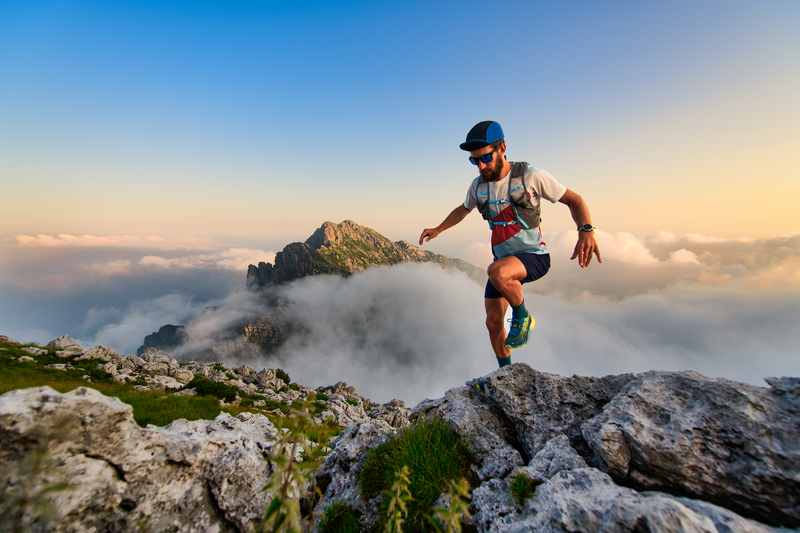
95% of researchers rate our articles as excellent or good
Learn more about the work of our research integrity team to safeguard the quality of each article we publish.
Find out more
MINI REVIEW article
Front. Cardiovasc. Med. , 25 September 2018
Sec. Atherosclerosis and Vascular Medicine
Volume 5 - 2018 | https://doi.org/10.3389/fcvm.2018.00130
This article is part of the Research Topic Vascular Smooth Muscles and Endothelial Cells in Atherosclerosis View all 4 articles
Seminal studies from Nikolai Anichckov identified the accumulation of cholesterol in the arteries as the initial event that lead to the formation of atherosclerotic plaques. Further studies by Gofman and colleagues demonstrated that high levels of circulating low-density lipoprotein cholesterol (LDL-C) was responsible for the accelerated atherosclerosis observed in humans. These findings were confirmed by numerous epidemiological studies which identified elevated LDL-C levels as a major risk factor for cardiovascular disease. LDL infiltrates in the arterial wall and interacts with the proteoglycan matrix promoting the retention and modification of LDL to a toxic form, which results in endothelial cell (EC) activation and vascular inflammation. Despite the relevance of LDL transport across the endothelium during atherogenesis, the molecular mechanism that control this process is still not fully understood. A number of studies have recently demonstrated that low density lipoprotein (LDL) transcytosis across the endothelium is dependent on the function of caveolae, scavenger receptor B1 (SR-B1), activin receptor-like kinase 1 (ALK1), and LDL receptor (LDLR), whereas high-density lipoproteins (HDL) and its major protein component apolipoprotein AI transcytose ECs through SR-B1, ATP-Binding cassette transporter A1 (ABCA1) and ABCG1. In this review article, we briefly summarize the function of the EC barrier in regulating lipoprotein transport, and its relevance during the progression of atherosclerosis. A better understanding of the mechanisms that mediate lipoprotein transcytosis across ECs will help to develop therapies targeting the early events of atherosclerosis and thus exert potential benefits for treating atherosclerotic vascular disease.
Atherosclerosis is a chronic inflammatory process involving complex interactions of normal and modified lipoproteins, monocytes, macrophage-foam cells, T lymphocytes, endothelial cells (ECs), smooth muscle cells, and fibroblasts. The transendothelial transport of apoB-lipoproteins plays a pivotal role in the pathogenesis of atherosclerosis. According to the “infiltration theory,” the development of atherosclerosis is triggered by the entry and subendothelial retention of lipoprotein from the bloodstream, particularly low density lipoprotein (LDL), and apolipoprotein-B (apoB)-containing remnants within the arterial wall (1). HDL must also cross the endothelial barrier in the arterial wall to exert its athero-protective properties mediating cholesterol efflux from lipid laden macrophages. Increased cholesterol influx relative to efflux through ECs and enhanced binding to extracellular matrix result in the retention of both pro-atherogenic (apoB-lipoproteins) and anti-atherogenic lipoproteins (HDL) in atherosclerotic arteries (2). Although, lipoprotein transport is a critical for the initiation and progression of atherosclerosis, the fluxes of lipoproteins into and out of the artery wall have not been completely investigated and the mechanisms by which LDL and HDL enters into the subendothelial space remains unclear.
Arteries and veins are composed of various layers of smooth muscle cells, connective tissue, and a thin single sheet of ECs. The endothelium forms a barrier due to the presence of specialized cell-to-cell junctions which selectively regulate the passage of molecules and cells between the bloodstream and tissues by the paracellular route (3). The EC barrier is involved in many systemic processes including vascular tone, fluid homeostasis and host defense. The endothelium is permeable to water and small molecules with a diameter below 6 nm, but nearly impermeable to macromolecules with different endothelia endowed with unique “perm-selectivity” (4). The transport of macromolecules, including lipoproteins, across the endothelium is actively controlled by ECs via the transcellular route, or transcytosis (5, 6). The process of transcytosis involves fluid phase or receptor-mediated ligand uptake by endocytosis, transition of the cargo through the cytoplasm, and exocytic release of the cargo (7).
The transcytosis can be separated into indirect and direct pathways dependent on their transportation routes. In the case of the indirect transport pathway, molecules are endocytosed into early endosomes, transferred to recycling endosomes, and exocytosed on the opposite side of EC layer (8). The indirect transcytosis routes are also receptor-specific, and rely on the interaction of molecules and their endogenous receptors. For example, LDL can transcytose across the EC layer by binding to LDL receptor (LDLR) or activin-like kinase 1 (ALK1) protein, which will be described in more detail in section Transcytosis of LDL in EC of this review article (9, 10). The direct transcytosis route involves the direct transport of the molecules from the incoming side to the opposite side, which in turn fuse with the basolateral aspects of the plasma membrane (8). Caveolae-mediated transport in endothelia is the most common route of direct transcytosis. Besides the receptor-specific indirect transcytosis and direct transcytosis routes, proteins or other macromolecules can be transported via nonspecifically binding to membranes through electrostatic interaction and fluid-phase transcytosis (11).
LDL particles contain one single apolipoprotein B-100 (apoB-100) molecule and carry the majority of the cholesterol in circulation. Elevated circulating LDL levels are highly related to the development of atherosclerosis and coronary heart disease. Mutations of LDLR cause an autosomal dominant disorder, familial hypercholesterolemia (FH), which is characterized by elevated plasma concentrations of LDL cholesterol and early coronary heart disease (12). During the initial stages of atherosclerosis, LDL particles are transported across the EC barrier and accumulate in the subendothelial space. The trapped LDL molecules are oxidized to form oxidized LDL (oxLDL), which facilitates the uptake of these modified lipoproteins by the scavenger receptors expressed in macrophages. This promotes the formation of macrophage foam cells within atherosclerotic lesions (13). Early electron microscopy studies examining LDL transport across the endothelium documented the uptake of LDL via two routes: a saturable, clathrin-mediated endocytic mechanism via LDLR; and fluid-phase non-saturable transcytotic mechanism through non-coated plasmalemmal vesicles, perhaps caveolae (14). In this section, we will review the receptors that have been associated with the transport of LDL across the endothelium and the relevance of caveolae in this process (Figure 1).
Figure 1. Schematic of low-density lipoprotein (LDL) transcytosis by ECs. Water and small molecules with a diameter below 6 nm are transported across endothelial cells by the paracellular route. The classical LDL receptor (LDLR) pathway mediates LDL uptake and its degradation in the lysosomes, which is not essential for transcytosis. LDL can transpose ECs through receptor-mediated transcytosis associated with scavenger receptor B1 (SR-B1), activin receptor-like kinase 1 (ALK1), or LDL receptor (LDLR, only occurred in brain ECs), as well as caveolae-mediated direct transcytosis. The endocytosed LDL particles are then transferred to the opposite side of the cell directly (caveolae-mediated transcytosis) or indirectly (receptor-mediated transcytosis) and exocytosed to subendothelial space.
The endocytosis of LDL by LDLR has been extensively characterized (15). ApoB in LDL particles is recognized by LDLR, promoting LDL uptake into the cells. The internalized particles are routed to endosomes, where LDL dissociates from LDLR because of the low PH in endosomal lumen. Dissociated LDL is transferred to late endosomes and lysosomes for further degradation, whereas LDLR is recycled back to the plasma membrane. Besides to its function in LDL for utilization and clearance for peripheral tissues, LDLR has been reported to mediate LDL transcytosis in the blood-brain barrier (BBB) (16). An antibody known to interact with the LDLR-binding domain inhibited the transcytosis of LDL, and the LDL transcytosis paralleled the increase in LDLR expression. This suggests that LDL is transcytosed by a receptor-mediated mechanism (16). The non-degradation of the LDL during transcytosis indicates that the transcytotic pathway in brain capillary endothelial cells is different from the classical LDL receptor pathway of endocytosis (16). However, this is unlikely to be the case in ECs of systemic circulation, whereas PCSK9-mediated LDLR degradation has no effect on LDL transcytosis (17), indicating that the transcytosis process in ECs of systemic circulation is LDLR-independent.
SR-B1 is well-known as high affinity HDL receptor which mediates the selective uptake of HDL cholesterol ester into the liver and the bidirectional flux of free cholesterol between cells and HDL (18, 19). Recent studies have revealed an unexpected role of SR-B1 in regulating LDL transcytosis in ECs (9, 17). Lee et al. using a novel total internal reflection fluorescence (TIRF) microscopy approach, demonstrated that SR-B1 silencing significantly attenuates LDL transcytosis in human coronary ECs (17). These results were further supported in vivo by assessing the accumulation of fluorescence-labeled LDL (DiI-LDL) in aortas isolated from WT and SR-B1 deficient mice. The authors found a significant reduction in LDL infiltration into the subendothelial space in aortas from SR-B1-deficient mice perfused ex vivo with DiI-LDL (17). Similar findings were observed by Sessa and collaborators, who identified SR-B1 as a relevant receptor that controls LDL uptake in ECs using a genome-wide RNAi screening strategy (9). Mechanistically, it is not clear how SR-B1 mediates LDL transport since there is no data showing that it directly binds apoB on LDL. However, it is feasible that SR-B1 is part of a complex that facilitates LDL uptake through additional pathways. Specific expression of SR-B1 in endothelial cells showed 37% less aortic lesions compared to control mice which was attributed to decreased plasma cholesterol and increased HDL levels (20), however, the contribution of SR-B1 in lipoprotein transcytosis on the development of atherosclerosis is still unclear.
In addition to SR-B1, we have identified ALK1 as a novel low-affinity, high-capacity receptor for LDL in EA.hy926 cells, an immortalized human endothelial-like cell (9). ALK1 is an EC-restricted TGF-β-type 1 receptor with high affinity to the bone morphogenetic protein (BMP) 9 and 10 ligands (21). By knockdown or overexpression studies, ALK1 was found to mediate LDL transcytosis independent of its kinase activity and that ALK1 can directly bind LDL (9). Moreover, the specific deletion of ALK1 in the endothelium significantly reduced DiI-LDL uptake into the aortic endothelium by en face confocal imaging of isolated vessels (9). Interestingly, ALK1-dependent uptake of LDL does not result in its lysosomal degradation, implying a distinct internalization pathway from LDLR (9).
Caveolae are small bulb-shaped plasma membrane invaginations present in most cell types with ~50–80 nm in diameter (22). Caveolin-1 (Cav-1) is the major structural protein essential to the formation of the caveolae in endothelial cells (23), which have been implicated in various physiological and pathological contexts based on their cellular functions in lipid homeostasis, signal transduction and endocytosis (24–26). We and others have demonstrated that the absence of Cav-1 protects mice against the progression of atherosclerosis (25, 27, 28). Importantly, re-expression of Cav-1 in ECs attenuates this effect and promotes lesion expansion (25). Mechanistically, we demonstrated that genetic ablation of Cav-1 significantly impairs LDL transport and retention in the arterial wall (25). Similar findings were observed by Lisanti and colleagues, who found a significant reduction in radioactively-labeled LDL accumulation in mice lacking Cav-1 (29). These findings suggest that caveolae plays a relevant role as a major regulator of LDL entry into the vessel wall and participates initiation of atherosclerosis. Moreover these findings reinforce the original observations identifying non-coated plasmalemmal vesicles as caveolae, and the major entry pathway for with LDL (14). Importantly, SR-B1 and ALK1 are located in caveolae, suggesting that both receptors might promote specific LDL binding and loading to caveolae facilitating the transport of LDL across the endothelium (30, 31). Additional experiments will be important to dissect the specific contribution of caveolae in SR-B1 and ALK1-mediated LDL transcytosis.
The protective effect of HDL is attributed to its ability in mediating reverse cholesterol transport (RCT) through which cholesterol is delivered from the periphery (such as arterial wall cells including lipid laden macrophages) back to the liver for biliary excretion (32, 33). To achieve the removal of excess cholesterol deposited in the atherosclerotic lesions, HDL must first cross the endothelial barrier to get access to macrophage foam cells in atherosclerotic plaques. The transcytosis of apolipoprotein A-I (apoAI), the major protein constituent of HDL, from the apical to the basolateral compartment was observed in ECs with minor amounts of the internalized apoAI degraded (34). The mechanism of apoAI transcytosis through ECs were further confirmed by the same group, who showed that apoAI is colocalized with ATP-Binding cassette transporter A1 (ABCA1) and that pharmacological intervention or RNA interference of ABCA1, but not SR-B1, modulated the transcytosis of apoAI through ECs (35). However, the endothelial transcytosis of mature HDL is different from that of apoAI, as it is dependent on SR-B1 and ABCG1 but not ABCA1 (36). Interestingly, the transcytosis of LDL mediated by SR-B1 appears to be regulated by (VEGF)-A, linking vascular permeability with enhance LDL transcytosis via SR-B1 (37). In addition, the transcytosis of HDL in brain microvascular ECs was demonstrated to be partially SR-B1-dependent and inhibition of Cav-1, or clathrin and adaptor protein PDZ Domain Containing 1 (PDZK1) had no impact on the HDL transcytosis (38).
Delivery of excess cholesterol from peripheral tissues and cells to the bloodstream by HDL is the initial steps in RCT process (32, 33). The lymphatic system is considered to be the primary location for the return of lipoproteins from the interstitial space to circulation (39). It has been shown that lymphatic transport of cholesterol by HDL is mediated via SR-B1 expressed on lymphatic endothelium using silencing RNA interference, neutralizing antibody, and transgenic mice (40–42). The specific function of SR-B1 is dependent on the uptake and transcytosis of HDL in lymphatic endothelial cells (40–42). Importantly, SR-B1 was found to be present in both the basolateral and apical membranes of ECs, but greater cellular uptake of cholesterol from HDL was found in the basolateral compartment (20). Enhanced expression of SR-B1 in ECs resulted in decreased atherosclerosis, supporting a possible role for endothelial SR-B1 in the flux of cholesterol across ECs (20).
Immunohistochemical studies have shown a partial co-localization of DiI-labeled HDL in caveolae and gold-labeled HDL with Cav-1, but the potential role of Cav-1/caveolae on HDL trafficking in ECs remains poorly understood (43). Caveolae have been also implicated in the regulation of cellular cholesterol homeostasis and a number of cholesterol-trafficking steps (44–46). The reconstitution of purified Cav-1 only with cholesterol-containing lipid vesicles revealed the first direct link between Cav-1 and cholesterol (47). A series of studies by Fielding and Fielding suggest that caveolae may act as portals for cholesterol efflux upon incubation of cells with HDL (44, 48, 49). These data was further supported by adenovirus-mediated overexpression of Cav-1 in the mouse liver, caused an increase in plasma HDL-cholesterol (45, 50, 51). Cav-1/caveolae may also regulate the activity of scavenger receptors and ATP-binding cassette transporters (ABC)s, that control the cholesterol homeostasis in ECs. While these studies suggest an important role for caveolae in regulating HDL transport, cholesterol efflux, and hepatic HDL biogenesis, a recent study showed that HDL internalized by ECs did not colocalize with clathrin or Cav-1 and is independent of fluid phase uptake (52). Instead, HDL appeared to be internalized and trafficked by ECs through a non-classical endocytic route involving dynamin and cytoskeletal networks (52). The mechanisms of caveolae and Cav-1 involved in HDL transcytosis in ECs require further study.
The subendothelial accumulation of pro-atherogenic lipoproteins including LDL represents a pivotal step in the initiation of atherosclerosis (53). On the contrary, removal of cholesterol from the subendothelial space to the circulation by HDL-mediated reverse cholesterol transport represents a relevant anti-atherogenic pathway (53). Endothelial transcytosis is considered to be a process that involves endocytosis, vehicle traffic through the cytoplasm and exocytic release of the cargo. So far, most of the studies on endothelial transcytosis have focused on the endocytosis step. The LDL transcytosis from the apical to the basolateral compartment in ECs is dependent on the function of caveolae, SR-B1, or ALK1 (Figure 1). ECs are quite heterogeneous depending on the tissue bed (54). LDLR-mediated LDL transcytosis only occurred in brain ECs, but not in ECs from system circulation. Unlike LDL, the transcytosis of HDL across ECs has been less investigated. SR-B1 is suggested to be involved in the dual transport of HDL between the bloodstream and peripheral tissues, whereas the role of other molecules including caveolae, ABCA1 and ABCG1 need to be further investigated.
The investigation of endothelial transcytosis of lipoproteins is hampered by limitations in our ability to observe or monitor the transcytosis process. The technological advances of an in vitro assay for endothelial transcytosis, by the continuing availability of super-resolution microscopy and live-cell imaging techniques, will help facilitate the delineation of the mechanisms and molecular regulation of endothelial transcytosis (17, 38). In addition, most of the present findings were investigated by in vitro assays, the observation of lipoprotein transcytosis through ECs in vivo are needed to prove the physiological relevance. Furthermore, the internalization step in endothelial lipoprotein transcytosis has been relatively well investigated, however, the regulation of intracellular vehicle traffic and exocytosis on the other side of cells remains poorly understood. The question of whether the composition of lipoproteins is altered during the process of endothelial transcytosis needs to be answered.
Numerous drug carriers targeting endothelial transcytosis, such as the caveolae-dependent pathway, have been developed for the treatment of cancer and lung injury patients (55). However, the precise control of lipoprotein transcytosis in ECs requires deeper understanding of the mechanisms and regulations involved in this process. For example, the dual role of SR-B1 in both LDL and HDL transcytosis raises a question of how to balance these different pathways to protect against atherogenesis. A better understanding of the mechanisms that mediate lipoprotein transcytosis across ECs may help to develop therapies targeting on the early events of atherosclerosis and thus exert potential benefits to the treatment of atherosclerotic vascular disease.
XZ, WCS, and CF-H drafted, edited, and approved the manuscript and figures.
The authors declare that the research was conducted in the absence of any commercial or financial relationships that could be construed as a potential conflict of interest.
This work was supported by grants from the National Institutes of Health (R35HL135820 to CF-H; and R35 HL139945 to WCS), the American Heart Association (16EIA27550005 to CF-H), and the Foundation Leducq Transatlantic Network of Excellence in Cardiovascular Research MIRVAD (to WCS and CF-H).
2. Nordestgaard BG. The vascular endothelial barrier–selective retention of lipoproteins. Curr Opin Lipidol. (1996) 7:269–73.
3. Rahimi N. Defenders and challengers of endothelial barrier function. Front Immunol. (2017) 8:1847. doi: 10.3389/fimmu.2017.01847
4. Santos JM, Graindorge A, Soldati-Favre D. New insights into parasite rhomboid proteases. Mol Biochem Parasitol. (2012) 182:27–36. doi: 10.1016/j.molbiopara.2011.11.010
5. Mehta D, Malik AB. Signaling mechanisms regulating endothelial permeability. Physiol Rev. (2006) 86:279–367. doi: 10.1152/physrev.00012.2005
6. Minshall RD, Malik AB. Transport across the endothelium: regulation of endothelial permeability. Handb Exp Pharmacol. (2006) (176 Pt 1):107–44. doi: 10.1007/3-540-32967-6_4
7. Fung KYY, Fairn GD, Lee WL. Transcellular vesicular transport in epithelial and endothelial cells: challenges and opportunities. Traffic (2018) 19:5–18. doi: 10.1111/tra.12533
8. Thuenauer R, Muller SK, Romer W. Pathways of protein and lipid receptor-mediated transcytosis in drug delivery. Expert Opin Drug Deliv. (2017) 14:341–51. doi: 10.1080/17425247.2016.1220364
9. Kraehling JR, Chidlow JH, Rajagopal C, Sugiyama MG, Fowler JW, Lee MY, et al. Genome-wide RNAi screen reveals ALK1 mediates LDL uptake and transcytosis in endothelial cells. Nat Commun. (2016) 7:13516. doi: 10.1038/ncomms13516
10. Goldstein JL, Brown MS, Anderson RG, Russell DW, Schneider WJ. Receptor-mediated endocytosis: concepts emerging from the LDL receptor system. Annu Rev Cell Biol. (1985) 1:1–39. doi: 10.1146/annurev.cb.01.110185.000245
11. Herve F, Ghinea N, Scherrmann JM. CNS delivery via adsorptive transcytosis. AAPS J. (2008) 10:455–72. doi: 10.1208/s12248-008-9055-2
12. Bourbon M, Alves AC, Sijbrands EJ. Low-density lipoprotein receptor mutational analysis in diagnosis of familial hypercholesterolemia. Curr Opin Lipidol. (2017) 28:120–9. doi: 10.1097/MOL.0000000000000404
13. Tabas I, Garcia-Cardena G, Owens GK. Recent insights into the cellular biology of atherosclerosis. J Cell Biol. (2015) 209:13–22. doi: 10.1083/jcb.201412052
14. Vasile E, Simionescu M, Simionescu N. Visualization of the binding, endocytosis, and transcytosis of low-density lipoprotein in the arterial endothelium in situ. J Cell Biol. (1983) 96:1677–89.
15. Goldstein JL, Brown MS. The LDL receptor. Arterioscler Thromb Vasc Biol. (2009) 29:431–8. doi: 10.1161/ATVBAHA.108.179564
16. Dehouck B, Fenart L, Dehouck MP, Pierce A, Torpier G, Cecchelli R. A new function for the LDL receptor: transcytosis of LDL across the blood-brain barrier. J Cell Biol. (1997) 138:877–89.
17. Armstrong SM, Sugiyama MG, Fung KY, Gao Y, Wang C, Levy AS, et al. A novel assay uncovers an unexpected role for SR-BI in LDL transcytosis. Cardiovasc Res. (2015) 108:268–77. doi: 10.1093/cvr/cvv218
18. Acton S, Rigotti A, Landschulz KT, Xu S, Hobbs HH, Krieger M. Identification of scavenger receptor SR-BI as a high density lipoprotein receptor. Science (1996) 271:518–20.
19. Yancey PG, de la Llera-Moya M, Swarnakar S, Monzo P, Klein SM, Connelly MA, et al. High density lipoprotein phospholipid composition is a major determinant of the bi-directional flux and net movement of cellular free cholesterol mediated by scavenger receptor BI. J Biol Chem. (2000) 275:36596–604. doi: 10.1074/jbc.M006924200
20. Vaisman BL, Vishnyakova TG, Freeman LA, Amar MJ, Demosky SJ, Liu C, et al. Endothelial expression of scavenger receptor class B, type I Protects against development of atherosclerosis in mice. Biomed Res Int. (2015) 2015:607120. doi: 10.1155/2015/607120
21. David L, Mallet C, Mazerbourg S, Feige JJ, Bailly S. Identification of BMP9 and BMP10 as functional activators of the orphan activin receptor-like kinase 1 (ALK1) in endothelial cells. Blood (2007) 109:1953–61. doi: 10.1182/blood-2006-07-034124
22. Chidlow JH Jr, Sessa WC. Caveolae, caveolins, and cavins: complex control of cellular signalling and inflammation. Cardiovasc Res. (2010) 86:219–25. doi: 10.1093/cvr/cvq075
23. Rothberg KG, Heuser JE, Donzell WC, Ying YS, Glenney JR, Anderson RG. Caveolin, a protein component of caveolae membrane coats. Cell (1992) 68:673–82.
24. Drab M, Verkade P, Elger M, Kasper M, Lohn M, Lauterbach B, Menne J, et al. Loss of caveolae, vascular dysfunction, and pulmonary defects in caveolin-1 gene-disrupted mice. Science (2001) 293:2449–52. doi: 10.1126/science.1062688
25. Fernandez-Hernando C, Yu J, Suarez Y, Rahner C, Davalos A, Lasuncion MA, Sessa WC. Genetic evidence supporting a critical role of endothelial caveolin-1 during the progression of atherosclerosis. Cell Metab. (2009) 10:48–54. doi: 10.1016/j.cmet.2009.06.003
26. Lamaze C, Tardif N, Dewulf M, Vassilopoulos S, Blouin CM. The caveolae dress code: structure and signaling. Curr Opin Cell Biol. (2017) 47:117–25. doi: 10.1016/j.ceb.2017.02.014
27. Engel D, Beckers L, Wijnands E, Seijkens T, Lievens D, Drechsler M, et al. Caveolin-1 deficiency decreases atherosclerosis by hampering leukocyte influx into the arterial wall and generating a regulatory T-cell response. FASEB J. (2011) 25:3838–48. doi: 10.1096/fj.11-183350
28. Frank PG, Lee H, Park DS, Tandon NN, Scherer PE, Lisanti MP. Genetic ablation of caveolin-1 confers protection against atherosclerosis. Arterioscler Thromb Vasc Biol. (2004) 24:98–105. doi: 10.1161/01.ATV.0000101182.89118.E5
29. Frank PG, Pavlides S, Lisanti MP. Caveolae and transcytosis in endothelial cells: role in atherosclerosis. Cell Tissue Res. (2009) 335:41–7. doi: 10.1007/s00441-008-0659-8
30. Babitt J, Trigatti B, Rigotti A, Smart EJ, Anderson RG, Xu S, Krieger M. Murine SR-BI, a high density lipoprotein receptor that mediates selective lipid uptake, is N-glycosylated and fatty acylated and colocalizes with plasma membrane caveolae. J Biol Chem. (1997) 272:13242–9.
31. Santibanez JF, Blanco FJ, Garrido-Martin EM, Sanz-Rodriguez F, del Pozo MA, Bernabeu C. Caveolin-1 interacts and cooperates with the transforming growth factor-beta type I receptor ALK1 in endothelial caveolae. Cardiovasc Res. (2008) 77:791–9. doi: 10.1093/cvr/cvm097
32. Rader DJ, Hovingh GK. HDL and cardiovascular disease. Lancet (2014) 384:618–25. doi: 10.1016/S0140-6736(14)61217-4
33. Rohatgi A, Khera A, Berry JD, Givens EG, Ayers CR, Wedin KE, et al. HDL cholesterol efflux capacity and incident cardiovascular events. N Engl J Med. (2014) 371:2383–93. doi: 10.1056/NEJMoa1409065
34. Rohrer L, Cavelier C, Fuchs S, Schluter MA, Volker W, von Eckardstein A. Binding, internalization and transport of apolipoprotein A-I by vascular endothelial cells. Biochim Biophys Acta (2006) 1761:186–94. doi: 10.1016/j.bbalip.2006.01.009
35. Cavelier C, Rohrer L, von Eckardstein A. ATP-Binding cassette transporter A1 modulates apolipoprotein A-I transcytosis through aortic endothelial cells. Circ Res. (2006) 99:1060–6. doi: 10.1161/01.RES.0000250567.17569.b3
36. Rohrer L, Ohnsorg PM, Lehner M, Landolt F, Rinninger F, von Eckardstein A. High-density lipoprotein transport through aortic endothelial cells involves scavenger receptor BI and ATP-binding cassette transporter G1. Circ Res. (2009) 104:1142–50. doi: 10.1161/CIRCRESAHA.108.190587
37. Velagapudi S, Yalcinkaya M, Piemontese A, Meier R, Norrelykke SF, Perisa D, et al. VEGF-A regulates cellular localization of SR-BI as well as transendothelial transport of HDL but not LDL. Arterioscler Thromb Vasc Biol. (2017) 37:794–803. doi: 10.1161/ATVBAHA.117.309284
38. Fung KY, Wang C, Nyegaard S, Heit B, Fairn GD, Lee WL. SR-BI Mediated transcytosis of HDL in brain microvascular endothelial cells is independent of caveolin, clathrin, and PDZK1. Front Physiol. (2017) 8:841. doi: 10.3389/fphys.2017.00841
39. Cooke CJ, Nanjee MN, Stepanova IP, Olszewski WL, Miller NE Variations in lipid and apolipoprotein concentrations in human leg lymph: effects of posture and physical exercise. Atherosclerosis (2004) 173:39–45. doi: 10.1016/j.atherosclerosis.2003.07.004
40. Fernandez-Hernando C. Lymphatic vessels clean up your arteries. J Clin Invest. (2013) 123:1417–9. doi: 10.1172/JCI68657
41. Lim HY, Thiam CH, Yeo KP, Bisoendial R, Hii CS, McGrath KC, et al. Lymphatic vessels are essential for the removal of cholesterol from peripheral tissues by SR-BI-mediated transport of HDL. Cell Metab. (2013) 17:671–84. doi: 10.1016/j.cmet.2013.04.002
42. Martel C, Li W, Fulp B, Platt AM, Gautier EL, Westerterp M, et al. Lymphatic vasculature mediates macrophage reverse cholesterol transport in mice. J Clin Invest. (2013) 123:1571–9. doi: 10.1172/JCI63685
43. Chao WT, Fan SS, Chen JK, Yang VC. Visualizing caveolin-1 and HDL in cholesterol-loaded aortic endothelial cells. J Lipid Res. (2003) 44:1094–9. doi: 10.1194/jlr.M300033-JLR200
44. Fielding PE, Fielding CJ. Plasma membrane caveolae mediate the efflux of cellular free cholesterol. Biochemistry (1995) 34:14288–92.
45. Fu Y, Hoang A, Escher G, Parton RG, Krozowski Z, Sviridov D. Expression of caveolin-1 enhances cholesterol efflux in hepatic cells. J Biol Chem. (2004) 279:14140–6. doi: 10.1074/jbc.M311061200
46. Li H, Chen W, Zhou Y, Abidi P, Sharpe O, Robinson WH, et al. Identification of mRNA binding proteins that regulate the stability of LDL receptor mRNA through AU-rich elements. J Lipid Res. (2009) 50:820–31. doi: 10.1194/jlr.M800375-JLR200
47. Murata M, Peranen J, Schreiner R, Wieland F, Kurzchalia TV, Simons K. VIP21/caveolin is a cholesterol-binding protein. Proc Natl Acad Sci USA. (1995) 92:10339–43.
49. Fielding CJ, Fielding PE. Cholesterol and caveolae: structural and functional relationships. Biochim Biophys Acta (2000) 1529:210–22. doi: 10.1016/S1388-1981(00)00150-5
50. Frank PG, Pedraza A, Cohen DE, Lisanti MP Adenovirus-mediated expression of caveolin-1 in mouse liver increases plasma high-density lipoprotein levels. Biochemistry (2001) 40:10892–900.
51. Moreno M, Molina H, Amigo L, Zanlungo S, Arrese M, Rigotti A, Miquel JF. Hepatic overexpression of caveolins increases bile salt secretion in mice. Hepatology (2003) 38:1477–88. doi: 10.1016/j.hep.2003.09.011
52. Perisa D, Rohrer L, Kaech A, von Eckardstein A. Itinerary of high density lipoproteins in endothelial cells. Biochim Biophys Acta (2016) 1861:98–107. doi: 10.1016/j.bbalip.2015.11.004
53. Glass CK, Witztum JL. Atherosclerosis the road ahead. Cell (2001) 104:503–16. doi: 10.1016/S0092-8674(01)00238-0
54. Aird WC. Endothelial cell heterogeneity. Cold Spring Harb Perspect Med. (2012) 2:a006429. doi: 10.1101/cshperspect.a006429
Keywords: transcytosis, lipoprotein, endothelial cell, atherosclerosis, LDL, HDL
Citation: Zhang X, Sessa WC and Fernández-Hernando C (2018) Endothelial Transcytosis of Lipoproteins in Atherosclerosis. Front. Cardiovasc. Med. 5:130. doi: 10.3389/fcvm.2018.00130
Received: 18 July 2018; Accepted: 03 September 2018;
Published: 25 September 2018.
Edited by:
Hong Chen, Harvard University, United StatesReviewed by:
Ying Hu Shen, Baylor College of Medicine, United StatesCopyright © 2018 Zhang, Sessa and Fernández-Hernando. This is an open-access article distributed under the terms of the Creative Commons Attribution License (CC BY). The use, distribution or reproduction in other forums is permitted, provided the original author(s) and the copyright owner(s) are credited and that the original publication in this journal is cited, in accordance with accepted academic practice. No use, distribution or reproduction is permitted which does not comply with these terms.
*Correspondence: William C. Sessa, d2lsbGlhbS5zZXNzYUB5YWxlLmVkdQ==
Carlos Fernández-Hernando, Y2FybG9zLmZlcm5hbmRlekB5YWxlLmVkdQ==
Disclaimer: All claims expressed in this article are solely those of the authors and do not necessarily represent those of their affiliated organizations, or those of the publisher, the editors and the reviewers. Any product that may be evaluated in this article or claim that may be made by its manufacturer is not guaranteed or endorsed by the publisher.
Research integrity at Frontiers
Learn more about the work of our research integrity team to safeguard the quality of each article we publish.