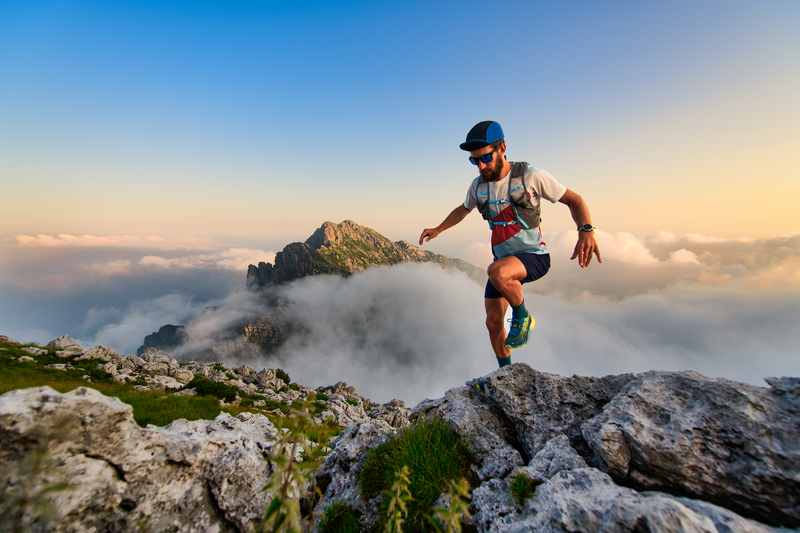
95% of researchers rate our articles as excellent or good
Learn more about the work of our research integrity team to safeguard the quality of each article we publish.
Find out more
MINI REVIEW article
Front. Cardiovasc. Med. , 31 March 2020
Sec. Atherosclerosis and Vascular Medicine
Volume 7 - 2020 | https://doi.org/10.3389/fcvm.2020.00039
High Density Lipoproteins (HDLs) have long been considered as “good cholesterol,” beneficial to the whole body and, in particular, to cardio-vascular health. However, HDLs are complex particles that undergoes dynamic remodeling through interactions with various enzymes and tissues throughout their life cycle, making the complete understanding of its functions and roles more complicated than initially expected. In this review, we explore the novel understanding of HDLs' behavior in health and disease as a multifaceted class of lipoprotein, with different size subclasses, molecular composition, receptor interactions, and functionality. Further, we report on emergent HDL-based therapeutics tested in small and larger scale clinical trials and their mixed successes.
The term “good cholesterol” is often used with reference to the cholesterol content (HDL-cholesterol) in high-density lipoproteins (HDLs). The 1980's Framingham study found a strong positive association between coronary heart disease and low HDL-C levels (1). Thus, approaches were developed to increase HDL-C and achieve cardioprotection (2, 3). Notably, the ILLUMINATE Phase 3 trial, using the drug torcetrapib, increased HDL-C content significantly through inhibition of cholesteryl ester transfer protein (CETPi), which normally catalyzes the transfer of cholesterol from HDL to low density lipoproteins (LDL), and triglycerides from LDL to HDL (see Figure 1A). However, the trial was prematurely terminated as patients on torcetrapib showed higher risk of death and adverse cardiovascular events than the control group on atorvastatin (4). Other clinical trials testing different CETPi yielded similarly disappointing results, where increasing HDL-C resulted in no (5, 6) or marginal improvement in the cardiovascular end-points (7), either myocardial infarction or mortality (8). Anacetrapib was the only CETPi to show modest reduction of major cardiovascular events over a follow-up period of 4 years in the REVEAL trial (7), however the achieved benefits seem more attributable to the concomitant decrease in LDL-C, than to the HDL-C raising effects of the drug (9). Pharmacogenetic interactions driven by still unknown genetic variants in the population may have confounded the CETPi trial results, although there is no clear evidence to date (10). Beyond the CETPi trial results, the fact that HDL-C per se is not causally associated with cardiovascular benefits was supported by Mendelian randomization studies, demonstrating that genetic polymorphisms associated with increased HDL-C had no impact on the risk of myocardial infarction (11, 12). Evidence coming also from meta-analysis could not find an improvement in cardiovascular outcome after raising HDL-C levels (13). Interestingly, what was proven to be inversely associated with cardiovascular risk (14) was the pivotal biological function of HDL, known as reverse cholesterol transport (RCT), whereby HDL accepts excessive cholesterol from macrophages in peripheral tissues and carries it to the liver for disposal. Overall, these results ushered in a new era of research on HDL, focusing more on quality of whole HDL, rather than merely cholesterol content. HDLs are a family of particles that can exhibit fundamentally different metabolism and functions based on their specific proteomic, lipidomic, and physico-chemical properties. Further, HDLs carry various proteins, enzymes, miRNAs, bile acids, and lipids, which all have a potential functional role. HDLs are dynamic particles, being either protective or deleterious agents in health or disease. Today's research aims to gain new understanding of HDLs to develop novel therapies and treatments for cardiovascular diseases. This review focuses on the relationship between structure and function as a multifaceted determinant of the complexity of HDLs. Moreover, attention is paid to future perspectives about HDL as a potential vehicle for drug delivery and therapeutic agent against cardiovascular atherosclerotic disease.
Figure 1. (A) HDL Lifecycle. Diagram detailing the three key stages of the HDL lifecycle. (1) Synthesis: ApoA1 is synthesized in the liver and the gut, where it can be gradually lipidated on-site or by the adipose tissue to produce pre-ß HDLs. Further lipidation results in mature HDL formation, which can in-turn become pre-ß HDL via the catabolic action of endothelial (EL) and hepatic (HL) lipases. (2) Function: HDLs main function are to efflux cholesterol and other lipids from peripheral tissues (such as the cardio-vascular system) and transport them either to (a) the liver for disposal, (b) steroidogenic tissues to support hormone production or (c) exchange lipids with apoB-containing particles. (3) Catabolism: finally, after a roughly 4 to 5 day lifecycle, HDLs are permanently catabolized either in the liver via the ecto-F1-ATPase or through complete delipidation by SR-B1 in the kidney and urinary excretion. (B) Diagram detailing the various actions of HDLs in health and disease. Healthy HDLs have a high PL content and are highly associated to beneficial molecules, such as S1P and PON-1 enzyme exerting a beneficial role on ECs, or anti-atherosclerotic miRNA 223. Throughout the pathogenesis of cardiovascular disease, HDLs becomes progressively more dysfunctional. The lipidome and proteome of HDLs are altered, with increased TG and decreased PL. SAA and SDA are become associated to HDL. Dysfunctional HDLs also present an altered miRNA profile, with increase in pro-inflammatory miRNA 24. Metabolic interventions have been shown to improve HDL functionality. RYGB, exercise, and diet restore HDL functionality and alter composition to varying degrees. SAA, Serum Amyloid A; SDA, Symmetric Dimethylarginine.
The backbone of HDL is apolipoprotein A1 (apoA1), which is synthesized via forkhead box protein A3 (15) in the liver and in the intestine. Then ApoA1 is lipidated by ABCA1-mediated cholesterol efflux to form nascent discoidal pre-β HDLs. Lipidation, as well as the conversion of free cholesterol to cholesterol esters, drives the formation of mature spherical α-HDL (16). Mature HDL undergoes constant dynamic remodeling in its 4 to 5 day lifecycle through interactions with a variety of enzymes, such as hepatic and endothelial lipase, generating smaller subspecies (e.g., pre-β HDL) from larger ones (e.g., α-HDL) (16). The dynamic remodeling of HDLs can now be visualized in-vivo using fluorescent probes (17). Of note, HDLs play an important role in uptake from the gut and transport into the systemic circulation of antioxidants, such as carotenoids and vitamins of dietary origin (18). Indeed, HDL structure and lipidome are modified post-prandially and in relation to the magnitude of post-prandial triglyceridemia HDL may acquire larger size and a triglyceride rich phenotype (19). Along this evidence, it has been suggested that non-fasting HDL concentrations may be more appropriate predictors of cardiovascular events than fasting levels (20, 21). The underlying biological explanation is still unclear as for instance, a major HDL antioxidant enzyme, paraoxonase-1 (PON-1) activity may not decrease along the postprandial stage (19). The post-prandial metabolism of HDL is still poorly detailed and would benefit from additional investigations in larger groups of individuals in health and disease conditions.
As previously mentioned, the main function of HDL is to scavenge excess cholesterol through RCT and shuttle it to the liver, to organs with high-cholesterol requirements or exchange it with apoB particles (e.g., LDL) (16) for disposal. HDLs deliver cholesterol to the liver and steroidogenic tissues through binding its receptor scavenger receptor B1 (SR-B1), which functions in stable multimers in the plasma membrane for binding HDLs (22). HDLs also interact with ATP-dependent transmembrane transporter proteins, ABCA1 and ABCG1 (23) expressed in macrophages, adipose tissue, gut and liver at high levels (24, 25) for cholesterol delivery.
HDL holoparticles are endocytosed into their target cell types by CD36 and potentially SR-B1 (26, 27), where they may accumulate in the cell or be rapidly retro-endocytosed through yet unknown mechanisms (28). HDLs also enter target cells through micropinocytosis in the lymphatic system (29) or via clathrin-coated pits in a receptor-independent manner in endothelial cells (30). Finally, HDLs undergo transcytosis through polarized cells, mediated by SR-B1 in hepatocytes and interactions between SR-B1 and vascular endothelial growth factor receptor 2 (VEGFR2) in endothelial cells (31).
The liver is the major organ responsible for HDL clearance through the canonical ecto-F1-ATPase/PY213 pathway, wherein upregulation of its components increases HDL clearance from the circulation (16). De-lipidated apoA1-particles are cleared, preferentially by the kidneys, through selective SR-B1 uptake (16). Recent evidence suggests that HDLs can integrate into the lipid bilayer of cells (32). Whether this mechanism is permanent or transient is unknown, but it could prove to be a novel method of HDL clearance. Figure 1A summarizes the HDL lifecycle in its key components.
HDLs are complex particles, which can be separated into several subclasses based on their differing physicochemical properties (33). There is no consensus regarding the definitive categories of HDL subclasses or exactly how to define them, which, combined with the various methods of HDL isolation (33) (Supplementary Table 1), hampers our understanding and ability to investigate HDL biology and role in vascular and metabolic disease. However, considerable efforts were made to classify HDLs in a systematic way. Experts ranging from basic science to clinical practice have devised a five-part sub-classification for HDLs, which encompasses all aforementioned properties: very large HDL, large HDL, medium HDL, small HDL and very small HDL (34). Although it has not replaced the previously detailed, heterogenous classification system, it can be a useful clinical tool. The function and metabolism of HDLs can be influenced by the subclass it belongs to (33, 35), and the ability to distinguish between HDL-subclasses may be both clinically relevant (36) and a reason for statin-therapy success (37). With new gold-standard techniques of classification, such as nuclear magnetic resonance (NMR) (38) which has the advantage of measuring HDL classes from whole plasma without preliminary isolation, major efforts are now focusing on elucidating the complex lipidome (39), proteome (40), and structural subtleties of HDL particles and subclasses (41). We recommend that further clinical studies should establish reference values for the technique adopted, in particular NMR, and should assess whether integration of HDL subclasses measurements and parameters of HDL functionality with patient-specific biomarkers can enhance the stratification of patients for differential diagnosis, disease progression and responses to therapy.
The diverse protein and lipid composition of HDL contribute to its atheroprotective function (41). In the vessel wall, HDL undergoes transcytosis through endothelial cells into the sub-endothelial space, where it can efflux cholesterol from foam cells (cholesterol-loaded macrophages), preventing plaque formation. Receptors mediating RCT vary between HDL subtypes, with small pre-β HDL having greater affinity for ABCA1-dependent cholesterol export and α-HDL for ABCG1 (42). Beyond RCT, HDLs have several other beneficial properties, such as anti-oxidant capacity, nitric oxide (NO) production stimulation, anti-inflammatory (i.e., anti-vascular adhesion molecule-1 expression) and anti-apoptotic actions (43). One of the most important properties of HDL is its ability to induce NO-production in endothelial cells, through activation of surface receptors, such as SR-B1 (44) and S1P3R (45), and intracellular signaling cascades, involving Akt, PI3K, and MAPK (46), converging, in-part, on endothelial nitric oxide synthase (eNOS). HDL may also act to stabilize eNOS away from catabolism (47). In atherosclerotic coronary artery disease patients, larger HDL particles have a less anti-oxidative capacity than smaller, denser ones (48), which could be explained by an altered proteome. Larger HDL particles are correlated to apolipoprotein A2, which has been shown to decrease the association between HDL and PON-1, an HDL-bound detoxifying enzyme, by displacing it in a broadly concentration-dependent manner (49). Further, small, dense HDL3 have a more potent anti-inflammatory effect than larger HDL2, demonstrated by their highly effective ability to inhibit TNF-α induced VCAM-1 expression in an in-vitro endothelial cell model. Here, proteomic modifications were not responsible, as the artificial substitution of apolipoprotein 1 by apolipoprotein 2 in HDL3 did not alter the beneficial anti-inflammatory profile (50). Interestingly, increasing evidence seems to point to a disease-specific HDL-size function relationship, while smaller HDLs seem to protect against atherosclerosis (51), in dysmetabolic diseases, like Type 2 Diabetes Mellitus (T2DM), larger HDLs seem beneficial (52), potentially due to improved RCT function or a different molecular composition.
The lipidome of HDL has been demonstrated to have functional properties (39). In healthy conditions, phospholipids (PL) are the dominant HDL lipid component (up to 50% of HDL lipids) and seem to stabilize the particle (53). A composition shift toward phosphatidylcholine promotes cholesterol efflux, while an increase in sphingomyelin decreases influx of cholesterol via SR-B1 (54). Most recently, the sphingosine-1-phosphate receptors (S1PR) have garnered increasing attention as an HDL target receptor, since 50 to 70% of plasmatic S1P is carried by HDL particles. The activation of S1PR1 and S1PR3 by HDL has protective effects on endothelial cells, reducing inflammation and apoptosis (55). Specifically, S1P enrichment of HDL inhibits oxidized low-density lipoprotein induced apoptosis and increases NO production (56). In-vitro, apolipoprotein M, a component of HDL, seems to facilitate the interaction between S1P-HDL and its receptor (57). Others report that there is crosstalk between SR-B1 and S1PR following activation by HDL particles, which would potentiate signaling efficiency. Finally, HDLs are an effective carrier of circulating microRNA (miRNA) to target cells (58), with miRNA potentially being important in stabilizing HDL (59). The miRNA function of miR-223 and miR-24 are best characterized, with miR-223 conferring a beneficial anti-inflammatory profile (60), while miR-24 may be atherogenic (61). As with HDL function, proteome and lipidome composition, the miRNA profile of HDL is altered in pathological conditions (62).
Disease states can cause HDL dysfunction as visualized in Figure 1B. In 2011, Besler et al. showed that HDLs isolated from patients with chronic coronary disease and acute coronary syndrome were significantly less able to stimulate NO production in-vitro, and exerted pro-oxidative and pro-inflammatory actions (43). Recently, the strong association with acute coronary syndrome (63) has been further extended to low cholesterol efflux capacity values and low HDL levels of S1P and apoA1.
In chronic kidney disease, the increased association of symmetric dimethylarginine to HDL alters HDL functionality and directly leads to the development of cardiovascular disease, as it impairs HDL RCT capacity and decreases its anti-inflammatory properties (64). HDLs from patients with valvular heart disease, including rheumatic heart disease, HDLs are pro-inflammatory and uncouple eNOS, which in turn impairs endothelial ability to produce NO (65). Similarly, HDLs from patients with T2DM impair NO production and are pro-inflammatory (66). Alterations in the lipidome, such as increase in triglycerides or decrease in phospholipids (67, 68), or a concomitant increase in surface rigidity due to an altered sphingomyelin to cholesterol ratio, reduce the RCT ability of HDLs, and its ability to associate to beneficial enzymes and proteins (69). Recent studies suggest that HDL-triglycerides measurement may be a useful biomarker to determine HDL quality and HDL function over HDL-C (70). While it has been widely accepted that oxidation and glycation of HDLs are a major driver of HDL dysfunction in-vivo (71, 72), a few studies challenge this view, finding either no dysfunction (73) or improved function (24, 74) following either endogenous or artificial oxidation of HDL.
Lipid composition, size and structure of HDLs are closely linked. In T2DM patients, several studies show that there is a shift toward smaller HDL particles, and an increase in triglyceride presence on HDL (75), which may render them more hydrophobic and therefore challenging the idea that small HDL is always protective, but rather suggesting a close interplay between HDL size-composition-function and each specific disease condition. HDLs are direct players of whole-body glucose homeostasis (76), through activating AMPK-dependent glucose uptake (77), increasing insulin secretion (78), and protecting pancreatic ß-cells from apoptosis (79). Thus, T2DM may influence HDL function, and HDL function may in turn influence T2DM pathogenesis. However, to date we do not yet have clear evidence about the functional consequences of all structural alterations, which may contribute to the dysfunction of HDLs in T2DM. Moreover, macrophage-associated enzyme myeloperoxidase, which is increased in atherosclerotic cardiovascular disease, can catalyze deleterious changes to HDL associated proteins, namely apoA1, causing an impaired RCT ability and increase in inflammatory pathways (51). Serum amyloid A is a causal factor of HDL dysfunction, inducing a loss of anti-inflammatory and RCT function and a decreased ability of HDLs to interact with the plasma membrane of adipocytes (80). Beyond the above described roles of HDL, there are additional key roles of HDL in immunity (81, 82), Alzheimer's prevention (83), and even cancer survival (84) as mentioned in Table 1, which could not be covered in this mini-review.
Recent data points to high levels of HDL-C as potentially deleterious to cardio-vascular health, showing a distinct U-shape association between HDL-C above 100 mg.dL−1 and disease risk (91, 92) in men. Raised HDL-C may increase disease risk for several reasons, including potential undisclosed confounders. Genetic mutations causing elevated HDL may also be a risk factor for disease, a potential reverse causation arising from the severity of disease in the studied at-risk population, or the possibility that HDLs becomes dysfunctional at such elevated circulating levels. The cut-off for pathologically high HDL is not clearly defined, but has tentatively been placed as HDL-C levels ranging from 60 to 80 mg.dL−1. A recent cross-sectional study determined that in men, and after adjusting for cardiovascular risk factors, extremely high HDL-C was associated to endothelial dysfunction, as measured in-vivo by flow mediated vasodilation (FMD), while low HDL-C was not (93). Less than 10% (93) of the population present extremely high HDL-C levels, but this feature is more frequent in Type 1 Diabetes Mellitus (T1DM) (94). HDLs isolated from young T1DM patients are dysfunctional, less able to induce NO production by endothelial cells and pro-oxidant. Further, T1DM patients with extremely high HDL levels and inflammation have a substantially decreased FMD (94), suggesting that high levels of HDL associated to systemic inflammation, as found in several cardiovascular and metabolic disease, may be a driver of vascular dysfunction and not merely a reflection of an overall pathological state.
Recovery from metabolic and cardiovascular disease parallels restored HDL functionality and increased HDL concentration (95). Roux-en-Y gastric bypass (RYGB) is a bariatric surgery able to decrease cardio-vascular mortality (96) and resolve T2DM in a rapid and body weight-independent manner (97). We have demonstrated in both humans and rodent models that RYGB promotes an early improvement of HDL function, including cholesterol efflux capacity, anti-apoptotic, anti-oxidant and anti-inflammatory activity, and increased capacity to produce NO (98). BMI-matched controls to the 12 week post-surgery patient group did show impaired HDL function, demonstrating that post-surgical improvements in HDL function occurred in a body weight-independent manner (98). Evidence from follow-up studies indicates that the restoration of HDL function is stable long term after bariatric surgery. Interestingly, evidence shows that HDLs tend to be larger post-RYGB, further increasing the complexity of HDL-size-composition-function relationship discussed above (99).
Exercise and diet also improve HDL function. In chronic heart failure patients, a 15 week exercise intervention significantly improved the ability of HDLs to activate eNOS and produce NO (100). One study shows that HDLs isolated before and after an exercise-based weight loss intervention showed significant correlation between RCT and amount of weight lost (101), and HDL levels significantly increase post-exercise training across different studies (101–103). While RYGB seems to acts via additional mechanisms (Figure 1B) (98), body weight loss has beneficial effects on HDLs, leading for instance to increased HDL2 particle number after dieting (103), to improved efflux-capacity (104) and altered miR223 expression (105). Further, increases in brown fat metabolism, which is impaired in obese subjects, correlates to beneficial HDL remodeling, in both humans and mouse models (106).
Manipulation of HDL components have beneficial effects. Enrichment of S1P to reconstituted HDL (rHDL) induce better vasorelaxation than control rHDL (107). In humans, a small trial found that short-term infusion (4 weeks, 1 infusion per week) of rHDL was able to significantly decrease endothelial progenitor cell (EPC) apoptosis in patients with acute coronary dysfunction, and increase the circulating chemokine levels known to be important in EPC recruitment, such as stromal cell-derived factor-1 or vascular endothelial growth factor (108). Another small-scale human trial found that rHDL infusion resulted in decreased plaque lipid content and decreased expression of VCAM-1 on the plaque surface (109). Preliminary results from a larger clinical trial found that while plaque size per se had not regressed following rHDL infusion, there was a significant improvement in the plaque characterization index and overall coronary score (110). Furthermore, increasing apoA1 levels alone, either through genetic manipulation in animal models (111) or through exogenous infusion in animals (112) and humans (113), was enough to provide beneficial effects on atherosclerotic plaque regression (114). For certain parameters HDL still outperformed apoA1 mimetic alone (115). Animal studies show that raising an apoA1 and functional HDL can promote atherosclerosis plaque regression through inhibition of inflammation and decreased activation of immune cells (116). Larger trials paint a more controversial story, showing no detectable effect after supplementation of an engineered pre-β HDL mimetic on atherosclerotic plaque composition or regression compared to placebo (117).
For over 10 years, rHDLs have been used in research for treatment delivery (118). The delivery to organs of interest is efficient and the cargo is protected from degradation. While conjugations of HDLs have mostly been used to target the liver, where SR-B1 expression is high, it has been found that the addition of folic acid to HDLs expands the target organ pool to cells expressing the folate receptor (119). The current understanding of how to encapsulate vaso-protective compounds within rHDL allows us to consider using it a treatment (120). The infusion of rHDL loaded with a potent LXR agonist enabled atherosclerotic plaque regression in the apoE-knock out mouse model, with significant accumulation of the synthetic HDL found in the atherosclerotic lesions (121). Similarly, rHDL encapsulating statins (S-rHDL) are more effective at reducing atherosclerosis-induced inflammation than statin or rHDL alone in mice (122). Moreover, rHDLs have been used for contrast imaging in MRI (123). Predictions around the future developments in rHDL-based therapies evolve around developing rHDL particles that act simultaneously as drug delivery and imaging systems, termed “theranostics” (120) included in Table 1.
Today, HDLs are considered as multifaceted entities beyond their cholesterol-carrying action. We attempt to understand the multiple HDL functions and the responsible mechanisms. Indeed, we are now moving away from the dualistic model of “good” and “bad” cholesterol and are constructing a more complex and realistic image of HDLs, including identifying various subclasses of HDLs using new techniques, and defining the proteome and lipidome of different HDL subclasses in health, disease and after therapies. In this review, we report new evidence about changes in size and composition as determinants of functionality. Further, the emergence of data from patients with ultra-high HDL levels challenges our understanding of HDL roles and functions. While clinical results on HDL-based therapies remain controversial, a more refined understanding of HDLs can lead to design more efficient clinical treatments involving these complex particles. Similarly, HDLs potential as therapeutics, although promising, is contingent on further research.
AJ and EO wrote the manuscript and figures. EO conceived the manuscript.
The continued financial support by the Swiss National Science Foundation Ambizione and Prima Grants (PZ00P3_161506 and PR00P3_179861/1 to EO); the Swiss Cardio-Onco-Grant - Alfred and Annemarie von Sick Grant, the Hartmann Müller Foundation, Olga Mayenfisch and Swiss Life Foundation (all to EO) are gratefully acknowledged.
The authors declare that the research was conducted in the absence of any commercial or financial relationships that could be construed as a potential conflict of interest.
We gratefully acknowledge the intellectual input and manuscript editing provided by Anita Nasrallah, Ph.D.
The Supplementary Material for this article can be found online at: https://www.frontiersin.org/articles/10.3389/fcvm.2020.00039/full#supplementary-material
Supplementary Table 1. Details of the different HDL sub-classification categories based on the different methods of HDL separation. Nuclear magnetic resonance (NMR) [method described in Otvos et al. (38)] has the advantage of measuring HDL classes from whole plasma and thus does not require preliminary isolation.
ABCA1, ATP-Binding Cassette Transporter 1; apoA1, Apolipoprotein A1; ApoA2, Apolipoprotein A2; CD36, Cluster of Differentiation 36; CETP(i), Cholesteryl Ester Transfer Protein (inhibitor); eNOS, Endothelial Nitric Oxide Synthase; EPC, Endothelial Progenitor Cells; FMD, Flow Mediated Vasodilation; HDL, High Density Lipoproteins; HDL-C, HDL cholesterol; HDL-TG, HDL triglycerides; LDL, Low Density Lipoprotein; miRNA, Micro Ribonucleic Acid; NO, Nitric Oxide; NMR, nuclear magnetic resonance; PL, Phospholipid; PON-1, Paraoxonase-1; RCT, Reverse Cholesterol Transport; rHDL, Reconstituted HDL; RYGB, Roux-en-Y Gastric Bypass; S1P, Sphingosine-1-Phosphate; S1PR, Sphingosine-1-Phosphate Receptor; SM, Sphingomyelin; S-rHDL, Reconstituted HDL encapsulated statin; T2DM, Type 2 Diabetes Mellitus; TNF-α, Tumor Necrosis Factor α; TG, Triglycerides; VEGF-A, Vascular Endothelial Growth Factor A; VEGFR2, Vascular Endothelial Growth Factor Receptor 2; VLDL, Very Low-Density Lipoproteins
1. Castelli WP, Anderson K, Wilson PWF, Levy D. Lipids and risk of coronary heart disease. The framingham study. Ann Epidemiol. (1992) 2:23–8. doi: 10.1016/1047-2797(92)90033-M
2. Di Angelantonio E, Sarwar N, Perry P, Kaptoge S, Ray KK, Thompson A, et al. Major lipids, apolipoproteins, and risk of vascular disease. JAMA. (2009) 302:1993–2000. doi: 10.1001/jama.2009.1619
3. Parini P, Rudel LL. Is there a need for cholesteryl ester transfer protein inhibition. Arterioscler Thromb Vasc Biol. (2003) 23:374–5. doi: 10.1161/01.ATV.0000060447.25136.1C
4. Toth PP, Barter PJ, Rosenson RS, Boden WE, Chapman MJ, Cuchel M, et al. High-density lipoproteins: a consensus statement from the National Lipid Association. J Clin Lipidol. (2013) 7:484–525. doi: 10.1016/j.jacl.2013.08.001
5. Boden WE, Probstfield JL, Anderson T, Chaitman BR, Desvignes-Nickens P, Koprowicz K, et al. Niacin in patients with low HDL cholesterol levels receiving intensive statin therapy. N Engl J Med. (2011) 365:2255–67. doi: 10.1056/NEJMoa1107579
6. Lincoff AM, Nicholls SJ, Riesmeyer JS, Barter PJ, Brewer HB, Fox KAA, et al. Evacetrapib and cardiovascular outcomes in high-risk vascular disease. N Engl J Med. (2017) 376:1933–42. doi: 10.1056/NEJMoa1609581
7. Bowman L, Hopewell JC, Chen F, Wallendszus K, Stevens W, Collins R, et al. Effects of anacetrapib in patients with atherosclerotic vascular disease. N Engl J Med. (2017) 377:1217–27. doi: 10.1056/NEJMoa1706444
8. Tall AR, Rader DJ. Trials and tribulations of CETP inhibitors. Circ Res. (2018) 122:106–12. doi: 10.1161/CIRCRESAHA.117.311978
9. Di Bartolo BA, Nicholls SJ. Anacetrapib as a potential cardioprotective strategy. Drug Des Devel Ther. (2017) 11:3497–502. doi: 10.2147/DDDT.S114104
10. Hopewell JC, Ibrahim M, Hill M, Shaw PM, Braunwald E, Blaustein RO, et al. Impact of ADCY9 genotype on response to anacetrapib. Circulation. (2019) 140:891–8. doi: 10.1161/CIRCULATIONAHA.119.041546
11. Palmer TM, Lawlor DA, Harbord RM, Sheehan NA, Tobias JH, Timpson NJ, et al. Using multiple genetic variants as instrumental variables for modifiable risk factors. Stat Methods Med Res. (2012) 21:223–42. doi: 10.1177/0962280210394459
12. Voight BF, Peloso GM, Orho-Melander M, Frikke-Schmidt R, Barbalic M, Jensen MK, et al. Plasma HDL cholesterol and risk of myocardial infarction: a mendelian randomisation study. Lancet. (2012) 380:572–80. doi: 10.1016/S0140-6736(12)60312-2
13. Kaur N, Pandey A, Negi H, Shafiq N, Reddy S, Kaur H, et al. Effect of HDL-raising drugs on cardiovascular outcomes: a systematic review and meta-regression. PLoS ONE. (2014) 9:e94585. doi: 10.1371/journal.pone.0094585
14. Qiu C, Zhao X, Zhou Q, Zhang Z. High-density lipoprotein cholesterol efflux capacity is inversely associated with cardiovascular risk: a systematic review and meta-analysis. Lipids Health Dis. (2017) 16:212. doi: 10.1186/s12944-017-0604-5
15. Li Y, Xu Y, Jadhav K, Zhu Y, Yin L, Zhang Y. Hepatic forkhead box protein A3 Regulates ApoA-I (Apolipoprotein A-I) expression, cholesterol efflux, and atherogenesis. Arterioscler Thromb Vasc Biol. (2019) 39:1574–87. doi: 10.1161/ATVBAHA.119.312610
16. Zannis VI, Fotakis P, Koukos G, Kardassis D, Ehnholm C, Jauhiainen M, et al. HDL biogenesis, remodeling, and catabolism. In: von Eckardstein A, Kardassis D, editors. Handbook of Experimental Pharmacology. Cham: Springer International Publishing (2015). p. 53–111.
17. Neufeld EB, Sato M, Gordon SM, Durbhakula V, Francone N, Aponte A, et al. ApoA-I-mediated lipoprotein remodeling monitored with a fluorescent phospholipid. Biology. (2019). 8:E53. doi: 10.3390/biology8030053
18. Niesor EJ, Chaput E, Mary J-L, Staempfli A, Topp A, Stauffer A, et al. Effect of compounds affecting ABCA1 expression and CETP activity on the HDL pathway involved in intestinal absorption of lutein and zeaxanthin. Lipids. (2014) 49:1233–43. doi: 10.1007/s11745-014-3958-8
19. Quintanilla-Cantú A, Peña-de-la-Sancha P, Flores-Castillo C, Mejía-Domínguez AM, Posadas-Sánchez R, Pérez-Hernández N., et al. Small HDL subclasses become cholesterol-poor during postprandial period after a fat diet intake in subjects with high triglyceridemia increases. Clin Chim Acta. (2017) 464:98–105. doi: 10.1016/j.cca.2016.11.018
20. Mora S, Rifai N, Buring JE, Ridker PM. Fasting compared with nonfasting lipids and apolipoproteins for predicting incident cardiovascular events. Circulation. (2008) 118:993–1001. doi: 10.1161/CIRCULATIONAHA.108.777334
21. Rahman F, Blumenthal RS, Jones SR, Martin SS, Gluckman TJ, Whelton SP. Fasting or non-fasting lipids for atherosclerotic cardiovascular disease risk assessment and treatment. Curr Atheroscler Rep. (2018) 20:14. doi: 10.1007/s11883-018-0713-2
22. Marques PE, Nyegaard S, Collins RF, Troise F, Freeman SA, Trimble WS, et al. Multimerization and retention of the scavenger receptor SR-B1 in the plasma membrane. Dev Cell. (2019) 50:283–95.e5. doi: 10.1016/j.devcel.2019.05.026
23. Zhou L, Li C, Gao L, Wang A. High-density lipoprotein synthesis and metabolism (Review). Mol Med Rep. (2015) 12:4015–21. doi: 10.3892/mmr.2015.3930
24. Bergt C, Oram JF, Heinecke JW. Oxidized HDL. Arterioscler Thromb Vasc Biol. (2003) 23:1488–90. doi: 10.1161/01.ATV.0000090570.99836.9C
25. Bojanic DD, Tarr PT, Gale GD, Smith DJ, Bok D, Chen B, et al. Differential expression and function of ABCG1 and ABCG4 during development and aging. J Lipid Res. (2010) 51:169–81. doi: 10.1194/jlr.M900250-JLR200
26. Gu X, Trigatti B, Xu S, Acton S, Babitt J, Krieger M. The efficient cellular uptake of high density lipoprotein lipids via scavenger receptor class B type I requires not only receptor-mediated surface binding but also receptor-specific lipid transfer mediated by its extracellular domain. J Biol Chem. (1998) 273:26338–48. doi: 10.1074/jbc.273.41.26338
27. Connelly MA, Klein SM, Azhar S, Abumrad NA, Williams DL. Comparison of class B scavenger receptors, CD36 and scavenger receptor BI (SR-BI), shows that both receptors mediate high density lipoprotein-cholesteryl ester selective uptake but SR-BI exhibits a unique enhancement of cholesteryl ester uptake. J Biol Chem. (1999) 274:41–7. doi: 10.1074/jbc.274.1.41
28. Sun B, Eckhardt ER, Shetty S, van der Westhuyzen DR, Webb NR. Quantitative analysis of SR-BI-dependent HDL retroendocytosis in hepatocytes and fibroblasts. J Lipid Res. (2006) 47:1700–13. doi: 10.1194/jlr.M500450-JLR200
29. Randolph GJ, Miller NE. Lymphatic transport of high-density lipoproteins and chylomicrons. J Clin Invest. (2014) 124:929–35. doi: 10.1172/JCI71610
30. Zanoni P, Velagapudi S, Yalcinkaya M, Rohrer L, von Eckardstein A. Endocytosis of lipoproteins. Atherosclerosis. (2018) 275:273–95. doi: 10.1016/j.atherosclerosis.2018.06.881
31. Velagapudi S, Yalcinkaya M, Piemontese A, Meier R, Norrelykke SF, Perisa D, et al. VEGF-A regulates cellular localization of SR-BI as well as transendothelial transport of HDL but not LDL. Arterioscler Thromb Vasc Biol. (2017) 37:794–803. doi: 10.1161/ATVBAHA.117.309284
32. Plochberger B, Röhrl C, Preiner J, Rankl C, Brameshuber M, Madl J, et al. HDL particles incorporate into lipid bilayers - a combined AFM and single molecule fluorescence microscopy study. Sci Rep. (2017) 7:15886. doi: 10.1038/s41598-017-15949-7
33. Kontush A, Lindahl M, Lhomme M, Calabresi L, Chapman MJ, Davidson WS. Structure of HDL: particle subclasses and molecular components. In: von Eckardstein A, Kardassis D, editors. Handbook of Experimental Pharmacology. Cham: Springer International Publishing (2015). p. 3–51.
34. Rosenson RS, Brewer HBJ, Chapman MJ, Fazio S, Hussain MM, Kontush A, et al. HDL measures, particle heterogeneity, proposed nomenclature, and relation to atherosclerotic cardiovascular events. Clin Chem. (2011) 57:392–410. doi: 10.1373/clinchem.2010.155333
35. Kontush A. HDL particle number and size as predictors of cardiovascular disease. Front Pharmacol. (2015) 6:218. doi: 10.3389/fphar.2015.00218
36. Martin SS, Khokhar AA, May HT, Kulkarni KR, Blaha MJ, Joshi PH, et al. HDL cholesterol subclasses, myocardial infarction, and mortality in secondary prevention: the Lipoprotein Investigators Collaborative. Eur Heart J. (2015) 36:22–30. doi: 10.1093/eurheartj/ehu264
37. Mora S, Glynn RJ, Ridker PM. High-density lipoprotein cholesterol, size, particle number, and residual vascular risk after potent statin therapy. Circulation. (2013) 128:1189–97. doi: 10.1161/CIRCULATIONAHA.113.002671
38. Otvos JD, Jeyarajah EJ, Bennett DW, Krauss RM. Development of a proton nuclear magnetic resonance spectroscopic method for determining plasma lipoprotein concentrations and subspecies distributions from a single, rapid measurement. Clin Chem. (1992) 38:1632–8. doi: 10.1093/clinchem/38.9.1632
39. Kontush A, Lhomme M, Chapman MJ. Unraveling the complexities of the HDL lipidome. J Lipid Res. (2013) 54:2950–63. doi: 10.1194/jlr.R036095
40. O'Reilly M, Dillon E, Guo W, Finucane O, McMorrow A, Murphy A, et al. High-density lipoprotein proteomic composition, and not efflux capacity, reflects differential modulation of reverse cholesterol transport by saturated and monounsaturated fat diets. Circulation. (2016) 133:1838–50. doi: 10.1161/CIRCULATIONAHA.115.020278
41. Kajani S, Curley S, McGillicuddy FC. Unravelling HDL-Looking beyond the cholesterol surface to the quality within. Int J Mol Sci. (2018) 19:E1971. doi: 10.3390/ijms19071971
42. Brunham LR, Kruit JK, Iqbal J, Fievet C, Timmins JM, Pape TD, et al. Intestinal ABCA1 directly contributes to HDL biogenesis in vivo. J Clin Invest. (2006) 116:1052–62. doi: 10.1172/JCI27352
43. Besler C, Heinrich K, Rohrer L, Doerries C, Riwanto M, Shih DM, et al. Mechanisms underlying adverse effects of HDL on eNOS-activating pathways in patients with coronary artery disease. J Clin Invest. (2011) 121:2693–208. doi: 10.1172/JCI42946
44. Yuhanna IS, Zhu Y, Cox BE, Hahner LD, Osborne-Lawrence S, Lu P, et al. High-density lipoprotein binding to scavenger receptor-BI activates endothelial nitric oxide synthase. Nat Med. (2001) 7:853–7. doi: 10.1038/89986
45. Nofer JR, van der Giet M, Tolle M, Wolinska I, von Wnuck Lipinski K, Baba HA, et al. HDL induces NO-dependent vasorelaxation via the lysophospholipid receptor S1P3. J Clin Invest. (2004) 113:569–81. doi: 10.1172/JCI200418004
46. Mineo C, Yuhanna IS, Quon MJ, Shaul PW. High density lipoprotein-induced endothelial nitric-oxide synthase activation is mediated by Akt and MAP kinases. J Biol Chem. (2003) 278:9142–9. doi: 10.1074/jbc.M211394200
47. Ramet ME, Ramet M, Lu Q, Nickerson M, Savolainen MJ, Malzone A, et al. High-density lipoprotein increases the abundance of eNOS protein in human vascular endothelial cells by increasing its half-life. J Am Coll Cardiol. (2003) 41:2288–97. doi: 10.1016/S0735-1097(03)00481-9
48. Karlsson H, Kontush A, James RW. Functionality of HDL: antioxidation and detoxifying effects. In: von Eckardstein A, Kardassis D, editors. Handbook of Experimental Pharmacology. Cham: Springer International Publishing (2015). p. 207–28.
49. Ribas V, Sanchez-Quesada JL, Anton R, Camacho M, Julve J, Escola-Gil JC, et al. Human apolipoprotein A-II enrichment displaces paraoxonase from HDL and impairs its antioxidant properties: a new mechanism linking HDL protein composition and antiatherogenic potential. Circ Res. (2004) 95:789–97. doi: 10.1161/01.RES.0000146031.94850.5f
50. Ashby DT, Rye KA, Clay MA, Vadas MA, Gamble JR, Barter PJ. Factors influencing the ability of HDL to inhibit expression of vascular cell adhesion molecule-1 in endothelial cells. Arterioscler Thromb Vasc Biol. (1998) 18:1450–5. doi: 10.1161/01.ATV.18.9.1450
51. Rosenson RS, Brewer HBJ, Ansell BJ, Barter P, Chapman MJ, Heinecke JW, et al. Dysfunctional HDL and atherosclerotic cardiovascular disease. Nat Rev Cardiol. (2016) 13:48–60. doi: 10.1038/nrcardio.2015.124
52. Femlak M, Gluba-Brzózka A, Ciałkowska-Rysz A, Rysz J. The role and function of HDL in patients with diabetes mellitus and the related cardiovascular risk. Lipids Health Dis. (2017) 16:207. doi: 10.1186/s12944-017-0594-3
53. Wiesner P, Leidl K, Boettcher A, Schmitz G, Liebisch G. Lipid profiling of FPLC-separated lipoprotein fractions by electrospray ionization tandem mass spectrometry. J Lipid Res. (2009) 50:574–85. doi: 10.1194/jlr.D800028-JLR200
54. Yancey PG, de la Llera-Moya M, Swarnakar S, Monzo P, Klein SM, Connelly MA, et al. High density lipoprotein phospholipid composition is a major determinant of the bi-directional flux net movement of cellular free cholesterol mediated by scavenger receptor BI. J Biol Chem. (2000) 275:36596–604. doi: 10.1074/jbc.M006924200
55. Wang H, Huang H, Ding SF. Sphingosine-1-phosphate promotes the proliferation and attenuates apoptosis of Endothelial progenitor cells via S1PR1/S1PR3/PI3K/Akt pathway. Cell Biol Int. (2018) 42:1492–502. doi: 10.1002/cbin.10991
56. Persegol L, Darabi M, Dauteuille C, Lhomme M, Chantepie S, Rye KA, et al. Small dense HDLs display potent vasorelaxing activity, reflecting their elevated content of sphingosine-1-phosphate. J Lipid Res. (2018) 59:25–34. doi: 10.1194/jlr.M076927
57. Ruiz M, Okada H, Dahlback B. HDL-associated ApoM is anti-apoptotic by delivering sphingosine 1-phosphate to S1P1 and S1P3 receptors on vascular endothelium. Lipids Health Dis. (2017) 16:36. doi: 10.1186/s12944-017-0429-2
58. Rayner KJ, Hennessy EJ. Extracellular communication via microRNA: lipid particles have a new message. J Lipid Res. (2013) 54:1174–81. doi: 10.1194/jlr.R034991
59. Tsui NB, Ng EK, Lo YM. Stability of endogenous and added RNA in blood specimens, serum, and plasma. Clin Chem. (2002) 48:1647–53. doi: 10.1093/clinchem/48.10.1647
60. Tabet F, Vickers KC, Cuesta Torres LF, Wiese CB, Shoucri BM, Lambert G, et al. HDL-transferred microRNA-223 regulates ICAM-1 expression in endothelial cells. Nat Commun. (2014) 5:3292. doi: 10.1038/ncomms4292
61. Ren K, Zhu X, Zheng Z, Mo ZC, Peng XS, Zeng YZ, et al. MicroRNA-24 aggravates atherosclerosis by inhibiting selective lipid uptake from HDL cholesterol via the post-transcriptional repression of scavenger receptor class B type I. Atherosclerosis. (2018) 270:57–67. doi: 10.1016/j.atherosclerosis.2018.01.045
62. Vickers KC, Palmisano BT, Shoucri BM, Shamburek RD, Remaley AT. MicroRNAs are transported in plasma and delivered to recipient cells by high-density lipoproteins. Nat Cell Biol. (2011) 13:423–33. doi: 10.1038/ncb2210
63. Soria-Florido MT, Castañer O, Lassale C, Estruch R, Salas-Salvadó J, Martínez-González MÁ, et al. Dysfunctional high-density lipoproteins are associated with a greater incidence of acute coronary syndrome in a population at high cardiovascular risk: a nested-case-control study. Circulation. (2020). 141:444–53. doi: 10.1161/CIRCULATIONAHA.119.041658
64. Zewinger S, Kleber ME, Rohrer L, Lehmann M, Triem S, Jennings RT, et al. Symmetric dimethylarginine, high-density lipoproteins and cardiovascular disease. Eur Heart J. (2017) 38:1597–607. doi: 10.1093/eurheartj/ehx118
65. Chang FJ, Yuan HY, Hu XX, Ou ZJ, Fu L, Lin ZB, et al. High density lipoprotein from patients with valvular heart disease uncouples endothelial nitric oxide synthase. J Mol Cell Cardiol. (2014) 74:209–19. doi: 10.1016/j.yjmcc.2014.05.015
66. Vaisar T, Couzens E, Hwang A, Russell M, Barlow CE, DeFina LF, et al. (2018) Type 2 diabetes is associated with loss of HDL endothelium protective functions. PLoS ONE. 13:e0192616. doi: 10.1371/journal.pone.0192616
67. Giraud C, Tournadre A, Pereira B, Dutheil F, Soubrier M, Lhomme M, et al. Alterations of HDL particle phospholipid composition and role of inflammation in rheumatoid arthritis. J Physiol Biochem. (2019) 75:453–62. doi: 10.1007/s13105-019-00694-4
68. Greene DJ, Skeggs JW, Morton RE. Elevated triglyceride content diminishes the capacity of high density lipoprotein to deliver cholesteryl esters via the scavenger receptor class B type I (SR-BI). J Biol Chem. (2001) 276:4804–11. doi: 10.1074/jbc.M008725200
69. Rosenson RS, Brewer HBJ, Ansell B, Barter P, Chapman MJ, Heinecke JW, et al. Translation of high-density lipoprotein function into clinical practice: current prospects and future challenges. Circulation. (2013) 128:1256–67. doi: 10.1161/CIRCULATIONAHA.113.000962
70. Girona J, Amigo N, Ibarretxe D, Plana N, Rodriguez-Borjabad C, Heras M, et al. HDL triglycerides: a new marker of metabolic and cardiovascular risk. Int J Mol Sci. (2019) 20:3151. doi: 10.3390/ijms20133151
71. Ru D, Zhiqing H, Lin Z, Feng W, Feng Z, Jiayou Z, et al. Oxidized high-density lipoprotein accelerates atherosclerosis progression by inducing the imbalance between treg and teff in LDLR knockout mice. APMIS. (2015) 123:410–21. doi: 10.1111/apm.12362
72. Kashyap SR, Osme A, Ilchenko S, Golizeh M, Lee K, Wang S, et al. Glycation reduces the stability of ApoAI and increases HDL dysfunction in diet-controlled type 2 Diabetes. J Clin Endocrinol Metab. (2018) 103:388–96. doi: 10.1210/jc.2017-01551
73. Wang G, Mathew AV, Yu H, Li L, He L, Gao W, et al. Myeloperoxidase mediated HDL oxidation and HDL proteome changes do not contribute to dysfunctional HDL in Chinese subjects with coronary artery disease. PLoS ONE. (2018) 13:e0193782. doi: 10.1371/journal.pone.0193782
74. Macdonald DL, Terry TL, Agellon LB, Nation PN, Francis GA. Administration of tyrosyl radical–oxidized HDL inhibits the development of atherosclerosis in apolipoprotein E–deficient mice. Arter Thromb Vasc Biol. (2003) 23:1583–8. doi: 10.1161/01.ATV.0000085840.67498.00
75. Amigó N, Mallol R, Heras M, Martínez-Hervás S, Blanco Vaca F, Escolà-Gil JC, et al. Lipoprotein hydrophobic core lipids are partially extruded to surface in smaller HDL: “Herniated” HDL, a common feature in diabetes. Sci Rep. (2016) 6:19249. doi: 10.1038/srep19249
76. Siebel AL, Heywood SE, Kingwell BA. HDL and glucose metabolism: current evidence and therapeutic potential. Front Pharmacol. (2015) 6:258. doi: 10.3389/fphar.2015.00258
77. Dalla-Riva J, Stenkula KG, Petrlova J, Lagerstedt JO. Discoidal HDL and apoA-I-derived peptides improve glucose uptake in skeletal muscle. J Lipid Res. (2013) 54:1275–82. doi: 10.1194/jlr.M032904
78. Stenkula KG, Lindahl M, Petrlova J, Dalla-Riva J, Göransson O, Cushman SW, et al. Single injections of apoA-I acutely improve in vivo glucose tolerance in insulin-resistant mice. Diabetologia. (2014) 57:797–800. doi: 10.1007/s00125-014-3162-7
79. von Eckardstein A, Widmann C. High-density lipoprotein, beta cells, and diabetes. Cardiovasc Res. (2014) 103:384–94. doi: 10.1093/cvr/cvu143
80. Han CY, Tang C, Guevara ME, Wei H, Wietecha T, Shao B, et al. Serum amyloid A impairs the antiinflammatory properties of HDL. J Clin Invest. (2016) 126:266–81. doi: 10.1172/JCI83475
81. Macpherson ME, Halvorsen B, Yndestad A, Ueland T, Mollnes TE, Berge RK, et al. Impaired HDL function amplifies systemic inflammation in common variable immunodeficiency. Sci Rep. (2019) 9:9427. doi: 10.1038/s41598-019-45861-1
82. Catapano AL, Pirillo A, Bonacina F, Norata GD. HDL in innate and adaptive immunity. Cardiovasc Res. (2014) 103:372–83. doi: 10.1093/cvr/cvu150
83. Button EB, Robert J, Caffrey TM, Fan J, Zhao W, Wellington CL. HDL from an Alzheimer's disease perspective. Curr Opin Lipidol. (2019) 30:224–34. doi: 10.1097/MOL.0000000000000604
84. Hao B, Bi B, Sang C, Yu M, Di D, Luo G, et al. Systematic review and meta-analysis of the prognostic value of serum high-density lipoprotein cholesterol levels for solid tumors. Nutr Cancer. (2019) 71:547–56. doi: 10.1080/01635581.2019.1577983
85. Sun X, Wang W, Huang J, Lai H, Guo C, Wang C. A biomimic reconstituted high density lipoprotein nanosystem for enhanced VEGF gene therapy of myocardial ischemia. J Nanomater. (2015) 2015:693234. doi: 10.1155/2015/693234
86. Khan M, Lalwani ND, Drake SL, Crokatt JG, Dasseux JL. Single-dose intravenous infusion of ETC-642, a 22-Mer ApoA-I analogue and phospholipids complex, elevates HDL-C in atherosclerosis patients. Circ. (2003) 108:563–4.
87. White CR, Garber DW, Anantharamaiah GM. Anti-inflammatory and cholesterol-reducing properties of apolipoprotein mimetics: a review. J Lipid Res. (2014) 55:2007–21. doi: 10.1194/jlr.R051367
88. Raut S, Dasseux JL, Sabnis NA, Mooberry L, Lacko A. Lipoproteins for therapeutic delivery: recent advances and future opportunities. Ther Deliv. (2018) 9:257–68. doi: 10.4155/tde-2017-0122
89. Mutharasan RK, Foit L, Thaxton CS. High-density lipoproteins for therapeutic delivery systems. J Mater Chem B. (2016) 4:188–97. doi: 10.1039/C5TB01332A
90. Cormode DP, Frias JC, Ma Y, Chen W, Skajaa T, Briley-Saebo K, et al. HDL as a contrast agent for medical imaging. Clin Lipidol. (2009) 4:493–500. doi: 10.2217/clp.09.38
91. Madsen CM, Varbo A, Tybjaerg-Hansen A, Frikke-Schmidt R, Nordestgaard BG. U-shaped relationship of HDL and risk of infectious disease: two prospective population-based cohort studies. Eur Heart J. (2018) 39:1181–90. doi: 10.1093/eurheartj/ehx665
92. Allard–Ratick M, Khambhati J, Topel M, Sandesara P, Sperling L, Quyyumi A. Elevated HDL-C is associated with adverse cardiovascular outcomes. ESC Congress. (2018) 39:ehy564.50. doi: 10.1093/eurheartj/ehy564.50
93. Takaeko Y, Matsui S, Kajikawa M, Maruhashi T, Kishimoto S, Hashimoto H, et al. Association of extremely high levels of high-density lipoprotein cholesterol with endothelial dysfunction in men. J Clin Lipidol. (2019) 13:664–72.e1. doi: 10.1016/j.jacl.2019.06.004
94. Chiesa ST, Charakida M, McLoughlin E, Nguyen HC, Georgiopoulos G, Motran L, et al. Elevated high-density lipoprotein in adolescents with type 1 diabetes is associated with endothelial dysfunction in the presence of systemic inflammation. Eur Heart J. (2019) 40:3559–66. doi: 10.1093/eurheartj/ehz114
95. Aminian A, Zelisko A, Kirwan JP, Brethauer SA, Schauer PR. Exploring the impact of bariatric surgery on high density lipoprotein. Surg Obes Relat Dis. (2015) 11:238–47. doi: 10.1016/j.soard.2014.07.017
96. Aminian A, Zajichek A, Arterburn DE, Wolski KE, Brethauer SA, Schauer PR, et al. Association of metabolic surgery with major adverse cardiovascular outcomes in patients with type 2 diabetes and obesity. JAMA. (2019) 322:1271–82. doi: 10.1001/jama.2019.14231
97. Schauer PR, Bhatt DL, Kirwan JP, Wolski K, Aminian A, Brethauer SA, et al. Bariatric surgery versus intensive medical therapy for diabetes 5-Year outcomes. N Engl J Med. (2017) 376:641–51. doi: 10.1056/NEJMoa1600869
98. Osto E, Doytcheva P, Corteville C, Bueter M, Dorig C, Stivala S, et al. Rapid and body weight-independent improvement of endothelial and high-density lipoprotein function after Roux-en-Y gastric bypass: role of glucagon-like peptide-1. Circulation. (2015) 131:871–81. doi: 10.1161/CIRCULATIONAHA.114.011791
99. Heffron SP, Lin BX, Parikh M, Scolaro B, Adelman SJ, Collins HL, et al. Changes in high-density lipoprotein cholesterol efflux capacity after bariatric surgery are procedure dependent. Arterioscler Thromb Vasc Biol. (2018) 38:245–54. doi: 10.1161/ATVBAHA.117.310102
100. Adams V, Besler C, Fischer T, Riwanto M, Noack F, Hollriegel R, et al. Exercise training in patients with chronic heart failure promotes restoration of high-density lipoprotein functional properties. Circ Res. (2013) 113:1345–55. doi: 10.1161/CIRCRESAHA.113.301684
101. Kralova Lesna I, Suchanek P, Kovar J, Poledne R. Life style change and reverse cholesterol transport in obese women. Physiol Res. (2009) 58(Suppl. 1):S33–8.
102. Olchawa B, Kingwell BA, Hoang A, Schneider L, Miyazaki O, Nestel P, et al. Physical fitness and reverse cholesterol transport. Arterioscler Thromb Vasc Biol. (2004) 24:1087–91. doi: 10.1161/01.ATV.0000128124.72935.0f
103. Williams PT, Krauss RM, Vranizan KM, Wood PD. Changes in lipoprotein subfractions during diet-induced and exercise-induced weight loss in moderately overweight men. Circulation. (1990) 81:1293–304. doi: 10.1161/01.CIR.81.4.1293
104. Hernaez A, Castaner O, Elosua R, Pinto X, Estruch R, Salas-Salvado J, et al. Mediterranean diet improves high-density lipoprotein function in high-cardiovascular-risk individuals: a randomized controlled trial. Circulation. (2017) 135:633–43. doi: 10.1161/CIRCULATIONAHA.116.023712
105. Tabet F, Cuesta Torres LF, Ong KL, Shrestha S, Choteau SA, Barter PJ, et al. High-density lipoprotein-associated miR-223 is altered after diet-induced weight loss in overweight and obese males. PLoS ONE. (2016) 11:e0151061. doi: 10.1371/journal.pone.0151061
106. Bartelt A, John C, Schaltenberg N, Berbee JFP, Worthmann A, Cherradi ML, et al. Thermogenic adipocytes promote HDL turnover and reverse cholesterol transport. Nat Commun. (2017) 8:15010. doi: 10.1038/ncomms15010
107. Sattler K, Graler M, Keul P, Weske S, Reimann CM, Jindrova H, et al. Defects of high-density lipoproteins in coronary artery disease caused by low sphingosine-1-phosphate content: correction by sphingosine-1-phosphate-loading. J Am Coll Cardiol. (2015) 66:1470–85. doi: 10.1016/j.jacc.2015.07.057
108. Gebhard C, Rheaume E, Berry C, Brand G, Kernaleguen AE, Theberge-Julien G, et al. Beneficial effects of reconstituted high-density lipoprotein (rHDL) on circulating CD34+ cells in patients after an acute coronary syndrome. PLoS ONE. (2017) 12:e0168448. doi: 10.1371/journal.pone.0168448
109. Shaw JA, Bobik A, Murphy A, Kanellakis P, Blombery P, Mukhamedova N, et al. Infusion of reconstituted high-density lipoprotein leads to acute changes in human atherosclerotic plaque. Circ Res. (2008) 103:1084–91. doi: 10.1161/CIRCRESAHA.108.182063
110. Tardif JC, Gregoire J, L'Allier PL, Ibrahim R, Lesperance J, Heinonen TM, et al. Effects of reconstituted high-density lipoprotein infusions on coronary atherosclerosis: a randomized controlled trial. JAMA. (2007) 297:1675–82. doi: 10.1001/jama.297.15.jpc70004
111. Zhang Y, Zanotti I, Reilly MP, Glick JM, Rothblat GH, Rader DJ. Overexpression of apolipoprotein A-I promotes reverse transport of cholesterol from macrophages to feces in vivo. Circulation. (2003) 108:661–3. doi: 10.1161/01.CIR.0000086981.09834.E0
112. Bailey D, Jahagirdar R, Gordon A, Hafiane A, Campbell S, Chatur S, et al. RVX-208: a small molecule that increases apolipoprotein A-I and high-density lipoprotein cholesterol in vitro and in vivo. J Am Coll Cardiol. (2010) 55:2580–9. doi: 10.1016/j.jacc.2010.02.035
113. Nissen SE, Tsunoda T, Tuzcu EM, Schoenhagen P, Cooper CJ, Yasin M, et al. Effect of recombinant ApoA-I Milano on coronary atherosclerosis in patients with acute coronary syndromes: a randomized controlled trial. JAMA. (2003) 290:2292–300. doi: 10.1001/jama.290.17.2292
114. Degoma EM, Rader DJ. Novel HDL-directed pharmacotherapeutic strategies. Nat Rev Cardiol. (2011) 8:266–77. doi: 10.1038/nrcardio.2010.200
115. Xu W, Qian M, Huang C, Cui P, Li W, Du Q, et al. Comparison of mechanisms of endothelial cell protections between high-density lipoprotein and apolipoprotein A-I mimetic peptide. Front Pharmacol. (2019) 10:817. doi: 10.3389/fphar.2019.00817
116. Barrett TJ, Distel E, Murphy AJ, Hu J, Garshick MS, Ogando Y, et al. Apolipoprotein AI) promotes atherosclerosis regression in diabetic mice by suppressing myelopoiesis and plaque inflammation. Circulation. (2019) 140:1170–84. doi: 10.1161/CIRCULATIONAHA.119.039476
117. Andrews J, Janssan A, Nguyen T, Pisaniello AD, Scherer DJ, Kastelein JJ, et al. Effect of serial infusions of reconstituted high-density lipoprotein (CER-001) on coronary atherosclerosis: rationale and design of the CARAT study. Cardiovasc Diagn Ther. (2017) 7:45–51. doi: 10.21037/cdt.2017.01.01
118. Wolfrum C, Shi S, Jayaprakash KN, Jayaraman M, Wang G, Pandey RK, et al. Mechanisms and optimization of in vivo delivery of lipophilic siRNAs. Nat Biotechnol. (2007) 25:1149–57. doi: 10.1038/nbt1339
119. Muller A, Beck K, Rancic Z, Muller C, Fischer CR, Betzel T, et al. Imaging atherosclerotic plaque inflammation via folate receptor targeting using a novel 18F-folate radiotracer. Mol Imaging. (2014) 13:1–11. doi: 10.2310/7290.2013.00074
120. Kornmueller K, Vidakovic I, Prassl R. Artificial high density lipoprotein nanoparticles in cardiovascular research. Molecules. (2019) 24:E2829. doi: 10.3390/molecules24152829
121. Guo Y, Yuan W, Yu B, Kuai R, Hu W, Morin EE, et al. Synthetic high-density lipoprotein-mediated targeted delivery of liver x receptors agonist promotes atherosclerosis regression. EBioMedicine. (2018) 28:225–33. doi: 10.1016/j.ebiom.2017.12.021
122. Duivenvoorden R, Tang J, Cormode DP, Mieszawska AJ, Izquierdo-Garcia D, Ozcan C, et al. A statin-loaded reconstituted high-density lipoprotein nanoparticle inhibits atherosclerotic plaque inflammation. Nat Commun. (2014) 5:3065. doi: 10.1038/ncomms4065
Keywords: high density lipoprotein, cardiovascular risk, obesity, endothelial function, HDL-therapy, bariatric surgery, lipoproteins
Citation: Jomard A and Osto E (2020) High Density Lipoproteins: Metabolism, Function, and Therapeutic Potential. Front. Cardiovasc. Med. 7:39. doi: 10.3389/fcvm.2020.00039
Received: 05 January 2020; Accepted: 28 February 2020;
Published: 31 March 2020.
Edited by:
Rory R. Koenen, Maastricht University, NetherlandsReviewed by:
Oscar Perez-Mendez, Instituto Nacional de Cardiología, MexicoCopyright © 2020 Jomard and Osto. This is an open-access article distributed under the terms of the Creative Commons Attribution License (CC BY). The use, distribution or reproduction in other forums is permitted, provided the original author(s) and the copyright owner(s) are credited and that the original publication in this journal is cited, in accordance with accepted academic practice. No use, distribution or reproduction is permitted which does not comply with these terms.
*Correspondence: Elena Osto, ZWxlbmEub3N0b0B1emguY2g=
Disclaimer: All claims expressed in this article are solely those of the authors and do not necessarily represent those of their affiliated organizations, or those of the publisher, the editors and the reviewers. Any product that may be evaluated in this article or claim that may be made by its manufacturer is not guaranteed or endorsed by the publisher.
Research integrity at Frontiers
Learn more about the work of our research integrity team to safeguard the quality of each article we publish.