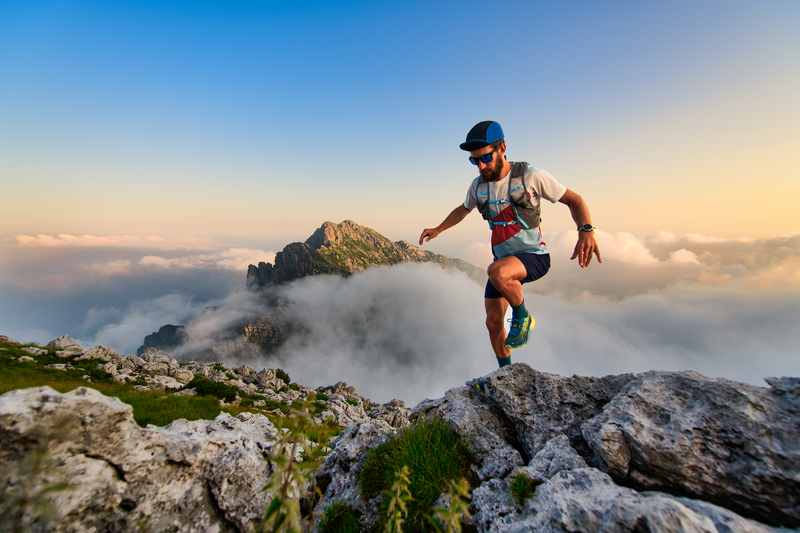
95% of researchers rate our articles as excellent or good
Learn more about the work of our research integrity team to safeguard the quality of each article we publish.
Find out more
REVIEW article
Front. Cardiovasc. Med. , 10 June 2021
Sec. Atherosclerosis and Vascular Medicine
Volume 8 - 2021 | https://doi.org/10.3389/fcvm.2021.688546
Maintaining cholesterol homeostasis is essential for normal cellular and systemic functions. Long non-coding RNAs (lncRNAs) represent a mechanism to fine-tune numerous biological processes by controlling gene expression. LncRNAs have emerged as important regulators in cholesterol homeostasis. Dysregulation of lncRNAs expression is associated with lipid-related diseases, suggesting that manipulating the lncRNAs expression could be a promising therapeutic approach to ameliorate liver disease progression and cardiovascular disease (CVD). However, given the high-abundant lncRNAs and the poor genetic conservation between species, much work is required to elucidate the specific role of lncRNAs in regulating cholesterol homeostasis. In this review, we highlighted the latest advances in the pivotal role and mechanism of lncRNAs in regulating cholesterol homeostasis. These findings provide novel insights into the underlying mechanisms of lncRNAs in lipid-related diseases and may offer potential therapeutic targets for treating lipid-related diseases.
Cholesterol is a crucial organic molecule that exerts pleiotropic functions. Although its presence is crucial to the cell membranes' permeability and fluidity, excessive cholesterol in the bloodstream can be harmful. Therefore, maintaining cholesterol homeostasis is vital to normal cellular functioning. Cellular cholesterol maintains homeostasis by regulating cholesterol synthesis, cholesterol efflux, and cholesterol uptake from lipoprotein carriers (1, 2). The aberrant trafficking and cellular cholesterol homeostasis usually lead to various diseases, including obesity, diabetes, cardiovascular disease, and cancer (2, 3). Recent research suggest that disrupted cholesterol homeostasis can also cause various congenital diseases (4, 5). A growing body of evidence offers a close relationship between cholesterol homeostasis and acquired diseases, including cardiovascular disorders, liver diseases, and several types of cancer.
Long non-coding RNAs (lncRNAs), non-protein coding transcripts longer than 200 nucleotides (>200 bp) are important regulators of genome structure and gene expression. Recently, the contribution of lncRNAs in cholesterol homeostasis has just started to emerge (6, 7). Numerous studies demonstrated that through various regulatory mechanisms, lncRNAs regulate cell development and cell type-specific expression patterns. LncRNAs influenced cholesterol homeostasis and the progression and development of lipid-related diseases, including liver and cardiovascular disease (8, 9). With progress in next-generation sequencing technology, novel lncRNAs have been recognized and their diverse functions identified. However, the regulation by which many of the lncRNAs exhibit their functions is poorly understood. The low homology and conservation of lncRNAs across species pose a problem for developing lncRNA-based therapies. This review summarizes the latest insights on the roles of lncRNAs in cholesterol homeostasis and their potential implication for the treatment of lipid-related diseases.
N6-methyladenosine (m6A) modification, the most prevalent epigenetic methylated modification of lncRNAs in eukaryotes, plays a critical role in RNA splicing, export, stability and translation (10, 11). m6A modification is a dynamic and reversible process that is modulated by m6A regulators, including “writers” (methyltransferases), “readers” (signal transducers) and “erasers” (demethylases) (12). Recent research has revealed that m6A modification regulated lipid metabolism and lipid metabolic disorders in various lipid-related diseases, including liver disease, glioblastoma (GBM) and atherosclerosis (13, 14). For example, EGFR/SRC/ERK signaling-stabilized YTHDF2 (YTH N6-methyladenosine RNA binding protein 2) protein promoted cholesterol dysregulation and facilitated tumorigenesis of GBM cells (15); knockdown of methyltransferase like 14 (METTL14) limited cholesterol efflux (16). The resveratrol alleviated lipid metabolism disorder under HDF by increasing methyltransferase-like 3 (METTL3) expression and decreasing the level of YTHDF3 and m6A abundance (17). Thus, dysregulation of m6A modification is involved in the development of lipid-related disease (18).
Aberrant lncRNA expression is also strongly related to lipid metabolism disorders, and dysregulation of lncRNAs has been confirmed to play a vital role in the progression of liver disease and atherosclerosis (19, 20). For instance, liver-specific triglyceride regulator (lncLSTR), a liver-enriched lncRNA, increased triglyceride levels in a hyperlipidemia mouse model (21). RP11-728F11.4 promoted intracellular cholesterol accumulation and augmented atherosclerotic lesions (22). Knockdown of lncRNA MAARS (macrophage-associated atherosclerosis lncRNA sequence) inhibited atherosclerotic lesion formation in LDLR−/− mice through tethering HuR in the nucleus (23).
Numerous studies have shown that m6A modification is closely related to the dysregulation of lncRNAs (24, 25). METTL3/YTHDF3 complex increased the stability and levels of MALAT1 and X inactive-specific transcript (XIST) (26, 27). MALAT1 and XIST were up-regulated in patients with cardiovascular disease and Stanford type A aortic dissection (TAAD) (28–30). MALAT1 facilitated hepatic lipid accumulation and promote atherosclerotic plaque formation (31, 32). XIST regulated the progression of atherosclerosis and TAAD (33). Thus, m6A modifications led to the lncRNA-dependent lipid-related disease development. Understanding how m6A modifications of lncRNAs are involved in lipid-related disease progression may help identify biomarkers that can act as useful therapeutic targets.
In the last few years, evidence has emerged that lncRNAs play a key role in cholesterol accumulation, cholesterol efflux, cholesterol metabolism, and cholesterol biosynthesis (Table 1 and Figure 1), which were implicated in lipid-related diseases, including liver diseases and cardiovascular diseases (52, 53). Moreover, several lncRNAs participated in the regulation of cholesterol homeostasis in the aorta/macrophages (Figure 2). Some lncRNAs played crucial roles in cholesterol homeostasis in the liver (Figure 3). This review provides a comprehensive insight into the current knowledge regarding lncRNAs involved in regulating cholesterol homeostasis, which identifies potentially useful therapeutic targets for cholesterol modulation.
Figure 1. LncRNAs regulate cholesterol homeostasis in hepatocytes and macrophages. LncRNAs in the liver regulate cholesterol accumulation, cholesterol efflux, cholesterol biosynthesis, and cholesterol metabolism. LncRNAs control cholesterol accumulation and cholesterol efflux in atherosclerotic plaques. LncRNAs regulate ABCA1 expression and inhibit cholesterol efflux to lipid poor apoA-I, which initiates nascent high-density lipoproteins (HDLs). LncRNAs control SREBP1, ACC1, SCD1, FASN, CYP7A1, and ACSL1 expression in hepatocytes. Meantime, lncRNAs also regulate NFIA, ApoE, CDKN2B, RAB22A, CD36, ABCG1, and ADAM10 expression in the macrophages accumulated in atherosclerotic plaques. Free cholesterol in the nascent HDL is further esterified to cholesteryl ester by lecithin-cholesterol acyltransferase (LCAT), which results in the formation of mature HDL particles. Purple indicates lncRNAs. Black represents the target genes regulated by LncRNAs. Blue, inhibit; Red, promote.
Figure 2. The lncRNAs affect cholesterol accumulation and cholesterol efflux from THP-1 macrophage-derived foam cells in atherosclerotic disease. CTCF, CCCTC-binding factor; DNMT1, DNA methyltransferase 1; EZH2, enhancer of zeste homolog 2; HOXC-AS1, lncRNA HOXC cluster antisense RNA 1; Kcnq1ot1, Kcnq1 overlapping transcript 1; LncRNA MAARS, Macrophage-Associated Atherosclerosis lncRNA Sequence; NFIA, nuclear factor IA.
Figure 3. The lncRNA-associated ceRNA networks affect the four common cholesterol transport models of liver diseases. Representative lncRNA-ceRNA networks are listed. They highlighted the involvement of lncRNA-ceRNA networks in four common cholesterol transport models of liver diseases: cholesterol accumulation, cholesterol efflux, cholesterol biosynthesis, and cholesterol metabolism. ACCα, Acetyl-CoA carboxylase α; CaM, calmodulin; FAS, fatty acid synthase; G6Pase, glucose-6-phosphatase; hnRNPA1, heterogeneous nuclear ribonucleoprotein A1; LncLSTR, liver-specific triglyceride regulator; LncSHGL, hepatic gluconeogenesis, and lipogenesis; Mirt2, long non-coding RNA myocardial infarction associated transcript 2; PEPCK, phosphoenolpyruvate carboxykinase.
The lncRNA myocardial infarction-associated transcript (MIAT), as a hypoxia-response gene, is located in chromosome 22q12.1 region. MIAT was markedly elevated in the serum of patients with symptoms of vulnerable atherosclerotic plaque (54). MIAT increased the blood lipids levels, promoted atherosclerotic plaque formation, increased the lipid content, and decreased the collagen content of atherosclerotic plaques in apoE−/− mice (55). Silencing of MIAT attenuated atherosclerosis progression in an advanced atherosclerosis mouse model (54). However, MIAT overexpression aggravated the atherosclerotic damage in apoE−/− mice (55). MIAT facilitated angiogenesis and the expression of inflammatory factors (IL-1β, IL-6, and TNF-a) by activating the PI3K/Akt pathway. MIAT was the target gene of m6A modification. m6A level was reduced with enlarged carotid plaque size and thickness in 207 patients with atherosclerosis compared with 142 healthy people (49). ox-LDL-induced AlkB homolog 1 (ALKBH1) and m6A demethylation further promoted MIAT activity with the hypoxia-inducible factor 1α (HIF1α) motif (−1,940/+166-Luc plasmids) but not with deletion (56). Deficiency of ALKBH1 or HIF1α by siRNA transfection strongly upregulated MIAT expression and the m6A levels in vitro (49). Therefore, MIAT may provide a novel target for the treatment of atherosclerotic disease.
Long intergenic non-protein coding RNA 958 (LINC00958), a lipogenesis-related lncRNA, is located on chromosome 11p15.3 regions. LINC00958 was upregulated in hepatocellular carcinoma (HCC) cells, especially in those with moderate/low differentiation, TNM III/IV stage, and microvascular invasion. LINC00958 knockdown in HCC cells decreased cellular cholesterol and triglyceride levels, whereas LINC00958 overexpression increased cholesterol and triglyceride levels (51). METTL3-mediated m6A modification upregulated LINC00958 expression by stabilizing its RNA transcript and increased lipogenesis to promote HCC progression (51). LINC00958 upregulated hepatoma-derived growth factor (HDGF) expression by sponged miR-3619-5p (51). HDGF facilitated the expression of lipogenic genes, which promoted de novo lipogenesis and tumorigenesis. Thereby, LINC00958 augmented HCC lipogenesis and progression, implying that LINC00958 provided a novel perspective for targeted therapy of HCC.
The H19 gene belongs to the H19-Igf2 locus, is located in an imprinted region of chromosome 11p15.5 near the insulin-like growth factor 2 (IGF2) gene in humans. Compared with normal healthy people, the expression of H19 was higher in the blood of patients with atherosclerosis (57), suggesting that H19 may be involved in atherosclerosis progression. In apoE-/- mice, overexpression of H19 aggravated atherosclerosis progression (58); however, silencing of H19 protected against atherosclerosis (59). Recently, H19 was reported to modulate hepatic metabolic homeostasis in non-alcoholic fatty liver disease (NAFLD). H19 promoted lipogenesis by directly inhibiting miR-130a expression in hepatocytes (60). Meanwhile, miR-130a inhibited lipid accumulation by directly down-regulating peroxisome proliferator-activated receptor γ (PPARγ) expression (60, 61). PPARγ was shown to promote cholesterol efflux by regulating ABCA1 and ABCG1 in plaque in vivo and phagocytes in vitro, which could be blocked by PPARγ siRNA (61). Overexpression of H19 in hepatocytes also promoted lipid accumulation and upregulated the expression of multiple genes involved in lipid synthesis, storage, and breakdown, while deficiency of H19 resulted in decreased lipid accumulation in hepatocytes (39). Therefore, H19 may become a new target for the therapy of lipid-related diseases, such as liver disease and cardiovascular disease.
LncRNA growth arrest-specific 5 (GAS5), located on human chromosome 1q25.1, plays a crucial role in atherosclerosis's pathogenesis. GAS5 was significantly increased in atherosclerosis patients' plaque than in normal people (62).
Overexpression of GAS5 increased lipid accumulation via inhibiting enhancer of zeste homolog 2 (EZH2)-mediated ABCA1 expression by histone methylation in THP-1 macrophage. In contrast, GAS5 knockdown promoted reverse-transportation of cholesterol and inhibited lipid accumulation by upregulating the expression of ABCA1 (63). GAS5 overexpression in apoE−/− mice with atherosclerosis also increased total cholesterol (TC), free cholesterol (FC), cholesterol ester (CE), low-density lipoprotein (LDL) levels, aortic plaque, and lipid accumulation; however, silencing of GAS5 prevented the progression of atherosclerosis (63). Previous studies have shown that GAS5 silencing repressed atherosclerosis's malignant progression (37). Thus, targeting GAS5 might be a promising way for atherosclerosis therapy.
Cholesterol-induced regulator of metabolism RNA (CHROME), also known as PRKRA-AS1, is located in a locus on human chromosome 2q31.2, regulates cellular and systemic cholesterol homeostasis. Analysis of blood and tissue samples from healthy individuals and coronary artery disease (CAD) patients revealed that CHROME is upregulated in the plasma and atherosclerotic plaques of patients with the atherosclerotic disease (35). Using gain- and loss-of-function approaches, CHROME promoted cholesterol efflux and HDL biogenesis in the liver and macrophages via inhibiting the actions of functionally related miRNAs, such as miR-27b, miR-33a/b, and miR-128. Conversely, CHROME knockdown inhibited ABCA1 expression in human hepatocytes and macrophages, which blocks cholesterol efflux and the formation of nascent high-density lipoprotein (HDL) (35). Therefore, CHROME may be a clinical biomarker for treating cholesterol-related diseases.
Maternally expressed gene 3 (MEG3) is a lncRNA located in a locus on chromosome 14q32.2 thought to be associated with human lipid metabolic disorders. A study recently demonstrated that the expression of MEG3 was reduced in serum samples from patients with atherosclerosis (64). MEG3 deficiency remarkably abolished hepatic TG accumulation in HFD mice and ob/ob mice (65, 66). MEG3 alleviated NAFLD after high-content hydrogen water treatment in a mouse model (65). MEG3 expression is negatively correlated with lipogenesis-related genes, including sterol regulatory element-binding protein-1 (SREBP-1), LXRα, Carbohydrate response element-binding protein (ChREBP), Stearyl-coenzyme A desaturase 1 (SCD1), acetyl-CoA carboxylase 1 (ACC1), and fatty acid synthase (FAS), in NAFLD mice (38). MEG3 overexpression significantly inhibited the expression levels of lipogenesis-related genes and lowered FFA-induced lipid accumulation in HepG2 cells. Bioinformatic analysis and mechanistic studies illustrated that MEG3 competitively bound to the miR-21 with LRP6, followed by the inhibition of the mTOR pathway and inhibited hepatic lipogenesis (38). Therefore, the targeted suppression of MEG3 may serve as a potential therapy for lipid-related diseases.
LeXis is a lipid-responsive lncRNA, highly expressed in the hepatic tissue and robustly induced by Western diet (high in fat and cholesterol) and pharmacologic liver X receptors (LXRs) activation (46). Hepatic overexpression of LeXis in mice decreased plasma cholesterol, whereas LeXis knockout mice had the opposite phenotype of increased serum cholesterol level and upregulated cholesterol biosynthetic gene expression (67). Raising or lowering LeXis levels in the liver and plasma affected cholesterol biosynthesis and altered the cholesterol levels by LXRs activation. LXRs are transcriptional regulators of cholesterol homeostasis. Under conditions of excess cholesterol, LXR activation-induced apoE, ABCA1, and ABCG1 expression, which was involved in cholesterol efflux, facilitated cholesterol esterification and inhibited cholesterol uptake (46, 68). Overexpression of LXRs significantly promoted cholesterol efflux via the upregulation of ABCA1 and ABCG1 (69); conversely, shRNA-mediated knockdown suppressed ABCA1 and ABCG1 expression and promoted intracellular cholesterol accumulation (70). Taken together, LeXis has important implications in developing novel therapeutic strategies for treating lipid-related diseases.
CDKN2B-AS1, also known as ANRIL, is located within the CDKN2B-CDKN2A gene cluster at chromosome 9p21 in humans. Prior studies have demonstrated that it was expressed significantly higher in hypertension patients than in healthy controls and was particularly associated with cardiovascular disease (71). Transcript variants of CDKN2B-AS1 have also been shown to play critical regulatory roles in various diseases, including malignant tumors, atherosclerosis, hypertension, and diabetes (72–75). CDKN2B-AS1 promoted cholesterol efflux by inhibiting A disintegrin and metalloprotease 10 (ADAM10) expression in atherosclerosis (34). Overexpression of ADAM10 facilitated the intracellular accumulation of cholesterol, while knockdown of ADAM10 promoted cholesterol efflux. Hence, CDKN2B-AS1 may serve as a biomarker for atherosclerosis.
A novel lncRNA, lipid Associated Single nucleotide polymorphism gEne Region (LASER), is located near SNP rs486394 in chromosome 11q12 region. Clinical studies previously revealed that LASER expression is positively associated with cholesterol levels. LASER is highly expressed in both hepatocytes and peripheral mononuclear cells (PBMCs). siRNAs mediated knockdown of LASER improved intracellular cholesterol levels and affected the cholesterol metabolism genes expression at both protein and mRNA levels by inhibiting proprotein convertase subtilisin/kexin 9 (PCSK9) expression (76). PCSK9, a major determinant of cholesterol homeostasis, is mainly secreted from the liver and enhances circulating low-density lipoprotein cholesterol (LDL-C) concentrations in circulating blood (77). Thus, targeting LASER therapy may be a practical approach to ameliorate cholesterol levels in clinics.
LncRNA HOXC cluster antisense RNA 1 (HOXC-AS1) is located in chromosome 12q13.13 regions and has two exons. By performing microarray analysis and RT-PCR, the expression levels of HOXC-AS1 and homeobox C6 (HOXC6) were both downregulated in human atherosclerotic plaques when compared to normal intima tissues (41). Lentivirus-mediated overexpression of HOXC-AS1 suppressed ox-LDL-induced cholesterol accumulation by promoting HOXC6 expression in THP-1 macrophages (41). Numerous studies have reported that HOX gene networks are involved in human adipogenesis; notably, HOXC6 inhibited intracellular lipid accumulation (78). Thus, HOXC-AS1 could be a promising therapeutic target in preventing atherosclerosis.
lncRNA regulator of Akt signaling associated with HCC and RCC (LncARSR) is located in chromosome 9q21.31 regions. The lncARSR expression level was increased both in patients with hypercholesterolemia and high-cholesterol diet-fed mice (79). Adenoviruses-mediated overexpression of lncARSR in mice contributed to elevated lipid levels in both serum and liver fragments. However, knockdown of lncARSR in mice fed with a high cholesterol diet exhibited a marked reduction in plasma lipid levels than control mice (79). Moreover, lncARSR overexpression facilitated HMG-CoA reductase (HMGCR) expression and the rate-limiting enzyme of cholesterol synthesis, accompanied by the augment of hepatic de novo cholesterol synthesis rate. Mechanistically, lncARSR promoted the expression of SREBP-2, which regulated the expression of cholesterol-related genes, such as HMGCR and LDLR (80). Hence, lncARSR promoted hepatic cholesterol biosynthesis and implied that lncARSR might serve as a therapeutic target for cholesterol homeostasis disorder.
ENST00000602558.1 is located on a CAD, triglyceride (TG), and HDL susceptibility region (chr12q24.31) (81, 82). Li et al. performed a transcriptome-wide overview of aberrantly expressed lncRNAs in CAD patients, ENST00000444488.1 was identified as a novel lncRNA biomarker for diagnosing CAD (83). Overexpression of ENST00000602558.1 downregulated ABCG1 expression and exacerbated lipid accumulation in VSMCs, while knockdown of ENST00000602558.1 upregulated ABCG1 expression and decreased lipid accumulation (36). Thus, ENST00000602558.1 may be a novel biomarker for diagnosing atherosclerosis.
LOC286367 is located in the chromosome 9q31.1 region. By performing bioinformatic analysis of lncRNAs and mRNA differentially expressed in THP-1 macrophages, Ma et al. proposed that LOC286367 and ABCA1 were located on the same chromosome with opposite transcription directions (84). Overexpression of LOC286367 inhibited ABCA1 expression, which resulted in intracellular lipid accumulation (84). ABCA1 overexpression in C57BL/6 mice resulted in an anti-atherogenic profile with reduced plasma cholesterol, free cholesterol, cholesteryl ester, and non-high-density lipoprotein cholesterol (HDL-C) levels, but with increased HDL-C, apoA-I, and apoE levels (85). However, ABCA1 knockout mice displayed increased atherosclerosis compared to control mice (86). Hence, targeting LOC286367 might bring significant benefits to the clinical outcome of atherosclerotic cardiovascular diseases.
RP5-833A20.1 is located in intron 2 of the nuclear factor IA (NFIA) gene. RP5-833A20.1 expression was upregulated, whereas NFIA expression was downregulated in human acute monocytic leukemia macrophage-derived foam cells using microarray analysis (50). RP5-833A20.1 regulated cholesterol homeostasis by NFIA. Lentivirus-mediated NFIA overexpression increased HDL-C circulation, decreased LDL-C cholesterol, and very-low-density lipoprotein cholesterol (VLDL-C) circulation (50), which resulted in the regression of atherosclerosis in apoE−/− mice. Thus, RP5-833A20.1 may represent a therapeutic target to ameliorate lipid-related diseases.
During the last decades, developments in genome-wide analyses have confirmed that almost all human genomes are transcribed with lncRNAs. Many lncRNAs have been known to be functional in mammals and are involved in various physiological and pathophysiological processes by epigenetics and transcriptional or post-transcriptional regulatory mechanisms (87, 88). Recently, many studies have demonstrated that lncRNAs are involved in the pathophysiology of various pathological conditions, including cancers (89), autoimmune diseases (90), and neurological disorders (91) and cardiovascular diseases (92).
Previous studies have demonstrated novel lncRNA biomarkers and identify therapeutic lncRNA targets (93, 94). A novel lnc030 was highly expressed in breast cancer (95). Inhibition of lnc030 expression by lentivirus-mediated short hairpins RNAs (shRNAs) reported markedly impaired colony formation. It also inhibited breast cancer initiation and progression, whereas ectopic lnc030 overexpression significantly increased colony formation and promoted initiation and progression of breast cancer (95). These results demonstrated that lnc030 could act as a therapeutic target and biomarker in breast cancer. Similarly, lncRNA PVT1 was verified to function as a tumor promoter in gastric cancer. It is reported that PVT1 was highly expressed in gastric cancer (GC) tissues, and high PVT1 level was correlated with tumor stage, lymph node metastasis, and poor prognosis (96). Overexpression of PVT1 greatly promoted the GC cell epithelial-to-mesenchymal transition (EMT) process and tumor metastasis in vitro and in vivo (96). These findings indicated that PVT1 has an important implication for future therapy of the GC. A similar vector has already been demonstrated as effective in animal studies for thyroid cancer therapy (97). Other circulating lncRNAs also have been verified as biomarkers in the diagnosis and prognosis of many diseases. For example, prostate-specific lncRNA prostate cancer antigen 3 (PCA3) levels have been suggested as a diagnostic biomarker of prostate cancer (98). Other lncRNAs used as biomarkers include circulating plasma H19 for gastric cancer (99), HULC in hepatocellular carcinoma (100), circulating lncRNA in SNHG11 colorectal cancer (101), circulating exosomal lncRNA-GC1 in gastric cancer (102), and HOTAIR in various cancers, including breast, colorectal, liver, gastric, lung, and thyroid (103–107).
Other than cancers, lncRNAs also have been investigated as promising biomarkers for atherosclerotic disease (108). CoroMarker was highly expressed in circulating peripheral blood monocytes (PBMCs) and plasma from patients with coronary artery disease (CAD) (109). CoroMarker acts as a candidate biomarker for CAD with an AUC of 0.920 and a 95% confidence interval of 0.892–0.947, and it could successfully distinguish CAD out of patients (109). CoroMarker is stable, sensitive, and mainly in the extracellular vesicle, probably from monocytes (110). LIPCAR has been identified from the plasma RNA from patients with myocardial infarction (111). LIPCAR is consistently detectable in the plasma and significantly increases in patients with myocardial infarction during later stages and ischemic and non-ischemic heart failure. Importantly, higher LIPCAR levels identified patients developing cardiac remodeling and were also reported to be an independent biomarker of future cardiovascular deaths (111). Another study compared the expression of lncRNAs in the peripheral blood cells between healthy and myocardial infarction patients. It demonstrated that in the cardiac hypertrophy-associated transcript (CHAST), MALAT1 was significantly upregulated in myocardial infarction patients (112–114). These findings provided a promising therapeutic strategy for treating atherosclerotic diseases and shed light on the clinical implication of lncRNA-associated ceRNA mechanisms in atherosclerotic disease deterioration.
lncRNAs may serve as biomarkers in human disease for diagnosis, prognosis, and therapy. This possibility is supported by preclinical studies and human studies that represent some of their characteristics. The characteristics of lncRNAs, including high tissue-specificity, disease specificity, cell-type specificity, and relative ease in detection methods, make them suitable for patients with diseases. Meantime, these data also suggested that lncRNAs are superior to proteins in terms of potential, targeted-related toxic effects. Moreover, lack of translation, rapid turnover and low expression levels may promote faster effects with lower doses.
Oligonucleotide therapeutics such as specific antisense oligonucleotides (ASOs), small interfering RNA technology, or small molecule inhibitors can also be used in treating a variety of diseases (Table 2), including cancer, infectious diseases, atherosclerosis, liver, and kidney disease (72, 129, 130). The most advanced therapeutic lncRNAs targeting currently are based on the use of ASOs. These molecules are short single-stranded DNA that could be synthesized based on unique RNA sequence and accessibility. Importantly, ASOs represent a valuable approach to antagonize lncRNAs, leading to premature transcription termination and inhibited lncRNA expression (131, 132). ASOs silencing of the expression of disease-related genes is a novel therapeutic approach. For example, LINC02273-targeting ASO significantly inhibited breast cancer metastasis in vivo (115). However, treatments using ASOs are limited in the clinic, mainly because of in vivo toxicity and the lack of a system for delivering ASOs to cells and tissues. To improve their pharmacological properties, ASOs are modified to improve potency, efficacy, stability (133), thereby increasing resistance to nucleases degradation and decreasing non-specific immune-stimulating activity. Several mRNA-therapeutic ASOs have already been approved by the Food and Drug Administration (FDA), and the European Medicines Agency and several others are in late-stage clinical development (134, 135). Some ASOs targeting lncRNA-dependent lipid-related disease are under development and protected by patents.
Small molecule inhibitors have been used for lncRNAs targeted therapies (136, 137). Obtaining small molecule inhibitors that bind lncRNAs with high affinity and specificity requires the identification of lncRNA motifs. This level of structural knowledge is available only for limited lncRNAs, suggesting that lncRNAs can form multiple functional domains involved in various molecular interactions to fight the disease (138, 139). Small molecule inhibitors can hamper interactions between lncRNAs and their associated protein partners, which could be desirable from a therapeutic perspective for disease. Recent studies have reported that lncRNA-protein interactions such as H19-EZH2 (140), HOTAIR-polycomb repressive complex 2 (PRC2) (141), and ANRIL-chromobox 7 (CBX7) (142) have become potential targets. Small molecule inhibitor, AC1Q3QWB, hampered the interaction between HOTAIR-EZH2 interaction in various cancer cell lines and orthotopic breast cancer models (123). In addition, synthetic functional molecules that mimic the lncRNAs' structure and binding properties may compete with lncRNA for protein binding, and thereby interfering with its function. These approaches will become more practical as the structural and binding properties of lncRNAs become better understood.
Clustered regularly interspaced short palindromic repeats (CRISPR) and CRISPR-associated proteins (Cas) (CRISPR/Cas) systems are the most versatile and promising within the past decade for modulation of lncRNAs (143). The CRISPR/Cas systems include CRISPR/Cas9, CRISPR interference (CRISPRi) (144) or CRISPR activator system (CRISPRa) (145), which were used for editing lncRNA-encoding genes. These tools ensure relatively rapid knockout, knockdown or overexpression of lncRNAs in vivo and in vitro. It has recently been widely used for research applications of a single lncRNA locus. It is increasingly applied to thousands of loci for high throughput functional screens in various experimental settings (146). However, because of their lack of protein-coding capacity, targeting lncRNAs in vivo using CRISPR/Cas systems is more difficult than targeting mRNA-coding genes. Therefore, we speculate that the therapeutic application of CRISPR/Cas systems at lncRNA loci will lag behind that of mRNA-coding genes.
Therapies based on the ASO and CRISPR/Cas systems have shown prominently good and long-lasting results, and are moving toward a portfolio of preventive treatments at the lipid levels. However, it should be noted that maintaining cholesterol homeostasis is a complicated process, and novel promising therapies for interventions affecting lipid metabolism are being discovered. For example, LASER has been implicated to participate in cholesterol homeostasis in hepatocytes and PBMCs (76). Likely, there are other yet unidentified lncRNAs and other RNA species that are also involved in this regulation.
The importance of cholesterol homeostasis function is underscored by the diverse regulatory pathways that maintain cellular cholesterol levels within a narrow range. Cellular cholesterol deficiency and accumulation, hallmarks of some lipid-related diseases involving the liver and angiogenesis, highlight the importance of maintaining cholesterol homeostasis in various cell lines. In humans, cholesterol homeostasis is maintained by multiple feedback and compensatory mechanisms. LncRNAs have been involved in regulating cholesterol homeostasis, and several studies focused on lncRNAs regulated by cholesterol. These lncRNAs function in normal metabolism and cholesterol homeostasis as well as in the progression of lipid-related diseases. In this review, the discovery of lncRNAs has provided novel and sensitive biomarkers and therapeutic targets for patients with lipid-related diseases. Dysregulation in lncRNA expression could also be a cause of lipid-related diseases. Further, a lack of specific, secure, and effective delivery systems limit lncRNAs' use in treating lipid-related diseases. Future studies should focus on tissue-specific interference or overexpression of lncRNAs to achieve the targeted therapy of patients with lipid-related diseases.
Most lncRNAs frequently display tissue and disease-specific expression patterns and less conserved than protein-coding genes (147). Due to unique features, lncRNAs may be superior therapeutic targets than existing protein-coding genes for various disease diagnoses and prognoses. Additionally, lncRNAs act as biologically functional molecules, and their expression may be better biomarker candidates for various disease states (148). However, the function of most lncRNAs is still unknown, their role in physiology, development, and disease. Their effective use as therapeutic targets requires an enormous number of studies. Investigations are required to check the pharmacokinetics and toxicity of lncRNAs. It is also critical to further explore these regulatory RNAs' novel biologic characteristics and provide potential novel treatment options. However, lncRNA holds great therapeutic promise for potential intervention. Because of its tissue and disease-specific expression, lncRNA has great therapeutic interventions as a biomarker and an important therapeutic target for treating diseases. Considerable research is currently investigating the biological function of these lncRNAs for diagnostic, prognostic, and therapeutic. In this field, the research is increasing exponentially over the next decade will teach us more about the diagnostic, prognostic, and therapeutic of these lncRNAs.
G-JZ, CH, and W-CY designed and outlined the article. W-CY, L-JH, G-JZ, and CH conducted the literature article. W-CY, S-FH, and H-JL analyzed the literature and provided suggestions. W-CY, S-FH, and L-JH provided ideas and wrote the article. All the authors have approved the manuscript for submission.
The authors declare that the research was conducted in the absence of any commercial or financial relationships that could be construed as a potential conflict of interest.
The authors gratefully acknowledge the financial support from the National Natural Sciences Foundation of China (81870337 and 82000407), the Guangdong Natural Sciences Foundation (2019A1515110080), and the Medical Scientific Research Foundation of Guangdong Province (B2021153).
1. Goldstein JL, Brown MS. Regulation of the mevalonate pathway. Nature. (1990) 343:425–30. doi: 10.1038/343425a0
2. Cruz PM, Mo H, McConathy WJ, Sabnis N, Lacko AG. The role of cholesterol metabolism and cholesterol transport in carcinogenesis: a review of scientific findings, relevant to future cancer therapeutics. Front Pharmacol. (2013) 4:119. doi: 10.3389/fphar.2013.00119
3. Luo J, Yang H, Song BL. Mechanisms and regulation of cholesterol homeostasis. Nat Rev Mol Cell Biol. (2020) 21:225–45. doi: 10.1038/s41580-019-0190-7
4. Benito-Vicente A, Uribe KB, Jebari S, Galicia-Garcia U, Ostolaza H, Martin C. Familial hypercholesterolemia: the most frequent cholesterol metabolism disorder caused disease. Int J Mol Sci. (2018) 19:3426. doi: 10.3390/ijms19113426
5. Defesche J, Gidding S, Harada-Shiba M, Hegele R, Santos R, Wierzbicki A. Familial hypercholesterolaemia. Nat Rev Dis Primers. (2017) 3:17093. doi: 10.1038/nrdp.2017.93
6. Singh AK, Aryal B, Zhang X, Fan Y, Price NL, Suarez Y, et al. Posttranscriptional regulation of lipid metabolism by non-coding RNAs and RNA binding proteins. Semin Cell Dev Biol. (2018) 81:129–40. doi: 10.1016/j.semcdb.2017.11.026
7. Liu Y, Zheng L, Wang Q, Hu YW. Emerging roles and mechanisms of long noncoding RNAs in atherosclerosis. Int J Cardiol. (2017) 228:570–82. doi: 10.1016/j.ijcard.2016.11.182
8. Ou M, Li X, Zhao S, Cui S, Tu J. Long non-coding RNA CDKN2B-AS1 contributes to atherosclerotic plaque formation by forming RNA-DNA triplex in the CDKN2B promoter. EBioMedicine. (2020) 55:102694. doi: 10.1016/j.ebiom.2020.102694
9. Han X, Huang S, Xue P, Fu J, Liu L, Zhang C, et al. PTPRE-AS1LncRNA modulates M2 macrophage activation and inflammatory diseases by epigenetic promotion of PTPRE. Sci Adv. (2019) 5:eaax9230. doi: 10.1126/sciadv.aax9230
10. Zhao B, Roundtree I, He C. Post-transcriptional gene regulation by mRNA modifications. Nat Rev Mol Cell Biol. (2017) 18:31–42. doi: 10.1038/nrm.2016.132
11. Dai D, Wang H, Zhu L, Jin H, Wang X. N6-methyladenosine links RNA metabolism to cancer progression. Cell Death Dis. (2018) 9:124. doi: 10.1038/s41419-017-0129-x
12. Zaccara S, Ries R, Jaffrey S. Reading, writing and erasing mRNA methylation. Nat Rev Mol Cell Biol. (2019) 20:608–24. doi: 10.1038/s41580-019-0168-5
13. Zhao Z, Meng J, Su R, Zhang J, Chen J, Ma X, et al. Epitranscriptomics in liver disease: Basic concepts and therapeutic potential. J Hepatol. (2020) 73:664–79. doi: 10.1016/j.jhep.2020.04.009
14. Jian D, Wang Y, Jian L, Tang H, Rao L, Chen K, et al. METTL14 aggravates endothelial inflammation and atherosclerosis by increasing FOXO1 N6-methyladeosine modifications. Theranostics. (2020) 10:8939–56. doi: 10.7150/thno.45178
15. Fang R, Chen X, Zhang S, Shi H, Ye Y, Shi H, et al. EGFR/SRC/ERK-stabilized YTHDF2 promotes cholesterol dysregulation and invasive growth of glioblastoma. Nat Commun. (2021) 12:177. doi: 10.1038/s41467-020-20379-7
16. Park M, Jeong E, Choudhury M. Mono-(2-Ethylhexyl)phthalate regulates cholesterol efflux via MicroRNAs regulated mA RNA methylation. Chem Res Toxicol. (2020) 33:461–9. doi: 10.1021/acs.chemrestox.9b00367
17. Wu J, Li Y, Yu J, Gan Z, Wei W, Wang C, et al. Resveratrol attenuates high-fat diet induced hepatic lipid homeostasis disorder and decreases mA RNA methylation. Front Pharmacol. (2020) 11:568006. doi: 10.3389/fphar.2020.568006
18. Qin Y, Li L, Luo E, Hou J, Yan G, Wang D, et al. Role of m6A RNA methylation in cardiovascular disease (Review). Int J Mol Med. (2020) 46:1958–72. doi: 10.3892/ijmm.2020.4746
19. Poller W, Dimmeler S, Heymans S, Zeller T, Haas J, Karakas M, et al. Non-coding RNAs in cardiovascular diseases: diagnostic and therapeutic perspectives. Eur Heart J. (2018) 39:2704–16. doi: 10.1093/eurheartj/ehx165
20. Zhao X, Xiong X, Liu T, Mi L, Peng X, Rui C, et al. Long noncoding RNA licensing of obesity-linked hepatic lipogenesis and NAFLD pathogenesis. Nat Commun. (2018) 9:2986. doi: 10.1038/s41467-018-05383-2
21. Li P, Ruan X, Yang L, Kiesewetter K, Zhao Y, Luo H, et al. A liver-enriched long non-coding RNA, lncLSTR, regulates systemic lipid metabolism in mice. Cell Metab. (2015) 21:455–67. doi: 10.1016/j.cmet.2015.02.004
22. Dong X, Lu Z, Kang C, Li X, Haworth K, Ma X, et al. The long noncoding RNA RP11-728F11.4 promotes atherosclerosis. Arterioscl Thromb Vasc Biol. (2021) 41:1191–204. doi: 10.1161/ATVBAHA.120.315114
23. Simion V, Zhou H, Haemmig S, Pierce J, Mendes S, Tesmenitsky Y, et al. A macrophage-specific lncRNA regulates apoptosis and atherosclerosis by tethering HuR in the nucleus. Nat Commun. (2020) 11:6135. doi: 10.1038/s41467-020-19664-2
24. Yang D, Qiao J, Wang G, Lan Y, Li G, Guo X, et al. N6-Methyladenosine modification of lincRNA 1281 is critically required for mESC differentiation potential. Nucl Acids Res. (2018) 46:3906–20. doi: 10.1093/nar/gky130
25. Lan T, Li H, Zhang D, Xu L, Liu H, Hao X, et al. KIAA1429 contributes to liver cancer progression through N6-methyladenosine-dependent post-transcriptional modification of GATA3. Mol Cancer. (2019) 18:186. doi: 10.1186/s12943-019-1106-z
26. Jin D, Guo J, Wu Y, Du J, Yang L, Wang X, et al. mA mRNA methylation initiated by METTL3 directly promotes YAP translation and increases YAP activity by regulating the MALAT1-miR-1914-3p-YAP axis to induce NSCLC drug resistance and metastasis. J Hematol Oncol. (2019) 12:135. doi: 10.1186/s13045-019-0830-6
27. Patil D, Chen C, Pickering B, Chow A, Jackson C, Guttman M, et al. m(6)A RNA methylation promotes XIST-mediated transcriptional repression. Nature. (2016) 537:369–73. doi: 10.1038/nature19342
28. Li H, Zhao Q, Chang L, Wei C, Bei H, Yin Y, et al. LncRNA MALAT1 modulates ox-LDL induced EndMT through the Wnt/β-catenin signaling pathway. Lipids Health Dis. (2019) 18:62. doi: 10.1186/s12944-019-1006-7
29. Durbin A, Gotlieb A. Advances towards understanding heart valve response to injury. Cardiovasc Pathol. (2002) 11:69–77. doi: 10.1016/S1054-8807(01)00109-0
30. Zhang X, Wu H, Mai C, Qi Y. Long noncoding RNA XIST/miR-17/PTEN axis modulates the proliferation and apoptosis of vascular smooth muscle cells to affect stanford Type A aortic dissection. J Cardiovasc Pharmacol. (2020) 76:53–62. doi: 10.1097/FJC.0000000000000835
31. Yan C, Chen J, Chen N. Long noncoding RNA MALAT1 promotes hepatic steatosis and insulin resistance by increasing nuclear SREBP-1c protein stability. Sci Rep. (2016) 6:22640. doi: 10.1038/srep22640
32. Cremer S, Michalik K, Fischer A, Pfisterer L, Jaé N, Winter C, et al. Hematopoietic deficiency of the long noncoding RNA MALAT1 promotes atherosclerosis and plaque inflammation. Circulation. (2019) 139:1320–34. doi: 10.1161/CIRCULATIONAHA.117.029015
33. Gao H, Guo Z. LncRNA XIST regulates atherosclerosis progression in ox-LDL-induced HUVECs. Open Med. (2021) 16:117–27. doi: 10.1515/med-2021-0200
34. Li H, Han S, Sun Q, Yao Y, Li S, Yuan C, et al. Long non-coding RNA CDKN2B-AS1 reduces inflammatory response and promotes cholesterol efflux in atherosclerosis by inhibiting ADAM10 expression. Aging. (2019) 11:1695–715. doi: 10.18632/aging.101863
35. Hennessy E, van Solingen C, Scacalossi K, Ouimet M, Afonso M, Prins J, et al. The long noncoding RNA CHROME regulates cholesterol homeostasis in primate. Nat Metab. (2019) 1:98–110. doi: 10.1016/j.atherosclerosis.2019.06.890
36. Cai C, Zhu H, Ning X, Li L, Yang B, Chen S, et al. LncRNA ENST00000602558.1 regulates ABCG1 expression and cholesterol efflux from vascular smooth muscle cells through a p65-dependent pathway. Atherosclerosis. (2019) 285:31–9. doi: 10.1016/j.atherosclerosis.2019.04.204
37. Shen S, Zheng X, Zhu Z, Zhao S, Zhou Q, Song Z, et al. Silencing of GAS5 represses the malignant progression of atherosclerosis through upregulation of miR-135a. Biomed Pharmacother. (2019) 118:109302. doi: 10.1016/j.biopha.2019.109302
38. Huang P, Huang F, Liu H, Zhang T, Yang M, Sun C. LncRNA MEG3 functions as a ceRNA in regulating hepatic lipogenesis by competitively binding to miR-21 with LRP6. Metab Clin Experi. (2019) 94:1–8. doi: 10.1016/j.metabol.2019.01.018
39. Wang H, Cao Y, Shu L, Zhu Y, Peng Q, Ran L, et al. Long non-coding RNA (lncRNA) H19 induces hepatic steatosis through activating MLXIPL and mTORC1 networks in hepatocytes. J Cell Mol Med. (2020) 24:1399–412. doi: 10.1111/jcmm.14818
40. Liu L, Tan L, Yao J, Yang L. Long non-coding RNA MALAT1 regulates cholesterol accumulation in ox-LDL-induced macrophages via the microRNA-17-5p/ABCA1 axis. Mol Med Rep. (2020) 21:1761–70. doi: 10.3892/mmr.2020.10987
41. Huang C, Hu Y, Zhao J, Ma X, Zhang Y, Guo F, et al. Long noncoding RNA HOXC-AS1 suppresses Ox-LDL-induced cholesterol accumulation through promoting HOXC6 expression in THP-1 macrophages. DNA Cell Biol. (2016) 35:722–9. doi: 10.1089/dna.2016.3422
42. Pang J, Wang J, Hu P, Jiang J, Yu C. HOTAIR alleviates ox-LDL-induced inflammatory response in Raw264.7 cells via inhibiting NF-κB pathway. Eur Rev Med Pharmacol Sci. (2018) 22:6991–8. doi: 10.26355/eurrev_201810_16170
43. Chen X, Tan X, Li S, Zhang X. LncRNA NEAT1 promotes hepatic lipid accumulation via regulating miR-146a-5p/ROCK1 in nonalcoholic fatty liver disease. Life Sci. (2019) 235:116829. doi: 10.1016/j.lfs.2019.116829
44. Lan X, Wu L, Wu N, Chen Q, Li Y, Du X, et al. Long noncoding RNA lnc-HC regulates PPARγ-mediated hepatic lipid metabolism through miR-130b-3p. Mol Therapy Nucleic Acids. (2019) 18:954–65. doi: 10.1016/j.omtn.2019.10.018
45. Li P, Yan X, Xu G, Pang Z, Weng J, Yin J, et al. A novel plasma lncRNA ENST00000416361 is upregulated in coronary artery disease and is related to inflammation and lipid metabolism. Mol Med Rep. (2020) 21:2375–84. doi: 10.3892/mmr.2020.11067
46. Sallam T, Jones M, Gilliland T, Zhang L, Wu X, Eskin A, et al. Feedback modulation of cholesterol metabolism by the lipid-responsive non-coding RNA LeXis. Nature. (2016) 534:124–8. doi: 10.1038/nature17674
47. Zhang X, Wu J, Wu C, Chen W, Lin R, Zhou Y, et al. The LINC01138 interacts with PRMT5 to promote SREBP1-mediated lipid desaturation and cell growth in clear cell renal cell carcinoma. Biochem Biophys Res Commun. (2018) 507:337–42. doi: 10.1016/j.bbrc.2018.11.036
48. Mohamadi M, Ghaedi H, Kazerouni F, Erfanian Omidvar M, Kalbasi S, Shanaki M, et al. Deregulation of long noncoding RNA SNHG17 and TTC28-AS1 is associated with type 2 diabetes mellitus. Scand J Clin Lab Investig. (2019) 79:519–23. doi: 10.1080/00365513.2019.1664760
49. Wu L, Pei Y, Zhu Y, Jiang M, Wang C, Cui W, et al. Association of N-methyladenine DNA with plaque progression in atherosclerosis via myocardial infarction-associated transcripts. Cell Death Dis. (2019) 10:909. doi: 10.1038/s41419-019-2152-6
50. Hu Y, Zhao J, Li S, Huang J, Qiu Y, Ma X, et al. RP5-833A20.1/miR-382-5p/NFIA-dependent signal transduction pathway contributes to the regulation of cholesterol homeostasis and inflammatory reaction. Arterioscl Thromb Vasc Biol. (2015) 35:87–101. doi: 10.1161/ATVBAHA.114.304296
51. Zuo X, Chen Z, Gao W, Zhang Y, Wang J, Wang J, et al. M6A-mediated upregulation of LINC00958 increases lipogenesis and acts as a nanotherapeutic target in hepatocellular carcinoma. J Hematol Oncol. (2020) 13:5. doi: 10.1186/s13045-019-0839-x
52. Zeng Y, Ren K, Zhu X, Zheng Z, Yi G. Long noncoding RNAs: advances in lipid metabolism. Adv Clin Chem. (2018) 87:1–36. doi: 10.1016/bs.acc.2018.07.001
53. Chen Z. Progress and prospects of long noncoding RNAs in lipid homeostasis. Mol Metab. (2016) 5:164–70. doi: 10.1016/j.molmet.2015.12.003
54. Ye Z, Yang S, Xia Y, Hu R, Chen S, Li B, et al. LncRNA MIAT sponges miR-149-5p to inhibit efferocytosis in advanced atherosclerosis through CD47 upregulation. Cell Death Dis. (2019) 10:138. doi: 10.1038/s41419-019-1409-4
55. Sun G, Li Y, Ji Z. Up-regulation of MIAT aggravates the atherosclerotic damage in atherosclerosis mice through the activation of PI3K/Akt signaling pathway. Drug Deliv. (2019) 26:641–9. doi: 10.1080/10717544.2019.1628116
56. Li H, Zhang Y, Guo Y, Liu R, Yu Q, Gong L, et al. ALKBH1 promotes lung cancer by regulating m6A RNA demethylation. Biochem Pharmacol. (2020) 14:114284. doi: 10.1016/j.bcp.2020.114284
57. Pan JX. LncRNA H19 promotes atherosclerosis by regulating MAPK and NF-kB signaling pathway. Eur Rev Med Pharmacol Sci. (2017) 21:322–8.
58. Huang Y, Wang L, Mao Y, Nan G. Long noncoding RNA-H19 contributes to atherosclerosis and induces ischemic stroke via the upregulation of acid phosphatase 5. Front Neurol. (2019) 10:32. doi: 10.3389/fneur.2019.00032
59. Yang Y, Tang F, Wei F, Yang L, Kuang C, Zhang H, et al. Silencing of long non-coding RNA H19 downregulates CTCF to protect against atherosclerosis by upregulating PKD1 expression in ApoE knockout mice. Aging. (2019) 11:10016–30. doi: 10.18632/aging.102388
60. Liu J, Tang T, Wang G, Liu B. LncRNA-H19 promotes hepatic lipogenesis by directly regulating miR-130a/PPARγ axis in non-alcoholic fatty liver disease. Biosci Rep. (2019) 39:BSR20181722. doi: 10.1042/BSR20181722
61. Wang H, Yang Y, Sun X, Tian F, Guo S, Wang W, et al. Sonodynamic therapy-induced foam cells apoptosis activates the phagocytic PPARγ-LXRα-ABCA1/ABCG1 pathway and promotes cholesterol efflux in advanced plaque. Theranostics. (2018) 8:4969–84. doi: 10.7150/thno.26193
62. Chen L, Yao H, Hui J, Ding S, Fan Y, Pan Y, et al. Global transcriptomic study of atherosclerosis development in rats. Gene. (2016) 592:43–8. doi: 10.1016/j.gene.2016.07.023
63. Meng X, Yao H, Wang L, Yu M, Shi S, Yuan Z, et al. Knockdown of GAS5 inhibits atherosclerosis progression via reducing EZH2-mediated ABCA1 transcription in ApoE mice. Mol Ther Nucleic Acids. (2020) 19:84–96. doi: 10.1016/j.omtn.2019.10.034
64. Liu Y, Jia L, Min D, Xu Y, Zhu J, Sun Z. Baicalin inhibits proliferation and promotes apoptosis of vascular smooth muscle cells by regulating the MEG3/p53 pathway following treatment with ox-LDL. Int J Mol Med. (2019) 43:901–13. doi: 10.3892/ijmm.2018.4009
65. Yang X, Wang C, Lee W, Trovik J, Chung T, Kwong J. Long non-coding RNA HAND2-AS1 inhibits invasion and metastasis in endometrioid endometrial carcinoma through inactivating neuromedin U. Cancer Lett. (2018) 413:23–34. doi: 10.1016/j.canlet.2017.10.028
66. Zhu X, Li H, Wu Y, Zhou J, Yang G, Wang W. lncRNA MEG3 promotes hepatic insulin resistance by serving as a competing endogenous RNA of miR-214 to regulate ATF4 expression. Int J Mol Med. (2019) 43:345–57. doi: 10.3892/ijmm.2018.3975
67. Ha E, Van Camp A, Bauer R. Genetics-driven discovery of novel regulators of lipid metabolism. Curr Opin Lipidol. (2019) 30:157–64. doi: 10.1097/MOL.0000000000000605
68. Zhao Y, Wu J, Liangpunsakul S, Wang L. Long non-coding RNA in liver metabolism and disease: current status. Liver Res. (2017) 1:163–7. doi: 10.1016/j.livres.2017.09.001
69. Ceroi A, Masson D, Roggy A, Roumier C, Chagué C, Gauthier T, et al. LXR agonist treatment of blastic plasmacytoid dendritic cell neoplasm restores cholesterol efflux and triggers apoptosis. Blood. (2016) 128:2694–707. doi: 10.1182/blood-2016-06-724807
70. Nakaya K, Ayaori M, Hisada T, Sawada S, Tanaka N, Iwamoto N, et al. Telmisartan enhances cholesterol efflux from THP-1 macrophages by activating PPARgamma. J Atheroscl Thromb. (2007) 14:133–41. doi: 10.5551/jat.14.133
71. Bayoglu B, Yuksel H, Cakmak H, Dirican A, Cengiz M. Polymorphisms in the long non-coding RNA CDKN2B-AS1 may contribute to higher systolic blood pressure levels in hypertensive patients. Clin Biochem. (2016) 49:821–7. doi: 10.1016/j.clinbiochem.2016.02.012
72. Haag SM, Gulen MF, Reymond L, Gibelin A, Abrami L, Decout A, et al. Targeting STING with covalent small-molecule inhibitors. Nature. (2018) 559:269–23. doi: 10.1038/s41586-018-0287-8
73. Huang Y, Xiang B, Liu Y, Wang Y, Kan H. LncRNA CDKN2B-AS1 promotes tumor growth and metastasis of human hepatocellular carcinoma by targeting let-7c-5p/NAP1L1 axis. Cancer Lett. (2018) 437:56–66. doi: 10.1016/j.canlet.2018.08.024
74. Restrepo N, Laper S, Farber-Eger E, Crawford D. Local genetic ancestry in CDKN2B-AS1 is associated with primary open-angle glaucoma in an African American cohort extracted from de-identified electronic health records. BMC Med Genom. (2018) 11:70. doi: 10.1186/s12920-018-0392-4
75. Hubberten M, Bochenek G, Chen H, Häsler R, Wiehe R, Rosenstiel P, et al. Linear isoforms of the long noncoding RNA CDKN2B-AS1 regulate the c-myc-enhancer binding factor RBMS1. Eur J Human Genet: EJHG. (2019) 27:80–9. doi: 10.1038/s41431-018-0210-7
76. Li C, Hu Z, Zhang W, Yu J, Yang Y, Xu Z, et al. Regulation of cholesterol homeostasis by a novel long non-coding RNA LASER. Sci Rep. (2019) 9:7693. doi: 10.1038/s41598-019-44195-2
77. Seidah N, Awan Z, Chrétien M, Mbikay M. PCSK9: a key modulator of cardiovascular health. Circul Res. (2014) 114:1022–36. doi: 10.1161/CIRCRESAHA.114.301621
78. Gao Y, Wang Y, Chen X, Peng Y, Chen F, He Y, et al. MiR-127 attenuates adipogenesis by targeting MAPK4 and HOXC6 in porcine adipocytes. J Cell Physiol. (2019) 234:21838–50. doi: 10.1002/jcp.28660
79. Huang J, Chen S, Cai D, Bian D, Wang F. Long noncoding RNA lncARSR promotes hepatic cholesterol biosynthesis via modulating Akt/SREBP-2/HMGCR pathway. Life Sci. (2018) 203:48–53. doi: 10.1016/j.lfs.2018.04.028
80. Cirera-Salinas D, Pauta M, Allen R, Salerno A, Ramírez C, Chamorro-Jorganes A, et al. Mir-33 regulates cell proliferation and cell cycle progression. Cell Cycle. (2012) 11:922–33. doi: 10.4161/cc.11.5.19421
81. Zhao W, Rasheed A, Tikkanen E, Lee J, Butterworth A, Howson J, et al. Identification of new susceptibility loci for type 2 diabetes and shared etiological pathways with coronary heart disease. Nat Genet. (2017) 49:1450–7. doi: 10.1038/ng.3943
82. Klarin D, Zhu Q, Emdin C, Chaffin M, Horner S, McMillan B, et al. Genetic analysis in UK Biobank links insulin resistance and transendothelial migration pathways to coronary artery disease. Nat Genet. (2017) 49:1392–7. doi: 10.1038/ng.3914
83. Li L, Wang L, Li H, Han X, Chen S, Yang B, et al. Characterization of LncRNA expression profile and identification of novel LncRNA biomarkers to diagnose coronary artery disease. Atherosclerosis. (2018) 275:359–67. doi: 10.1016/j.atherosclerosis.2018.06.866
84. Ma X, Wang T, Zhao Z, Jiang Y, Ye S. Propofol suppresses proinflammatory cytokine production by increasing ABCA1 expression via mediation by the long noncoding RNA LOC286367. Media Inflamm. (2018) 2018:8907143. doi: 10.1155/2018/8907143
85. Joyce C, Amar M, Lambert G, Vaisman B, Paigen B, Najib-Fruchart J, et al. The ATP binding cassette transporter A1 (ABCA1) modulates the development of aortic atherosclerosis in C57BL/6 and apoE-knockout mice. Proc Natl Acad Sci USA. (2002) 99:407–12. doi: 10.1073/pnas.012587699
86. Gao J, He L, Yu X, Zhao Z, Wang G, Zou J, et al. CXCL12 promotes atherosclerosis by downregulating ABCA1 expression via the CXCR4/GSK3β/β-catenin/TCF21 pathway. J Lipid Res. (2019) 60:2020–33. doi: 10.1194/jlr.RA119000100
87. Correia de Sousa M, Gjorgjieva M, Dolicka D, Sobolewski C, Foti M. Deciphering miRNAs' action through miRNA editing. Int J Mol Sci. (2019) 20:24. doi: 10.3390/ijms20246249
88. Zhang X, Hamblin M, Yin K. The long noncoding RNA Malat1: Its physiological and pathophysiological functions. RNA Biol. (2017) 14:1705–14. doi: 10.1080/15476286.2017.1358347
89. Bhan A, Soleimani M, Mandal S. Long noncoding RNA and cancer: a new paradigm. Cancer Res. (2017) 77:3965–81. doi: 10.1158/0008-5472.CAN-16-2634
90. Lodde V, Murgia G, Simula E, Steri M, Floris M, Idda M. Long noncoding RNAs and circular RNAs in autoimmune diseases. Biomolecules. (2020) 10:1044. doi: 10.3390/biom10071044
91. Wu S, Bono J, Tao Y. Long noncoding RNA (lncRNA): a target in neuropathic pain. Exp Opin Therap Targets. (2019) 23:15–20. doi: 10.1080/14728222.2019.1550075
92. Uchida S, Dimmeler S. Long noncoding RNAs in cardiovascular diseases. Circul Res. (2015) 116:737–50. doi: 10.1161/CIRCRESAHA.116.302521
93. Zhang X, Sun S, Pu J, Tsang A, Lee D, Man V, et al. Long non-coding RNA expression profiles predict clinical phenotypes in glioma. Neurobiol Dis. (2012) 48:1–8. doi: 10.1016/j.nbd.2012.06.004
94. Yu H, Xu Q, Liu F, Ye X, Wang J, Meng X. Identification and validation of long noncoding RNA biomarkers in human non-small-cell lung carcinomas. J Thorac Oncol. (2015) 10:645–54. doi: 10.1097/JTO.0000000000000470
95. Qin Y, Hou Y, Liu S, Zhu P, Wan X, Zhao M, et al. A novel long non-coding RNA lnc030 maintains breast cancer stem cell stemness by stabilizing SQLE mRNA and increasing cholesterol synthesis. Adv Sci. (2021) 8:2002232. doi: 10.1002/advs.202002232
96. Wang L, Xiao B, Yu T, Gong L, Wang Y, Zhang X, et al. lncRNA PVT1 promotes the migration of gastric cancer by functioning as ceRNA of miR-30a and regulating Snail. J Cell Physiol. (2021) 236:536–48. doi: 10.1002/jcp.29881
97. Yuan J, Song Y, Pan W, Li Y, Xu Y, Xie M, et al. LncRNA SLC26A4-AS1 suppresses the MRN complex-mediated DNA repair signaling and thyroid cancer metastasis by destabilizing DDX5. Oncogene. (2020) 39:6664–76. doi: 10.1038/s41388-020-01460-3
98. Lemos A, Matos A, Ferreira L, Gimba E. PCA3The long non-coding RNA: an update of its functions and clinical applications as a biomarker in prostate cancer. Oncotarget. (2019) 10:6589–603. doi: 10.18632/oncotarget.27284
99. Yörüker E, Keskin M, Kulle C, Holdenrieder S, Gezer U. Diagnostic and prognostic value of circulating lncRNA H19 in gastric cancer. Biomed Rep. (2018) 9:181–6. doi: 10.3892/br.2018.1116
100. Liu Y, Feng J, Sun M, Yang G, Yuan H, Wang Y, et al. Long non-coding RNA HULC activates HBV by modulating HBx/STAT3/miR-539/APOBEC3B signaling in HBV-related hepatocellular carcinoma. Cancer Lett. (2019) 454:158–70. doi: 10.1016/j.canlet.2019.04.008
101. Xu W, Zhou G, Wang H, Liu Y, Chen B, Chen W, et al. Circulating lncRNA SNHG11 as a novel biomarker for early diagnosis and prognosis of colorectal cancer. Int J Cancer. (2020) 146:2901–12. doi: 10.1002/ijc.32747
102. Guo X, Lv X, Ru Y, Zhou F, Wang N, Xi H, et al. Circulating exosomal gastric cancer-associated long noncoding RNA1 as a biomarker for early detection and monitoring progression of gastric cancer: a multiphase study. JAMA Surg. (2020) 155:572–9. doi: 10.1001/jamasurg.2020.1133
103. Cantile M, Di Bonito M, Cerrone M, Collina F, De Laurentiis M, Botti G. HOTAIRLong non-coding RNA in breast cancer therapy. Cancers. (2020) 12:508–17. doi: 10.3390/cancers12051197
104. Kim J, Jun H, Kim E, Lee J, Park H, Ryu C, et al. HOTAIRGenetic variants of associated with colorectal cancer susceptibility and mortality. Front Oncol. (2020) 10:72. doi: 10.3389/fonc.2020.00072
105. Zheng F, Li J, Ma C, Tang X, Tang Q, Wu J, et al. Novel regulation of miR-34a-5p and HOTAIR by the combination of berberine and gefitinib leading to inhibition of EMT in human lung cancer. J Cell Mol Med. (2020) 24:5578–92. doi: 10.1111/jcmm.15214
106. Zhang J, Qiu W, Zhu H, Liu H, Sun J, Chen Y, et al. HOTAIR contributes to the carcinogenesis of gastric cancer via modulating cellular and exosomal miRNAs level. Cell Death Dis. (2020) 11:780. doi: 10.1038/s41419-020-02946-4
107. Liu X, Liu G, Lu Y, Shi Y. Long non-coding RNA HOTAIR promotes cell viability, migration and invasion in thyroid cancer cells by sponging miR-17-5p. Neoplasma. (2020) 67:229–37. doi: 10.4149/neo_2019_190310N208
108. Lu Q, Guo P, Liu A, Ares I, Martínez-Larrañaga M, Wang X, et al. The role of long noncoding RNA in lipid, cholesterol, and glucose metabolism and treatment of obesity syndrome. Med Res Rev. (2020) 41:1751–74. doi: 10.1002/med.21775
109. Cai Y, Yang Y, Chen X, Wu G, Zhang X, Liu Y, et al. Circulating 'lncRNA OTTHUMT00000387022' from monocytes as a novel biomarker for coronary artery disease. Cardiovas Res. (2016) 112:714–24. doi: 10.1093/cvr/cvw022
110. Yang Y, Cai Y, Wu G, Chen X, Liu Y, Wang X, et al. Plasma long non-coding RNA, CoroMarker, a novel biomarker for diagnosis of coronary artery disease. Clin Sci. (2015) 129:675–85. doi: 10.1042/CS20150121
111. Kumarswamy R, Bauters C, Volkmann I, Maury F, Fetisch J, Holzmann A, et al. Circulating long noncoding RNA, LIPCAR, predicts survival in patients with heart failure. Circul Res. (2014) 114:1569–75. doi: 10.1161/CIRCRESAHA.114.303915
112. Wang X, Wang L, Ma Z, Liang W, Li J, Li Y, et al. Early expressed circulating long noncoding RNA CHAST is associated with cardiac contractile function in patients with acute myocardial infarction. Int J Cardiol. (2020) 302:15–20. doi: 10.1016/j.ijcard.2019.12.058
113. Vausort M, Wagner D, Devaux Y. Long noncoding RNAs in patients with acute myocardial infarction. Circul Res. (2014) 115:668–77. doi: 10.1161/CIRCRESAHA.115.303836
114. Zheng M, Liu X, Han R, Yuan W, Sun K, Zhong J, et al. Circulating exosomal long non-coding RNAs in patients with acute myocardial infarction. J Cell Mol Med. (2020) 24:9388–96. doi: 10.1111/jcmm.15589
115. Xiu B, Chi Y, Liu L, Chi W, Zhang Q, Chen J, et al. LINC02273 drives breast cancer metastasis by epigenetically increasing AGR2 transcription. Mol Cancer. (2019) 18:187. doi: 10.1186/s12943-019-1115-y
116. Li M, Ding X, Zhang Y, Li X, Zhou H, Yang L, et al. Antisense oligonucleotides targeting lncRNA AC104041.1 induces antitumor activity through Wnt2B/β-catenin pathway in head and neck squamous cell carcinomas. Cell Death Dis. (2020) 11:672. doi: 10.1038/s41419-020-02820-3
117. Arun G, Diermeier S, Akerman M, Chang K, Wilkinson J, Hearn S, et al. Differentiation of mammary tumors and reduction in metastasis upon Malat1 lncRNA loss. Genes Dev. (2016) 30:34–51. doi: 10.1101/gad.270959.115
118. Gutschner T, Hämmerle M, Eissmann M, Hsu J, Kim Y, Hung G, et al. The noncoding RNA MALAT1 is a critical regulator of the metastasis phenotype of lung cancer cells. Cancer Res. (2013) 73:1180–9. doi: 10.1158/0008-5472.CAN-12-2850
119. Gao H, Kerr A, Jiao H, Hon C, Rydén M, Dahlman I, et al. Long non-coding RNAs associated with metabolic traits in human white adipose tissue. EBioMedicine. (2018) 30:248–60. doi: 10.1016/j.ebiom.2018.03.010
120. Shi Y, Parag S, Patel R, Lui A, Murr M, Cai J, et al. Stabilization of lncRNA GAS5 by a small molecule and its implications in diabetic adipocytes. Cell Chem Bio. (2019) 26:319–30.e6. doi: 10.1016/j.chembiol.2018.11.012
121. Wu X, Wang Y, Yu T, Nie E, Hu Q, Wu W, et al. Blocking MIR155HG/miR-155 axis inhibits mesenchymal transition in glioma. Neuro-oncology. (2017) 19:1195–205. doi: 10.1093/neuonc/nox017
122. Mercatelli N, Fortini D, Palombo R, Paronetto M. Small molecule inhibition of Ewing sarcoma cell growth via targeting the long non coding RNA HULC. Cancer Lett. (2020) 469:111–23. doi: 10.1016/j.canlet.2019.10.026
123. Li Y, Ren Y, Wang Y, Tan Y, Wang Q, Cai J, et al. A compound AC1Q3QWB selectively disrupts HOTAIR-mediated recruitment of PRC2 and enhances cancer therapy of DZNep. Theranostics. (2019) 9:4608–23. doi: 10.7150/thno.35188
124. Cho S, Xu J, Sun R, Mumbach M, Carter A, Chen Y, et al. Promoter of lncRNA gene PVT1 is a tumor-suppressor DNA boundary element. Cell. (2018) 173:1398–412.e22. doi: 10.1016/j.cell.2018.03.068
125. Zhen S, Hua L, Liu Y, Sun X, Jiang M, Chen W, et al. Inhibition of long non-coding RNA UCA1 by CRISPR/Cas9 attenuated malignant phenotypes of bladder cancer. Oncotarget. (2017) 8:9634–46. doi: 10.18632/oncotarget.14176
126. Beaver L, Kuintzle R, Buchanan A, Wiley M, Glasser S, Wong C, et al. Long noncoding RNAs and sulforaphane: a target for chemoprevention and suppression of prostate cancer. J Nutrit Biochem. (2017) 42:72–83. doi: 10.1016/j.jnutbio.2017.01.001
127. Liu J, Ben Q, Lu E, He X, Yang X, Ma J, et al. Long noncoding RNA PANDAR blocks CDKN1A gene transcription by competitive interaction with p53 protein in gastric cancer. Cell Death Dis. (2018) 9:168. doi: 10.1038/s41419-017-0246-6
128. Leisegang M, Fork C, Josipovic I, Richter F, Preussner J, Hu J, et al. Long noncoding RNA MANTIS facilitates endothelial angiogenic function. Circulation. (2017) 136:65–79. doi: 10.1161/CIRCULATIONAHA.116.026991
129. Bernardo B, Ooi J, Lin R, McMullen J. miRNA therapeutics: a new class of drugs with potential therapeutic applications in the heart. Future Med Chem. (2015) 7:1771–92. doi: 10.4155/fmc.15.107
130. Gong N, Teng X, Li J, Liang X. Antisense oligonucleotide-conjugated nanostructure-targeting lncRNA MALAT1 inhibits cancer metastasis. ACS Appl Mater Interfaces. (2019) 11:37–42. doi: 10.1021/acsami.8b18288
131. Amodio N, Raimondi L, Juli G, Stamato M, Caracciolo D, Tagliaferri P, et al. MALAT1 a druggable long non-coding RNA for targeted anti-cancer approaches. J Hematol Oncol. (2018) 11:63. doi: 10.1186/s13045-018-0606-4
132. Lee J, Mendell J. Antisense-mediated transcript knockdown triggers premature transcription termination. Mol Cell. (2020) 77:1044–54.e3. doi: 10.1016/j.molcel.2019.12.011
133. Wan W, Seth P. The medicinal chemistry of therapeutic oligonucleotides. J Med Chem. (2016) 59:9645–67. doi: 10.1021/acs.jmedchem.6b00551
134. Stein C, Castanotto D. FDA-Approved oligonucleotide therapies in 2017. Mol Ther. (2017) 25:1069–75. doi: 10.1016/j.ymthe.2017.03.023
135. Dhuri K, Bechtold C, Quijano E, Pham H, Gupta A, Vikram A, et al. Antisense oligonucleotides. An emerging area in drug discovery and development. J Clin Med. (2020) 9:2004. doi: 10.3390/jcm9062004
136. Chandra Gupta S., Nandan Tripathi Y. Potential of long non-coding RNAs in cancer patients: From biomarkers to therapeutic targets. Int J Cancer. (2017) 140:1955–67. doi: 10.1002/ijc.30546
137. Yang J, Wang X. Role of long non-coding RNAs in lymphoma: a systematic review and clinical perspectives. Crit Rev Oncol Hematol. (2019) 141:13–22. doi: 10.1016/j.critrevonc.2019.05.007
138. Uroda T, Anastasakou E, Rossi A, Teulon J, Pellequer J, Annibale P, et al. Conserved pseudoknots in lncRNA MEG3 are essential for stimulation of the p53 pathway. Mol Cell. (2019) 75:982–95.e9. doi: 10.1016/j.molcel.2019.07.025
139. Somarowthu S, Legiewicz M, Chillón I, Marcia M, Liu F, Pyle A. HOTAIR forms an intricate and modular secondary structure. Mol Cell. (2015) 58:353–61. doi: 10.1016/j.molcel.2015.03.006
140. Yuan Z, Bian Y, Ma X, Tang Z, Chen N, Shen M. LncRNA H19 knockdown in human amniotic mesenchymal stem cells suppresses angiogenesis by associating with EZH2 and activating vasohibin-1. Stem Cells Dev. (2019) 28:781–90. doi: 10.1089/scd.2019.0014
141. Balas M, Hartwick E, Barrington C, Roberts J, Wu S, Bettcher R, et al. Establishing RNA-RNA interactions remodels lncRNA structure and promotes PRC2 activity. Sci Adv. (2021) 7:eabc9191. doi: 10.1126/sciadv.abc9191
142. Yap K, Li S, Muñoz-Cabello A, Raguz S, Zeng L, Mujtaba S, et al. Molecular interplay of the noncoding RNA ANRIL and methylated histone H3 lysine 27 by polycomb CBX7 in transcriptional silencing of INK4a. Mol Cell. (2010) 38:662–74. doi: 10.1016/j.molcel.2010.03.021
143. van der Oost Westra JE, Jackson R, Wiedenheft B. Unravelling the structural and mechanistic basis of CRISPR-Cas systems. Nat Rev Microbiol. (2014) 12:479–92. doi: 10.1038/nrmicro3279
144. Qi L, Larson M, Gilbert L, Doudna J, Weissman J, Arkin A, et al. Repurposing CRISPR as an RNA-guided platform for sequence-specific control of gene expression. Cell. (2013) 152:1173–83. doi: 10.1016/j.cell.2013.02.022
145. Mali P, Aach J, Stranges P, Esvelt K, Moosburner M, Kosuri S, et al. CAS9 transcriptional activators for target specificity screening and paired nickases for cooperative genome engineering. Nat Biotechnol. (2013) 31:833–8. doi: 10.1038/nbt.2675
146. Esposito R, Bosch N, Lanzós A, Polidori T, Pulido-Quetglas C, Johnson R. Hacking the cancer genome. Profiling therapeutically actionable long non-coding RNAs using CRISPR-Cas9 screening. Cancer Cell. (2019) 35:545–57. doi: 10.1016/j.ccell.2019.01.019
147. Wilusz J, Sunwoo H, Spector D. Long noncoding RNAs: functional surprises from the RNA world. Genes Dev. (2009) 23:1494–504. doi: 10.1101/gad.1800909
Keywords: cholesterol homeostasis, lncRNAs, liver disease, lipid-related diseases, cardiovascular disease
Citation: Ye W-C, Huang S-F, Hou L-J, Long H-J, Yin K, Hu CY and Zhao G-J (2021) Potential Therapeutic Targeting of lncRNAs in Cholesterol Homeostasis. Front. Cardiovasc. Med. 8:688546. doi: 10.3389/fcvm.2021.688546
Received: 20 April 2021; Accepted: 19 May 2021;
Published: 10 June 2021.
Edited by:
Shizuka Uchida, Aalborg University Copenhagen, DenmarkReviewed by:
Jun-ichiro Koga, Kyushu University, JapanCopyright © 2021 Ye, Huang, Hou, Long, Yin, Hu and Zhao. This is an open-access article distributed under the terms of the Creative Commons Attribution License (CC BY). The use, distribution or reproduction in other forums is permitted, provided the original author(s) and the copyright owner(s) are credited and that the original publication in this journal is cited, in accordance with accepted academic practice. No use, distribution or reproduction is permitted which does not comply with these terms.
*Correspondence: Guo-Jun Zhao, emhhb2d1b2p1bkBnemhtdS5lZHUuY24=; Ching Yuan Hu, Y2hpbmdodUBoYXdhaWkuZWR1
†These authors have contributed equally to this work
Disclaimer: All claims expressed in this article are solely those of the authors and do not necessarily represent those of their affiliated organizations, or those of the publisher, the editors and the reviewers. Any product that may be evaluated in this article or claim that may be made by its manufacturer is not guaranteed or endorsed by the publisher.
Research integrity at Frontiers
Learn more about the work of our research integrity team to safeguard the quality of each article we publish.