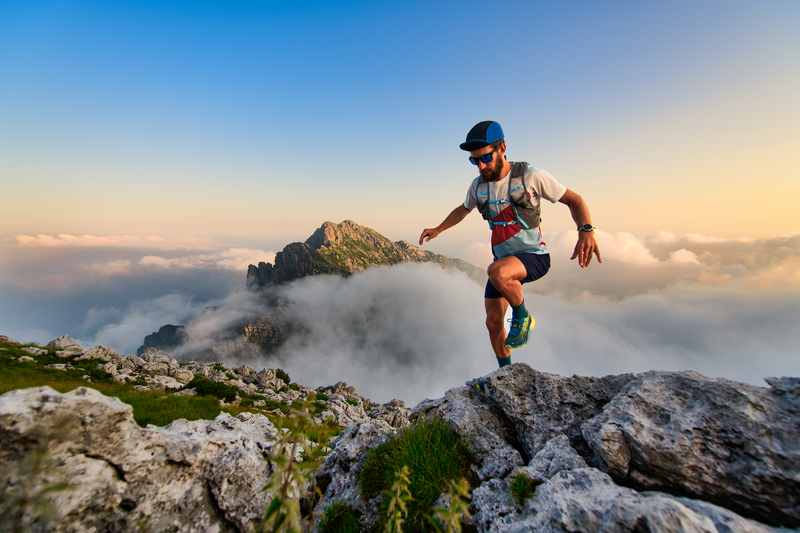
95% of researchers rate our articles as excellent or good
Learn more about the work of our research integrity team to safeguard the quality of each article we publish.
Find out more
REVIEW article
Front. Cardiovasc. Med. , 26 January 2021
Sec. Cardiovascular Therapeutics
Volume 8 - 2021 | https://doi.org/10.3389/fcvm.2021.633300
This article is part of the Research Topic New Strategies to Inhibit Cell Death in Myocardial Ischemia-Reperfusion Injury: How to succeed? View all 17 articles
Mesenchymal stem cell (MSC) transplantation after myocardial infarction (MI) has been shown to effectively limit the infarct area in numerous clinical and preclinical studies. However, the primary mechanism associated with this activity in MSC transplantation therapy remains unclear. Blood supply is fundamental for the survival of myocardial tissue, and the formation of an efficient vascular network is a prerequisite for blood flow. The paracrine function of MSCs, which is throughout the neovascularization process, including MSC mobilization, migration, homing, adhesion and retention, regulates angiogenesis and vasculogenesis through existing endothelial cells (ECs) and endothelial progenitor cells (EPCs). Additionally, MSCs have the ability to differentiate into multiple cell lineages and can be mobilized and migrate to ischemic tissue to differentiate into ECs, pericytes and smooth muscle cells in some degree, which are necessary components of blood vessels. These characteristics of MSCs support the view that these cells improve ischemic myocardium through angiogenesis and vasculogenesis. In this review, the results of recent clinical and preclinical studies are discussed to illustrate the processes and mechanisms of neovascularization in ischemic heart disease.
Ischemic heart disease (IHD) is characterized by reduced blood supply to the heart and is the leading cause of death and disability worldwide. Long-term myocardial ischemia and acute massive myocardial infarction often result in decreased left ventricular function. Although the development of new drugs and the use of stent implantations have benefited numerous patients with coronary heart disease, some patients still have no effective treatment due to issues associated with diffuse coronary artery lesion, postoperative restenosis and heart failure after myocardial infarction (MI).
The foundation of IHD treatment is the reconstruction of vessels and the recovery of blood flow. Over the past decades, with the introduction of the concept of therapeutic angiogenesis, more and more studies have demonstrated that neovascularization can effectively improve the blood supply of ischemic myocardium. There are two primary mechanisms by which neovascularization occurs: vasculogenesis and angiogenesis. Vasculogenesis is the in situ assembly of endothelial progenitors into capillaries, while angiogenesis is a process through which new blood vessels form from pre-existing vessels through sprouting and intussusception (1). Cytokine-based therapeutic angiogenesis from the bench to clinical trials has been a major focus of medical research, and the efficacy of vascular endothelial growth factor (VEGF) blockers has led to the approval of anti-angiogenesis drugs for cancer and eye disease. Conversely, the use of angiogenesis factors, such as VEGF and basic fibroblast growth factor (bFGF), has been shown to promote notable increases in collateral vessel and myocardial perfusion in ischemic myocardium, reduced infarct size and improved cardiac function (2), demonstrating the theoretical and experimental promise of this approach in treating ischemic diseases. Unfortunately, despite the exciting results obtained using angiogenesis factors to treat IHD, gene therapy is also limited by its restricted efficacy and resistance (3). For example, VEGF also accelerates angiogenesis in atherosclerotic plaques and promotes plaque growth, which may eventually lead to plaque instability, while it promotes angiogenesis in ischemic tissue, an observation referred to as the famous Janus phenomenon (4). Angiogenesis greatly improves blood flow in myocardial ischemia, but the safety of growth factor-based angiogenesis therapy is an issue that remains to be overcome. Thus, how to avoid the risks associated with angiogenesis therapy is a problem that must be considered.
Stem cell-based therapies provide a promising new method for the formation of new blood vessels. MSCs have become the most promising seed cells for the treatment of IHD, with advantages of rapid self-renewal, multidifferentiation potential, and weak immunogenicity in autologous transplantation. Clinical and preclinical studies have shown that MSCs therapy effectively limits the infarcted area and improves heart function. However, the mechanisms associated with the activities of MSCs in IHD therapy remain controversial. We primarily attribute the cardiac protective effect of MSCs to their ability to promote neovascularization for the following two reasons. First, MSCs secrete soluble paracrine factors that contribute to angiogenesis and vasculogenesis. Second, MSCs are able to differentiate into ECs, pericytes and smooth muscle cells (SMCs), which form the foundation of vessels, processes that both participate in the protective ability of MSCs toward IHD. In this review, we focus on the mechanisms and clinical applications of MSCs in IHD therapy through neovascularization to provide reference for the application of stem cells in IHD.
MSCs can be isolated from bone marrow, adipose tissue, umbilical cord blood, peripheral blood and almost every tissues in adults. Although MSCs can be harvested from different sources, regardless of their origin, they all have the capability of differentiating into adipocytes, osteoblasts and chondroblasts in vitro under specific conditions and can adhere to plastic under culture conditions. Furthermore, the surface of MSCs displays CD73, CD90, and CD105 but lack CD34, CD45, HLA-DR, CD14 or CD11b, CD79a or CD19. The International Society for Cell Therapy proposed the three criteria described above as identification standards for MSCs (5). Although MSCs from different sources share many of the same biological features, there are also some differences between distinct MSC populations. Bone marrow-derived MSCs (BMSCs), adipose-derived MSCs (AMSCs) and umbilical cord-derived MSCs (UCMSCs) are the most popular MSCs in clinical and preclinical experiments and trials, and some of their capabilities are compared below (Table 1).
MSCs have the ability to differentiate into adipocytes, osteoblasts and chondroblasts. The amount of calcium deposits and sulfated proteoglycans stained by Alizarin red and Alcian blue, respectively were both higher in BMSCs than that observed in AMSCs, indicating that BMSCs have a higher capacity toward osteogenic and chondrogenic differentiation than AMSCs. While similar adipogenic differentiation potential was observed between these two types of cells (6), some studies have reported that AMSCs are more prone to adipogenic differentiation than BMSCs (7). Baksh et al. (8) observed that compared to BMSCs, UCMSCs underwent osteogenic differentiation more rapidly, exhibited higher alkaline phosphatase activity, and generated significantly more fat-containing cells when grown under adipogenic conditions by day 21. The differentiation ability of stem cells is affected by donor sex, age, isolation and culture conditions, etc. (9). Thus, which types of MSCs have a greater ability to differentiate into adipocytes, osteoblasts and chondroblasts remains disputed. In addition, MSCs also have the ability to differentiate into ECs, pericytes and SMCs, which are necessary components of blood vessels (10–13). Lu et al. (14) showed that MSCs from adipose tissue may have significantly greater ability to promote angiogenesis both in vitro and in vivo than UCMSCs and endometrial MSCs.
MSCs from different tissue sources do not have the same proliferative ability in vitro. Choudhery et al. (15) observed that UCMSCs have higher population doublings than AMSCs (33.0 ± 1.5 vs. 25.8 ± 0.6), with the doubling time being longer for AMSCs (2.7 ± 0.03 days) than UCMSCs (2.0 ± 0.04 days). Moreover, after prolonged passaging (30 times), the proliferative ability of UCMSCs did not change significantly, while BMSCs showed decreased proliferation after 6 passages (8), indicating that UCMSCs have a stronger proliferative ability than BMMSCs and AMSCs. Under human platelet lysate-supplemented culture conditions, AMSCs were observed to have greater proliferative potential than BMSCs (6). Therefore, the proliferative ability of UCMSCs is the strongest, followed by AMSCs and BMSCs.
MSCs play an important role in posttraumatic tissue repair and cell therapy, and their migration ability is a key factor affecting their therapeutic efficacy. The migration capacity of BMSCs and placenta-derived MSCs (PMSCs) was observed to be 5.9- and 3.2-fold higher than that of UCMSCs, respectively. These results were consistent with the observed levels of migration-enhancing proteins in UCMSCs, including cathepsin B, cathepsin D and prohibitin, which were significantly lower than those observed in BMSCs and PMSCs, while the levels of migration-inhibiting proteins such as plasminogen activator inhibitor-1 and manganese superoxide dismutase were higher (16). Vimentin also contributed to the higher migration capability of BMSCs than UCMSCs (17). In contrast, UCMSCs exhibited an enhanced migration capacity toward factors released by hepatocellular carcinoma compared with BMSCs (18).
Tube formation is the last step in the formation of vessels and is necessary to supply blood for ischemia. Pill et al. (19) showed that AMSCs and BMSCs are both promising cell types to induce vascularization with ECs in vitro and are promising candidates to support in vivo vascularization. Nevertheless, Kim et al. (20) observed that conditioned medium from human AMSCs showed better tube formation-promoting effects than that from BMSCs in vitro, and AMSC group showed better recovery of blood flow than BMSC group in hindlimb ischemia model of nude mice. Furthermore, young AMSCs may have a higher tube formation capacity than old ones (21). UCMSCs are also capable of forming tubular networks (22). Du et al. (23) reported tube numbers of 11.65 ± 2.92, 0.91 ± 0.76 and 0.41 ± 0.20 for BMSC, AMSC and UMSC groups, respectively, indicating that BMSCs may have better angio-vasculogenic capacities than UMSCs and AMSCs. In contrast, Panepucci et al. (24) thought that UCMSCs would be more committed to angiogenesis and BMMSCs would be more committed to osteogenesis. Above all, there is still no consensus on which cell type has the greater capacity to promote tube formation.
The mechanism of MSC therapy is still controversial, because few MSCs can be found in myocardium after injection in vivo study. Wang et al. (25) showed that most intravenously injected MSCs remain in the lungs and liver, with only a small portion reaching the myocardial tissue. Similarly, Uemura et al. (26) observed only a few GFP-labeled MSCs in the periinfarct myocardium. Even so, clinical and preclinical studies still indicated the cardiac function of ischemic heart was improved, and infarct size and the number of apoptotic cardiomyocytes were significantly reduced after MSCs intervention. Which suggested the efficacy of MSCs did not benefited from themselves in some degree.
Paracrine hypothesis was firstly advanced by Gnecchi et al. (27). They found genetically modified BMSCs overexpressing the Akt1 released paracrine factors that exert cytoprotective effects on cardiomyocytes exposed to hypoxia and limited infarct size and improved ventricular function (27, 28). Furthermore, high VEGF, bFGF, IGF-1 and SDF-1 expression in hypoxia-preconditioned MSCs medium was examined, the results of which indicated that the paracrine function of MSCs may play more important role than their differentiation ability (26). Recently, it has been reported that MSCs secreted a wide array of cytokines that exerted beneficial angiogenesis in ischemic tissue, including PDGF, thrombopoietin, and angiogenin (29, 30). These factors are all involved in the neovascularization process, including MSC mobilization, migration, homing, adhesion and retention, and the differentiation of ECs. Especially VEGF and bFGF, which both have high affinity toward heparin and participate in angiogenic processes such as migration and amplification of ECs, are also necessary substances to induce the transformation of stem cells into ECs (10, 31). MSCs overexpressing Akt and angiopoietin-1 showed higher Flk1 and Flt1 positivity and promoted intrinsic Flk1+ and Flt1+ cell mobilization into the infarcted heart (32). Huang et al. (33) observed that overexpression of miR-126 promoted the differentiation of MSCs toward ECs through activation of the PI3K/Akt and MAPK/ERK pathways and the release of VEGF and bFGF factors. Therefore, paracrine factors secreted by MSCs may have pivotal functions throughout the neovascularization process. The role of various secretory factors in the neovascularization process will be discussed below.
The process by which MSCs promote neovascularization involves in a number of steps. First, once ischemia occurs which also follows stress change, MSCs can perceive the associated changes and are mobilized from their niches to migrate and adhere to ischemic tissue to proliferate and differentiate. Notably, MSCs secrete various factors, including chemokines and growth factors, and this paracrine function is carried out throughout the neovascularization process (Figure 1). The completion of all biological processes depends on the cooperation of different types of cells, and the neovascularization process requires the collaboration of ECs, endothelial progenitor cells (EPCs) and pericytes. In addition, exosomes derived from MSCs act as a messenger that participate in cell-to-cell communication.
Figure 1. The complete process by which MSCs respond to ischemia In general, MSCs are stored in their niches, which retain adult stem cell in a dormant state. Once tissue is damaged, signals, including those involve in ischemia-associated pathways, cell-cell interaction and stress mobilize stem cells to migrate from the stem-cell niche to damaged tissues, where they adhere, self-renew and differentiate. Once ischemia occurs, MSCs have the ability to secrete a number of growth factors through their paracrine function to promote new tube formation of ECs to provide new blood for ischemic tissue.
Despite the benefits of MSC transplantation in cardiac tissue, detailed in vivo observations have shown that MSCs only survive for a brief period after engraftment due to harsh microenvironmental conditions (including ischemia, inflammation and anoikis) in the infarcted myocardium (34). However, this environment contributes to the mobilization of MSCs from their niches.
MSCs originated from the bone marrow microenvironmental niche exhibit low oxygen tension. O2 is a necessary factor in the maintenance of cell life as the final receptor in the intracellular aerobic respiration electron transport chain and is a substrate of some enzymes. Once the supply of O2 is insufficient, the hypoxia signal will be rapidly transmitted to nucleus and initiate related gene expression to maintain oxygen homeostasis and the balance of energy metabolism between the cells and organism. Hypoxia inducible factor 1 (HIF-1), which has a dimeric complex composed of HIF-1a and HIF-b subunits, is oxygen-sensitive and the most important transcription factor affecting gene regulation under hypoxia (35). Once ischemia occurs, HIF-1 increases the expression of angiogenesis-associated genes, including VEGF, its receptors Flk-1 and Flt-1, bFGF and the fibrinogen system (36, 37). At the same time, HIF-1 improves the expression of proteases, such as membrane type matrix metalloproteinases, which hydrolyzes extracellular protein to promote cell migration, matrix reconstruction and the formation of tubule-like structures (38).
Hypoxia is also a basic aspect of the microenvironment that determines the differentiation of MSCs. Compared with a normoxia group, VEGF expression in embryonic and MSCs under hypoxia was observed to be significantly increased (39–41). Likewise, the in vivo administration of hypoxia-inducible VEGF-engineered MSCs was shown to induce ischemia-responsive VEGF production and lead to a significant increase in myocardial neovascularization after myocardial infarction in rats (39).
In 1997, Asahara et al. (42) identified and named a small population of CD34+ cells as “EC progenitors.” Indeed, EPCs are involved in a number of processes during angiogenesis, including mobilization, differentiation into ECs, homing, paracrine function and others (43, 44). Coculture of EPCs and MSCs significantly increased the transcription levels of endothelial specific markers, including vWF, CD31, VE-cadherin, Flk-1 and Flt-1 (45) and enhanced tube-like formation (46) through platelet derived growth factor (PDGF), Notch and TACE/TNF alpha signaling (45, 47). Joensuu et al. (48) noted that in cocultures of human MSCs and peripheral blood mononuclear cells, the previously nonadherent cells attached and started to elongate and form tube-like structures within 1 week concomitant with VEGFR1 upregulation, and platelet endothelial cell adhesion molecule 1 (PECAM-1) and endoglin-positive vessel-like structures were observed after 20 days. In addition, MSC-EC interactions were observed to decrease endothelial permeability induced by lipopolysaccharide through hepatocyte growth factor (HGF) by restoring the integrity of endothelial monolayers and remodeling endothelial intercellular junctions (49). VEGF secreted by stem cells from apical papilla is also used by human umbilical vein endothelial cells to increase the number of endothelial tubules, tubule lengths, and branching points (50).
There are many force-sensitive molecules on the cell surface, such as cilia, integrins, ion channels and plaque proteins. Integrins connect the cytoskeleton and extracellular matrix through adhesive plaque and transform the force signals into intracellular biological signals through this plaque (51, 52). Considering the key role of shear force in the differentiation of ECs, researchers reported that such mechanical stimulation in cell culture in vitro was equally effective for the differentiation of stem cells into ECs (53). MSCs are highly reactive to mechanical stimuli in the environment, and different types of stress on the same MSC population will lead to different differentiation results (54). After generating canine BMSCs under shear stress provided by a pulsatile bioreactor for 4 days, the expression of endothelial cell markers, such as PECAM-1, VE-cadherin and CD34 was observed to be significantly increased (55). Fisher et al. (56) noted that AMSCs could form cords but failed to take up acetylated low density lipoprotein (acLDL) or express molecular markers after being cultured in endothelial cell growth supplement. Only the subsequent exposure of stem cells to shear stress did the cells exhibit realignment, acLDL uptake and CD31expression, indicating that stem cells differentiation to ECs requires the synergism of biochemical and shear force.
MSC mobilization is key for its involvement in tissue repair following their sensing of hypoxia, stress or other signals. An anoxic environment is one of the factors that induces stem cells to migrate out of their niches. Prolyl hydroxylase (PHD) and factor inhibiting HIF-1 (FIH) are key oxygen sensors in MSCs. HIF-1α upregulation by double knockdown of PHD and FIH synergistically increases stem cell mobilization and myocardial angiogenesis and improves cardiac function (57). The high concentration of growth factors outside of stem-cell niches may be another factor causing MSCs to mobilize from their original niches. Stromal cell-derived factor-1 (SDF-1 α)/Cxc chemokine receptor 4 (CXCR-4) are part of the most important chemotactic axis regulating MSC mobilization and migration. VEGF and insulin-like growth factor-1 (IGF-1)-overexpressing MSCs accelerate BMSC mobilization via the activation of SDF-1 α/CXCR4 signaling to promote myocardial repair (58, 59). Wan et al. (60) showed that active transforming growth factor β (TGF-β) also control the mobilization and recruitment of MSCs to participate in vascular repair. In addition, high-intensity exercise may be a potent stimulus that promotes circulating mesenchymal cells mobilization in patients with stable coronary artery disease (61).
Homing and migration comprise a key step after MSC mobilization. Microenvironmental interactions between hypoxia and MSCs may control the ability of MSCs to migrate and their migration direction. In hypoxic tissue, SDF-1 and CXCR-4 are also important factors for cell migration. Ischemic myocardial and vascular tissues secrete SDF-1 to attract CXCR-4-expressing cells, particularly their therapeutic progenitors. Yu et al. (62) showed that SDF-1/CXCR-4 may mediate the migration of BMSCs toward heart MI through activation of PI3K/Akt signaling. Growth factors play an important role in the process of MSC migration. The stimulation of SDF-1α expression in infarcted hearts by VEGF-overexpressing MSCs was observed to result in the massive mobilization and homing of BMSCs (59). TGF-β1, HGF, IGF-1 and endothelial nitric oxide synthase (eNOS) also promoted the migration and homing BMSCs to the ischemic myocardium (63–65). Schmidt et al. (31) showed that low concentrations of bFGF attracted cells, indicating that bFGF may direct the migration of MSCs. In addition, Yan et al. (66) observed that C1q/tumor necrosis factor-related protein-9 (CTRP9) enhances AMSC proliferation and migration through the ERK1/2-MMP-9 signaling pathway and also promotes anti-apoptotic/cell survival via ERK-Nrf2/anti-oxidative protein expression. MiRNA, like miR-206 also involved in migration of MSCs by targeting Pim-1 (67).
Although MSCs transplantation is a promising therapeutic approach for IHD, the low viability of MSCs after transplantation needs to be improved. Hypoxic preconditioning may improve the functional survival and therapeutic efficiencies of engrafted BMSCs, at least in part through autophagy regulation (68). Some growth factors, including increased VEGF, TGF-β, IGF-1, SDF-1a and angiogenin were shown to enhance MSC survival and vasculogenesis in an MI model (69). Preconditioning with other factors, such as protein kinase C epsilon (εPKC), CTRP9, dimethyloxalylglycine and connexin-43 improves the retention and survival of transplanted MSCs in rat MI through the SDF-1/CXC and PI3K/AKT pathways (66, 70–72). Qu et al. (73) showed that atorvastatin, a hypolipidemic agent, has a protective effect on cardiomyocytes against apoptotic cell death in infarct and peri-infarct areas and could also increase the survival rate of implanted BMSCs in acute myocardial ischemia.
MSCs need to stay and adhere to ischemic tissue to play their important role in ischemic tissue repair. Molecular imaging studies have shown that <5% of MSCs engraft in ischemic tissues after being intravenously injected, with most of them dying within few hours after administration (74). This poor engraftment may be attributed to the constant blood flow and the harsh environmental conditions after acute ischemic injury. Since the long-term efficacy of cell therapy is proportional to the number of retained cells, this low retention and viability needs to be improved. Increasing the ability of MSCs to adhere to the ischemic tissues is key to improving their retention and viability. IGF-1 can increase the adhesion of MSCs and prolong their survival under hypoxia in vitro through PI3K activation (75). MSC adhesion can also be promoted by increasing the expression of integrin-linked kinase, periostin, and 2, 4-dinitrophenol (76–78). Reactive oxygen species (ROS) inhibit the cellular adhesion of engrafted MSCs, indicating that the elimination of ROS may be a novel strategy for improving the survival of engrafted MSCs (79). Bortolotti et al. (80) demonstrated that cardiotrophin-1 (CTF1) increases the retention and adhesion of BMMSCs to protect BMSCs from apoptosis, identifying it as a new powerful cytokine promoting cell engraftment. The retention and survival of transplanted MSCs was also shown to be improved by the overexpression of εPKC in AMI rats through the SDF-1/CXC and PI3K/AKT pathways (70).
Multiple cell types are known to be involved in the processes of angiogenesis and vasculogenesis, including MSCs, ECs, EPCs and pericytes. In particular, ECs are indispensable for angiogenesis and the relationship between MSCs and ECs mainly attribute to the following aspects. First, MSCs secret growth factors which can repair the injured but not dead ECs through their paracrine function. Second, the interaction and crosstalk between MSCs and existing ECs promotes the formation of new ECs and the repair of injured ECs. Third, MSCs have the potential to differentiate into new ECs although it is controversial. Last, MSCs also interact with EPCs and pericytes to influence EC formation and function (Figure 2).
Figure 2. MSCs and endothelial cells The relationship between MSCs and ECs can be summarized as follows. First, MSCs can repair the injured but not dead ECs through their paracrine function to induce the release of growth factors. Second, MSCs have the potential to differentiate into new ECs although it is controversial. Third, the interaction and crosstalk between MSCs and existing ECs promotes the formation of new ECs and the repair of injured ECs. Last, MSCs also interact with EPCs to influence EC formation and function.
The ability of MSCs to limit infarct size may attributed to their pro-angiogenesis activity through existing ECs (Figure 2). The stimulated angiogenic activity of ECs is associated with the secretion of various growth factors and cytokines, including VEGF, HGF, IL-6, TGF-β1 and monocyte chemoattractant protein-1 (81). Lu et al. (82) showed that nestin(+) BMSC transplantation improved cardiac function in a mouse AMI model by recruiting resident cardiac ECs to the infarcted border region. BMSCs also rescued injured ECs through modulation of mitophagy or activation of signaling pathways such as PI-3K/AKT/m-TOR/eNOS and p38/MAPK (83, 84). Hypoxia also influences the interactions between the endothelium and MSCs (85).
In addition to the interactions between MSCs and ECs, studies showed MSCs had the ability to differentiate into ECs to promote angiogenesis in some degree although it was still controversial. For example, Otto et al. (86) did not observe MSC transdifferentiation into cardiomyocytes, ECs or SMCs and that the transdifferentiation of MSCs into cardiomyocytes or vascular cells did not significantly contribute to the improvement of cardiac function. Conversely, Silva et al. (87) showed that BMSCs promoted the angiogenesis of dog ischemic myocardium by differentiating into ECs, which accelerated the establishment of collateral circulation. Studies support MSCs own the potential to differentiated into ECs according to the below reasons. First, MSCs are multipotent stem cells derived from the mesoderm. Theoretically, MSCs can be differentiated into all mesoderm derived cells, and since ECs are mesoderm-derived cells, MSCs have the potential to differentiate into ECs. Second, MSCs express molecular markers of early ECs, such as VEGF receptor 2 (VEGFR-2/Flk-1/KDR) and bFGF, indicating that ECs can be derived from mesenchymal colonies and that MSCs arise from precursors with angiogenic potential (31, 88). Last, a series of in vivo and in vitro experiments proved that MSCs can differentiate into ECs. Oswald et al. (10) successfully used 2% fetal bovine serum supplemented with 50 ng/mL VEGF to induce BMSCs to differentiate into ECs in vitro. They observed that differentiated cells increased the expression of endothelial-specific markers, such as KDR and VEGF receptor 1 (VEGFR-1/Flt-1), and formed capillary-like structures. Furthermore, the process of MSC differentiation into ECs may require the synergy of bFGF, IGF, epidermal growth factor (EGF) (89, 90). ERK signaling may also involve in the differentiation of porcine AMSCs into ECs (90).
Furthermore, MSCs also function with EPCs to promote tissue repair. As a precursor of ECs, EPCs also differentiate into ECs and promote ischemia angiogenesis through their paracrine function (91, 92). MSCs could attract and promote the migration and vascularization of EPCs, which may depend on a positive feedback loop between CXCR-2 and CXCR-4 (93, 94). The viability and ability of MSCs to promote nerve regeneration is also improved by EPCs through PDGF-BB/PDGFR-β signaling (95). Rossi et al. (96) found MSCs and EPCs into the hind limbs of ischemia model together accelerated ischemic muscle recovery through an endoglin-dependent mechanism. Consequently, MSCs, ECs and EPCs may have a synergistic effect in ischemic tissue repair (Figure 2).
Pericytes, also known as mural cells, wrap around ECs in arterioles, capillaries and venules to regulate the maturation of ECs, stabilize the microvascular wall and promote angiogenesis. Although pericytes are surrounded by a basement membrane, they contact the ECs with through a “peg and socket” mechanism through holes in the basement membrane. Studies have shown that pericytes also communicate with ECs via paracrine signaling to improve tissue repair (97, 98). It is notable that pericytes also have stem cell-like properties and exhibit the morphology, mitotic activity and surface antigens of MSCs (99) and are seemingly able to differentiate into adipocytes, chondrocytes, osteoblasts, neurons, astrocytes and oligodendrocytes, leading them to be identified as MSCs (100–102). However, it is still debated whether pericytes are MSCs. Guimaraes-Camboa et al. (103) challenged this concept and suggested that mural cells do not intrinsically behave as MSCs during aging and repair in multiple adult organs using a transgenic cell line. Over 2 years, the study showed that Tbx18 lineage-derived cells maintained their perivascular identity in the brain, heart, muscle and fat, indicating that mural cells do not exhibit an overt potential to give rise to other cell types. In contrast, MSCs can serve as a potential source of pericytes and induce vasculogenesis as mentioned previously (13, 104, 105), but similar to the multi-differentiation potential of MSCs, there needs to be standard guidelines for assessing pericyte differentiation in future studies. Furthermore, MSCs secrete various growth factors, including PDGF, which serves as a biomarker and crucial factor controlling the differentiation and recruitment of pericytes (106–108). These findings indicate that MSCs may regulate the recruitment of pericytes to injured tissue to participate in angiogenesis, but the associated mechanisms between MSCs and pericytes need to be further elucidated.
Exosomes are a type of extracellular microvesicle secreted by multiple eukaryotes. Compared with cell therapy, MSC-derived exosomes (MSC-exos) have lower immunogenicity and are safer and more efficient, providing a new strategy for tissue regeneration via cell-free therapy (109, 110). MSC-exos are a type of message carrier that harbor a modifiable content of microRNAs, mRNAs and proteins, mediating communication between cells and functioning as key mediators of the paracrine effect of MSCs (111, 112). The pro-angiogenesis function of MSC-exos has been demonstrated in a number of studies. For instance, exosomes from MSCs overexpressing Akt, HIF-1α or CXCR-4 were shown to accelerate EC proliferation, migration and tube-like structure formation in vitro, as well as blood vessel formation to improve cardiac function in an MI model (113–115). MSC-exosomes may also have anti-inflammatory activities in MI model (116). Currently, the application of MSC-exos primarily focuses on preclinical experiments. One of the key problems for exosome clinical therapy is how to collect and purify enough exosomes so that they can be used safely. Andriolo et al. (117) developed a GMP-class method for the mass preparation of stem cell-derived exosomes to enable them to be used in future clinical applications. Indeed, as they are secreted by MSCs, MSC-exos have similar biological properties to MSCs to some extent. MSC-exos also have paracrine functions and mediate communication between MSCs and ECs, and they are also influenced by microenvironmental stress conditions, such as hypoxia and irradiation (118, 119). Furthermore, as they harbor a part of and not the entirety of MSC contents, MSC-exos are not an MSC “mini-me” and cannot replace MSCs in some respects, including their multiple differentiation and proliferation abilities.
The results of numerous clinical and preclinical studies have indicated that MSC transplantation is safe, significantly improves cardiac function and decreases infarct size and fibrosis in ischemic patients, which may be associated with the survival, retention, angiogenesis, paracrine action and the anti-apoptosis activities of MSCs (Tables 2, 3). Although cellular therapies hold great promise for the treatment of human IHD and have good safety, the efficacy of MSCs remains disputable, especially when used in clinical trials. Meta-analyses of randomized clinical trials showed that the transplantation of BMSCs resulted in limited improvement on cardiac function for MI patients (171, 172). As it was showed in Table 2, some clinical trials proved MSC transplantation did not improve LVEF although it may limit infarct size. Different from clinical trials, MSC transplantation in animal experiments showed significantly elevated LVEF in most studies (Table 3).
Table 2. Completed clinical trials using MSCs to treat ischemic cardiovascular diseases registered at clinicaltrials.gov.
Clinical patients are different from animal ischemia models, and the efficacy of MSCs in clinical practice is influenced by many different factors, such as (1) disease etiology and severity of patients, and (2) the type, number, delivery route and time, retention, survival, proliferation and differentiation of MSCs. Another meta-analysis showed that MSCs are more effective in patients with lower baseline left ventricular ejection fraction (LVEF) (≤50%), and the effects of cells that were transferred at 3–7 days post-AMI was superior to those transferred within 24 h or more than 7 days in improving LVEF and decreasing LV end-systolic and diastolic dimensions (173), which suggested transplantation time was a key factor to influence cardiac function. Compared with clinical trials, animal experiments are easier to obtain positive results because of their simplicity, such as the MI model can be established uniformly by ligation of the left anterior descending coronary artery. Compared with MSCs intervention alone, pretreated MSCs with some growth factors together may get more efficacy (Table 3).
It is notable except for growth factors, more attention has been paid to natural botanical medicines. EGb761, an extract of Ginkgo biloba, was shown to exhibit a biphasic effect on hypoxia/serum deprivation-induced BMSC apoptosis, and its effect was closely associated with the PI3K/Akt and caspase-9 signaling pathways (174). Salvia miltiorrhiza is a widely used traditional Chinese medicine in cardiovascular diseases, and its constituent Tanshinone IIA was observed to decrease infarct size by increasing the recruitment of BMSCs to the infarct region by upregulating the SDF-1/CXCR-4 axis in a rat MI model (175). In addition to botanical medicines, chemicals such as statins, as the most commonly used lipid-lowering agents, exert activity toward a wide spectrum of cellular functions in addition to their lipid-lowering effects, including anti-inflammatory, anti-apoptotic, anti-fibrotic and pro-angiogenesis effects (176, 177). The results of multiple studies have suggested that atorvastatin has the ability to increase the survival rate of implanted BMSCs in an MI model, and combined with MSCs, it also ameliorated the cardiac milieu by reducing inflammatory cell infiltration, myeloperoxidase activity and cardiac fibrosis (178, 179). Furthermore, activation of the JAK-STAT pathway may play important role in the ability of rosuvastatin to increase the efficacy of transplanted MSCs (178). Other factors, including glucagon-like peptide-1-eluting (GLP-1), lipopolysaccharide and lysophosphatidic acid also exert pro-angiogenesis effect by promoting the enhanced expression of cytokines and growth factors (180–183).
MSCs display robust reparative properties through their paracrine and differentiation abilities that can limit apoptosis, enhance neovascularization and direct positive tissue remodeling. However, some problems with MSCs remain and must be solved before they can have widespread use. MSCs are important infiltrating cells that are also drived by blood and vasoconstriction. So the first problem is the low survival and retention of transplanted cells in vivo which limits their overall effectiveness in clinical usage. Consequently, identifying strategies to improve cell survival and retention in vivo is a priority. However, cell transplantation is affected by many factors, each of which may have an impact on the survival of transplanted cells, and there is still no consistent recommendation for each factor. The microenvironment of transplanted cells directly affects the survival of stem cells. The blood supply in the marginal area of myocardial infarction is well known to directly affect the survival rate and recovery of cardiac function after cell transplantation. One important goal of cell transplantation is to promote angiogenesis in the ischemic area and reduce the generation of myocardial scars. Studies have shown that hypoxia-induced stem cells release a variety of factors to improve the microenvironment through anti-inflammatory and anti-fibrosis effects and by promoting angiogenesis (170). Hypoxia or other growth factors used to precondition stem cells may allow MSC survival and retention to be improved, but additional comparisons and a set of standards are needed to identify the most powerful factors.
Another important factor limiting the clinical application of stem cells is the shortage of effective monitoring methods for stem cells. The successful implementation of cell therapies requires a better understanding of cell fate after transplantation. Currently, there are three primary labeling methods for stem cells, including reporter genes, fluorescent dyes and nanoparticles, which require optical imaging, MRI and radionuclide imaging to trace the transplanted stem cells, respectively or in combination, with each technique having its advantages and disadvantages (74, 184). Thus, there is an urgent need to develop a nontoxic and noninvasive tracer technology that exhibits long term stability and that can also be used to dynamically monitor the survival status of transplanted cells with respect to processes such as migration and differentiation in vivo.
WS contributed to the design and manuscript writing. QX, RY, and YY collected and assembled data. WC contributed the review design and financial support. KC was responsible for proofreading and final approval of the review. All authors contributed to the article and approved the submitted version.
This work was supported by the National Natural Science Foundation of China (No. 81803771).
The authors declare that the research was conducted in the absence of any commercial or financial relationships that could be construed as a potential conflict of interest.
1. Carmeliet P, Jain RK. Molecular mechanisms and clinical applications of angiogenesis. Nature. (2011) 473:298–307. doi: 10.1038/nature10144
2. Zachary I, Morgan RD. Therapeutic angiogenesis for cardiovascular disease: biological context, challenges, prospects. Heart. (2010) 97:181–9. doi: 10.1136/hrt.2009.180414
3. Henry TD, Annex BH, McKendall GR, Azrin MA, Lopez JJ, Giordano FJ, et al. The VIVA trial: vascular endothelial growth factor in ischemia for vascular angiogenesis. Circulation. (2003) 107:1359–65. doi: 10.1161/01.CIR.0000061911.47710.8A
4. Epstein SE. Janus phenomenon: the interrelated tradeoffs inherent in therapies designed to enhance collateral formation and those designed to inhibit atherogenesis. Circulation. (2004) 109:2826–31. doi: 10.1161/01.CIR.0000132468.82942.F5
5. Dominici M, Le Blanc K, Mueller I, Slaper-Cortenbach I, Marini FC, Krause DS, et al. Minimal criteria for defining multipotent mesenchymal stromal cells. The International Society for Cellular Therapy position statement. Cytotherapy. (2006) 8:315–7. doi: 10.1080/14653240600855905
6. Li C, Wu X, Tong J, Yang X, Zhao J, Zheng Q, et al. Comparative analysis of human mesenchymal stem cells from bone marrow and adipose tissue under xeno-free conditions for cell therapy. Stem Cell Res Ther. (2015) 6:55. doi: 10.1186/s13287-015-0066-5
7. Pachón-Peña G, Yu G, Tucker A, Wu X, Vendrell J, Bunnell BA, et al. Stromal stem cells from adipose tissue and bone marrow of age-matched female donors display distinct immunophenotypic profiles. J Cell Physiol. (2011) 226:843–51. doi: 10.1002/jcp.22408
8. Baksh D, Yao R, Tuan RS. Comparison of proliferative and multilineage differentiation potential of human mesenchymal stem cells derived from umbilical cord and bone marrow. Stem Cells. (2007) 25:1384–92. doi: 10.1634/stemcells.2006-0709
9. Zhu M, Kohan E, Bradley J, Hedrick M, Benhaim P, Zuk P. The effect of age on osteogenic, adipogenic and proliferative potential of female adipose-derived stem cells. J Tissue Eng Regen M. (2009) 3:290–301. doi: 10.1002/term.165
10. Oswald J, Boxberger S, Jorgensen B, Feldmann S, Ehninger G, Bornhauser M, et al. Mesenchymal stem cells can be differentiated into endothelial cells in vitro. Stem Cells. (2004) 22:377–84. doi: 10.1634/stemcells.22-3-377
11. Gu W, Hong X, Le Bras A, Nowak WN, Issa BS, Deng J, et al. Smooth muscle cells differentiated from mesenchymal stem cells are regulated by microRNAs and suitable for vascular tissue grafts. J Biol Chem. (2018) 293:8089–102. doi: 10.1074/jbc.RA118.001739
12. Jeon ES, Park WS, Lee MJ, Kim YM, Han J, Kim JH. A Rho kinase/myocardin-related transcription factor-A-dependent mechanism underlies the sphingosylphosphorylcholine-induced differentiation of mesenchymal stem cells into contractile smooth muscle cells. Circ Res. (2008) 103:635–42. doi: 10.1161/CIRCRESAHA.108.180885
13. Wang HH, Cui YL, Zaorsky NG, Lan J, Deng L, Zeng XL, et al. Mesenchymal stem cells generate pericytes to promote tumor recurrence via vasculogenesis after stereotactic body radiation therapy. Cancer Lett. (2016) 375:349–59. doi: 10.1016/j.canlet.2016.02.033
14. Lu H, Wang F, Mei H, Wang S, Cheng L. Human adipose mesenchymal stem cells show more efficient angiogenesis promotion on endothelial colony-forming cells than umbilical cord and endometrium. Stem Cells Int. (2018) 2018:7537589. doi: 10.1155/2018/7537589
15. Choudhery MS, Badowski M, Muise A, Harris DT. Comparison of human mesenchymal stem cells derived from adipose and cord tissue. Cytotherapy. (2013) 15:330–43. doi: 10.1016/j.jcyt.2012.11.010
16. Li G, Zhang X, Wang H, Wang X, Meng C, Chan C, et al. Comparative proteomic analysis of mesenchymal stem cells derived from human bone marrow, umbilical cord, and placenta: Implication in the migration. Proteomics. (2009) 9:20–30. doi: 10.1002/pmic.200701195
17. Huang L, Niu C, Willard B, Zhao W, Liu L, He W, et al. Proteomic analysis of porcine mesenchymal stem cells derived from bone marrow and umbilical cord: implication of the proteins involved in the higher migration capability of bone marrow mesenchymal stem cells. Stem Cell Res Ther. (2015) 6:77. doi: 10.1186/s13287-015-0061-x
18. Bayo J, Fiore E, Aquino JB, Malvicini M, Rizzo M, Peixoto E, et al. Human umbilical cord perivascular cells exhibited enhanced migration capacity towards hepatocellular carcinoma in comparison with bone marrow mesenchymal stromal cells: a role for autocrine motility factor receptor. Biomed Res Int. (2014) 2014:1–9. doi: 10.1155/2014/837420
19. Pill K, Hofmann S, Redl H, Holnthoner W. Vascularization mediated by mesenchymal stem cells from bone marrow and adipose tissue: a comparison. Cell Regener. (2015) 4:4–8. doi: 10.1186/s13619-015-0025-8
20. Kim Y, Kim H, Cho H, Bae Y, Suh K, Jung J. Direct comparison of human mesenchymal stem cells derived from adipose tissues and bone marrow in mediating neovascularization in response to vascular ischemia. Cell Physiol Biochem. (2007) 20:867–76. doi: 10.1159/000110447
21. Efimenko A, Starostina E, Kalinina N, Stolzing A. Angiogenic properties of aged adipose derived mesenchymal stem cells after hypoxic conditioning. J Transl Med. (2011) 9:10. doi: 10.1186/1479-5876-9-10
22. Arutyunyan I, Fatkhudinov T, Kananykhina E, Usman N, Elchaninov A, Makarov A, et al. Role of VEGF-A in angiogenesis promoted by umbilical cord-derived mesenchymal stromal/stem cells: in vitro study. Stem Cell Res Ther. (2016) 7:46. doi: 10.1186/s13287-016-0305-4
23. Du WJ, Chi Y, Yang ZX, Li ZJ, Cui JJ, Song BQ, et al. Heterogeneity of proangiogenic features in mesenchymal stem cells derived from bone marrow, adipose tissue, umbilical cord, and placenta. Stem Cell Res Ther. (2016) 7:163. doi: 10.1186/s13287-016-0418-9
24. Panepucci RA, Siufi JL, Silva WJ, Proto-Siquiera R, Neder L, Orellana M, et al. Comparison of gene expression of umbilical cord vein and bone marrow-derived mesenchymal stem cells. Stem Cells. (2004) 22:1263–78. doi: 10.1634/stemcells.2004-0024
25. Wang C, Cheng L, Xu H, Liu Z. Towards whole-body imaging at the single cell level using ultra-sensitive stem cell labeling with oligo-arginine modified upconversion nanoparticles. Biomaterials. (2012) 33:4872–81. doi: 10.1016/j.biomaterials.2012.03.047
26. Uemura R, Xu M, Ahmad N, Ashraf M. Bone marrow stem cells prevent left ventricular remodeling of ischemic heart through paracrine signaling. Circ Res. (2006) 98:1414–21. doi: 10.1161/01.RES.0000225952.61196.39
27. Gnecchi M, He H, Liang OD, Melo LG, Morello F, Mu H, et al. Paracrine action accounts for marked protection of ischemic heart by Akt-modified mesenchymal stem cells. Nat Med. (2005) 11:367–8. doi: 10.1038/nm0405-367
28. Gnecchi M, He H, Noiseux N, Liang OD, Zhang L, Morello F, et al. Evidence supporting paracrine hypothesis for Akt-modified mesenchymal stem cell-mediated cardiac protection and functional improvement. FASEB J. (2006) 20:661–9. doi: 10.1096/fj.05-5211com
29. Danieli P, Malpasso G, Ciuffreda MC, Cervio E, Calvillo L, Copes F, et al. Conditioned medium from human amniotic mesenchymal stromal cells limits infarct size and enhances angiogenesis. Stem Cell Transl Med. (2015) 4:448–58. doi: 10.5966/sctm.2014-0253
30. Yoon BS, Moon J, Jun EK, Kim J, Maeng I, Kim JS, et al. Secretory profiles and wound healing effects of human amniotic fluid–derived mesenchymal stem cells. Stem Cells Dev. (2010) 19:887–902. doi: 10.1089/scd.2009.0138
31. Schmidt A, Ladage D, Schinköthe T, Klausmann U, Ulrichs C, Klinz F, et al. Basic fibroblast growth factor controls migration in human mesenchymal stem cells. Stem Cells. (2006) 24:1750–8. doi: 10.1634/stemcells.2005-0191
32. Lai VK, Afzal MR, Ashraf M, Jiang S, Haider HK. Non-hypoxic stabilization of HIF-Iα during coordinated interaction between Akt and angiopoietin-1 enhances endothelial commitment of bone marrow stem cells. J Mol Med. (2012) 90:719–30. doi: 10.1007/s00109-011-0852-1
33. Huang F, Fang Z, Hu X, Tang L, Zhou S, Huang J. Overexpression of miR-126 promotes the differentiation of mesenchymal stem cells toward endothelial cells via activation of PI3K/Akt and MAPK/ERK pathways and release of paracrine factors. Biol Chem. (2013) 394:1223–33. doi: 10.1515/hsz-2013-0107
34. Lu W, Xie Z, Tang Y, Bai L, Yao Y, Fu C, et al. Photoluminescent mesoporous silicon nanoparticles with siCCR2 improve the effects of mesenchymal stromal cell transplantation after acute myocardial infarction. Theranostics. (2015) 5:1068–82. doi: 10.7150/thno.11517
35. Ramirez-Bergeron DL, Simon MC. Hypoxia-inducible factor and the development of stem cells of the cardiovascular system. Stem Cells. (2001) 19:279–86. doi: 10.1634/stemcells.19-4-279
36. Kanichai M, Ferguson D, Prendergast PJ, Campbell VA. Hypoxia promotes chondrogenesis in rat mesenchymal stem cells: A role for AKT and hypoxia-inducible factor (HIF)-1α. J Cell Physiol. (2008) 216:708–15. doi: 10.1002/jcp.21446
37. Koong AC, Denko NC, Hudson KM, Schindler C, Swiersz L, Koch C, et al. Candidate genes for the hypoxic tumor phenotype. Cancer Res. (2000) 60:883–7. Available online at: https://cancerres.aacrjournals.org/content/60/4/883.long
38. Kajita M, Itoh Y, Chiba T, Mori H, Okada A, Kinoh H, et al. Membrane-type 1 matrix metalloproteinase cleaves CD44 and promotes cell migration. J Cell Biol. (2001) 153:893–904. doi: 10.1083/jcb.153.5.893
39. Kim SH, Moon HH, Kim HA, Hwang KC, Lee M, Choi D. Hypoxia-inducible vascular endothelial growth factor-engineered mesenchymal stem cells prevent myocardial ischemic injury. Mol Ther. (2011) 19:741–50. doi: 10.1038/mt.2010.301
40. Han Y, Kuang S, Gomer A, Ramirez-Bergeron DL. Hypoxia influences the vascular expansion and differentiation of embryonic stem cell cultures through the temporal expression of vascular endothelial growth factor receptors in an ARNT-dependent manner. Stem Cells. (2010) 28:799–809. doi: 10.1002/stem.316
41. Prado-Lopez S, Conesa A, Armià à N A, Martà Nez-Losa M, Escobedo-Lucea C, Gandia C, et al. Hypoxia promotes efficient differentiation of human embryonic stem cells to functional endothelium. Stem Cells. (2010) 28:799–809. doi: 10.1002/stem.295
42. Asahara T, Murohara T, Sullivan A, Silver M, van der Zee R, Li T, et al. Isolation of putative progenitor endothelial cells for angiogenesis. Science. (1997) 275:964–7. doi: 10.1126/science.275.5302.964
43. Hristov M, Erl W, Weber PC. Endothelial progenitor cells: mobilization, differentiation, and homing. Arterioscler Thromb Vasc Biol. (2003) 23:1185–9. doi: 10.1161/01.ATV.0000073832.49290.B5
44. Laurenzana A, Fibbi G, Margheri F, Biagioni A, Luciani C, Del RM, et al. Endothelial progenitor cells in sprouting angiogenesis: proteases pave the way. Curr Mol Med. (2015) 15:606–20. doi: 10.2174/1566524015666150831131214
45. Xu J, Liu X, Chen J, Zacharek A, Cui X, Savant-Bhonsale S, et al. Cell-cell interaction promotes rat marrow stromal cell differentiation into endothelial cell via activation of TACE/TNF-α Signaling. Cell Transplant. (2010) 19:43–53. doi: 10.3727/096368909X474339
46. Aguirre A, Planell JA, Engel E. Dynamics of bone marrow-derived endothelial progenitor cell/mesenchymal stem cell interaction in co-culture and its implications in angiogenesis. Biochem Bioph Res Co. (2010) 400:284–91. doi: 10.1016/j.bbrc.2010.08.073
47. Liang T, Zhu L, Gao W, Gong M, Ren J, Yao H, et al. Coculture of endothelial progenitor cells and mesenchymal stem cells enhanced their proliferation and angiogenesis through PDGF and Notch signaling. Febs Open Bio. (2017) 7:1722–36. doi: 10.1002/2211-5463.12317
48. Joensuu K, Paatero I, Alm JJ, Elenius K, Aro HT, Heino TJ, et al. Interaction between marrow-derived human mesenchymal stem cells and peripheral blood mononuclear cells in endothelial cell differentiation. Scand J Surg. (2011) 100:216–22. doi: 10.1177/145749691110000314
49. Chen Q, Liu A, Qiu H, Yang Y. Interaction between mesenchymal stem cells and endothelial cells restores endothelial permeability via paracrine hepatocyte growth factor in vitro. Stem Cell Res Ther. (2015) 6:44. doi: 10.1186/s13287-015-0025-1
50. Yuan C, Wang P, Zhu L, Dissanayaka WL, Green DW, Tong EHY, et al. Coculture of stem cells from apical papilla and human umbilical vein endothelial cell under hypoxia increases the formation of three-dimensional vessel-like structures in vitro. Tissue Eng. (2015) 21:1163–72. doi: 10.1089/ten.tea.2014.0058
51. Shyy JYJ. Role of integrins in endothelial mechanosensing of shear stress. Circ Res. (2002) 91:769–75. doi: 10.1161/01.RES.0000038487.19924.18
52. Schwartz MA, Assoian RK. Integrins and cell proliferation: regulation of cyclin-dependent kinases via cytoplasmic signaling pathways. J Cell Sci. (2001) 114(Pt 14):2553–60.
53. Ando J, Yamamoto K. Vascular mechanobiology: endothelial cell responses to fluid shear stress. Circ J. (2009) 73:1983–92. doi: 10.1253/circj.CJ-09-0583
54. Maul TM, Chew DW, Nieponice A, Vorp DA. Mechanical stimuli differentially control stem cell behavior: morphology, proliferation, and differentiation. Biomech Model Mechan. (2011) 10:939–53. doi: 10.1007/s10237-010-0285-8
55. Dong JD, Gu YQ, Li CM, Wang CR, Feng ZG, Qiu RX, et al. Response of mesenchymal stem cells to shear stress in tissue-engineered vascular grafts. Acta Pharmacol Sin. (2009) 30:530–6. doi: 10.1038/aps.2009.40
56. Fischer LJ, McIlhenny S, Tulenko T, Golesorkhi N, Zhang P, Larson R, et al. Endothelial differentiation of adipose-derived stem cells: effects of endothelial cell growth supplement and shear force. J Surg Res. (2009) 152:157–66. doi: 10.1016/j.jss.2008.06.029
57. Huang M, Nguyen P, Jia F, Hu S, Gong Y, de Almeida PE, et al. Double knockdown of prolyl hydroxylase and factor-inhibiting hypoxia-inducible factor with nonviral minicircle gene therapy enhances stem cell mobilization and angiogenesis after myocardial infarction. Circulation. (2011) 124(11 Suppl.):S46–54. doi: 10.1161/CIRCULATIONAHA.110.014019
58. Haider H, Jiang S, Idris NM, Ashraf M. IGF-1-overexpressing mesenchymal stem cells accelerate bone marrow stem cell mobilization via paracrine activation of SDF-1alpha/CXCR4 signaling to promote myocardial repair. Circ Res. (2008) 103:1300–8. doi: 10.1161/CIRCRESAHA.108.186742
59. Tang JM, Wang JN, Zhang L, Zheng F, Yang JY, Kong X, et al. VEGF/SDF-1 promotes cardiac stem cell mobilization and myocardial repair in the infarcted heart. Cardiovasc Res. (2011) 91:402–11. doi: 10.1093/cvr/cvr053
60. Wan M, Li C, Zhen G, Jiao K, He W, Jia X, et al. Injury-activated transforming growth factor β controls mobilization of mesenchymal stem cells for tissue remodeling. Stem Cells. (2012) 30:2498–511. doi: 10.1002/stem.1208
61. Lucia A, De La Rosa A, Silvan MA, Lopez-Mojares LM, Boraita A, Perez M, et al. Mobilisation of mesenchymal cells in cardiac patients: is intense exercise necessary? Br J Sports Med. (2009) 43:221–3. doi: 10.1136/bjsm.2007.044693
62. Yu J, Li M, Qu Z, Yan D, Li D, Ruan Q. SDF-1/CXCR4-mediated migration of transplanted bone marrow stromal cells toward areas of heart myocardial infarction through activation of PI3K/Akt. J Cardiovasc Pharmacol. (2010) 55:496–505. doi: 10.1097/FJC.0b013e3181d7a384
63. Zhang SJ, Song XY, He M, Yu SB. Effect of TGF-beta1/SDF-1/CXCR4 signal on BM-MSCs homing in rat heart of ischemia/perfusion injury. Eur Rev Med Pharmacol Sci. (2016) 20:899–905.
64. Zhang GW, Gu TX, Guan XY, Sun XJ, Qi X, Li XY, et al. HGF and IGF-1 promote protective effects of allogeneic BMSC transplantation in rabbit model of acute myocardial infarction. Cell Prolif. (2015) 48:661–70. doi: 10.1111/cpr.12219
65. Li N, Lu X, Zhao X, Xiang FL, Xenocostas A, Karmazyn M, et al. Endothelial nitric oxide synthase promotes bone marrow stromal cell migration to the ischemic myocardium via upregulation of stromal cell-derived factor-1alpha. Stem Cells. (2009) 27:961–70. doi: 10.1002/stem.6
66. Yan W, Guo Y, Tao L, Lau WB, Gan L, Yan Z, et al. C1q/tumor necrosis factor–related protein-9 regulates the fate of implanted mesenchymal stem cells and mobilizes their protective effects against ischemic heart injury via multiple novel signaling pathways. Circulation. (2017) 136:2162–77. doi: 10.1161/CIRCULATIONAHA.117.029557
67. Zhang Y, Lei W, Yan W, Li X, Wang X, Zhao Z, et al. microRNA-206 is involved in survival of hypoxia preconditioned mesenchymal stem cells through targeting Pim-1 kinase. Stem Cell Res Ther. (2016) 7:61. doi: 10.1186/s13287-016-0318-z
68. Zhang Z, Yang C, Shen M, Yang M, Jin Z, Ding L, et al. Autophagy mediates the beneficial effect of hypoxic preconditioning on bone marrow mesenchymal stem cells for the therapy of myocardial infarction. Stem Cell Res Ther. (2017) 8:89. doi: 10.1186/s13287-017-0543-0
69. Liu XH, Bai CG, Xu ZY, Huang SD, Yuan Y, Gong DJ, et al. Therapeutic potential of angiogenin modified mesenchymal stem cells: angiogenin improves mesenchymal stem cells survival under hypoxia and enhances vasculogenesis in myocardial infarction. Microvasc Res. (2008) 76:23–30. doi: 10.1016/j.mvr.2008.02.005
70. He H, Zhao ZH, Han FS, Liu XH, Wang R, Zeng YJ. Overexpression of protein kinase C varepsilon improves retention and survival of transplanted mesenchymal stem cells in rat acute myocardial infarction. Cell Death Dis. (2016) 7:e2056. doi: 10.1038/cddis.2015.417
71. Liu XB, Wang JA, Ji XY, Yu SP, Wei L. Preconditioning of bone marrow mesenchymal stem cells by prolyl hydroxylase inhibition enhances cell survival and angiogenesis in vitro and after transplantation into the ischemic heart of rats. Stem Cell Res Ther. (2014) 5:111. doi: 10.1186/scrt499
72. Wang D, Shen W, Zhang F, Chen M, Chen H, Cao K. Connexin43 promotes survival of mesenchymal stem cells in ischaemic heart. Cell Biol Int. (2010) 34:415–23. doi: 10.1042/CBI20090118
73. Qu Z, Xu H, Tian Y, Jiang X. Atorvastatin improves microenvironment to enhance the beneficial effects of BMSCs therapy in a rabbit model of acute myocardial infarction. Cell Physiol Biochem. (2013) 32:380–9. doi: 10.1159/000354445
74. Nguyen PK, Riegler J, Wu JC. Stem cell imaging: from bench to bedside. Cell Stem Cell. (2014) 14:431–44. doi: 10.1016/j.stem.2014.03.009
75. Enoki C, Otani H, Sato D, Okada T, Hattori R, Imamura H. Enhanced mesenchymal cell engraftment by IGF-1 improves left ventricular function in rats undergoing myocardial infarction. Int J Cardiol. (2010) 138:9–18. doi: 10.1016/j.ijcard.2009.04.012
76. Song SW, Chang W, Song BW, Song H, Lim S, Kim HJ, et al. Integrin-linked kinase is required in hypoxic mesenchymal stem cells for strengthening cell adhesion to ischemic myocardium. Stem Cells. (2009) 27:1358–65. doi: 10.1002/stem.47
77. Cho YH, Cha MJ, Song BW, Kim IK, Song H, Chang W, et al. Enhancement of MSC adhesion and therapeutic efficiency in ischemic heart using lentivirus delivery with periostin. Biomaterials. (2012) 33:1376–85. doi: 10.1016/j.biomaterials.2011.10.078
78. Khan I, Ali A, Akhter MA, Naeem N, Chotani MA, Mustafa T, et al. Preconditioning of mesenchymal stem cells with 2,4-dinitrophenol improves cardiac function in infarcted rats. Life Sci. (2016) 162:60–9. doi: 10.1016/j.lfs.2016.08.014
79. Song H, Cha MJ, Song BW, Kim IK, Chang W, Lim S, et al. Reactive oxygen species inhibit adhesion of mesenchymal stem cells implanted into ischemic myocardium via interference of focal adhesion complex. Stem Cells. (2010) 28:555–63. doi: 10.1002/stem.302
80. Bortolotti F, Ruozi G, Falcione A, Doimo S, Dal Ferro M, Lesizza P, et al. In vivo functional selection identifies cardiotrophin-1 as a cardiac engraftment factor for mesenchymal stromal cells. Circulation. (2017) 136:1509–24. doi: 10.1161/CIRCULATIONAHA.117.029003
81. Kwon HM, Hur S, Park K, Kim C, Kim Y, Kim H, et al. Multiple paracrine factors secreted by mesenchymal stem cells contribute to angiogenesis. Vasc Pharmacol. (2014) 63:19–28. doi: 10.1016/j.vph.2014.06.004
82. Lu D, Liao Y, Zhu SH, Chen QC, Xie DM, Liao JJ, et al. Bone-derived Nestin-positive mesenchymal stem cells improve cardiac function via recruiting cardiac endothelial cells after myocardial infarction. Stem Cell Res Ther. (2019) 10:127. doi: 10.1186/s13287-019-1217-x
83. Zhu W, Yuan Y, Liao G, Li L, Liu J, Chen Y, et al. Mesenchymal stem cells ameliorate hyperglycemia-induced endothelial injury through modulation of mitophagy. Cell Death Dis. (2018) 9:837. doi: 10.1038/s41419-018-0861-x
84. Xu T, Lv Z, Chen Q, Guo M, Wang X, Huang F. Vascular endothelial growth factor over-expressed mesenchymal stem cells-conditioned media ameliorate palmitate-induced diabetic endothelial dysfunction through PI-3K/AKT/m-TOR/eNOS and p38/MAPK signaling pathway. Biomed Pharmacother. (2018) 106:491–8. doi: 10.1016/j.biopha.2018.06.129
85. Xu L, Willumeit-Romer R, Luthringer-Feyerabend B. Hypoxia influences the effects of magnesium degradation products on the interactions between endothelial and mesenchymal stem cells. Acta Biomater. (2020) 101:624–36. doi: 10.1016/j.actbio.2019.10.018
86. Otto BJ, Oie E, Shahdadfar A, Karlsen T, Muller RM, Aakhus S, et al. Intramyocardial injections of human mesenchymal stem cells following acute myocardial infarction modulate scar formation and improve left ventricular function. Cell Transplant. (2012) 21:1697–709. doi: 10.3727/096368911X627462
87. Silva GV. Mesenchymal stem cells differentiate into an endothelial phenotype, enhance vascular density, and improve heart function in a canine chronic ischemia model. Circulation. (2005) 111:150–6. doi: 10.1161/01.CIR.0000151812.86142.45
88. Vodyanik MA, Yu J, Zhang X, Tian S, Stewart R, Thomson JA, et al. A mesoderm-derived precursor for mesenchymal stem and endothelial cells. Cell Stem Cell. (2010) 7:718–29. doi: 10.1016/j.stem.2010.11.011
89. Wang C, Li Y, Yang M, Zou Y, Liu H, Liang Z, et al. Efficient differentiation of bone marrow mesenchymal stem cells into endothelial cells in vitro. Eur J Vasc Endovasc. (2018) 55:257–65. doi: 10.1016/j.ejvs.2017.10.012
90. Almalki SG, Agrawal DK. ERK signaling is required for VEGF-A/VEGFR2-induced differentiation of porcine adipose-derived mesenchymal stem cells into endothelial cells. Stem Cell Res Ther. (2017) 8:113. doi: 10.1186/s13287-017-0568-4
91. Murasawa S, Asahara T. Endothelial progenitor cells for vasculogenesis. Physiology. (2005) 20:36–42. doi: 10.1152/physiol.00033.2004
92. Zisch AH. Tissue engineering of angiogenesis with autologous endothelial progenitor cells. Curr Opin Biotechnol. (2004) 15:424–9. doi: 10.1016/j.copbio.2004.08.005
93. Tan Y, Shu L, Xu P, Bai S. Mesenchymal stem cells attract endothelial progenitor cells via a positive feedback loop between CXCR2 and CXCR4. Stem Cells Int. (2019) 2019:4197164. doi: 10.1155/2019/4197164
94. Li Z, Yang A, Yin X, Dong S, Luo F, Dou C, et al. Mesenchymal stem cells promote endothelial progenitor cell migration, vascularization, and bone repair in tissue-engineered constructs via activating CXCR2-Src-PKL/Vav2-Rac1. Faseb J. (2018) 32:2197–211. doi: 10.1096/fj.201700895R
95. Fang J, Huang X, Han X, Zheng Z, Hu C, Chen T, et al. Endothelial progenitor cells promote viability and nerve regenerative ability of mesenchymal stem cells through PDGF-BB/PDGFR-beta signaling. Aging. (2020) 12:106–21. doi: 10.18632/aging.102604
96. Rossi E, Smadja D, Goyard C, Cras A, Dizier B, Bacha N, et al. Co-injection of mesenchymal stem cells with endothelial progenitor cells accelerates muscle recovery in hind limb ischemia through an endoglin-dependent mechanism. Thromb Haemost. (2017) 117:1908–18. doi: 10.1160/TH17-01-0007
97. Yonekura H, Sakurai S, Liu X, Migita H, Wang H, Yamagishi S, et al. Placenta growth factor and vascular endothelial growth factor B and C expression in microvascular endothelial cells and pericytes. Implication in autocrine and paracrine regulation of angiogenesis. J Biol Chem. (1999) 274:35172–8. doi: 10.1074/jbc.274.49.35172
98. Watanabe S, Morisaki N, Tezuka M, Fukuda K, Ueda S, Koyama N, et al. Cultured retinal pericytes stimulate in vitro angiogenesis of endothelial cells through secretion of a fibroblast growth factor-like molecule. Atherosclerosis. (1997) 130:101–7. doi: 10.1016/S0021-9150(96)06050-9
99. Corselli M, Chen C, Crisan M, Lazzari L, Péault B. Perivascular ancestors of adult multipotent stem cells. Arterioscler Thromb Vasc Biol. (2010) 30:1104–9. doi: 10.1161/ATVBAHA.109.191643
100. Crisan M, Yap S, Casteilla L, Chen CW, Corselli M, Park TS, et al. A perivascular origin for mesenchymal stem cells in multiple human organs. Cell Stem Cell. (2008) 3:301–13. doi: 10.1016/j.stem.2008.07.003
101. Tang W, Zeve D, Suh JM, Bosnakovski D, Kyba M, Hammer RE, et al. White fat progenitor cells reside in the adipose vasculature. Science. (2008) 322:583–6. doi: 10.1126/science.1156232
102. Dore-Duffy P, Katychev A, Wang X, Van Buren E. CNS microvascular pericytes exhibit multipotential stem cell activity. J Cereb Blood Flow Metab. (2006) 26:613–24. doi: 10.1038/sj.jcbfm.9600272
103. Guimaraes-Camboa N, Cattaneo P, Sun Y, Moore-Morris T, Gu Y, Dalton ND, et al. Pericytes of multiple organs do not behave as mesenchymal stem cells in vivo. Cell Stem Cell. (2017) 20:345–59. doi: 10.1016/j.stem.2016.12.006
104. Xu J, Gong T, Heng BC, Zhang CF. A systematic review: differentiation of stem cells into functional pericytes. FASEB J. (2017) 31:1775–86. doi: 10.1096/fj.201600951RRR
105. Natesan S, Zhang G, Baer DG, Walters TJ, Christy RJ, Suggs LJ. A bilayer construct controls adipose-derived stem cell differentiation into endothelial cells and pericytes without growth factor stimulation. Tissue Eng Part A. (2011) 17:941–53. doi: 10.1089/ten.tea.2010.0294
106. Betsholtz C, Keller A. PDGF, pericytes and the pathogenesis of idiopathic basal ganglia calcification (IBGC). Brain Pathol. (2014) 24:387–95. doi: 10.1111/bpa.12158
107. Lindblom P, Gerhardt H, Liebner S, Abramsson A, Enge M, Hellstrom M, et al. Endothelial PDGF-B retention is required for proper investment of pericytes in the microvessel wall. Genes Dev. (2003) 17:1835–40. doi: 10.1101/gad.266803
108. Bergers G, Song S. The role of pericytes in blood-vessel formation and maintenance. Neuro-Oncology. (2005) 7:452–64. doi: 10.1215/S1152851705000232
109. Yu B, Zhang X, Li X. Exosomes derived from mesenchymal stem cells. Int J Mol Sci. (2014) 15:4142–57. doi: 10.3390/ijms15034142
110. Andaloussi SE, Mager I, Breakefield XO, Wood MJ. Extracellular vesicles: biology and emerging therapeutic opportunities. Nat Rev Drug Discov. (2013) 12:347–57. doi: 10.1038/nrd3978
111. Sahoo S, Klychko E, Thorne T, Misener S, Schultz KM, Millay M, et al. Exosomes from human CD34+ stem cells mediate their proangiogenic paracrine activity. Circ Res. (2011) 109:724–8. doi: 10.1161/CIRCRESAHA.111.253286
112. Turturici G, Tinnirello R, Sconzo G, Geraci F. Extracellular membrane vesicles as a mechanism of cell-to-cell communication: advantages and disadvantages. Am J Physiol-Cell Ph. (2014) 306:C621–33. doi: 10.1152/ajpcell.00228.2013
113. Gonzalez-King H, Garcia NA, Ontoria-Oviedo I, Ciria M, Montero JA, Sepulveda P. Hypoxia inducible factor-1alpha potentiates jagged 1-mediated angiogenesis by mesenchymal stem cell-derived exosomes. Stem Cells. (2017) 35:1747–59. doi: 10.1002/stem.2618
114. Kang K, Ma R, Cai W, Huang W, Paul C, Liang J, et al. Exosomes secreted from CXCR4 overexpressing mesenchymal stem cells promote cardioprotection via Akt signaling pathway following myocardial infarction. Stem Cells Int. (2015) 2015:1–14. doi: 10.1155/2015/659890
115. Ma J, Zhao Y, Sun L, Sun X, Zhao X, Sun X, et al. Exosomes derived from Akt-modified human umbilical cord mesenchymal stem cells improve cardiac regeneration and promote angiogenesis via activating platelet-derived growth factor D. Stem Cells Transl Med. (2017) 6:51–9. doi: 10.5966/sctm.2016-0038
116. Teng X, Chen L, Chen W, Yang J, Yang Z, Shen Z. Mesenchymal stem cell-derived exosomes improve the microenvironment of infarcted myocardium contributing to angiogenesis and anti-inflammation. Cell Physiol Biochem. (2015) 37:2415–24. doi: 10.1159/000438594
117. Andriolo G, Provasi E, Lo CV, Brambilla A, Soncin S, Torre T, et al. Exosomes from human cardiac progenitor cells for therapeutic applications: development of a GMP-grade manufacturing method. Front Physiol. (2018) 9:1169. doi: 10.3389/fphys.2018.01169
118. Xu S, Wang J, Ding N, Hu W, Zhang X, Wang B, et al. Exosome-mediated microRNA transfer plays a role in radiation-induced bystander effect. Rna Biol. (2015) 12:1355–63. doi: 10.1080/15476286.2015.1100795
119. Zhu LP, Tian T, Wang JY, He JN, Chen T, Pan M, et al. Hypoxia-elicited mesenchymal stem cell-derived exosomes facilitates cardiac repair through miR-125b-mediated prevention of cell death in myocardial infarction. Theranostics. (2018) 8:6163–77. doi: 10.7150/thno.28021
120. Steinhoff G, Nesteruk J, Wolfien M, Kundt G, Börgermann J, David R, et al. Cardiac function improvement and bone marrow response -: outcome analysis of the randomized PERFECT phase III clinical trial of intramyocardial CD133+ application after myocardial infarction. Ebiomedicine. (2017) 22:208–24. doi: 10.1016/j.ebiom.2017.07.022
121. Wöhrle J, Merkle N, Mailänder V, Nusser T, Schauwecker P, von Scheidt F, et al. Results of intracoronary stem cell therapy after acute myocardial infarction. Am J Cardiol. (2010) 105:804–12. doi: 10.1016/j.amjcard.2009.10.060
122. Wöhrle J, von Scheidt F, Schauwecker P, Wiesneth M, Markovic S, Schrezenmeier H, et al. Impact of cell number and microvascular obstruction in patients with bone-marrow derived cell therapy: final results from the randomized, double-blind, placebo controlled intracoronary Stem Cell therapy in patients with Acute Myocardial Infarction (SCAMI) trial. Clin Res Cardiol. (2013) 102:765–70. doi: 10.1007/s00392-013-0595-9
123. Makkar RR, Smith RR, Cheng K, Malliaras K, Thomson LE, Berman D, et al. Intracoronary cardiosphere-derived cells for heart regeneration after myocardial infarction (CADUCEUS): a prospective, randomised phase 1 trial. Lancet. (2012) 379:895–904. doi: 10.1016/S0140-6736(12)60195-0
124. Malliaras K, Makkar RR, Smith RR, Cheng K, Wu E, Bonow RO, et al. Intracoronary cardiosphere-derived cells after myocardial infarction. J Am Coll Cardiol. (2014) 63:110–22. doi: 10.1016/j.jacc.2013.08.724
125. Noiseux N, Mansour S, Weisel R, Stevens L, Der Sarkissian S, Tsang K, et al. The IMPACT-CABG trial: a multicenter, randomized clinical trial of CD133+ stem cell therapy during coronary artery bypass grafting for ischemic cardiomyopathy. J Thorac Cardiovasc Surg. (2016) 152:1582–8. doi: 10.1016/j.jtcvs.2016.07.067
126. Schachinger V, Erbs S, Elsasser A, Haberbosch W, Hambrecht R, Holschermann H, et al. Improved clinical outcome after intracoronary administration of bone-marrow-derived progenitor cells in acute myocardial infarction: final 1-year results of the REPAIR-AMI trial. Eur Heart J. (2006) 27:2775–83. doi: 10.1093/eurheartj/ehl388
127. Schächinger V, Erbs S, Elsässer A, Haberbosch W, Hambrecht R, Hölschermann H, et al. Intracoronary bone marrow–derived progenitor cells in acute myocardial infarction. New Engl J Med. (2006) 355:1210–21. doi: 10.1056/NEJMoa060186
128. Hare JM, Traverse JH, Henry TD, Dib N, Strumpf RK, Schulman SP, et al. A randomized, double-blind, placebo-controlled, dose-escalation study of intravenous adult human mesenchymal stem cells (prochymal) after acute myocardial infarction. J Am Coll Cardiol. (2009) 54:2277–86. doi: 10.1016/j.jacc.2009.06.055
129. Gao LR, Chen Y, Zhang NK, Yang XL, Liu HL, Wang ZG, et al. Intracoronary infusion of Wharton's jelly-derived mesenchymal stem cells in acute myocardial infarction: double-blind, randomized controlled trial. BMC Med. (2015) 13:162. doi: 10.1186/s12916-015-0399-z
130. Chullikana A, Majumdar AS, Gottipamula S, Krishnamurthy S, Kumar AS, Prakash VS, et al. Randomized, double-blind, phase I/II study of intravenous allogeneic mesenchymal stromal cells in acute myocardial infarction. Cytotherapy. (2015) 17:250–61. doi: 10.1016/j.jcyt.2014.10.009
131. Choudry F, Hamshere S, Saunders N, Veerapen J, Bavnbek K, Knight C, et al. A randomized double-blind control study of early intra-coronary autologous bone marrow cell infusion in acute myocardial infarction: the REGENERATE-AMI clinical trialdagger. Eur Heart J. (2016) 37:256–63. doi: 10.1093/eurheartj/ehv493
132. Janssens S, Dubois C, Bogaert J, Theunissen K, Deroose C, Desmet W, et al. Autologous bone marrow-derived stem-cell transfer in patients with ST-segment elevation myocardial infarction: double-blind, randomised controlled trial. Lancet. (2006) 367:113–21. doi: 10.1016/S0140-6736(05)67861-0
133. Hopp E, Lunde K, Solheim S, Aakhus S, Arnesen H, Forfang K, et al. Regional myocardial function after intracoronary bone marrow cell injection in reperfused anterior wall infarction - a cardiovascular magnetic resonance tagging study. J Cardiovasc Magn Reson. (2011) 13:22. doi: 10.1186/1532-429X-13-22
134. Solheim S, Seljeflot I, Lunde K, Bratseth V, Aakhus S, Forfang K, et al. The influence of intracoronary injection of bone marrow cells on prothrombotic markers in patients with acute myocardial infarction. Thromb Res. (2012) 130:765–8. doi: 10.1016/j.thromres.2011.11.045
135. Quyyumi AA, Waller EK, Murrow J, Esteves F, Galt J, Oshinski J, et al. CD34(+) cell infusion after ST elevation myocardial infarction is associated with improved perfusion and is dose dependent. Am Heart J. (2011) 161:98–105. doi: 10.1016/j.ahj.2010.09.025
136. Traverse JH. Effect of intracoronary delivery of autologous bone marrow mononuclear cells 2 to 3 weeks following acute myocardial infarction on left ventricular function. JAMA. (2011) 306:2110. doi: 10.1001/jama.2011.1670
137. Bhatnagar A, Bolli R, Johnstone BH, Traverse JH, Henry TD, Pepine CJ, et al. Bone marrow cell characteristics associated with patient profile and cardiac performance outcomes in the LateTIME-Cardiovascular Cell Therapy Research Network (CCTRN) trial. Am Heart J. (2016) 179:142–50. doi: 10.1016/j.ahj.2016.06.018
138. Cogle CR, Wise E, Meacham AM, Zierold C, Traverse JH, Henry TD, et al. Detailed analysis of bone marrow from patients with ischemic heart disease and left ventricular dysfunction. Circ Res. (2014) 115:867–74. doi: 10.1161/CIRCRESAHA.115.304353
139. Traverse JH, Henry TD, Pepine CJ, Willerson JT, Zhao DXM, Ellis SG, et al. Effect of the use and timing of bone marrow mononuclear cell delivery on left ventricular function after acute myocardial infarction. JAMA. (2012) 308:2380–9. doi: 10.1001/jama.2012.28726
140. Traverse JH, Henry TD, Pepine CJ, Willerson JT, Ellis SG. One-year follow-up of intracoronary stem cell delivery on left ventricular function following ST-elevation myocardial infarction. JAMA. (2014) 311:301. doi: 10.1001/jama.2013.282674
141. Sürder D, Manka R, Lo Cicero V, Moccetti T, Rufibach K, Soncin S, et al. Intracoronary injection of bone marrow–derived mononuclear cells early or late after acute myocardial infarction. Circulation. (2013) 127:1968–79. doi: 10.1161/CIRCULATIONAHA.112.001035
142. Sürder D, Manka R, Moccetti T, Lo Cicero V, Emmert MY, Klersy C, et al. Effect of bone marrow–derived mononuclear cell treatment, early or late after acute myocardial infarction. Circ Res. (2016) 119:481–90. doi: 10.1161/CIRCRESAHA.116.308639
143. Naseri MH, Madani H, Ahmadi TS, Moshkani FM, Kazemi SD, Hosseinnejad H, et al. COMPARE CPM-RMI trial: intramyocardial transplantation of autologous bone marrow-derived CD133+ cells and MNCs during CABG in patients with recent MI: a phase II/III, multicenter, placebo-controlled, randomized, double-blind clinical trial. Cell J. (2018) 20:267–77.
144. Traverse JH, McKenna DH, Harvey K, Jorgenso BC, Olson RE, Bostrom N, et al. Results of a phase 1, randomized, double-blind, placebo-controlled trial of bone marrow mononuclear stem cell administration in patients following ST-elevation myocardial infarction. Am Heart J. (2010) 160:428–34. doi: 10.1016/j.ahj.2010.06.009
145. Fernández-Avilés F, Sanz-Ruiz R, Bogaert J, Casado Plasencia A, Gilaberte I, Belmans A, et al. Safety and efficacy of intracoronary infusion of allogeneic human cardiac stem cells in patients with ST-segment elevation myocardial infarction and left ventricular dysfunction. Circ Res. (2018) 123:579–89. doi: 10.1161/CIRCRESAHA.118.312823
146. Hu S, Liu S, Zheng Z, Yuan X, Li L, Lu M, et al. Isolated coronary artery bypass graft combined with bone marrow mononuclear cells delivered through a graft vessel for patients with previous myocardial infarction and chronic heart failure. J Am Coll Cardiol. (2011) 57:2409–15. doi: 10.1016/j.jacc.2011.01.037
147. Pätilä T, Lehtinen M, Vento A, Schildt J, Sinisalo J, Laine M, et al. Autologous bone marrow mononuclear cell transplantation in ischemic heart failure: a prospective, controlled, randomized, double-blind study of cell transplantation combined with coronary bypass. J Heart Lung Transplant. (2014) 33:567–74. doi: 10.1016/j.healun.2014.02.009
148. Lehtinen M, Pätilä T, Vento A, Kankuri E, Suojaranta-Ylinen R, Pöyhiä R, et al. Prospective, randomized, double-blinded trial of bone marrow cell transplantation combined with coronary surgery — perioperative safety study. Interact Cardiov Thorac. (2014) 19:990–6. doi: 10.1093/icvts/ivu265
149. Huikuri HV, Kervinen K, Niemelä M, Ylitalo K, Säily M, Koistinen P, et al. Effects of intracoronary injection of mononuclear bone marrow cells on left ventricular function, arrhythmia risk profile, and restenosis after thrombolytic therapy of acute myocardial infarction. Eur Heart J. (2008) 29:2723–32. doi: 10.1093/eurheartj/ehn436
150. Miettinen JA, Ylitalo K, Hedberg P, Jokelainen J, Kervinen K, Niemela M, et al. Determinants of functional recovery after myocardial infarction of patients treated with bone marrow-derived stem cells after thrombolytic therapy. Heart. (2010) 96:362–7. doi: 10.1136/hrt.2009.171694
151. Quyyumi AA, Vasquez A, Kereiakes DJ, Klapholz M, Schaer GL, Abdel-Latif A, et al. PreSERVE-AMI: a randomized, double-blind, placebo-controlled clinical trial of intracoronary administration of autologous CD34+ cells in patients with left ventricular dysfunction post STEMI. Circ Res. (2017) 120:324–31. doi: 10.1161/CIRCRESAHA.115.308165
152. Assmus B, Honold J, Schachinger V, Britten MB, Fischer-Rasokat U, Lehmann R, et al. Transcoronary transplantation of progenitor cells after myocardial infarction. N Engl J Med. (2006) 355:1222–32. doi: 10.1056/NEJMoa051779
153. Hu X, Huang X, Yang Q, Wang L, Sun J, Zhan H, et al. Safety and efficacy of intracoronary hypoxia-preconditioned bone marrow mononuclear cell administration for acute myocardial infarction patients: the CHINA-AMI randomized controlled trial. Int J Cardiol. (2015) 184:446–51. doi: 10.1016/j.ijcard.2015.02.084
154. Guijarro D, Lebrin M, Lairez O, Bourin P, Piriou N, Pozzo J, et al. Intramyocardial transplantation of mesenchymal stromal cells for chronic myocardial ischemia and impaired left ventricular function: results of the MESAMI 1 pilot trial. Int J Cardiol. (2016) 209:258–65. doi: 10.1016/j.ijcard.2016.02.016
155. Hare JM, Fishman JE, Gerstenblith G, DiFede Velazquez DL, Zambrano JP, Suncion VY, et al. Comparison of allogeneic vs autologous bone marrow–derived mesenchymal stem cells delivered by transendocardial injection in patients with ischemic cardiomyopathy. JAMA. (2012) 308:2369. doi: 10.1001/jama.2012.25321
156. Yan W, Lin C, Guo Y, Chen Y, Du Y, Lau WB, et al. N-Cadherin overexpression mobilizes the protective effects of mesenchymal stromal cells against ischemic heart injury through A Beta-catenin dependent manner. Circ Res. (2020) 126:857–74. doi: 10.1161/CIRCRESAHA.119.315806
157. Zhang Z, Tian H, Yang C, Liu J, Zhang H, Wang J, et al. Mesenchymal stem cells promote the resolution of cardiac inflammation after ischemia reperfusion via enhancing efferocytosis of neutrophils. J Am Heart Assoc. (2020) 9:e14397. doi: 10.1161/JAHA.119.014397
158. Hobby A, Sharp TR, Berretta RM, Borghetti G, Feldsott E, Mohsin S, et al. Cortical bone-derived stem cell therapy reduces apoptosis after myocardial infarction. Am J Physiol Heart Circ Physiol. (2019) 317:H820–9. doi: 10.1152/ajpheart.00144.2019
159. Sharp TE, Schena GJ, Hobby AR, Starosta T, Berretta RM, Wallner M, et al. Cortical bone stem cell therapy preserves cardiac structure and function after myocardial infarction. Circ Res. (2017) 121:1263–78. doi: 10.1161/CIRCRESAHA.117.311174
160. Gong XH, Liu H, Wang SJ, Liang SW, Wang GG. Exosomes derived from SDF1-overexpressing mesenchymal stem cells inhibit ischemic myocardial cell apoptosis and promote cardiac endothelial microvascular regeneration in mice with myocardial infarction. J Cell Physiol. (2019) 234:13878–93. doi: 10.1002/jcp.28070
161. Xiao C, Wang K, Xu Y, Hu H, Zhang N, Wang Y, et al. Transplanted mesenchymal stem cells reduce autophagic flux in infarcted hearts via the exosomal transfer of miR-125b. Circ Res. (2018) 123:564–78. doi: 10.1161/CIRCRESAHA.118.312758
162. Natsumeda M, Florea V, Rieger AC, Tompkins BA, Banerjee MN, Golpanian S, et al. A combination of allogeneic stem cells promotes cardiac regeneration. J Am Coll Cardiol. (2017) 70:2504–15. doi: 10.1016/j.jacc.2017.09.036
163. Bobi J, Solanes N, Fernández Jiménez R, Galán Arriola C, Dantas AP, Fernández Friera L, et al. Intracoronary administration of allogeneic adipose tissue–derived mesenchymal stem cells improves myocardial perfusion but not left ventricle function, in a translational model of acute myocardial infarction. J Am Heart Assoc. (2017) 6:e005771. doi: 10.1161/JAHA.117.005771
164. Liu X, Hu D, Zeng Z, Zhu W, Zhang N, Yu H, et al. SRT1720 promotes survival of aged human mesenchymal stem cells via FAIM: a pharmacological strategy to improve stem cell-based therapy for rat myocardial infarction. Cell Death Dis. (2017) 8:e2731. doi: 10.1038/cddis.2017.107
165. Luo L, Tang J, Nishi K, Yan C, Dinh P, Cores J, et al. Fabrication of synthetic mesenchymal stem cells for the treatment of acute myocardial infarction in mice. Circ Res. (2017) 120:1768–75. doi: 10.1161/CIRCRESAHA.116.310374
166. Liu G, Li L, Huo D, Li Y, Wu Y, Zeng L, et al. A VEGF delivery system targeting MI improves angiogenesis and cardiac function based on the tropism of MSCs and layer-by-layer self-assembly. Biomaterials. (2017) 127:117–31. doi: 10.1016/j.biomaterials.2017.03.001
167. Gómez-Mauricio G, Moscoso I, Martín-Cancho M, Crisóstomo V, Prat-Vidal C, Báez-Díaz C, et al. Combined administration of mesenchymal stem cells overexpressing IGF-1 and HGF enhances neovascularization but moderately improves cardiac regeneration in a porcine model. Stem Cell Res Ther. (2016) 7:94. doi: 10.1186/s13287-016-0350-z
168. Hnatiuk AP, Ong SG, Olea FD, Locatelli P, Riegler J, Lee WH, et al. Allogeneic mesenchymal stromal cells overexpressing mutant human hypoxia-inducible factor 1-α (HIF1-α) in an ovine model of acute myocardial infarction. J Am Heart Assoc. (2016) 5:e003714. doi: 10.1161/JAHA.116.003714
169. Liu C, Huang H, Sun P, Ma S, Liu A, Xue J, et al. Human umbilical cord-derived mesenchymal stromal cells improve left ventricular function, perfusion, and remodeling in a porcine model of chronic myocardial ischemia. Stem Cell Transl Med. (2016) 5:1004–13. doi: 10.5966/sctm.2015-0298
170. Hu X, Xu Y, Zhong Z, Wu Y, Zhao J, Wang Y, et al. A large-scale investigation of hypoxia-preconditioned allogeneic mesenchymal stem cells for myocardial repair in nonhuman primates. Circ Res. (2016) 118:970–83. doi: 10.1161/CIRCRESAHA.115.307516
171. de Jong R, Houtgraaf JH, Samiei S, Boersma E, Duckers HJ. Intracoronary stem cell infusion after acute myocardial infarction: a meta-analysis and update on clinical trials. Circ Cardiovasc Interv. (2014) 7:156–67. doi: 10.1161/CIRCINTERVENTIONS.113.001009
172. Liu B, Duan C, Luo C, Ou C, Sun K, Wu Z, et al. Effectiveness and safety of selected bone marrow stem cells on left ventricular function in patients with acute myocardial infarction: A meta-analysis of randomized controlled trials. Int J Cardiol. (2014) 177:764–70. doi: 10.1016/j.ijcard.2014.11.005
173. Xu J, Liu D, Zhong Y, Huang R. Effects of timing on intracoronary autologous bone marrow-derived cell transplantation in acute myocardial infarction: a meta-analysis of randomized controlled trials. Stem Cell Res Ther. (2017) 8:231. doi: 10.1186/s13287-017-0680-5
174. Li S, Tang D, Xue Z, Zhang Z, Sun X, Liu Y, et al. Biphasic effect of EGb761 on simulated ischemia-induced rat BMSC survival in vitro and in vivo. Life Sci. (2011) 88:853–63. doi: 10.1016/j.lfs.2011.03.002
175. Tong Y, Xu W, Han H, Chen Y, Yang J, Qiao H, et al. Tanshinone IIA increases recruitment of bone marrow mesenchymal stem cells to infarct region via up-regulating stromal cell-derived factor-1/CXC chemokine receptor 4 axis in a myocardial ischemia model. Phytomedicine. (2011) 18:443–50. doi: 10.1016/j.phymed.2010.10.009
176. Jaumdally RJ, Goon PK, Varma C, Blann AD, Lip GY. Effects of atorvastatin on circulating CD34+/CD133+/ CD45- progenitor cells and indices of angiogenesis (vascular endothelial growth factor and the angiopoietins 1 and 2) in atherosclerotic vascular disease and diabetes mellitus. J Intern Med. (2010) 267:385–93. doi: 10.1111/j.1365-2796.2009.02151.x
177. Yamada Y, Takeuchi S, Yoneda M, Ito S, Sano Y, Nagasawa K, et al. Atorvastatin reduces cardiac and adipose tissue inflammation in rats with metabolic syndrome. Int J Cardiol. (2017) 240:332–8. doi: 10.1016/j.ijcard.2017.04.103
178. Xu H, Yang YJ, Qian HY, Tang YD, Wang H, Zhang Q. Rosuvastatin treatment activates JAK-STAT pathway and increases efficacy of allogeneic mesenchymal stem cell transplantation in infarcted hearts. Circ J. (2011) 75:1476–85. doi: 10.1253/circj.CJ-10-1275
179. Cai A, Zheng D, Dong Y, Qiu R, Huang Y, Song Y, et al. Efficacy of Atorvastatin combined with adipose-derived mesenchymal stem cell transplantation on cardiac function in rats with acute myocardial infarction. Acta Biochim Biophys Sin. (2011) 43:857–66. doi: 10.1093/abbs/gmr087
180. de Jong R, van Hout GP, Houtgraaf JH, Kazemi K, Wallrapp C, Lewis A, et al. Intracoronary infusion of encapsulated glucagon-like peptide-1-eluting mesenchymal stem cells preserves left ventricular function in a porcine model of acute myocardial infarction. Circ Cardiovasc Interv. (2014) 7:673–83. doi: 10.1161/CIRCINTERVENTIONS.114.001580
181. Montzka K, Fuhrmann T, Muller-Ehmsen J, Woltje M, Brook GA. Growth factor and cytokine expression of human mesenchymal stromal cells is not altered in an in vitro model of tissue damage. Cytotherapy. (2010) 12:870–80. doi: 10.3109/14653249.2010.501789
182. Yao Y, Zhang F, Wang L, Zhang G, Wang Z, Chen J, et al. Lipopolysaccharide preconditioning enhances the efficacy of mesenchymal stem cells transplantation in a rat model of acute myocardial infarction. J Biomed Sci. (2009) 16:74. doi: 10.1186/1423-0127-16-74
183. Liu X, Hou J, Shi L, Chen J, Sang J, Hu S, et al. Lysophosphatidic acid protects mesenchymal stem cells against ischemia-induced apoptosis in vivo. Stem Cells Dev. (2009) 18:947–54. doi: 10.1089/scd.2008.0352
Keywords: mesenchymal stem cells, ischemic heart disease, neovascularization, angiogenesis, vasculogenesis
Citation: Shi W, Xin Q, Yuan R, Yuan Y, Cong W and Chen K (2021) Neovascularization: The Main Mechanism of MSCs in Ischemic Heart Disease Therapy. Front. Cardiovasc. Med. 8:633300. doi: 10.3389/fcvm.2021.633300
Received: 25 November 2020; Accepted: 05 January 2021;
Published: 26 January 2021.
Edited by:
Sarawut Kumphune, Chiang Mai University, ThailandReviewed by:
Junjie Yang, University of Alabama at Birmingham, United StatesCopyright © 2021 Shi, Xin, Yuan, Yuan, Cong and Chen. This is an open-access article distributed under the terms of the Creative Commons Attribution License (CC BY). The use, distribution or reproduction in other forums is permitted, provided the original author(s) and the copyright owner(s) are credited and that the original publication in this journal is cited, in accordance with accepted academic practice. No use, distribution or reproduction is permitted which does not comply with these terms.
*Correspondence: Weihong Cong, Y29uZ2Nhb0AxODguY29t
Disclaimer: All claims expressed in this article are solely those of the authors and do not necessarily represent those of their affiliated organizations, or those of the publisher, the editors and the reviewers. Any product that may be evaluated in this article or claim that may be made by its manufacturer is not guaranteed or endorsed by the publisher.
Research integrity at Frontiers
Learn more about the work of our research integrity team to safeguard the quality of each article we publish.