- Cardiology, Department of Biomedical Research, Bern University Hospital, Bern, Switzerland
Three-dimensional (3D) cell culture is often mentioned in the context of regenerative medicine, for example, for the replacement of ischemic myocardium with tissue-engineered muscle constructs. Additionally, 3D cell culture is used, although less commonly, in basic research, toxicology, and drug development. These applications have recently benefited from innovations in stem cell technologies allowing the mass-production of hiPSC-derived cardiomyocytes or other cardiovascular cells, and from new culturing methods including organ-on-chip and bioprinting technologies. On the analysis side, improved sensors, computer-assisted image analysis, and data collection techniques have lowered the bar for switching to 3D cell culture models. Nevertheless, 3D cell culture is not as widespread or standardized as traditional cell culture methods using monolayers of cells on flat surfaces. The many possibilities of 3D cell culture, but also its limitations, drawbacks and methodological pitfalls, are less well-known. This article reviews currently used cardiovascular 3D cell culture production methods and analysis techniques for the investigation of cardiotoxicity, in drug development and for disease modeling.
The Need for More Tissue-Like Cell Culture Models
Standard cell culture using adherent cells on multi-well plastic plates, dishes and flasks is an effective technique for expanding cell lines, bioproduction, and inspection of cells in defined conditions. However, as soon as cultured cells are expected to respond to drugs, toxins or signaling modifiers like in vivo, cell culture on flat surfaces, i.e., two-dimensional (2D) culture, turns out to be an imperfect or downright misleading (1–4). Cells in an intact tissue are embedded in extracellular matrix proteins (ECM) and are exposed to an abundance of biochemical, mechanical, electrical and other types of stimuli that lead to appropriate responses and fine-tuned changes in gene expression. In the heart, cells undergo cyclic deformation, show rapid calcium transients and electrical signals or experience shear stress from blood flow (1). In order to retain organotypic functionality as much as possible, a straightforward approach is to use fully differentiated cells directly isolated from living tissue, as these are considered to be in a native state (2). Animal models, explanted hearts and later freshly isolated primary cells have been used for the assessments of various parameters of cardiac cell physiology and electrophysiology for more than a century, which has increased our knowledge of the basic mechanisms of the heart tremendously (5–7). Large animal models such as minipigs or goats are also still needed as they provide the essential anatomical structures to study surgical interventions and to observe in situ clinically relevant pathophysiological changes of heart and vessels, such as the response to pressure overload, myocarditis or atherosclerosis and infarcts (3). However, animal models are relatively expensive, need experienced personnel, long-term housing, strict quality control, and there are ethical concerns (4). Also, there are differences between the human heart and those of animals, and these differences can become more pronounced and limiting in pathological conditions (8). For these reasons, it would be advantageous to have access to in vitro screening models of the myocardium that allow the study of long-term effects of drugs, environmental factors and gene mutations, preferentially on a human genetic background.
Because of the above-mentioned limitations of classic models, three-dimensional (3D) culture systems have been developed that attempt to restore in vivo conditions in some sort of a multicellular micro-tissue (MT) with and without additional, natural or synthetic biomaterials, also called scaffolds. Historically, 3D cultures have first been used in a systematic manner for drug testing in cancer biology, which is explained in part by the fact that cellular aggregates with a hypoxic core show many similarities with avascular solid tumors (9). It has been repeatedly found, that only 3D technologies using co-cultures are able to mimic key aspects of the phenotypical and cellular heterogeneity as well as microenvironmental aspects of tumor growth (10). In the cardiovascular field, current 3D cell culture model systems that are in use for drug testing and toxicology applications mostly fall into two main categories: They contain A. a scaffold matrix, typically a hydrogel, which is mixed with and populated by cells and forms a strip or hourglass-shaped contracting MT between attachment sites, also called an engineered heart tissue (EHT) (Figure 1) (5), or B. smaller cellular aggregates (spheroids) forming by self-assembly without scaffold proteins in hanging drops or in multi-well plates with non-adhesive surfaces (Figure 2). An increasing number of studies making use of cardiac scaffold-free spheroids for drug testing and toxicology has been published in the last couple of years (6, 7) and frequently, a mix or co-culture of several cell types is used, such as rodent or human primary- or human induced pluripotent stem cell-derived cardiomyocytes (hiPSC-CMs), fibroblasts, stem cells, and endothelial cells (19–23). Additionally, microfluidic systems, micro-patterns, and microphysiological platforms, including various sensors, pumps and perfusion, and other technologies have been developed around the living components of such model systems (24). Larger tissue formats like multilayered cell sheets, re-cellularized hearts or large biomaterial patches usually are too expensive and slow in the making for drug screening purposes and are instead developed for regenerative medicine (25). Recently, the term “organoid” is more often used in the literature. However, this term should not be used for every 3D cell culture as it implies, at least by the original definition, self-organization of stem cells that leads to differentiated organotypic structures and functionality (26). In the cardiovascular field, self-organization in vitro has been observed in vascular networks (27), but for cardiac muscle cells, it is mostly limited to self-assembly of clusters by aggregation, alignment, concerted contractions and some degree of cellular maturation, while the actual embryonic development of a vascularized organ with chambers, working pump function and conduction system currently is not feasible to replicate in vitro.
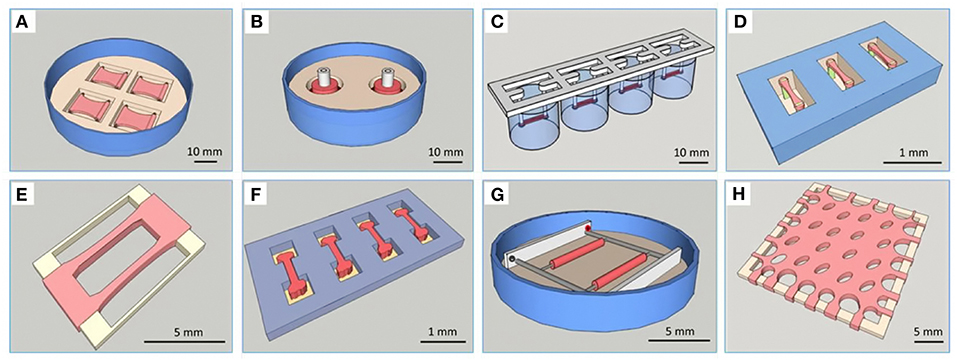
Figure 1. Overview of different types of EHTs. Reprinted with permission from Weinberger et al. (11). (A) Plane EHT on Velcro-covered rods (5), (B) fabrication of ring-shaped EHTs (12), (C) fibrin-based mini-EHT on polydimethylsiloxane (PDMS) racks (13), (D) cardiac micro tissues (CMT) on fluorescent pillars (14), (E) cardiobundles on a PDMS frame (15), (F) micro heart muscle (16), (G) cardiac biowires (17), (H) cardiac patch (18).
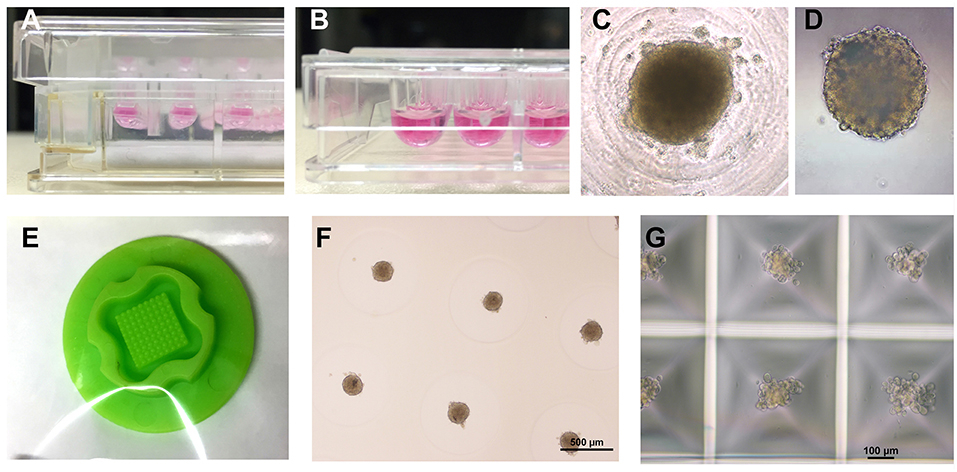
Figure 2. Overview of different spheroid production methods. (A) Hanging drops (InSphero, GravityPlus), (B) U-shaped multi-well plate with non-adhesive coating (Greiner bio-one, Cellstar), (C) view inside a U-shaped well at day 2 of culture, (D) Spheroid made in hanging drop for 3 days was transferred to a non-adhesive multi-well plate for further culture (InSphero, GravityTrap), (E) Silicone micro-mold (3D Petri-dish, microtissues.com) for making an agarose cast for 81 spheroids, (F) Cardiac spheroids reside inside the 3D petri-dish agarose cast submerged in medium 4 days after seeding of the cell solution, (G) Small cardiac spheroids forming 3 days after seeding in a micro-patterned multi-well plate with 12 × 750 microwells (Kugelmeiers, Sphericalplate 5D). All photos by the author.
The Cellular Components of Cardiac 3D Culture and Disease Models
The availability of differentiated cells for research is a challenge in the cardiac field as postnatal mammalian cardiomyocytes do not proliferate, and primary human cells are not available in significant quantities (28). The use of primary ventricular cardiomyocytes from adult animals or patients is not recommended for 3D culture as these rod-shaped cells do not integrate well in spherical aggregates and many cells become necrotic when kept floating for longer time (own observation). Fortunately, the development of hiPSC-CMs has helped to improve this issue and promoted research with human cardiomyocytes and 3D models in the cardiovascular field, even if the currently available hiPSC-CM are relatively immature (28–30). Also, the cell population is not entirely uniform as ventricular-type, nodal and atrial-like action potentials are found when single cells are analyzed using electrophysiological methods (29). Furthermore, there is heterogeneity in the expression of cytoskeleton and sarcomeric proteins such chamber-specific myosin light chains or troponins, and different grades of structural organization of sarcomeric proteins has been observed (30, 31). Another practical issue encountered with current protocols is the inconstant efficacy of the differentiation process and batch-to-batch variations that lead to baseline variations when cells from the same patient are repeatedly re-programmed (11, 32). The incomplete maturation of the cells may reduce the predictive power of the model system, considering that cardiovascular diseases predominantly occur in the elderly human population, although this demerit is not limited to hiPSC-CMs (33, 34). Several strategies, particularly with 3D cultures, have been employed to enhance the maturation of cultured hiPSC-CM such as novel iPSC reprogramming methods, changing the energy sources in specialized media, finding the ideal developmental time window for experiments, and electric and mechanical training of EHTs (35–38).
With the technology of hiPSC-CMs arrived the option of using patient-derived cell lines with disease-specific phenotypes and known mutations on a human genetic background, and with the full knowledge of the patient's medical history. This is an exciting prospect as it might enable new options in personalized medicine and gene therapies in vitro (39, 40). Similarly, hiPSC-CM technology has been used to model a number of inherited heart diseases, among them are Duchenne muscular dystrophy, Fabry disease, Danon disease, familial hypertrophic cardiomyopathy and others (41). Many of these hiPSC cell lines are publicly available in stem cell banks for use in different model systems including 3D culture approaches (42). The hypothesis that 3D culture models can provide tissue-like features was supported by using hiPSC-CMs with a disease-specific genotype in an EHT-model: The contractile deficit of hiPSC-CMs with a truncation in the sarcomeric protein titin was not visible in 2D cultured cardiomyocytes but became obvious in EHTs working against the elastic resistance of PDMS or silicone pillars (39). Regarding disease models, applications in the field of cardio-oncology have motivated our lab to explore different cardiomyocyte model systems from primary adult rat cardiomyocytes to monolayers and cardiac spheroids made of hiPSC-CM and to study cancer therapy-associated changes of contractility and calcium handling (40, 43–45). Other cardiomyopathies that have been modeled in 3D cultures include cardiac fibrosis (46), hypertrophy (47), Chagas disease (48), atrial fibrillation (49), cardiotoxic cancer therapies, and other toxins (19, 20, 22, 44, 50).
Production Methods
A number of different types of 3D culture models and production methods have been developed in the cardiovascular field (Table 1). The most basic form of 3D culture is the multicellular aggregate as it occurs by self-assembly of floating cells on low-attachment surfaces (also called liquid-overlay method). Such a suspension culture can be made in inexpensive ways, for example by using a sterile dish with a thin film of agarose. Spontaneous formation of this type of multicellular aggregate has already been observed at the time of early cardiomyocyte isolations from fetal or newborn animals (57). Many suppliers of cell culture products make variants of U-shaped bottom multi-well plates with special coating for ultra-low attachment that leads to self-assembly of spheroids (Figures 2B,C), or special formats for the mass-production of small aggregates (Figure 2G). Similarly, soft silicone molds can be used to make agarose-casts with many small wells for the production of microtissues (Figures 2E,F). The hanging drop technique has allowed producing uniform microtissues in a reliable way (44, 55), and sophisticated systems have been developed with hanging drops as part of microfluidic systems including perfusion and sensors (56). Overall, an advantage of the spheroid as 3D cell culture is the option of using semi-automatic methods to produce spheroids by using a pipetting robot for filling multi-well plates, exchanging medium, drug treatments, and finally analyzing the samples in high-content readers. Advantages and disadvantages of the spheroid culture vs. the EHT-models are listed in Table 2. The EHT model was conceived in the 90ies for the purpose of tissue engineering (5). Soon it was also used for drug testing and disease models with the option to measure contractile force, either directly or by the deflection of silicone poles, as well as calcium transients and electrical signals (13, 32, 61). Variants of the EHT model were developed that have in common the casting of a hydrogel, usually containing fibrin/thrombin and/or collagen and Matrigel components, with the addition of either primary newborn rodent cardiomyocytes or stem-cell derived cells as single and co-cultures and different geometries and analysis options (Figure 1). Elastic silicone posts deflect with the contractions and allow the tissue to contract auxotonically and perform contractile work, the physiological form of cardiac contraction (11). Smaller formats were developed for the purpose of drug treatment and optimization of maturation protocols (38, 41), while larger formats are suitable for regenerative therapies (11).
Vascular in vitro models have improved in recent years with the advent of microfluidic systems and the hope is, that these systems may partially replace animal experimentation that has been common in this field of research (62). Current vascular in vitro models apply different molding techniques, bioprinting, and combinations of these technologies for producing micro vessels on organ-on-chip platforms (51–53, 59). The challenge of oxygen supply in larger artificial tissues has been a matter of active research in tissue engineering for some time (24, 63). However, establishing a perfused vascular network in vitro turned out more difficult than initially anticipated since these processes are inherently multi-factorial and require a fine-tuned expression and post-translational processing of growth factors, a complex spatial localization of angiogenic signals in the ECM, and the collaboration of multiple cell types (organ-specific endothelial cells, pericytes, vascular smooth muscle cells) (64, 65). Instead of relying on cellular self-organization for establishing vascular networks, recent studies rather use pre-formed channels or bioprinting approaches to reach this goal (60). Additional concepts have been published that are making use of combined techniques, such as cell layers with pre-formed vascular trees obtained from animals, bioprinted and microcontact models as components of micro-physiological platforms and larger tissues for surgical applications (51, 66, 67).
Analysis Methods
For endpoint analysis, classic lab methods like tissue fixation, paraffin embedding, histology, cryosectioning, immunolabeling, and cell viability/cytotoxicity assays are feasible with most types of 3D cultures where the cells are accessible. Protein chemistry, RNA isolation and histology usually require pooling of groups of smaller cellular aggregates (own observations). A number of cell viability/toxicology assays are commercially available that can be performed with either live MT or lysed material (67). Cell physiology methods for the investigation of cardiac features in living tissues such as contractions, force, calcium cycling or electric signals require specialized instrumentation depending on the sensitivity of the sensors and the desired temporal and spatial resolution (68). Although methods exist to directly measure contractile force in single cardiac cells and small muscle strips, these methods require a skilled workforce in order to provide good reproducibility and have a slow throughput (69). Instead, a variety of optical methods have been developed to measure length changes of the whole cells or sarcomers during the contractile cycle of mammalian cardiomyocytes (70–72). These video-based systems are either commercially available as complete bundles of hardware and software (IonOptix, Sony, EHT technologies), or as open access software for image analysis that can be used with existing microscopes and cameras (73, 74). Such a video-based analysis can be realized in inexpensive ways using modified consumer cameras (so-called action cameras with high frame rates up to 240 frames per second) and open source software (68). Optical measurement using white light has the advantage of being label-free and non-invasive, so the measurement can be repeated many times, even while the cell culture is in the incubator if a camera is placed inside an atmosphere- and temperature-controlled environment (68). Besides classic electrophysiology methods using patching and impaling with sharp microelectrode pipettes, multi-electrode arrays, and impedance spectroscopy have been used to measure electrical signals and contractile activity in cardiac 3D models (75–78). Calcium-binding fluorescent dyes and voltage-sensitive dyes have been used in 2D- and 3D-cultures as this method allows to measure a greater number of samples in relatively short time, and for some applications high-content readers can be used for this purpose (Hamamatsu Photonics, Molecular Devices, PerkinElmer) (50, 79). Although these optical methods provide good results for live 3D cultures as a whole tissue, obtaining data at (sub-)cellular level from inside these MT is challenging without relying on time-consuming histology methods. High optical resolution comes at the price of limited penetration depth and technical issues such as the permeability for fluorescent dyes and antibodies, the working distance of lenses and the geometry of sample holders limit these whole-mount microscopy applications. Recently, several approaches to “clearing” MTs (i.e., homogenizing the refractive index of the fixed tissue so that it becomes transparent) have been published, and commercial solutions have become available, especially for the use with spheroids and fluorescence high-content confocal imaging (80).
When it comes to choosing a 3D culture model system, the requirements of the project dictate crucial analysis methods. For a study of mechanical features of a muscle-construct, a hydrogel-based EHT model system might be chosen, where the cells experience mechanical load and have the ability to align longitudinally. Contractile force can then be measured either directly or using video methods by measuring the deflection of attached micro-posts or pillars of known strength (39, 40, 49, 50). Otherwise, if mainly the spontaneous or electrically paced beating pattern and viability of cardiac tissues is of interest in a larger number of samples, cardiac spheroids may be the model of choice. Cardiac functionality of these spheroids can be analyzed A) using label-free methods either by computational video analysis or electrical impedance spectroscopy methods, or B) using calcium- or voltage-sensitive dyes in multi-well plate readers, or by using more advanced microscope equipment for multiparametric assessment (19, 44, 56, 68, 81–84). A comparison of features and comparative advantages or disadvantages of these scaffold-containing or scaffold-free models is shown in Table 2. Finally, it could be summarized that spheroid models are easier to integrate in existing drug developmental pipelines and to upscale the number of tests in the same batch, while the ETH models provide a better physiological representation of the myocardium and thereby enable the analysis of advanced disease models.
Why is 3D Cell Culture Not Used More Often?
Although many tools and reagents for making and analyzing 3D cell culture models are commercially available, and the number of publications in all fields of life science is increasing (85), the technology is infrequently used, outside of regenerative medicine, in academic research labs or industry several decades after the publication of the first studies (86). Reasons for this situation include that standard 2D culture is well established, with ample literature available, and previous studies to compare results (87). Furthermore, 2D culture has gained uncritical acceptance in the past, is less expensive, is more standardized, and is often easier and less time-consuming to analyze and to handle in the lab. When considering practical aspects of working with 3D culture, seemingly trivial tasks such as regular checking for culture health and growth are more difficult with most 3D models, because even smaller tissues usually are opaque and single cells not discernable unless stained (Figures 2C,D). Additionally, manual handling of the microtissues and culture medium can be challenging when the MTs are free floating, fragile or access to the tissue(s) is obstructed by surrounding containers and technical equipment. Some systems facilitate the handling of spheroids by trapping them in conical wells, in perfused chambers inside organ-on-chip designs or by incorporation of magnetic nanoparticles (88, 89) (Table 1). Fortunately, there are more technologies and products coming to the market that are targeted at 3D cell culture applications such as different formats of spheroid and EHT production systems, microfluidic technology and suitable ready-to-use equipment, reagents for the clarification of thicker tissues and adapted microscopes and software for live imaging. Finally, and despite the above-mentioned technological advancements, the research community has to agree to a set of standards and read-outs to use in efficacy and toxicity screening (90). Replacement of animal research by in vitro technologies using human cells is another substantial incentive for 3D cell culture, and already had a significant impact, for example, on how cosmetics are tested in vitro as the use of animals is banned for this purpose in Europe (4, 67). Still, it can be debated if existing 3D models already provide sufficient evidence for superior predictions of the clinical outcome of a new drug, and if these models show enough physiological relevance compared to animal or human tissue, or still lack complexity, for example regarding the role of the vasculature or the immune system (12, 90).
Conclusions and Outlook
Each in vitro model has advantages and disadvantages for using it with certain assays, regarding organotypic features and production methods. Therefore, it needs to be decided upfront, which parameters and organotypic features are essential to be included in the study and if going 3D is making sense in the context of a particular project. Apparently, if the project deals with features of single cells isolated from its myocardial environment, then 2D cultured cardiomyocytes offer the option to use classic electrophysiology, high-resolution microscopy, and other methods. Instead 3D culture mimics features of larger tissues or entire organs and is the method of choice for co-culture models. 3D cardiac cell culture technologies hold great potential for applications in tissue engineering, drug development, cardio-toxicology and disease modeling. But a considerable effort is still needed to assure the accuracy, relevance, and reproducibility of these models and to improve automation and readout techniques. Instead of pushing many different systems to the market, careful analysis of core concepts may be instrumental for establishing 3D cell culture as a widespread and validated tool in life science.
Author Contributions
All content is prepared by the author CZ with the exception of Figure 1 that is reprinted with permission from Wolters Kluwer Health, Inc.
Funding
This work was supported by the Swiss Heart Foundation to CZ.
Conflict of Interest Statement
The author declares that the research was conducted in the absence of any commercial or financial relationships that could be construed as a potential conflict of interest.
Acknowledgments
We thank Sarah Longnus Ph.D. (Cardiac Surgery Department, Univ. Hospital Bern) for support and discussions.
References
1. Tirziu D, Giordano FJ, Simons M. Cell communications in the heart. Circulation. (2010) 122:928–37. doi: 10.1161/CIRCULATIONAHA.108.847731
2. Louch WE, Sheehan KA, Wolska BM. Methods in cardiomyocyte isolation, culture, and gene transfer. J Mol Cell Cardiol. (2011) 51:288–98. doi: 10.1016/j.yjmcc.2011.06.012
3. Savoji H, Mohammadi MH, Rafatian N, Toroghi MK, Wang EY, Zhao Y, et al. Cardiovascular disease models: a game changing paradigm in drug discovery and screening. Biomaterials. (2019) 198:3–26. doi: 10.1016/j.biomaterials.2018.09.036
4. Hartung T. Look back in anger - what clinical studies tell us about preclinical work. ALTEX. (2013) 30:275–91. doi: 10.14573/altex.2013.3.275
5. Eschenhagen T, Fink C, Remmers U, Scholz H, Wattchow J, Weil J, et al. Three-dimensional reconstitution of embryonic cardiomyocytes in a collagen matrix: a new heart muscle model system. FASEB J. (1997) 11:683–94. doi: 10.1096/fasebj.11.8.9240969
6. Kelm JM, Ehler E, Nielsen LK, Schlatter S, Perriard J-C, Fussenegger M. Design of artificial myocardial microtissues. Tissue Eng. (2004) 10:201–14. doi: 10.1089/107632704322791853
7. Figtree GA, Bubb KJ, Tang O, Kizana E, Gentile C. Vascularized cardiac spheroids as novel 3d in vitro models to study cardiac fibrosis. Cells Tissues Organs. (2017) 204:191–8. doi: 10.1159/000477436
8. Milani-Nejad N, Janssen PML. Small and large animal models in cardiac contraction research: advantages and disadvantages. Pharmacol Ther. (2014) 141:235–49. doi: 10.1016/j.pharmthera.2013.10.007
9. Inch WR, McCredie JA, Sutherland RM. Growth of nodular carcinomas in rodents compared with multi-cell spheroids in tissue culture. Growth. (1970) 34:271–82.
10. Thoma CR, Zimmermann M, Agarkova I, Kelm JM, Krek W. 3D cell culture systems modeling tumor growth determinants in cancer target discovery. Adv Drug Deliv Rev. (2014) 69–70:29–41. doi: 10.1016/j.addr.2014.03.001
11. Weinberger F, Mannhardt I, Eschenhagen T. Engineering cardiac muscle tissue: a maturating field of research. Circ Res. (2017) 120:1487–500. doi: 10.1161/CIRCRESAHA.117.310738
12. Zimmermann W-H, Schneiderbanger K, Schubert P, Didié M, Münzel F, Heubach JF, et al. Tissue engineering of a differentiated cardiac muscle construct. Circ Res. (2002) 90:223–30. doi: 10.1161/hh0202.103644
13. Hansen A, Eder A, Bönstrup M, Flato M, Mewe M, Schaaf S, et al. Development of a drug screening platform based on engineered heart tissue. Circ Res. (2010) 107:35–44. doi: 10.1161/CIRCRESAHA.109.211458
14. Boudou T, Legant WR, Mu A, Borochin MA, Thavandiran N, Radisic M, et al. A microfabricated platform to measure and manipulate the mechanics of engineered cardiac microtissues. Tissue Eng Part A. (2012) 18:910–9. doi: 10.1089/ten.tea.2011.0341
15. Jackman CP, Carlson AL, Bursac N. Dynamic culture yields engineered myocardium with near-adult functional output. Biomaterials. (2016) 111:66–79. doi: 10.1016/j.biomaterials.2016.09.024
16. Huebsch N, Loskill P, Deveshwar N, Spencer CI, Judge LM, Mandegar MA, et al. Miniaturized iPS-cell-derived cardiac muscles for physiologically relevant drug response analyses. Sci Rep. (2016) 6:24726. doi: 10.1038/srep24726
17. Nunes SS, Miklas JW, Liu J, Aschar-Sobbi R, Xiao Y, Zhang B, et al. Biowire: a platform for maturation of human pluripotent stem cell-derived cardiomyocytes. Nat Methods. (2013) 10:781–7. doi: 10.1038/nmeth.2524
18. Bian W, Jackman CP, Bursac N. Controlling the structural and functional anisotropy of engineered cardiac tissues. Biofabrication. (2014) 6:024109–9. doi: 10.1088/1758-5082/6/2/024109
19. Polonchuk L, Chabria M, Badi L, Hoflack J-C, Figtree G, Davies MJ, et al. Cardiac spheroids as promising in vitro models to study the human heart microenvironment. Sci Rep. (2017) 7:7005. doi: 10.1038/s41598-017-06385-8
20. Archer CR, Sargeant R, Basak J, Pilling J, Barnes JR, Pointon A. Characterization and validation of a human 3D cardiac microtissue for the assessment of changes in cardiac pathology. Sci Rep. (2018) 8:10160. doi: 10.1038/s41598-018-28393-y
21. Ravenscroft SM, Pointon A, Williams AW, Cross MJ, Sidaway JE. Cardiac non-myocyte cells show enhanced pharmacological function suggestive of contractile maturity in stem cell derived cardiomyocyte microtissues. Toxicol Sci. (2016) 152:99–112. doi: 10.1093/toxsci/kfw069
22. Verheijen M, Schrooders Y, Gmuender H, Nudischer R, Clayton O, Hynes J, et al. Bringing in vitro analysis closer to in vivo: Studying doxorubicin toxicity and associated mechanisms in 3D human microtissues with PBPK-based dose modelling. Toxicol Lett. (2018) 294:184–92. doi: 10.1016/j.toxlet.2018.05.029
23. Richards DJ, Coyle RC, Tan Y, Jia J, Wong K, Toomer K, et al. Inspiration from heart development: biomimetic development of functional human cardiac organoids. Biomaterials. (2017) 142:112–23. doi: 10.1016/j.biomaterials.2017.07.021
24. Mathur A, Ma Z, Loskill P, Jeeawoody S, Healy KE. In vitro cardiac tissue models: Current status and future prospects. Adv Drug Deliv Rev. (2016) 96:203–13. doi: 10.1016/j.addr.2015.09.011
25. Jackman CP, Ganapathi AM, Asfour H, Qian Y, Allen BW, Li Y, et al. Engineered cardiac tissue patch maintains structural and electrical properties after epicardial implantation. Biomaterials. (2018) 159:48–58. doi: 10.1016/j.biomaterials.2018.01.002
26. Lancaster MA, Knoblich JA. Organogenesis in a dish: modeling development and disease using organoid technologies. Science. (2014) 345:1247125–5. doi: 10.1126/science.1247125
27. Folkman J, Haudenschild C. Angiogenesis in vitro. Nature. (1980) 288:551–6. doi: 10.1038/288551a0
28. Mercola M, Ruiz-Lozano P, Schneider MD. Cardiac muscle regeneration: lessons from development. Genes Dev. (2011) 25:299–309. doi: 10.1101/gad.2018411
29. Veerman CC, Kosmidis G, Mummery CL, Casini S, Verkerk AO, Bellin M. Immaturity of human stem-cell-derived cardiomyocytes in culture: fatal flaw or soluble problem? Stem Cells Dev. (2015) 24:1035–52. doi: 10.1089/scd.2014.0533
30. Zuppinger C, Gibbons G, Dutta-Passecker P, Segiser A, Most H, Suter TM. Characterization of cytoskeleton features and maturation status of cultured human iPSC-derived cardiomyocytes. Eur J Histochem. (2017) 61:2763. doi: 10.4081/ejh.2017.2763
31. Bedada FB, Wheelwright M, Metzger JM. Maturation status of sarcomere structure and function in human iPSC-derived cardiac myocytes. Biochim Biophys Acta. (2015) 1863(7 Pt B):1829–38. doi: 10.1016/j.bbamcr.2015.11.005
32. Eschenhagen T, Mummery C, Knollmann BC. Modelling sarcomeric cardiomyopathies in the dish: from human heart samples to iPSC cardiomyocytes. Cardiovasc Res. (2015) 105:424–38. doi: 10.1093/cvr/cvv017
33. Bellin M, Marchetto MC, Gage FH, Mummery CL. Induced pluripotent stem cells: the new patient? Nat Rev Mol Cell Biol. (2012) 13:713–26. doi: 10.1038/nrm3448
34. Feric NT, Radisic M. Maturing human pluripotent stem cell-derived cardiomyocytes in human engineered cardiac tissues. Adv Drug Deliv Rev. (2016) 96:110–34. doi: 10.1016/j.addr.2015.04.019
35. Fong AH, Romero-López M, Heylman CM, Keating M, Tran D, Sobrino A, et al. Three-dimensional adult cardiac extracellular matrix promotes maturation of human induced pluripotent stem cell-derived cardiomyocytes. Tissue Eng Part A. (2016) 22:1016–25. doi: 10.1089/ten.TEA.2016.0027.
36. Correia C, Koshkin A, Duarte P, Hu D, Carido M, Sebastião MJ, et al. 3D aggregate culture improves metabolic maturation of human pluripotent stem cell derived cardiomyocytes. Biotechnol Bioeng. (2017) 87:521. doi: 10.1002/bit.26504
37. Ulmer BM, Stoehr A, Schulze ML, Patel S, Gucek M, Mannhardt I, et al. Contractile work contributes to maturation of energy metabolism in hiPSC-derived cardiomyocytes. Stem Cell Rep. (2018) 10:834–47. doi: 10.1016/j.stemcr.2018.01.039
38. Ronaldson-Bouchard K, Ma SP, Yeager K, Chen T, Song L, Sirabella D, et al. Advanced maturation of human cardiac tissue grown from pluripotent stem cells. Nature. (2018) 556:239–43. doi: 10.1038/s41586-018-0016-3
39. Hinson JT, Chopra A, Nafissi N, Polacheck WJ, Benson CC, Swist S, et al. HEART DISEASE. Titin mutations in iPS cells define sarcomere insufficiency as a cause of dilated cardiomyopathy. Science. (2015) 349:982–6. doi: 10.1126/science.aaa5458
40. Sawyer DB, Zuppinger C, Miller TA, Eppenberger HM. Modulation of anthracycline-induced myofibrillar disarray in rat ventricular myocytes by neuregulin-1β and anti-erbB2 Potential Mechanism for trastuzumab-induced cardiotoxicity. Circulation. (2002) 105:1551–4. doi: 10.1161/01.CIR.0000013839.41224.1C
41. Giacomelli E, Mummery CL, Bellin M. Human heart disease: lessons from human pluripotent stem cell-derived cardiomyocytes. Cell Mol Life Sci. (2017) 74:3711–39. doi: 10.1007/s00018-017-2546-5
42. Bellin M, Mummery CL. Inherited heart disease - what can we expect from the second decade of human iPS cell research? FEBS Lett. (2016) 590:2482–93. doi: 10.1002/1873-3468.12285
43. Timolati F, Ott D, Pentassuglia L, Giraud M-N, Perriard J-C, Suter TM, et al. Neuregulin-1 beta attenuates doxorubicin-induced alterations of excitation-contraction coupling and reduces oxidative stress in adult rat cardiomyocytes. J Mol Cell Cardiol. (2006) 41:845–54. doi: 10.1016/j.yjmcc.2006.08.002
44. Beauchamp P, Moritz W, Kelm JM, Ullrich ND, Agarkova I, Anson B, et al. Development and characterization of a scaffold-free 3D spheroid model of iPSC-derived human cardiomyocytes. Tissue Eng Part C Methods. (2015) 21:852–61. doi: 10.1089/ten.tec.2014.0376
45. Lim CC, Zuppinger C, Guo X, Kuster GM, Helmes M, Eppenberger HM, et al. Anthracyclines induce calpain-dependent titin proteolysis and necrosis in cardiomyocytes. Am Soc Biochem Mol Biol. (2004) 279:8290–9. doi: 10.1074/jbc.M308033200
46. Lee M-O, Jung KB, Jo S-J, Hyun S-A, Moon K-S, Seo J-W, et al. Modelling cardiac fibrosis using three-dimensional cardiac microtissues derived from human embryonic stem cells. J Biol Eng. (2019) 13:15. doi: 10.1186/s13036-019-0139-6
47. Hirt MN, Sörensen NA, Bartholdt LM, Boeddinghaus J, Schaaf S, Eder A, et al. Increased afterload induces pathological cardiac hypertrophy: a new in vitro model. Basic Res Cardiol. (2012) 107:307–16. doi: 10.1007/s00395-012-0307-z
48. Garzoni LR, Adesse D, Soares MJ, Rossi MID, Borojevic R, de Meirelles M de NL. Fibrosis and hypertrophy induced by Trypanosoma cruzi in a three-dimensional cardiomyocyte-culture system. J Infect Dis. (2008) 197:906–15. doi: 10.1086/528373
49. Zhao Y, Rafatian N, Feric NT, Cox BJ, Aschar-Sobbi R, Wang EY, et al. A platform for generation of chamber-specific cardiac tissues and disease modeling. Cell. (2019) 176:913–8. doi: 10.1016/j.cell.2018.11.042
50. Sirenko O, Grimm FA, Ryan KR, Iwata Y, Chiu WA, Parham F, et al. In vitro cardiotoxicity assessment of environmental chemicals using an organotypic human induced pluripotent stem cell-derived model. Toxicol Appl Pharmacol. (2017) 322:60–74. doi: 10.1016/j.taap.2017.02.020
51. Hasan A, Paul A, Vrana NE, Zhao X, Memic A, Hwang Y-S, et al. Microfluidic techniques for development of 3D vascularized tissue. Biomaterials. (2014) 35:7308–25. doi: 10.1016/j.biomaterials.2014.04.091
52. Sfriso R, Zhang S, Bichsel CA, Steck O, Despont A, Guenat OT, et al. 3D artificial round section micro-vessels to investigate endothelial cells under physiological flow conditions. Sci Rep. (2018) 8:5898. doi: 10.1038/s41598-018-24273-7
53. Bichsel CA, Hall SRR, Schmid RA, Guenat OT, Geiser T. Primary human lung pericytes support and stabilize in vitro perfusable microvessels. Tissue Eng Part A. (2015) 21:2166–76. doi: 10.1089/ten.tea.2014.0545
54. van Duinen V, Zhu D, Ramakers C, van Zonneveld AJ, Vulto P, Hankemeier T. Perfused 3D angiogenic sprouting in a high-throughput in vitro platform. Angiogenesis. (2018) 438:932–9. doi: 10.1007/s10456-018-9647-0
55. Kelm JM, Breitbach M, Fischer G, Odermatt B, Agarkova I, Fleischmann BK, et al. 3D microtissue formation of undifferentiated bone marrow mesenchymal stem cells leads to elevated apoptosis. Tissue Eng Part A. (2012) 18:692–702. doi: 10.1089/ten.tea.2011.0281
56. Frey O, Misun PM, Fluri DA, Hengstler JG, Hierlemann A. Reconfigurable microfluidic hanging drop network for multi-tissue interaction and analysis. Nat Commun. (2014) 5:4250. doi: 10.1038/ncomms5250
57. Halbert SP, Bruderer R, Lin TM. In vitro organization of dissociated rat cardiac cells into beating three-dimensional structures. J Exp Med. (1971) 133:677–95. doi: 10.1084/jem.133.4.677
58. Haisler WL, Timm DM, Gage JA, Tseng H, Killian TC, Souza GR. Three-dimensional cell culturing by magnetic levitation. Nat Protoc. (2013) 8:1940–9. doi: 10.1038/nprot.2013.125
59. Cui H, Miao S, Esworthy T, Zhou X, Lee S-J, Liu C, et al. 3D bioprinting for cardiovascular regeneration and pharmacology. Adv Drug Deliv Rev. (2018) 132:252–69. doi: 10.1016/j.addr.2018.07.014
60. Mironov V, Visconti RP, Kasyanov V, Forgacs G, Drake CJ, Markwald RR. Organ printing: tissue spheroids as building blocks. Biomaterials. (2009) 30:2164–74. doi: 10.1016/j.biomaterials.2008.12.084
61. Mannhardt I, Breckwoldt K, Letuffe-Brenière D, Schaaf S, Schulz H, Neuber C, et al. Human engineered heart tissue: analysis of contractile force. Stem Cell Rep. (2016) 7:29–42. doi: 10.1016/j.stemcr.2016.04.011
62. Suter-Dick L, Alves PM, Blaauboer BJ, Bremm K-D, Brito C, Coecke S, et al. Stem cell-derived systems in toxicology assessment. Stem Cells Dev. (2015) 24:1284–96. doi: 10.1089/scd.2014.0540
63. Kelm JM, Diaz Sanchez-Bustamante C, Ehler E, Hoerstrup SP, Djonov V, Ittner L, et al. VEGF profiling and angiogenesis in human microtissues. J Biotechnol. (2005) 118:213–29. doi: 10.1016/j.jbiotec.2005.03.016
64. Jain RK. Molecular regulation of vessel maturation. Nat Med. (2003) 9:685–93. doi: 10.1038/nm0603-685
65. Martino MM, Brkic S, Bovo E, Burger M, Schaefer DJ, Wolff T, et al. Extracellular matrix and growth factor engineering for controlled angiogenesis in regenerative medicine. Front Bioeng Biotechnol. (2015) 3:45. doi: 10.3389/fbioe.2015.00045
66. Lind JU, Busbee TA, Valentine AD, Pasqualini FS, Yuan H, Yadid M, et al. Instrumented cardiac microphysiological devices via multimaterial three-dimensional printing. Nat Mater. (2017) 16:303–8. doi: 10.1038/nmat4782
67. Rimann M, Graf-Hausner U. Synthetic 3D multicellular systems for drug development. Curr Opin Biotechnol. (2012) 23:803–9. doi: 10.1016/j.copbio.2012.01.011
68. Zuppinger C. Measurement of contractility and calcium release in cardiac spheroids. In: Heizmann CW, editor. Calcium-Binding Proteins of the EF-Hand Superfamily. Methods in Molecular Biology, Vol. 1929. New York, NY: Humana Press (2019). p. 41–52. doi: 10.1007/978-1-4939-9030-6_4
69. Palmer RE, Brady AJ, Roos KP. Mechanical measurements from isolated cardiac myocytes using a pipette attachment system. Am J Physiol. (1996) 270(2 Pt 1):C697–704. doi: 10.1152/ajpcell.1996.270.2.C697
70. Huebsch N, Loskill P, Mandegar MA, Marks NC, Sheehan AS, Ma Z, et al. Automated video-based analysis of contractility and calcium flux in human-induced pluripotent stem cell-derived cardiomyocytes cultured over different spatial scales. Tissue Eng Part C Methods. (2015) 21:467–79. doi: 10.1089/ten.tec.2014.0283
71. Kondo RP, Apstein CS, Eberli FR, Tillotson DL, Suter TM. Increased calcium loading and inotropy without greater cell death in hypoxic rat cardiomyocytes. Am J Physiol. (1998) 275(6 Pt 2):H2272–82. doi: 10.1152/ajpheart.1998.275.6.H2272
72. Timolati F, Anliker T, Groppalli V, Perriard J-C, Eppenberger HM, Suter TM, et al. The role of cell death and myofibrillar damage in contractile dysfunction of long-term cultured adult cardiomyocytes exposed to doxorubicin. Cytotechnology. (2009) 61:25–36. doi: 10.1007/s10616-009-9238-4
73. Sala L, van Meer BJ, Tertoolen LT, Bakkers J, Bellin M, Davis RP, et al. MUSCLEMOTION: a versatile open software tool to quantify cardiomyocyte and cardiac muscle contraction in vitro and in vivo. Circ Res. (2017) 122:e5–16. doi: 10.1161/CIRCRESAHA.117.312067
74. Laurila E, Ahola A, Hyttinen J, Aalto-Setälä K. Methods for in vitro functional analysis of iPSC derived cardiomyocytes - Special focus on analyzing the mechanical beating behavior. Biochim Biophys Acta. (2016) 1863(7 Pt B):1864–72. doi: 10.1016/j.bbamcr.2015.12.013
75. Schulze ML, Lemoine MD, Fischer AW, Scherschel K, David R, Riecken K, et al. Dissecting hiPSC-CM pacemaker function in a cardiac organoid model. Biomaterials. (2019) 206:133–45. doi: 10.1016/j.biomaterials.2019.03.023
76. Bürgel SC, Diener L, Frey O, Kim J-Y, Hierlemann A. Automated, multiplexed electrical impedance spectroscopy platform for continuous monitoring of microtissue spheroids. Anal Chem. (2016) 88:10876–83. doi: 10.1021/acs.analchem.6b01410
77. Bartholomä P, Gorjup E, Monz D, Reininger-Mack A, Thielecke H, Robitzki A. Three-dimensional in vitro reaggregates of embryonic cardiomyocytes: a potential model system for monitoring effects of bioactive agents. J Biomol Screen. (2005) 10:814–22. doi: 10.1177/1087057105280070
78. Frey U, Sanchez-Bustamante CD, Ugniwenko T, Heer F, Sedivy J, Hafizovic S, et al. Cell recordings with a CMOS high-density microelectrode array. Conf Proc IEEE Eng Med Biol Soc. (2007) 2007:167–70. doi: 10.1109/IEMBS.2007.4352249
79. Zeng H, Roman MI, Lis E, Lagrutta A, Sannajust F. Use of FDSS/μCell imaging platform for preclinical cardiac electrophysiology safety screening of compounds in human induced pluripotent stem cell-derived cardiomyocytes. J Pharmacol Toxicol Methods. (2016) 81:217–22. doi: 10.1016/j.vascn.2016.05.009
80. Boutin ME, Voss TC, Titus SA, Cruz-Gutierrez K, Michael S, Ferrer M. A high-throughput imaging and nuclear segmentation analysis protocol for cleared 3D culture models. Sci Rep. (2018) 8:11135. doi: 10.1038/s41598-018-29169-0
81. Jahnke H-G, Steel D, Fleischer S, Seidel D, Kurz R, Vinz S, et al. A novel 3D label-free monitoring system of hES-derived cardiomyocyte clusters: a step forward to in vitro cardiotoxicity testing. (2013) 8:e68971. doi: 10.1371/journal.pone.0068971
82. Sirenko O, Hancock MK, Crittenden C, Hammer M, Keating S, Carlson CB, et al. Phenotypic assays for characterizing compound effects on induced pluripotent stem cell-derived cardiac spheroids. Assay Drug Dev Technol. (2017) 15:280–96. doi: 10.1089/adt.2017.792
83. Zuppinger C. Edge-detection for contractility measurements with cardiac spheroids. In: Clements M, Roquemore L, editors. Stem Cell-Derived Models in Toxicology. New York, NY: Humana Press (2017) p. 211–27. doi: 10.1007/978-1-4939-6661-5_11
84. Lemme M, Ulmer BM, Lemoine MD, Zech ATL, Flenner F, Ravens U, et al. Atrial-like engineered heart tissue: an in vitro model of the human atrium. Stem Cell Rep. (2018) 11:1378–90. doi: 10.1016/j.stemcr.2018.10.008
85. Simian M, Bissell MJ. Organoids: a historical perspective of thinking in three dimensions. J Cell Biol. (2017) 216:31–40. doi: 10.1083/jcb.201610056
86. Horvath P, Aulner N, Bickle M, Davies AM, Nery ED, Ebner D, et al. Screening out irrelevant cell-based models of disease. Nat Rev Drug Discov. (2016) 15:751–69. doi: 10.1038/nrd.2016.175
87. Antoni D, Burckel H, Josset E, Noel G. Three-dimensional cell culture: a breakthrough in vivo. Int J Mol Sci. (2015) 16:5517–27. doi: 10.3390/ijms16035517
88. Kim J-Y, Fluri DA, Marchan R, Boonen K, Mohanty S, Singh P, et al. 3D spherical microtissues and microfluidic technology for multi-tissue experiments and analysis. J Biotechnol. (2015) 205:24–35. doi: 10.1016/j.jbiotec.2015.01.003
89. Haisler WL, Timm DM, Gage JA, Tseng H, Killian TC, Souza GR. Three-dimensional cell culturing by magnetic levitation. Nat Protoc. (2013) 8:1940–9. doi: 10.1038/nprot.2013.125
Keywords: 3D cell culture, induced pluripotent stem cells, cardiomyocyte, spheroid, engineered heart tissue, scaffold, high content screening
Citation: Zuppinger C (2019) 3D Cardiac Cell Culture: A Critical Review of Current Technologies and Applications. Front. Cardiovasc. Med. 6:87. doi: 10.3389/fcvm.2019.00087
Received: 19 December 2018; Accepted: 10 June 2019;
Published: 26 June 2019.
Edited by:
Maurizio Pesce, Centro Cardiologico Monzino (IRCCS), ItalyReviewed by:
Federico Quaini, University of Parma, ItalyAntonio Paolo Beltrami, University of Udine, Italy
Copyright © 2019 Zuppinger. This is an open-access article distributed under the terms of the Creative Commons Attribution License (CC BY). The use, distribution or reproduction in other forums is permitted, provided the original author(s) and the copyright owner(s) are credited and that the original publication in this journal is cited, in accordance with accepted academic practice. No use, distribution or reproduction is permitted which does not comply with these terms.
*Correspondence: Christian Zuppinger, Y2hyaXN0aWFuLnp1cHBpbmdlckBkYm1yLnVuaWJlLmNo