.atherosclerosis.2012.06.021
54. Kitagawa T, Yamamoto H, Horiguchi J, Ohhashi N, Tadehara F, Shokawa T, et al. Characterization of noncalcified coronary plaques and identification of culprit lesions in patients with acute coronary syndrome by 64-slice computed tomography. JACC Cardiovasc Imaging. (2009) 2:153–60. doi: 10.1016/j.jcmg.2008.09.015
55. Pflederer T, Marwan M, Schepis T, Ropers D, Seltmann M, Muschiol G, et al. Characterization of culprit lesions in acute coronary syndromes using coronary dual-source CT angiography. Atherosclerosis. (2010) 211:437–44. doi: 10.1016/j.atherosclerosis.2010.02.001
56. Ozaki Y, Okumura M, Ismail TF, Motoyama S, Naruse H, Hattori K, et al. Coronary CT angiographic characteristics of culprit lesions in acute coronary syndromes not related to plaque rupture as defined by optical coherence tomography and angioscopy. Eur Heart J. (2011) 32:2814–23. doi: 10.1093/eurheartj/ehr189
57. Otsuka F, Finn AV, Virmani R. Do vulnerable and ruptured plaques hide in heavily calcified arteries? Atherosclerosis. (2013) 229:34–7. doi: 10.1016/j.atherosclerosis.2012.12.032
58. Ong DS, Lee JS, Soeda T, Higuma T, Minami Y, Wang Z, et al. Coronary calcification and plaque vulnerability: an optical coherence tomographic study. Circ Cardiovasc Imaging. (2016) 9:e003929. doi: 10.1161/CIRCIMAGING.115.003929
59. Feuchtner G, Kerber J, Burghard P, Dichtl W, Friedrich G, Bonaros N, et al. The high-risk criteria low-attenuation plaque <60 HU and the napkin-ring sign are the most powerful predictors of MACE: a long-term follow-up study. Eur Heart J Cardiovasc Imaging. (2017) 18:772–779. doi: 10.1093/ehjci/jew167
60. Achenbach S. Imaging the vulnerable plaque on coronary CTA. JACC Cardiovasc Imaging. (2020) 13:1418–21. doi: 10.1016/j.jcmg.2019.11.006
61. Arbab-Zadeh A, Fuster V. The myth of the “vulnerable plaque”: transitioning from a focus on individual lesions to atherosclerotic disease burden for coronary artery disease risk assessment. J Am Coll Cardiol. (2015) 65:846–55. doi: 10.1016/j.jacc.2014.11.041
62. Schuurman AS, Vroegindewey MM, Kardys I, Oemrawsingh RM, Garcia-Garcia HM, van Geuns RJ, et al. Prognostic value of intravascular ultrasound in patients with coronary artery disease. J Am Coll Cardiol. (2018) 72:2003–11. doi: 10.1016/j.jacc.2018.08.2140
63. Lee SE, Sung JM, Rizvi A, Lin FY, Kumar A, Hadamitzky M, et al. Quantification of coronary atherosclerosis in the assessment of coronary artery disease. Circ Cardiovasc Imaging. (2018) 11:e007562. doi: 10.1161/CIRCIMAGING.117.007562
64. Weissberg PL, Bennett MR. Atherosclerosis–an inflammatory disease. N Engl J Med. (1999) 340:1928–9. doi: 10.1056/nejm199906173402418
65. Choi G, Lee JM, Kim HJ, Park JB, Sankaran S, Otake H, et al. Coronary artery axial plaque stress and its relationship with lesion geometry: application of computational fluid dynamics to coronary CT angiography. JACC Cardiovasc Imaging. (2015) 8:1156–66. doi: 10.1016/j.jcmg.2015.04.024
66. De Bruyne B, Fearon WF, Pijls NH, Barbato E, Tonino P, Piroth Z, et al. Fractional flow reserve-guided PCI for stable coronary artery disease. N Engl J Med. (2014) 371:1208–17. doi: 10.1056/NEJMoa1408758
67. Fairbairn TA, Nieman K, Akasaka T, Norgaard BL, Berman DS, Raff G, et al. Real-world clinical utility and impact on clinical decision-making of coronary computed tomography angiography-derived fractional flow reserve: lessons from the ADVANCE Registry. Eur Heart J. (2018) 39:3701–11. doi: 10.1093/eurheartj/ehy530
68. Leipsic J, Yang TH, Thompson A, Koo BK, Mancini GB, Taylor C, et al. CT angiography (CTA) and diagnostic performance of noninvasive fractional flow reserve: results from the Determination of Fractional Flow Reserve by Anatomic CTA (DeFACTO) study. AJR Am J Roentgenol. (2014) 202:989–94. doi: 10.2214/AJR.13.11441
69. Norgaard BL, Leipsic J, Gaur S, Seneviratne S, Ko BS, Ito H, et al. Diagnostic performance of noninvasive fractional flow reserve derived from coronary computed tomography angiography in suspected coronary artery disease: the NXT trial (Analysis of Coronary Blood Flow Using CT Angiography: Next Steps). J Am Coll Cardiol. (2014) 63:1145–55. doi: 10.1016/j.jacc.2013.11.043
70. Fearon WF, Bornschein B, Tonino PA, Gothe RM, Bruyne BD, Pijls NH, et al. Economic evaluation of fractional flow reserve-guided percutaneous coronary intervention in patients with multivessel disease. Circulation. (2010) 122:2545–50. doi: 10.1161/CIRCULATIONAHA.109.925396
71. Koo BK, Erglis A, Doh JH, Daniels DV, Jegere S, Kim HS, et al. Diagnosis of ischemia-causing coronary stenoses by noninvasive fractional flow reserve computed from coronary computed tomographic angiograms. Results from the prospective multicenter DISCOVER-FLOW (Diagnosis of Ischemia-Causing Stenoses Obtained Via Noninvasive Fractional Flow Reserve) study. J Am Coll Cardiol. (2011) 58:1989–97. doi: 10.1016/j.jacc.2011.06.066
72. Min JK, Leipsic J, Pencina MJ, Berman DS, Koo BK, van Mieghem C, et al. Diagnostic accuracy of fractional flow reserve from anatomic CT angiography. JAMA. (2012) 308:1237–45. doi: 10.1001/2012.jama.11274
73. Lee JM, Choi G, Hwang D, Park J, Kim HJ, Doh JH, et al. Impact of Longitudinal Lesion Geometry on Location of Plaque Rupture and Clinical Presentations. JACC Cardiovasc Imaging. (2017) 10:677–88. doi: 10.1016/j.jcmg.2016.04.012
74. Samady H, Eshtehardi P, McDaniel MC, Suo J, Dhawan SS, Maynard C, et al. Coronary artery wall shear stress is associated with progression and transformation of atherosclerotic plaque and arterial remodeling in patients with coronary artery disease. Circulation. (2011) 124:779–88. doi: 10.1161/CIRCULATIONAHA.111.021824
75. Fukumoto Y, Hiro T, Fujii T, Hashimoto G, Fujimura T, Yamada J, et al. Localized elevation of shear stress is related to coronary plaque rupture: a 3-dimensional intravascular ultrasound study with in-vivo color mapping of shear stress distribution. J Am Coll Cardiol. (2008) 51:645–50. doi: 10.1016/j.jacc.2007.10.030
76. Lee JM, Choi G, Koo BK, Hwang D, Park J, Zhang J, et al. Identification of high-risk plaques destined to cause acute coronary syndrome using coronary computed tomographic angiography and computational fluid dynamics. JACC Cardiovasc Imaging. (2019) 12:1032–43. doi: 10.1016/j.jcmg.2018.01.023
77. Ferencik M, Lu MT, Mayrhofer T, Puchner SB, Liu T, Maurovich-Horvat P, et al. Non-invasive fractional flow reserve derived from coronary computed tomography angiography in patients with acute chest pain: subgroup analysis of the ROMICAT II trial. J Cardiovasc Comput Tomogr. (2019) 13:196–202. doi: 10.1016/j.jcct.2019.05.009
78. Park J, Lee JM, Koo BK, Choi G, Hwang D, Rhee TM, et al. Relevance of anatomical, plaque, and hemodynamic characteristics of non-obstructive coronary lesions in the prediction of risk for acute coronary syndrome. Eur Radiol. (2019) 29:6119–28. doi: 10.1007/s00330-019-06221-9
79. Park JB, Choi G, Chun EJ, Kim HJ, Park J, Jung JH, et al. Computational fluid dynamic measures of wall shear stress are related to coronary lesion characteristics. Heart. (2016) 102:1655–61. doi: 10.1136/heartjnl-2016-309299
80. Han D, Starikov A. O Hartaigh B, Gransar H, Kolli KK, Lee JH, et al. Relationship between endothelial wall shear stress and high-risk atherosclerotic plaque characteristics for identification of coronary lesions that cause ischemia: a direct comparison with fractional flow. Reserve J Am Heart Assoc. (2016) 5:e004186. doi: 10.1161/JAHA.116.004186
81. Bourantas CV, Papadopoulou SL, Serruys PW, Sakellarios A, Kitslaar PH, Bizopoulos P, et al. Noninvasive prediction of atherosclerotic progression: the PROSPECT-MSCT study. JACC Cardiovasc Imaging. (2016) 9:1009–11. doi: 10.1016/j.jcmg.2015.07.005
82. Verhagen SN, Visseren FL. Perivascular adipose tissue as a cause of atherosclerosis. Atherosclerosis. (2011) 214:3–10. doi: 10.1016/j.atherosclerosis.2010.05.034
83. Tanaka K, Sata M. Roles of perivascular adipose tissue in the pathogenesis of atherosclerosis. Front Physiol. (2018) 9:3. doi: 10.3389/fphys.2018.00003
84. Antonopoulos AS, Sanna F, Sabharwal N, Thomas S, Oikonomou EK, Herdman L, et al. Detecting human coronary inflammation by imaging perivascular fat. Sci Transl Med. (2017) 9:eaal2658. doi: 10.1126/scitranslmed.aal2658
85. Margaritis M, Antonopoulos AS, Digby J, Lee R, Reilly S, Coutinho P, et al. Interactions between vascular wall and perivascular adipose tissue reveal novel roles for adiponectin in the regulation of endothelial nitric oxide synthase function in human vessels. Circulation. (2013) 127:2209–21. doi: 10.1161/CIRCULATIONAHA.112.001133
86. Akoumianakis I, Antoniades C. The interplay between adipose tissue and the cardiovascular system: is fat always bad? Cardiovasc Res. (2017) 113:999–1008. doi: 10.1093/cvr/cvx111
87. Hedgire Sa, Baliyan V, Zucker EJ, Bittner DO, Staziaki PV, Takx RAP, et al. Perivascular epicardial fat stranding at coronary CT angiography: a marker of acute plaque rupture and spontaneous coronary artery dissection. radiology. (2018) 287:808–15. doi: 10.1148/radiol.2017171568
88. Goeller M, Achenbach S, Cadet S, Kwan AC, Commandeur F, Slomka PJ, et al. Pericoronary adipose tissue computed tomography attenuation and high-risk plaque characteristics in acute coronary syndrome compared with stable coronary artery disease. JAMA Cardiol. (2018) 3:858–63. doi: 10.1001/jamacardio.2018.1997
89. Goeller M, Tamarappoo BK, Kwan AC, Cadet S, Commandeur F, Razipour A, et al. Relationship between changes in pericoronary adipose tissue attenuation and coronary plaque burden quantified from coronary computed tomography angiography. Eur Heart J Cardiovasc Imaging. (2019) 20:636–43. doi: 10.1093/ehjci/jez013
90. Crewe C, An YA, Scherer PE. The ominous triad of adipose tissue dysfunction: inflammation, fibrosis, and impaired angiogenesis. J Clin Invest. (2017) 127:74–82. doi: 10.1172/JCI88883
91. Oikonomou EK, Williams MC, Kotanidis CP, Desai MY, Marwan M, Antonopoulos AS, et al. A novel machine learning-derived radiotranscriptomic signature of perivascular fat improves cardiac risk prediction using coronary CT angiography. Eur Heart J. (2019) 40:3529–43. doi: 10.1093/eurheartj/ehz592
92. Lin FY, Villines TC, Narula J, Shaw LJ. What is the clinical role of non-invasive atherosclerosis imaging? J Cardiovasc Comput Tomogr. (2019) 13:261–6. doi: 10.1016/j.jcct.2019.05.010
93. Adamson PD, Newby DE. Non-invasive imaging of the coronary arteries. Eur Heart J. (2019) 40:2444–54. doi: 10.1093/eurheartj/ehy670
94. Erlinge D, Maehara A, Ben-Yehuda O, Botker HE, Maeng M, Kjoller-Hansen L, et al. Identification of vulnerable plaques and patients by intracoronary near-infrared spectroscopy and ultrasound (PROSPECT II): a prospective natural history study. Lancet. (2021) 397:985–95. doi: 10.1016/S0140-6736(21)00249-X
95. Daghem M, Bing R, Fayad ZA, Dweck MR. Noninvasive imaging to assess atherosclerotic plaque composition and disease activity: coronary and carotid applications. JACC Cardiovasc Imaging. (2020) 13:1055–68. doi: 10.1016/j.jcmg.2019.03.033
96. Li J, Montarello NJ, Hoogendoorn A, Verjans JW, Bursill CA, Peter K, et al. Multimodality intravascular imaging of high-risk coronary plaque. JACC Cardiovasc Imaging. (2021) 12:28. doi: 10.1016/j.jcmg.2021.03.028
97. Otsuka K, Villiger M, Karanasos A, van Zandvoort LJC, Doradla P, Ren J, et al. Intravascular polarimetry in patients with coronary artery disease. JACC Cardiovasc Imaging. (2020) 13:790–801. doi: 10.1016/j.jcmg.2019.06.015
98. Otsuka K, Villiger M, Nadkarni SK, Bouma BE. Intravascular polarimetry: clinical translation and future applications of catheter-based polarization sensitive optical frequency domain imaging. Front Cardiovasc Med. (2020) 7:146. doi: 10.3389/fcvm.2020.00146
99. Mark DB, Federspiel JJ, Cowper PA, Anstrom KJ, Hoffmann U, Patel MR, et al. Economic outcomes with anatomical versus functional diagnostic testing for coronary artery disease. Ann Intern Med. (2016) 165:94–102. doi: 10.7326/M15-2639
100. Levin DC, Parker L, Halpern EJ, Rao VM. Coronary CT angiography: reversal of earlier utilization trends. J Am Coll Radiol. (2019) 16:147–55. doi: 10.1016/j.jacr.2018.07.022
101. Chang HJ, Lin FY, Gebow D, An HY, Andreini D, Bathina R, et al. Selective referral using CCTA versus direct referral for individuals referred to invasive coronary angiography for suspected CAD: a randomized, controlled, open-label trial. JACC Cardiovasc Imaging. (2019) 12:1303–12. doi: 10.1016/j.jcmg.2018.09.018
102. Tarkin JM, Dweck MR, Evans NR, Takx RA, Brown AJ, Tawakol A, et al. Imaging atherosclerosis. Circ Res. (2016) 118:750–69. doi: 10.1161/CIRCRESAHA.115.306247
103. Goehler A, Mayrhofer T, Pursnani A, Ferencik M, Lumish HS, Barth C, et al. Long-term health outcomes and cost-effectiveness of coronary CT angiography in patients with suspicion for acute coronary syndrome. J Cardiovasc Comput Tomogr. (2020) 14:44–54. doi: 10.1016/j.jcct.2019.06.008
104. Cury RC, Abbara S, Achenbach S, Agatston A, Berman DS, Budoff MJ, et al. Coronary Artery Disease - Reporting and Data System (CAD-RADS): an expert consensus document of SCCT, ACR and NASCI: endorsed by the ACC. JACC Cardiovasc Imaging. (2016) 9:1099–113. doi: 10.1016/j.jcmg.2016.05.005
105. Costopoulos C, Maehara A, Huang Y, Brown AJ, Gillard JH, Teng Z, et al. Heterogeneity of plaque structural stress is increased in plaques leading to MACE: insights from the PROSPECT study. JACC Cardiovasc Imaging. (2020) 13:1206–18. doi: 10.1016/j.jcmg.2019.05.024
106. Doradla P, Otsuka K, Nadkarni A, Villiger M, Karanasos A, Zandvoort LJCV, et al. Biomechanical stress profiling of coronary atherosclerosis: identifying a multifactorial metric to evaluate plaque rupture risk. JACC Cardiovasc Imaging. (2020) 13:804–16. doi: 10.1016/j.jcmg.2019.01.033
107. Lee SE, Chang HJ, Sung JM, Park HB, Heo R, Rizvi A, et al. Effects of statins on coronary atherosclerotic plaques: the PARADIGM study. JACC Cardiovasc Imaging. (2018) 11:1475–84. doi: 10.1016/j.jcmg.2018.04.015
108. Ghekiere O, Salgado R, Buls N, Leiner T, Mancini I, Vanhoenacker P, et al. Image quality in coronary CT angiography: challenges and technical solutions. Br J Radiol. (2017) 90:20160567. doi: 10.1259/bjr.20160567
109. Cury RC, Abbara S, Achenbach S, Agatston A, Berman DS, Budoff MJ, et al. CAD-RADS(TM) coronary artery disease - reporting and data system. An expert consensus document of the Society of Cardiovascular Computed Tomography (SCCT), the American College of Radiology (ACR) and the North American Society for Cardiovascular Imaging (NASCI) endorsed by the American College of Cardiology. J Cardiovasc Comput Tomogr. (2016) 10:269–81. doi: 10.1016/j.jcct.2016.04.005
110. Smulders MW, Kietselaer BLJH, Wildberger JE, Dagnelie PC, Brunner-La Rocca HP, Mingels AMA, et al. Initial imaging-guided strategy versus routine care in patients with non-ST-segment elevation myocardial infarction. J Am Coll Cardiol. (2019) 74:2466–77. doi: 10.1016/j.jacc.2019.09.027
111. Stocker TJ, Deseive S, Leipsic J, Hadamitzky M, Chen MY, Rubinshtein R, et al. Reduction in radiation exposure in cardiovascular computed tomography imaging: results from the PROspective multicenter registry on radiaTion dose Estimates of cardiac CT angIOgraphy iN daily practice in 2017 (PROTECTION VI). Eur Heart J. (2018) 39:3715–23. doi: 10.1093/eurheartj/ehy546
112. Pessis E, Campagna R, Sverzut JM, Bach F, Rodallec M, Guerini H, et al. Virtual monochromatic spectral imaging with fast kilovoltage switching: reduction of metal artifacts at CT. Radiographics. (2013) 33:573–83. doi: 10.1148/rg.332125124
113. Kalisz K, Halliburton S, Abbara S, Leipsic JA, Albrecht MH, Schoepf UJ, et al. Update on cardiovascular applications of multienergy CT. Radiographics. (2017) 37:1955–74. doi: 10.1148/rg.2017170100
114. Hill KD, Frush DP, Han BK, Abbott BG, Armstrong AK, DeKemp RA, et al. Radiation safety in children with congenital and acquired heart disease: a scientific position statement on multimodality dose optimization from the image gently alliance. JACC Cardiovasc Imaging. (2017) 10:797–818. doi: 10.1016/j.jcmg.2017.04.003
115. Cauldwell C. Anesthesia risks associated with pediatric imaging. Pediatr Radiol. (2011) 41:949–50. doi: 10.1007/s00247-011-2160-x
116. Greenberg SB. Rebalancing the risks of computed tomography and magnetic resonance imaging. Pediatr Radiol. (2011) 41:951–2. doi: 10.1007/s00247-011-2159-3
117. Choi AD, Thomas DM, Lee J, Abbara S, Cury RC, Leipsic JA, et al. 2020 SCCT guideline for training cardiology and radiology trainees as independent practitioners (level II) and advanced practitioners (Level III) in cardiovascular computed tomography: a statement from the Society of Cardiovascular Computed Tomography. JACC Cardiovasc Imaging. (2021) 14:272–87. doi: 10.1016/j.jcmg.2020.09.004
118. Weir-McCall JR, Branch K, Ferencik M, Blankstein R, Choi AD, Ghoshhajra BB, et al. Highlights of the 15th annual scientific meeting of the Society of Cardiovascular Computed Tomography. J Cardiovasc Comput Tomogr. (2020) 14:466–70. doi: 10.1016/j.jcct.2020.09.008
119. Abdelrahman KM, Chen MY, Dey AK, Virmani R, Finn AV, Khamis RY, et al. Coronary computed tomography angiography from clinical uses to emerging technologies: JACC state-of-the-art review. J Am Coll Cardiol. (2020) 76:1226–43. doi: 10.1016/j.jacc.2020.06.076
120. Kumar V, Weerakoon S, Dey AK, Earls JP, Katz RJ, Reiner JS, et al. The evolving role of coronary CT angiography in Acute Coronary Syndromes. J Cardiovasc Comput Tomogr. (2021) 15:384–93. doi: 10.1016/j.jcct.2021.02.002
Keywords: coronary computed tomography angiography (CCTA), high-risk plaque, acute coronary syndrome (ACS), computational fluid dynamics–CFD, pericoronary adipose tissue attenuation, coronary artery
Citation: Lu G, Ye W, Ou J, Li X, Tan Z, Li T and Liu H (2021) Coronary Computed Tomography Angiography Assessment of High-Risk Plaques in Predicting Acute Coronary Syndrome. Front. Cardiovasc. Med. 8:743538. doi: 10.3389/fcvm.2021.743538
Received: 18 July 2021; Accepted: 07 September 2021;
Published: 01 October 2021.
Edited by:
Zhao Wang, University of Electronic Science and Technology of China, ChinaReviewed by:
Kenichiro Otsuka, Massachusetts General Hospital, United StatesShady Abohashem, Massachusetts General Hospital and Harvard Medical School, United States
Copyright © 2021 Lu, Ye, Ou, Li, Tan, Li and Liu. This is an open-access article distributed under the terms of the Creative Commons Attribution License (CC BY). The use, distribution or reproduction in other forums is permitted, provided the original author(s) and the copyright owner(s) are credited and that the original publication in this journal is cited, in accordance with accepted academic practice. No use, distribution or reproduction is permitted which does not comply with these terms.
*Correspondence: Hui Liu, bGl1aHVpaml1aml1JiN4MDAwNDA7Z21haWwuY29t