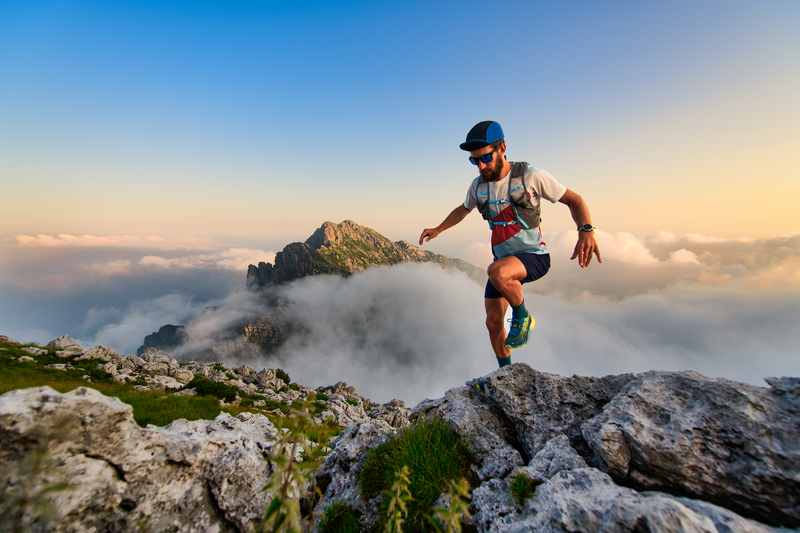
95% of researchers rate our articles as excellent or good
Learn more about the work of our research integrity team to safeguard the quality of each article we publish.
Find out more
REVIEW article
Front. Cardiovasc. Med. , 04 October 2021
Sec. Cardiovascular Biologics and Regenerative Medicine
Volume 8 - 2021 | https://doi.org/10.3389/fcvm.2021.734562
This article is part of the Research Topic Extracellular Vesicles in Cardiovascular Diseases View all 17 articles
Almost all nucleated cells secrete extracellular vesicles (EVs) that are heterogeneous spheroid patterned or round shape particles ranging from 30 to 200 nm in size. Recent preclinical and clinical studies have shown that endothelial progenitor cell-derived EVs (EPC-EVs) have a beneficial therapeutic effect in various diseases, including cardiovascular diseases and kidney, and lung disorders. Moreover, some animal studies have shown that EPC-EVs selectively accumulate at the injury site with a specific mechanism of binding along with angiogenic and restorative effects that are superior to those of their ancestors. This review article highlights current advances in the biogenesis, delivery route, and long-term storage methods of EPC-EVs and their favorable effects such as anti-inflammatory, angiogenic, and tissue protection in various diseases. Finally, we review the possibility of therapeutic application of EPC-EVs in the clinic.
Endothelial progenitor cells (EPCs) have been widely used to treat cardiovascular ischemic diseases since their discovery in 1997 (1). Initial clinical trials, in parallel with preclinical studies, raised hopes of cures for life-threatening ischemic diseases (2). In subsequent studies, EPC biology was further investigated and it was found that after long-term culture of between 15 and 21 days, cobblestone-shaped colonies emerged, called blood endothelial outgrowth cells (3). The phenotypes of these cells are similar to those of the adult endothelial cells and have a greater proliferative rate (3); Yoder‘s group (4) found similar cells from umbilical cord blood cells (4). Clinical studies have demonstrated that the origin of EPC is bone marrow, and considering pathological triggers, these cells migrate to damaged tissues and physically contribute to facilitating vasculature (3, 5–7). However, several groups are concerned about the existence of EPCs based on mouse data (8). In addition, the culture of EPCs in diverse systems, different methodologies, and various “misleading terms” has led to confusion in EPC biology and application. To this end, a recent consensus attempted to standardize EPC nomenclature based on cellular phenotypes and biological functions (9). A consensus statement on EPC nomenclature and culture standardization may facilitate progress toward the use of EPC-derived extracellular vesicle (EPC-EV) therapy. Depending on the sequence of appearance in culture, Hur et al. (10) reported two types of EPCs. The first were termed early EPCs or myeloid angiogenic cells that were positive for CD45, CD14, and CD31 markers, and mainly worked via paracrine mechanisms, such as growth factors and EVs (10, 11). The second cell population, named late-EPC or endothelial colony-forming cells (ECFCs), usually appeared in culture at 2 to 3 weeks after cell culturing, and had similar phenotypes as endothelial cells, and enhanced neovascularization in ischemic tissues (10, 12). Recent studies have demonstrated that ECFCs secrete EVs that are crucial for organ restoration (13–15).
Almost all nucleated cells secrete extracellular vesicles cargo which deliveries nucleic acid and proteins to the recipient cells. The International Society for Extracellular Vesicles consensus recommendation on nomenclature endorses to use “extracellular vesicles” as a generic term for a lipid bilayer particle released from the cell and cannot replicate. Moreover, it has a broader meaning which can cover subtypes like exosomes and microvesicles as well. Depending on the physical size range, EVs divide small (<100 nm), medium (<200 nm), or large (>200 nm), and usually express CD63+, CD81+, Annexin A5, etc., surface markers (16). The small EVs are generated within endosomes as intraluminal vesicles and this complex EVs biogenesis occurs by endosomal sorting complex required for transport (ESCRT) sorting machineries involvement (17). Whereas, medium or large EVs originate by an outward budding at the plasma membrane (Figure 1) (18). There are several methods to isolate secreted EVs such as classical differential centrifugation, density gradient centrifugation, size-exclusion chromatography, ultrafiltration, immunocapture, precipitation, and tangential flow filtration, etc. (Figure 1) (16). Each EV isolation strategy or its working principle along with their advantages and disadvantages was reviewed previously (19).
A seminal study showed that EPCs secrete microvesicles, and the latter activate an angiogenic program in endothelial cells via horizontal transfer of mRNA (20). Subsequently, preclinical studies showed that EPC-EVs have superior therapeutic effects on various ischemic diseases (Figure 2) (21–23). In the last decade, numerous studies on EPC-EVs have shed light on EV biogenesis, uptake, and mechanism of action (24–27). This review highlights recent advances in the biogenesis, biological functions, route of delivery, and long-term storage of EPC-EVs. Finally, we describe potential translation to the clinic and regions of application in the context of various ischemic and inflammatory diseases.
Figure 2. Extracellular vesicles biogenesis and isolation methods. UCF, ultracentrifuge; dUCF, density gradient centrifugation, size-exclusion chromatography.
Recently, it has been reported that therapeutic cell transplantation-related effects for cardiovascular diseases are a result of paracrine mechanisms and not from direct cell contribution to damaged organs (28). Regardless of the target delivery technique, the long-term engraftment of cells is limited; hence, the striking short-term improvement in ischemic organ function after cell transplantation is mainly associated with paracrine trophic factors such as EVs (29). It has been shown that early EPC populations are contaminated with hematopoietic cell subsets, such as monocytes (30), and the latter secrete various nanoparticles. In contrast, late EPCs have specific phenotypes and biological functions similar to endothelial cells, and secrete angiomiRs-shuttled EVs, which are a key genetic material for neovascularization of ischemic tissues (14, 31, 32). Dozens of preclinical studies have demonstrated EPC-EV effects that are superior to those of the ancestor (33). EVs possess numerous advantages over cell-based therapies in the context of regenerative medicine in terms of (1) cargo delivery of various favorable miRs responsible for angiogenesis, fibrosis, and cell proliferation; (2) potential for “off the shelf” availability and respective for repetitive transplantation; (3) cell-free biological products that may be utilized as drug carrier systems in the pharmaceutical industry, and finally (4) generally reduced immunogenicity owing to which allogenic transplantation is an additional benefit. The abovementioned benefits are crucial for treating either acute or chronic diseases. The latter listed major advantages of the EVs are linked to less immunogenic than their parental cells because of the lower abundance of transmembrane proteins such as MHC complexes on their surface (34). Unlike live cells, EVs have a long shelf life and may be transported and stored for long periods (see the section on long-term preservation and storage of EVs). In the representative Venn diagram (Figure 3) (transcriptome data from previous publication PMID: 28631889), we summarize the similarities and differences between ECFC-derived microRNAs (miRs) and ECFC-EV-derived miRs (35). It can be clearly seen that the majority of parent cell-derived miRs (ECFC-miRs) can be found in ECFC-EV-derived miRs, suggesting a similar transcriptome profile along with the mechanism of action (Figure 3). A previous study showed that the therapeutic potential of EPC-EVs is superior in terms of enhancing neovascularization and recovery in a murine hind limb ischemia model (12). The mechanism of activation of the angiogenic program in quiescent endothelial cells is linked by horizontal transfer of genetic materials such as angiomiR, RNA, and proteins (12, 13). Of note, ischemia itself is a trigger for angiogenesis. However, angiogenesis-qualified angiomiRs accelerate not only angiogenesis but also proliferative and anti-apoptotic effects (Figure 4). Collectively, well-packed EPC-EVs have a great advantage in preserving ischemic tissue from injury, and future studies are warranted to define the beneficial effects of EPC-EVs.
Figure 3. Endothelial colony forming cell (ECFC)-derived microRNAs (miRs) vs. ECFC-extracellular vesicle (EV)-derived miRs. As shown in the Venn diagram, the majority of the top upregulated human miRs of both ECFC-derived and ECFC-EVs-derived miRs comprise similar miRs. This provides evidence that EPCs work via paracrine factors in organ regeneration. This miR sequence data was generated from PMID: 28631889.
Figure 4. As shown in the flow chart that highly expressed ECFC-EC-derived miRs biological targets were predicted in silico. The most significantly enriched functional annotation were Angiogenesis, vasculogenesis, and lymphangiogenesis. This miR sequence data was generated from PMID: 28631889. MicroRNA target genes were predicted using IPA with information from TargetScan, miRecords, and TarBase databases.
Cardiovascular diseases are the leading cause of mortality and morbidity in the globe (36). It has been shown that therapeutic neoangiogenesis with EPCs is a promising strategy for treating advanced cardiovascular diseases and preventing major adverse events (37). Similar transcriptome profiles of EPC-derived EVs to the EPCs facilitate therapeutic application EPC-EVs in CVD. Yue et al. (38) demonstrated that EPC-derived exosome treatment enhanced left ventricle cardiac function, reduced cell apoptosis, diminished myocardial scar size, and promoted post-myocardial infarction neovascularization. Previous studies have shown that sonic hedgehog modified progenitor cells (CD34+) actively secrete exosome cargo and carry various reparative molecules to cure the ischemic myocardium (39, 40). EPC-EVs regulate cardioprotection by orchestrating cell angiogenesis, migration and adhesion, cell proliferation, and cell differentiation processes (Figure 5). Target gene expression analysis of EPC-EV-derived miR revealed that heart regeneration and protection enriched functional gene upregulation (Figure 5). Cardioprotective properties of EPC-derived EV is associated with miR-218-5p and miR-363-3p overexpression. The latter facilitated cardiac function via enhanced neoangiogenesis and inhibited myocardial fibrosis (41). Moreover, EPC-EVs treatment promoted mesenchymal-endothelial transition and along with protective effect to myocardial infarcted tissues (42). Recently, Chen et al. (33) showed that using EPC-EVs and encapsulation with a hydrogel could increase biological activity for up to 3 weeks through sustained release. Furthermore, the injected hydrogel system for sustained EPC-EV delivery into the ischemic myocardium augmented hemodynamics via increased vessel density in the peri-infarcted area along with reduction in myocardial scar formation. Interestingly, the regenerative efficacy of hydrogen-encapsulated EPC-EVs is not inferior to that of the parent cells or EPCs (33). Repetitive systemic transplantation of EVs is a simple delivery option. We have recently shown that systemic repetitive transplantation of EVs derived from regeneration-associated cells in a rat model of myocardial IR injury significantly enhanced cardiac functions, such as ejection fraction, and preserved mitral regurgitation. In addition, we could not observe anti-donor immune responses even when EV transplantation was performed in allogeneic settings.
Figure 5. Flow chart depicts highly expressed ECFC-EC-derived miRs biological targets that were predicted in silico. The significantly enriched functional annotation was associated with heart tissue repair. This miR sequence data was generated from PMID: 28631889. MicroRNA target genes were predicted using IPA with information from TargetScan, miRecords, and TarBase databases.
Taken together, EPC-EVs have anti-inflammatory and anti-fibrotic properties and may enhance angiogenesis in the ischemic myocardium.
Systematic reviews have demonstrated that the mortality rate in ALI/ARDS is between 36 and 44% and is usually induced by various etiologies such as sepsis, pneumonia, and severe traumas (43). The high mortality rate in ALI/ARDS facilitates various “cell-free” therapeutic EVs, including EPC-EVs. Emerging data shows that EPC-EV administration markedly reduced lipopolysaccharide-induced lung inflammation compared to that in the control groups, indicating a strong anti-inflammatory effect of EVs. Histological examination of the EPC-EV-administered group showed limited alveolar edema and lung neutrophil infiltration, and reduced cytokine/chemokine levels in the bronchoalveolar lavage fluid (44). Mechanistically, EPC-EV contains abundant miRNA-126, and overexpression of miRNA-126-3p can target phosphoinositide-3-kinase regulatory subunit 2, whereas overexpression of miRNA-126-5p inhibits the inflammatory alarmin high mobility group box 1 (HMGB1) and the permeability factor vascular endothelial growth factor (VEGF) α (44). Wu et al. (45) reported different mechanistic insights into EPC exosome-mediated transfer of miR-126 to endothelial cells such as the selective expression of SPRED1 and the enhancement of RAF/ERK signaling pathways that were primarily responsible for restoring the acute-injured lung. In summary, EPC-EVs have a beneficial effect in improving ALI/ARDS outcomes, and further studies are necessary to define optimal and targeted EV delivery methods to the site of injury.
Sepsis is a systemic inflammation induced mainly by microorganisms, leading to organ dysfunction. Recent studies have highlighted that EPC transplantation has a beneficial effect on animal models of sepsis (46, 47). Mechanistically, various pro-inflammatory cytokines induced by pathogen-associated molecular patterns (PAMPs) and damage-associated molecular patterns (DAMPs) in peripheral blood cause vascular injury and increase permeability (47). Consequently, in response to vascular injury, EPCs mobilize in an SDF1a-dependent manner and directly recruit to the injury site and differentiate into mature endothelial cells (47, 48). Fan et al. (46) demonstrated that EPCs and SDF1a administration synergistically improves survival in septic animals via enhanced miR-126 and miR-125b expression, which is believed to play key roles in the maintenance of endothelial cell function and inflammation. Later, they demonstrated that the protective effect of EPCs on the microvasculature after sepsis occurs via exosome-mediated transfer of miRs such as miR-126-3p and 5p (49). EPC-EVs miR-126-5p and 3p suppressed DAMP-induced HMGB1 and vascular cell adhesion molecule 1 (VCAM1) levels, whereas inhibition of miR-126-5p and 3p through transfection with miR-126-5p and 3p inhibitors disrupted the beneficial effect of EPC exosomes. Thus, EPC-EVs prevent adverse septic complications via miR-126 delivery (49).
Ischemia/reperfusion is a major cause of acute kidney injury (AKI) in humans, and is associated with tubular cell necrosis and endothelial cell dysfunction or loss. Growing evidence has shown that the therapeutic potential of EPC-EVs is superior in terms of acute kidney disease. Vinas et al. (15) used ECFC-derived EVs in an acute kidney injury mouse model and showed that miR-486-5p enriched ECFC exosomes significantly reduced ischemia-induced kidney injury. Histologically, exosome treatment decreased the infiltration of neutrophils along with diminished apoptosis and caspase-3 activation. Moreover, administration of exosomes to acute kidney injury-induced animals caused potent protection against kidney injury after 24 h, as evidenced by normalization of plasma creatinine and blood urea nitrogen to the same level as that in the healthy control. Mechanistically, miR-486-5p enriched ECFC exosomes target to reduce the phosphatase and tensin homolog, and stimulate the Akt phosphorylation pathway for ischemic tissue preservation (15). Cantaluppi et al. (13) demonstrated that EPC-EVs carrying miR-126 and miR-296 protect against experimental acute renal IRI, as evidenced by a significant decrease in serum creatinine and blood urea nitrogen levels and improvement in histological signs of microvascular and tubular injury. It is well-known that EPC-EVs exert miR-126 and have strong angiogenic and anti-apoptotic potential (23). In another study, EPC-EV transplantation rescued an experimental model of anti-Thy1.1-induced glomerulonephritis via inhibition of antibody- and complement-mediated injury of mesangial cells (50). In a review article, Sun et al. (51) summarized that stem/progenitor cell-derived EVs, including EPC-EVs, have beneficial effects such as anti-inflammatory, anti-apoptotic, anti-fibrotic, and may also promote renal cancer progression. In summary, EPC-EVs were shown to have a strong renoprotective effect in an acute kidney injury model, and future studies are warranted to extend their application to chronic kidney diseases.
Accumulating evidence demonstrates that EPCs have beneficial effects on bone regeneration by secreting trophic and paracrine factors (52, 53). Pang et al. (54) showed that EPCs modulate the survival, migration, and differentiation potential of osteoclast precursors through the VEGFR-2, CXCR4, Smad2/3, Akt, ERK1, and p38 MAPK pathways (Figure 6). Interestingly, target genes of highly expressed EPC-EV miRs yielded several significant bone and osteoblast differentiation-enriched functional categories (Figure 7). Through in silico experiments, Qin et al. (55) showed that EPC-EVs regulate the osteoblastic differentiation of bone marrow-derived mesenchymal stromal cells by inhibiting the expression of osteogenic genes and increasing proliferation. This suggests that EPC-EVs are able to control osteogenesis and have beneficial effects on connective tissue development, such as fibroblasts and chondrocytes (Figure 7). A preclinical study showed that EPC-EVs have a strong therapeutic effect on distraction osteogenesis by stimulating angiogenesis and osteogenesis (56). The aforementioned therapeutic advantage of EPC-EVs in bone and connective tissue regeneration expands its application to cure various skeletal muscle diseases.
Figure 6. Highly expressed ECFC-EC-derived miRs biological targets were predicted, and the most significantly enriched functional annotations were cell migration and cell cycle. This miR sequence data was generated from PMID: 28631889. MicroRNA target genes were predicted using IPA with information from TargetScan, miRecords, and TarBase databases.
Figure 7. Bioinformatics analysis showed that significantly enriched functional annotation related to bone and connective tissue regeneration. This miR sequence data was generated from PMID: 28631889. MicroRNA target genes were predicted using IPA with information from TargetScan, miRecords, and TarBase databases.
Recent studies have shed light on the biological activity and function of EPC-Ev-derived miRs in various in vitro and in vivo models. Dellet et al. (35) demonstrated the high expression levels of 15 miRs identified in ECFC and ECFC-derived EVs such as miR-10a/b, miR-21-5p, miR-30a-5p, miR-126-5p, let-7 families, and miR151a-3p (Figure 3 and Table 1). We further investigated the angiogenic/vasculogenic properties of EPC-EV-derived miRs in silico. As shown in Figure 4, the EPC-EVs-derived miR targets are expressed on angiogenesis- and vasculogenesis-related genes. The majority of the neovasculogenesis phenomenon is coupled with cardiovascular system development and function (Figure 4). Plummder et al. (59) reported that EPC-derived miR-10b and miR-196b overexpression activates VEGF, and the latter enhances breast tumor vasculature. Interestingly, downregulation of miR-10b and miR-196b significantly inhibited tumor angiogenesis in mice, indicating a strong angiogenic potential. miR-126-5p and miR-126-3p overexpression promoted EPC migration and tube-like structure formation in ischemic cardiomyopathy patients in vitro (57). Moreover, transplantation of miR-126-3p-overexpressing EPCs into a rat model of MI showed left ventricular hemodynamic functions along with histological improvements (57). Mathiyalagan et al. (23) also demonstrated that silencing miR-126-3p from CD34 cell-derived exosomes abolished their angiogenic activity and beneficial function both in vitro and in vivo. Furthermore, injection of CD34 cell-derived exosomes increased miR-126-3p levels in mouse ischemic limbs but did not affect the endogenous synthesis of miR-126-3p, indicating a direct transfer of functional miR-126-3p to the ischemic tissue (23).
Previous cell therapy trials have reported that the efficacy of cell therapy is limited by poor engraftment of cells or that engrafted cells disappear several months after transplantation, suggesting a paracrine-based effect on the tissues. Depending on the disease state and location, EV transplantation routes may differ. Classical intravenous transplantation of EPC-EVs has been widely used in preclinical and clinical studies (12, 67) (Table 2). Several beneficial functions of systemic transplantation are listed, including (i) no requirement for special sophisticated delivery techniques, (ii) the immunomodulatory effect of EPC-EVs, and (iii) option for repetitive transplantation that is advantageous for local delivery. Sometimes, the desired results cannot be obtained after one injection of EVs; consequently, repetitive systemic transplantation via the vein is needed, whereas in several diseases, local transplantation is not allowed for this technique. Recently, Yi et al. (27) reviewed 29 publications on the route of administration in preclinical studies and showed that the intravenous route was selected in ~80% of exosome injections, and the remaining exosome delivery routes were intraperitoneal, oral, or local. For instance, an ongoing phase one clinical trial (NCT04327635) on safety evaluation of intracoronary infusion of EV in patients with acute myocardial infarction performs within 20 min after stent placement or post-dilation (whichever is last) (Table 2). This kind of delivery methodology is widely used in previous/also current cell transplantation trials into stent placement or post-dilation vessels to enhance the treatment of damaged organs. Another completed clinical trial (NCT04134676) primary outcome revealed that stem cell-conditioned media-derived EVs therapeutic potential is promising in terms of chronic ulcer size reduction, edema decrease, and presence of granulation signs (Table 2). These macroscopic findings were reported 2 weeks after local delivery of EVs via gel.
In most cases, intravenously transplanted EVs accumulate in the liver, lung, spleen, and kidney (27). For target organ delivery, it has been shown that the local tissue inflammatory environment and activation of receptors and ligands (adhesion molecules) play essential roles in EV uptake. This information is valuable for in vivo biodistribution of exosomes and the control of dose and potential side effects.
To achieve better results, a targeted delivery system with sustained release to damaged organs may be required. Chen et al. (33) demonstrated that the injection of EPC-EVs incorporated with shear-thinning gel into the border zone of myocardial infarction improved the hemodynamic function of the heart. The average steady EPC-EV release from the gel continued for over 21 days. This EV delivery strategy may enhance EV retention by damaged tissue owing to the sustained release and has potential for active use in trophic ulcer treatment.
The mechanism of EV internalization into recipient or acceptor cells is crucial in terms of intercellular communication. Several EV internalization mechanisms have been presented previously in the scientific literature, such as direct uptake followed by fusion, phagocytosis, and macropinocytosis by the recipient cell membrane (25). Indirect EV uptake mechanisms are sophisticated and work through other pathways, such as the clathrin-dependent and clathrin-independent pathways and lipid raft-mediated, caveolin-mediated, and cell surface protein-mediated endocytosis (24, 25, 68). In addition, recent reports revealed that tissue microenvironment pH is a crucial factor for EV uptake and secretion (69); for instance, in a rodent myocardial ischemia injury model, MSC-EVs internalization into ischemic cardiomyocytes was enhanced compared to that in the non-ischemic counterparts, indicating a low pH condition as the likely mechanism (69, 70). Another factor that is common for the preferential accumulation of EPCs and hematopoietic cells in ischemic tissue is the SDF-1/CXCR4 system (71–73). Recently, Viñas et al. (14) showed that CXCR4/SDF-1α interaction plays an essential role in EPC-derived exosome uptake in a mouse acute kidney ischemia-reperfusion injury model. Interestingly, EPC-EVs selectively targeted the ischemic kidney tissues. Hence, transplanted EPC-EVs were detected 30 min to 4 h after reperfusion only within the proximal tubules, glomeruli, and endothelial cells. However, this preferential internalization into the ischemic kidney was interrupted when exosomes were pre-incubated with the CXCR4 inhibitor plerixafor, suggesting CXCR4/SDF-1 α-dependent EPC-EV uptake in ischemic tissues. Taken together, EPC-EVs internalize to the target cells of the CXCR4/SDF-1α system under ischemic conditions, similar to EPCs, although other EPC-EV internalization mechanisms are essential for non-ischemic diseases.
One of the major challenges for the prolonged clinical applicability of EVs is the establishment of proper and reproducible preservation and storage conditions without compromising their therapeutic potential. Several studies have shown that different methods of storage, chemical compounds, and temperature range optimization are crucial before translation to the clinic (74–76). Recently, Wu et al. (77) evaluated the effect of storage temperature by storing EVs at 4 °C, −20 °C, and −80 °C for up to 28 days and comparing them to fresh EVs. In comparison to fresh EVs, 1 month of storage at 4 °C and −20 °C changed the size distribution, decreased the quantity and content, and affected cellular uptake and biodistribution of EVs; however, storage at −80 °C did not show such effects. The authors concluded that storage at 4 °C or −20 °C is suitable for short-term preservation, whereas −80 °C would be preferable for long-term preservation of EVs for therapeutic applications (77). Jin et al. (78) reported that EVs are stable under the conditions of 4 °C (for 24, 72, and 168 h), at room temperature (for 6, 12, 24, and 48 h), and repeated freeze-thaw (from one to five times).
Moreover, the assessment of DNA content and functionality in EVs was stable in a changing environment over repeated freeze-thaw cycles (78). Freeze-drying or lyophilization seems to be the most reliable method for preserving EVs (76). The common stabilizers used in lyophilization are disaccharides such as glucose, lactose, sucrose, and trehalose. A comparative study of EV storage at 4 °C or −80 °C and freeze-drying showed that lyophilization preserves size and enzyme activity which are indicators of EV stability (79). In summary, for long-term EV storage, preferable conditions are deep freezing, such as at −80 °C or below, whereas 4 °C may be acceptable for short-term use. For advanced EV applications, it is preferable to store EV using lyophilization methods to optimize the biological function and therapeutic potential of EVs.
Intensive research on endothelial progenitor cells and translation to the clinic for various cardiovascular ischemia diseases has increased our understanding of their therapeutic mechanisms (e.g., paracrine mechanism-based action) and biological function (80–82). EPC-EVs may be considered as a primary candidate for use against certain ischemic diseases, owing to their strong angiogenic, anti-fibrosis, and immunomodulatory properties (12, 20, 21, 23, 33, 57, 59, 80, 82–86) and safety in clinical settings (Table 2). However, there are hurdles to overcome before EPC-EVs can be applied as therapies such as standardization of classification and nomenclature of EPCs and focusing on the question of which EPCs should be used (9, 87). In addition, depending on the origin, such as tissue-derived or circulating EPCs, EPC-EVs cargo may contain/comprise various genetic materials that could influence the clinical outcome and should be carefully considered before therapy. Another aspect that needs to be addressed is EPC culturing conditions, including the effect of culture media, ischemia preconditioning, and composition of EVs, all of which must be investigated precisely using large animal disease models. The development of optimized and scalable isolation of pure, clinical-grade EPC-EVs for off-the-shelf therapy use will increase their significance. To date, most EV-based studies have used intravenous bolus injection methods, although the choice of the EV delivery route depends on the location of the disease.
Nevertheless, completed and ongoing clinical trials (Table 2), as well as numerous preclinical studies (38, 42, 44, 45, 86, 88), indicate that EPC-EV therapy is feasible and that EVs are safe and well-tolerated.
AS and AK contributed to the literature research and data collection, and were involved in the draft of the manuscript. AK contributed to figure generation and bioinformatic analyses. AS, SK, and TA contributed to the coordination and design of the review and writing of the final draft of the manuscript. All authors have read and approved the final manuscript.
This research was supported by JSPS KAKENHI funding (grant no. 20K17163 to AS).
The authors declare that the research was conducted in the absence of any commercial or financial relationships that could be construed as a potential conflict of interest.
All claims expressed in this article are solely those of the authors and do not necessarily represent those of their affiliated organizations, or those of the publisher, the editors and the reviewers. Any product that may be evaluated in this article, or claim that may be made by its manufacturer, is not guaranteed or endorsed by the publisher.
1. Asahara T, Murohara T, Sullivan A, Silver M, van der Zee R, Li T, et al. Isolation of putative progenitor endothelial cells for angiogenesis. Science. (1997) 275:964–6. doi: 10.1126/science.275.5302.964
2. Tateishi-Yuyama E, Matsubara H, Murohara T, Ikeda U, Shintani S, Masaki G. Therapeutic angiogenesis for patients with limb ischaemia by autologous transplantation of bone-marrow cells: a pilot study and a randomised controlled trial. Lancet. (2002) 360:427–35. doi: 10.1016/S0140-6736(02)09670-8
3. Lin Y, Weisdorf JD, Solovey A, Hebbel PR. Origins of circulating endothelial cells and endothelial outgrowth from blood. J Clin Invest. (2000) 105:71–7. doi: 10.1172/JCI8071
4. Ingram DA, Mead LE, Tanaka H, Meade V, Fenoglio A, Mortell K, et al. Identification of a novel hierarchy of endothelial progenitor cells using human peripheral and umbilical cord blood. Blood. (2004) 104:2752–60. doi: 10.1182/blood-2004-04-1396
5. Asahara T, Masuda H, Takahashi T, Kalka C, Pastore C, Silver M, et al. Bone marrow origin of endothelial progenitor cells responsible for postnatal vasculogenesis in physiological and pathological neovascularization. Circ Res. (1999) 85:221–8. doi: 10.1161/01.RES.85.3.221
6. Bailey AS, Jiang S, Afentoulis M, Baumann CI, Schroeder AD, Olson SB, et al. Transplanted adult hematopoietic stems cells differentiate into functional endothelial cells. Blood. (2004) 103:13–9. doi: 10.1182/blood-2003-05-1684
7. Peters BA, Diaz LA, Polyak K, Meszler L, Romans K, Guinan EC, et al. Contribution of bone marrow-derived endothelial cells to human tumor vasculature. Nat Med. (2005) 11:261–2. doi: 10.1038/nm1200
8. Göthert JR, Gustin SE, van Eekelen JM, Schmidt U, Hall MA, Jane SM, et al. Genetically tagging endothelial cells in vivo: bone marrow-derived cells do not contribute to tumor endothelium. Blood. (2004) 104: 1769–77. doi: 10.1182/blood-2003-11-3952
9. Medina RJ, Barber CL, Sabatier F, Dignat-George F, Melero-Martin JM, Khosrotehrani K, et al. Endothelial progenitors: a consensus statement on nomenclature. Stem Cells Transl Med. (2017) 6:1316–20. doi: 10.1002/sctm.16-0360
10. Hur J, Yoon C, Kim H, Choi J, Kang H, Hwang K, et al. Characterization of two types of endothelial progenitor cells and their different contributions to neovasculogenesis. Arterioscler Thromb Vasc Biol. (2004) 24: 288–93. doi: 10.1161/01.ATV.0000114236.77009.06
11. O'Neill CL, Guduric-Fuchs J, Chambers SE, O'Doherty M, Bottazzi B, Stitt AW, et al. Endothelial cell-derived pentraxin 3 limits the vasoreparative therapeutic potential of circulating angiogenic cells. Cardiovasc Res. (2016) 112:677–88. doi: 10.1093/cvr/cvw209
12. Ranghino A, Cantaluppi V, Grange C, Vitillo L, Fop F, Biancone L, Deregibus MC, et al. Endothelial progenitor cell-derived microvesicles improve neovascularization in a murine model of hindlimb ischemia. Int J Immunopathol Pharmacol. (2012) 25:75–85. doi: 10.1177/039463201202500110
13. Cantaluppi V, Gatti S, Medica D, Figliolini F, Bruno S, Deregibus MC, et al. Microvesicles derived from endothelial progenitor cells protect the kidney from ischemia-reperfusion injury by microRNA-dependent reprogramming of resident renal cells. Kidney Int. (2012) 82: 412–27. doi: 10.1038/ki.2012.105
14. Viñas JL, Spence M, Gutsol A, Knoll W, Burger D, Zimpelmann J, et al. Receptor-ligand interaction mediates targeting of endothelial colony forming cell-derived exosomes to the kidney after ischemic injury. Sci Rep. (2018) 8:1–12. doi: 10.1038/s41598-018-34557-7
15. Viñas JL, Burger D, Zimpelmann J, Haneef R, Knoll W, Campbell P, et al. Transfer of microRNA-486-5p from human endothelial colony forming cell-derived exosomes reduces ischemic kidney injury. Kidney Int. (2016) 90:1238–50. doi: 10.1016/j.kint.2016.07.015
16. Théry C, Witwer KW, Aikawa E, Alcaraz MJ, Anderson JD, Andriantsitohaina R, et al. Minimal information for studies of extracellular vesicles 2018 (MISEV2018): a position statement of the International Society for Extracellular Vesicles and update of the MISEV2014 guidelines. J Extracell Vesicles. (2018) 7:1535750. doi: 10.1080/20013078.2018.1535750
17. Niel Gv, D'Angelo G, Raposo G. Shedding light on the cell biology of extracellular vesicles. Nat Rev Mol Cell Biol. (2018) 19:213–28. doi: 10.1038/nrm.2017.125
18. Raposo G, Stoorvogel W. Extracellular vesicles: exosomes, microvesicles, and friends. J Cell Biol. (2013) 200:373–83. doi: 10.1083/jcb.201211138
19. Sahoo S, Adamiak M, Mathiyalagan P, Kenneweg F, Kafert-Kasting S, Thum T. Therapeutic and diagnostic translation of extracellular vesicles in cardiovascular diseases: roadmap to the clinic. Circulation. (2021) 143:1426–49. doi: 10.1161/CIRCULATIONAHA.120.049254
20. Deregibus MC, Cantaluppi V, Calogero R, Iacono ML, Tetta C, Biancone L, et al. Endothelial progenitor cell derived microvesicles activate an angiogenic program in endothelial cells by a horizontal transfer of mRNA. Blood. (2007) 110:2440–8. doi: 10.1182/blood-2007-03-078709
21. Sahoo S, Klychko E, Thorne T, Misener S, Schultz KM, Millay M, et al. Exosomes from human CD34(+) stem cells mediate their proangiogenic paracrine activity. Circ Res. (2011) 109:724–8. doi: 10.1161/CIRCRESAHA.111.253286
22. Bitzer M, Ben-Dov IZ, Thum T. Microparticles and microRNAs of endothelial progenitor cells ameliorate acute kidney injury. Kidney Int. (2012) 82:375–7. doi: 10.1038/ki.2012.152
23. Mathiyalagan P, Liang Y, Kim D, Misener S, Thorne T, Kamide CE, et al. Angiogenic mechanisms of human CD34(+) stem cell exosomes in the repair of ischemic hindlimb. Circ Res. (2017) 120:1466–76. doi: 10.1161/CIRCRESAHA.116.310557
24. Mathieu M, Martin-Jaular L, Lavieu G, Théry C. Specificities of secretion and uptake of exosomes and other extracellular vesicles for cell-to-cell communication. Nat Cell Biol. (2019) 21:9–17. doi: 10.1038/s41556-018-0250-9
25. Mulcahy LA, Pink RC, Carter DR. Routes and mechanisms of extracellular vesicle uptake. J Extracell Vesicles. (2014) 3. doi: 10.3402/jev.v3.24641
26. Toribio V, Morales S, López-Martín S, Cardeñes B, Cabañas C, Yáñez-Mó M. Development of a quantitative method to measure EV uptake. Sci Rep. (2019) 9:10522. doi: 10.1038/s41598-019-47023-9
27. Yi YW, Lee JH, Kim S, Pack C, Ha DH, Park SR, et al. Advances in Analysis of Biodistribution of Exosomes by Molecular Imaging. Int J Mol Sci. (2020) 21:665. doi: 10.3390/ijms21020665
28. Sanganalmath SK, Bolli R. Cell therapy for heart failure: a comprehensive overview of experimental and clinical studies, current challenges, and future directions. Circ Res. (2013) 113:810–34. doi: 10.1161/CIRCRESAHA.113.300219
29. Afzal MR, Samanta A, Shah ZI, Jeevanantham V, Abdel-Latif A, Zuba-Surma EK, et al. Adult bone marrow cell therapy for ischemic heart disease: evidence and insights from randomized controlled trials. Circ Res. (2015) 117:558–75. doi: 10.1161/CIRCRESAHA.114.304792
30. Medina RJ, O'Neill CL, Sweeney M, Guduric-Fuchs J, Gardiner TA, Simpson DA, et al. Molecular analysis of endothelial progenitor cell (EPC) subtypes reveals two distinct cell populations with different identities. BMC Med Genomics. (2010) 3:18. doi: 10.1186/1755-8794-3-18
31. Liu W, Zhang H, Mai J, Chen Z, Huang T, Wang S, et al. Distinct anti-fibrotic effects of exosomes derived from endothelial colony-forming cells cultured under normoxia and hypoxia. Med Sci Monit. (2018) 24:6187–99. doi: 10.12659/MSM.911306
32. Minami Y, Nakajima T, Ikutomi M, Morita T, Komuro I, Sata M, et al. Angiogenic potential of early and late outgrowth endothelial progenitor cells is dependent on the time of emergence. Int J Cardiol. (2015) 186:305–14. doi: 10.1016/j.ijcard.2015.03.166
33. Chen CW, Wang LL, Zaman S, Gordon J, Arisi MF, Venkataraman CM, et al. Sustained release of endothelial progenitor cell-derived extracellular vesicles from shear-thinning hydrogels improves angiogenesis and promotes function after myocardial infarction. Cardiovasc Res. (2018) 114:1029–40. doi: 10.1093/cvr/cvy067
34. Ong S, Wu JC. Exosomes as potential alternatives to stem cell therapy in mediating cardiac regeneration. Circ Res. (2015) 117:7–9. doi: 10.1161/CIRCRESAHA.115.306593
35. Dellett M, Brown ED, Guduric-Fuchs J, O'Connor A, Stitt AW, Medina RJ, et al. MicroRNA-containing extracellular vesicles released from endothelial colony-forming cells modulate angiogenesis during ischaemic retinopathy. J Cell Mol Med. (2017) 21:3405–19. doi: 10.1111/jcmm.13251
36. Editorial TL. Life, death, and disability in 2016. Lancet. (2017) 390:1083. doi: 10.1016/S0140-6736(17)32465-0
37. Liu H, Gong Y, Yu C, Sun Y, Li X, Zhao D, et al. Endothelial progenitor cells in cardiovascular diseases: from biomarker to therapeutic agent. Regenerat Med Res. (2013) 1:1–5. doi: 10.1186/2050-490X-1-9
38. Yue Y, Wang C, Benedict C, Huang G, Truongcao M, Roy R, et al. Interleukin-10 deficiency alters endothelial progenitor cell-derived exosome reparative effect on myocardial repair via integrin-linked kinase enrichment. Circ Res. (2020) 126:315–29. doi: 10.1161/CIRCRESAHA.119.315829
39. Mackie AR, Klyachko E, Thorne T, Schultz KM, Millay M, Ito A, et al. Sonic hedgehog-modified human CD34+ cells preserve cardiac function after acute myocardial infarction. Circ Res. (2012) 122:312–21. doi: 10.1161/CIRCRESAHA.112.266015
40. Salybekov AA, Salybekova AK, Pola R, Asahara T. sonic hedgehog signaling pathway in endothelial progenitor cell biology for vascular medicine. Int J Mol Sci. (2018) 19:3040. doi: 10.3390/ijms19103040
41. Ke X, Yang R, Wu F, Wang X, Liang J, Hu X, et al. Exosomal miR-218-5p/miR-363-3p from endothelial progenitor cells ameliorate myocardial infarction by targeting the p53/JMY signaling pathway. Oxid Med Cell Longevity. (2021) 2021:5529430. doi: 10.1155/2021/5529430
42. Ke X, Yang D, Liang J, Wang X, Wu S, Wang X, et al. Human endothelial progenitor cell-derived exosomes increase proliferation and angiogenesis in cardiac fibroblasts by promoting the mesenchymal-endothelial transition and reducing high mobility group box 1 protein B1 expression. DNA Cell Biol. (2017) 36:1018–28. doi: 10.1089/dna.2017.3836
43. Dushianthan A, Grocott MPW, Postle AD, Cusack R. Acute Respiratory Distress Syndrome and Acute Lung Injury. BMJ Publishing Group Limited (2011). doi: 10.1136/pgmj.2011.118398
44. Zhou Y, Li P, Goodwin AJ, Cook JA, Halushka PV, Chang E, et al. Exosomes from endothelial progenitor cells improve outcomes of the lipopolysaccharide-induced acute lung injury. Crit Care. (2019) 23:1–12. doi: 10.1186/s13054-019-2339-3
45. Wu X, Liu Z, Hu L, Gu W, Zhu L. Exosomes derived from endothelial progenitor cells ameliorate acute lung injury by transferring miR-126. Exp Cell Res. (2018) 370:13–23. doi: 10.1016/j.yexcr.2018.06.003
46. Fan H, Goodwin AJ, Chang E, Zingarelli B, Borg K, Guan S, et al. Endothelial progenitor cells and a stromal cell–derived factor-1α analogue synergistically improve survival in sepsis. Am J Respir Crit Care Med. (2014) 189:1509–19. doi: 10.1164/rccm.201312-2163OC
47. Sun R, Huang J, Sun B. Mobilization of endothelial progenitor cells in sepsis. Inflamm Res. (2019) 69:1–9. doi: 10.1007/s00011-019-01299-9
48. Cao J, He X, Xu H, Zou Z, Shi X. Autologous transplantation of peripheral blood-derived circulating endothelial progenitor cells attenuates endotoxin-induced acute lung injury in rabbits by direct endothelial repair and indirect immunomodulation. Anesthesiology. (2012) 116:1278–87. doi: 10.1097/ALN.0b013e3182567f84
49. Zhou Y, Li P, Goodwin AJ, Cook JA, Halushka PV, Chang E, et al. Exosomes from endothelial progenitor cells improve the outcome of a murine model of sepsis. Mol Ther. (2018) 26:1375–84. doi: 10.1016/j.ymthe.2018.02.020
50. Cantaluppi V, Medica D, Mannari C, Stiaccini G, Figliolini F, Dellepiane S, et al. Endothelial progenitor cell-derived extracellular vesicles protect from complement-mediated mesangial injury in experimental anti-Thy1.1 glomerulonephritis. Nephrol Dialysis Transplant. (2015) 30:410–22. doi: 10.1093/ndt/gfu364
51. Sun X, Meng H, Wan W, Xie M, Wen C. Application potential of stem/progenitor cell-derived extracellular vesicles in renal diseases. Stem Cell Res Ther. (2019) 10. doi: 10.1186/s13287-018-1097-5
52. Bates BD, Godbout C, Ramnaraign DJ, Schemitsch EH, Nauth A. Delayed endothelial progenitor cell therapy promotes bone defect repair in a clinically relevant rat model. Stem Cells Int. (2017) 2017:7923826. doi: 10.1155/2017/7923826
53. Cui Y, Fu S, Sun D, Xing J, Hou T, Wu X. EPC-derived exosomes promote osteoclastogenesis through LncRNA-MALAT1. J Cell Mol Med. (2019) 23:3843–54. doi: 10.1111/jcmm.14228
54. Pang H, Wu X, Fu S, Luo F, Zhang Z, Hou T, et al. Co-culture with endothelial progenitor cells promotes survival, migration, and differentiation of osteoclast precursors. Biochem Biophys Res Commun. (2013) 430:729–34. doi: 10.1016/j.bbrc.2012.11.081
55. Qin Y, Zhang C. Endothelial progenitor cell-derived extracellular vesicle-meditated cell-to-cell communication regulates the proliferation and osteoblastic differentiation of bone mesenchymal stromal cells. Mol Med Rep. (2017) 16:7018–24. doi: 10.3892/mmr.2017.7403
56. Jia Y, Zhu Y, Qiu S, Xu J, Chai Y. Exosomes secreted by endothelial progenitor cells accelerate bone regeneration during distraction osteogenesis by stimulating angiogenesis. Stem Cell Res Ther. (2019) 10:1–13: doi: 10.1186/s13287-018-1115-7
57. Li H, Liu Q, Wang N, Xu Y, Kang L, Ren Y, et al. Transplantation of endothelial progenitor cells overexpressing miR-126-3p improves heart function in ischemic cardiomyopathy. Circ J. (2018) 82:2332–41. doi: 10.1253/circj.CJ-17-1251
58. Schober A, Nazari-Jahantigh M, Wei Y, Bidzhekov K, Gremse F, Grommes J, et al. MicroRNA-126-5p promotes endothelial proliferation and limits atherosclerosis by suppressing Dlk1. Nat Med. (2014) 20:368–76. doi: 10.1038/nm.3487
59. Plummer PN, Freeman R, Taft RJ, Vider J, Sax M, Umer BA, et al. MicroRNAs regulate tumor angiogenesis modulated by endothelial progenitor cells. Cancer Res. (2013) 73:341–52. doi: 10.1158/0008-5472.CAN-12-0271
60. Hassel D, Cheng P, White MP, Ivey KN, Kroll J, Augustin HG, et al. MicroRNA-10 regulates the angiogenic behavior of zebrafish and human endothelial cells by promoting vascular endothelial growth factor signaling. Circ Res. (2012) 111:1421–33. doi: 10.1161/CIRCRESAHA.112.279711
61. Roush S, Slack FJ. The let-7 family of microRNAs. Trends Cell Biol. (2008) 18:505–16. doi: 10.1016/j.tcb.2008.07.007
62. Zhao T, Li J, Chen AF. MicroRNA-34a induces endothelial progenitor cell senescence and impedes its angiogenesis via suppressing silent information regulator 1. Am J Physiol Endocrinol Metab. (2010) 299:E110–6. doi: 10.1152/ajpendo.00192.2010
63. Landskroner-Eiger S, Moneke I, Sessa WC. miRNAs as modulators of angiogenesis. Cold Spring Harbor Perspect Med. (2013) 3:a006643. doi: 10.1101/cshperspect.a006643
64. Wang Z, Xu L, Hu Y, Huang Y, Zhang Y, Zheng X, et al. miRNA let-7b modulates macrophage polarization and enhances tumor-associated macrophages to promote angiogenesis and mobility in prostate cancer. Sci Rep. (2016) 6:25602. doi: 10.1038/srep25602
65. Würdinger T, Tannous BA, Saydam O, Skog J, Grau S, Soutschek J, et al. miR-296 regulates growth factor receptor overexpression in angiogenic endothelial cells. Cancer Cell. (2008) 14:382–93. doi: 10.1016/j.ccr.2008.10.005
66. Wang W, Li C, Li W, Kong L, Qian A, Hu N, et al. MiR-150 enhances the motility of EPCs in vitro and promotes EPCs homing and thrombus resolving in vivo. Thromb Res. (2014) 133:590–8. doi: 10.1016/j.thromres.2013.12.038
67. Angulski ABB, Capriglione LGA, Barchiki F, Brofman P, Stimamiglio MA, Senegaglia AC, et al. Systemic infusion of expanded CD133 + cells and expanded CD133 + cell-derived EVs for the treatment of ischemic cardiomyopathy in a rat model of AMI. Stem Cells Int. (2019) 2019:4802578. doi: 10.1155/2019/4802578
68. Verdera HC, Gitz-Francois JJ, Schiffelers RM, Vader P. Cellular uptake of extracellular vesicles is mediated by clathrin-independent endocytosis and macropinocytosis. J Controll Release. (2017) 266:100–8. doi: 10.1016/j.jconrel.2017.09.019
69. Parolini I, Federici C, Raggi C, Lugini L, Palleschi S, De Milito A, et al. Microenvironmental pH is a key factor for exosome traffic in tumor cells. J Biol Chem. (2009) 284:34211–22. doi: 10.1074/jbc.M109.041152
70. Lai RC, Yeo RW, Tan KH, Lim SK. Mesenchymal stem cell exosome ameliorates reperfusion injury through proteomic complementation. Regenerat Med. (2013) 8:197–209. doi: 10.2217/rme.13.4
71. Ceradini DJ, Kulkarni AR, Callaghan MJ, Tepper OM, Bastidas N, Kleinman ME, et al. Progenitor cell trafficking is regulated by hypoxic gradients through HIF-1 induction of SDF-1. Nat Med. (2004) 10:858–64. doi: 10.1038/nm1075
72. van Solingen C, de Boer HC, Bijkerk R, Monge M, van Oeveren-Rietdijk AM, Seghers, et al. MicroRNA-126 modulates endothelial SDF-1 expression and mobilization of Sca-1(+)/Lin(-) progenitor cells in ischaemia. Cardiovasc Res. (2011) 92:449–55. doi: 10.1093/cvr/cvr227
73. Yamaguchi J, Kusano KF, Masuo O, Kawamoto A, Silver M, Murasawa S, et al. Stromal cell-derived factor-1 effects on ex vivo expanded endothelial progenitor cell recruitment for ischemic neovascularization. Circulation. (2003) 107:1322–8. doi: 10.1161/01.CIR.0000055313.77510.22
74. Gandham S, Su X, Wood J, Nocera AL, Alli SC, Milane L, et al. Technologies and standardization in research on extracellular vesicles. Trends Biotechnol. (2020) 38:1066–98. doi: 10.1016/j.tibtech.2020.05.012
75. Reiner AT, Witwer KW, van Balkom BWM, de Beer J, Brodie C, Corteling RL, et al. Concise review: developing best-practice models for the therapeutic use of extracellular vesicles. Stem Cells Transl Med. (2017) 6:1730–9. doi: 10.1002/sctm.17-0055
76. Kusuma GD, Barabadi M, Tan JL, Morton DAV, Frith JE, Lim R. To Protect and to preserve: novel preservation strategies for extracellular vesicles. Front Pharmacol. (2018) 9:1199. doi: 10.3389/fphar.2018.01199
77. Wu J, Li Y, Hu X, Huang S, Xiang D. Preservation of small extracellular vesicles for functional analysis and therapeutic applications: a comparative evaluation of storage conditions. Drug Delivery. (2021) 28:162–70. doi: 10.1080/10717544.2020.1869866
78. Jin Y, Chen K, Wang Z, Wang Y, Liu J, Lin L, et al. DNA in serum extracellular vesicles is stable under different storage conditions. BMC Cancer. (2016) 16:753. doi: 10.1186/s12885-016-2783-2
79. Frank J, Richter M, de Rossi C, Lehr C, Fuhrmann K, Fuhrmann G. Extracellular vesicles protect glucuronidase model enzymes during freeze-drying. Sci Rep. (2018) 8:1–8. doi: 10.1038/s41598-018-30786-y
80. Doyle B, Sorajja P, Hynes B, Kumar AHS, Araoz PA, Stalboerger PG, et al. Progenitor cell therapy in a porcine acute myocardial infarction model induces cardiac hypertrophy, mediated by paracrine secretion of cardiotrophic factors including TGFbeta1. Stem Cells Dev. (2008) 17:941–51. doi: 10.1089/scd.2007.0214
81. Di Santo S, Seiler S, Fuchs A, Staudigl J, Widmer HR. The secretome of endothelial progenitor cells promotes brain endothelial cell activity through PI3-kinase and MAP-kinase. PLoS ONE. (2014) 9:e95731. doi: 10.1371/journal.pone.0095731
82. Mirotsou M, Jayawardena TM, Schmeckpeper J, Gnecchi M, Dzau VJ. Paracrine mechanisms of stem cell reparative and regenerative actions in the heart. J Mol Cell Cardiol. (2011) 50:280–9. doi: 10.1016/j.yjmcc.2010.08.005
83. Bruyndonckx L, Hoymans VY, Frederix G, De Guchtenaere A, Franckx H, Vissers DK, et al. Endothelial progenitor cells and endothelial microparticles are independent predictors of endothelial function. J Pediatr. (2014) 165:300–5. doi: 10.1016/j.jpeds.2014.04.015
84. Gu S, Zhang W, Chen J, Ma R, Xiao X, Ma X, et al. EPC-derived microvesicles protect cardiomyocytes from Ang II-induced hypertrophy and apoptosis. PLoS ONE. (2014) 9:e85396. doi: 10.1371/journal.pone.0085396
85. Hu H, Wang B, Jiang C, Li R, Zhao J. Endothelial progenitor cell-derived exosomes facilitate vascular endothelial cell repair through shuttling miR-21-5p to modulate Thrombospondin-1 expression. Clin Sci. (2019) 133:1629–44. doi: 10.1042/CS20190188
86. Li X, Chen C, Wei L, Li Q, Niu X, Xu Y, et al. Exosomes derived from endothelial progenitor cells attenuate vascular repair and accelerate reendothelialization by enhancing endothelial function. Cytotherapy. (2016) 18:253–62. doi: 10.1016/j.jcyt.2015.11.009
87. Coumans FAW, Brisson AR, Buzas EI, Dignat-George F, Drees EEE, El-Andaloussi S, et al. Methodological guidelines to study extracellular vesicles. Circ Res. (2017) 120:1632–48. doi: 10.1161/CIRCRESAHA.117.309417
Keywords: extracellular vesicles, endothelial progenitor cells, exosomes, miR (microRNA), clinical application of EPC exosomes
Citation: Salybekov AA, Kunikeyev AD, Kobayashi S and Asahara T (2021) Latest Advances in Endothelial Progenitor Cell-Derived Extracellular Vesicles Translation to the Clinic. Front. Cardiovasc. Med. 8:734562. doi: 10.3389/fcvm.2021.734562
Received: 01 July 2021; Accepted: 30 August 2021;
Published: 04 October 2021.
Edited by:
Zhen-Ao Zhao, Hebei North University, ChinaReviewed by:
Jiacheng Sun, University of Alabama at Birmingham, United StatesCopyright © 2021 Salybekov, Kunikeyev, Kobayashi and Asahara. This is an open-access article distributed under the terms of the Creative Commons Attribution License (CC BY). The use, distribution or reproduction in other forums is permitted, provided the original author(s) and the copyright owner(s) are credited and that the original publication in this journal is cited, in accordance with accepted academic practice. No use, distribution or reproduction is permitted which does not comply with these terms.
*Correspondence: Amankeldi A. Salybekov, YS5zYWx5YmVrb3ZAc2hvbmFua2FtYWt1cmEub3IuanA=; Takayuki Asahara, dF9hc2FoYXJhQHNob25hbmthbWFrdXJhLm9yLmpw
Disclaimer: All claims expressed in this article are solely those of the authors and do not necessarily represent those of their affiliated organizations, or those of the publisher, the editors and the reviewers. Any product that may be evaluated in this article or claim that may be made by its manufacturer is not guaranteed or endorsed by the publisher.
Research integrity at Frontiers
Learn more about the work of our research integrity team to safeguard the quality of each article we publish.