- 1Department of Bioengineering, University of Pittsburgh, Pittsburgh, PA, United States
- 2McGowan Institute for Regenerative Medicine, University of Pittsburgh, Pittsburgh, PA, United States
- 3Tissue Engineering Research Group, Department of Anatomy, Royal College of Surgeons in Ireland, Dublin, Ireland
- 4Department of Pathology, University of Pittsburgh, Pittsburgh, PA, United States
- 5Trinity Centre for Bioengineering, Trinity College Dublin, Dublin, Ireland
- 6Advanced Materials and Bioengineering Research Centre, Royal College of Surgeons in Ireland and Trinity College Dublin, Dublin, Ireland
- 7Department of Surgery, University of Pittsburgh, Pittsburgh, PA, United States
- 8Department of Cardiothoracic Surgery, University of Pittsburgh, Pittsburgh, PA, United States
- 9Department of Chemical and Petroleum Engineering, University of Pittsburgh, Pittsburgh, PA, United States
Vascular tissue engineering is an area of regenerative medicine that attempts to create functional replacement tissue for defective segments of the vascular network. One approach to vascular tissue engineering utilizes seeding of biodegradable tubular scaffolds with stem (and/or progenitor) cells wherein the seeded cells initiate scaffold remodeling and prevent thrombosis through paracrine signaling to endogenous cells. Stem cells have received an abundance of attention in recent literature regarding the mechanism of their paracrine therapeutic effect. However, very little of this mechanistic research has been performed under the aegis of vascular tissue engineering. Therefore, the scope of this review includes the current state of TEVGs generated using the incorporation of stem cells in biodegradable scaffolds and potential cell-free directions for TEVGs based on stem cell secreted products. The current generation of stem cell-seeded vascular scaffolds are based on the premise that cells should be obtained from an autologous source. However, the reduced regenerative capacity of stem cells from certain patient groups limits the therapeutic potential of an autologous approach. This limitation prompts the need to investigate allogeneic stem cells or stem cell secreted products as therapeutic bases for TEVGs. The role of stem cell derived products, particularly extracellular vesicles (EVs), in vascular tissue engineering is exciting due to their potential use as a cell-free therapeutic base. EVs offer many benefits as a therapeutic base for functionalizing vascular scaffolds such as cell specific targeting, physiological delivery of cargo to target cells, reduced immunogenicity, and stability under physiological conditions. However, a number of points must be addressed prior to the effective translation of TEVG technologies that incorporate stem cell derived EVs such as standardizing stem cell culture conditions, EV isolation, scaffold functionalization with EVs, and establishing the therapeutic benefit of this combination treatment.
Introduction
Vascular tissue engineering is an area of regenerative medicine that attempts to restore defective segments of the vascular network. One approach to vascular tissue engineering is to implant biodegradable tubular scaffolds seeded with appropriate cells. Research has focused on lining the lumen of the scaffold with endothelial progenitor cells (1–5), self-assembly of vascular grafts by in vitro culture of fused vascular cell sheets (6–12), seeding scaffolds with native vascular cells (13–16), progenitor cells pre-differentiated into vascular phenotypes (17–22) using biomechanical/biochemical stimuli [as reviewed in Maul et al. (23)], and pluripotent stem cells pre-differentiated into vascular phenotypes (24, 25). However, employing native vascular cells, terminally differentiated progenitor/pluripotent cells, or self-assembled cell sheets requires extended culture periods and the use of expensive culture media that is often derived from xenogeneic sources. Seeding biodegradable scaffolds with undifferentiated stem (and/or progenitor) cells initiates scaffold remodeling through paracrine signaling to endogenous cells (26, 27). Seeding vascular scaffolds with stem cells also bypasses many of the aforementioned limitations due to the fact that a sufficient number of implant-ready cells can be acquired from a single harvest, therefore eliminating the time and resources spent culturing or differentiating cells. The motivation for this review is that stem/progenitor cells have received an abundance of attention in recent literature regarding the mechanism of their paracrine therapeutic effect. However, this parallel research has yet to translate fully to the field of vascular tissue engineering. Therefore, the scope of this review includes the current state of TEVGs generated using the incorporation of stem cells in biodegradable scaffolds and potential cell-free directions for TEVGs based on stem cell secreted products (Figure 1).
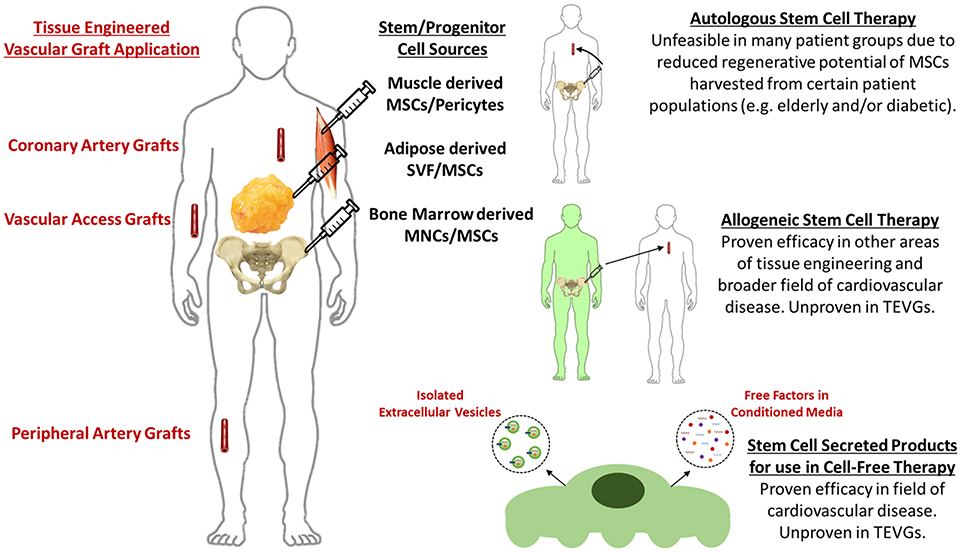
Figure 1. Current methods and future perspectives for stem cell-based tissue engineered vascular grafts.
Stem Cell Based TEVG Studies
Numerous studies have demonstrated that implanting biodegradable vascular scaffolds, seeded with stem cells from a variety of sources, triggers the development of functional, immuno-compatible, native-like vascular replacements (Table 1). Bone marrow mononuclear cells (BM-MNCs) have been employed in numerous preclinical (26, 28–31, 33, 36–38, 43, 44) and clinical studies (28, 32, 51, 52). BM-MNCs are a heterogeneous population comprised of mesenchymal stem cells (MSCs), endothelial precursor cells, mature endothelial cells, hematopoietic stem cells, monocytes, CD4+ T cells, CD8+ T cells, B cells, and natural killer cells (26). Recently, it has been shown that BM-MNCs have a dose dependent effect on scaffold development when implanted as an inferior vena cava interposition in a mouse model whereby increasing BM-MNC number increased graft patency and decreased the number of infiltrated macrophages (42). Purified MSCs have also been employed in vascular tissue engineering and are obtained from various sources. MSCs are adherent adult progenitor cells with the ability to self-renew and differentiate into a variety of cells with a more specialized function [as reviewed in Huang and Li (53)]. Furthermore, MSCs secrete a variety of angiogenic and arteriogenic growth factors and cytokines (as discussed in section Allogeneic MSCs). Recent literature suggests that MSCs could be renamed Medicinal Signaling Cells to emphasize that MSCs do not differentiate at the site of injury (and are therefore not true stem cells), but rather signal to endogenous cells to regenerate and/or replace the injured/absent tissue (54). Bone marrow derived MSCs (BM-MSCs), purified from BM-MNCs, have demonstrated favorable preclinical findings in TEVGs (45–47). Similarly, adipose derived MSCs (ADMSCs) (48, 55) and muscle derived MSCs (49, 56) have been used in TEVG studies. Studies employing pericytes are also included in this review (50) as they have been shown to express MSC markers and display the capacity for tri-lineage differentiation [as reviewed in Crisan et al. (57)].
Autologous Stem Cells
Numerous preclinical (28–31, 38) and clinical studies (32, 51, 52, 58) have used autologous stem cells as a cellular base for vascular scaffolds. Autologous stem cell studies have focused on restoring vascular integrity in pediatric/young patients with congenital heart defects and have demonstrated favorable long term clinical results (32). However, a combination of in vitro and in vivo studies has demonstrated the diminished regenerative potential of stem cells in vascular tissue engineering when harvested from elderly or diabetic patients (Figure 2). The ability of ADMSCs to prevent acute thrombosis and encourage graft remodeling in a murine model is reduced when cells are harvested from elderly or diabetic patient groups and seeded on a PEUU scaffold (48) using established methods (60, 61). Furthermore, the ability of ADMSCs from elderly or diabetic patients to encourage smooth muscle cell migration and secrete factors that promote fibrinolysis is also decreased (48, 59). The work of Krawiec et al. therefore highlights the limitations of an autologous stem cell approach as many of the patient groups in need of regenerative therapies are elderly and/or diabetic e.g., coronary/peripheral bypass patients and end stage renal disease patients. Furthermore, the diminished regenerative potential of elderly/diabetic stem cells may expand to include more degenerative conditions. However, the inherent limitations of an autologous stem cell approach can be largely bypassed by using allogeneic MSCs harvested from young healthy donors.
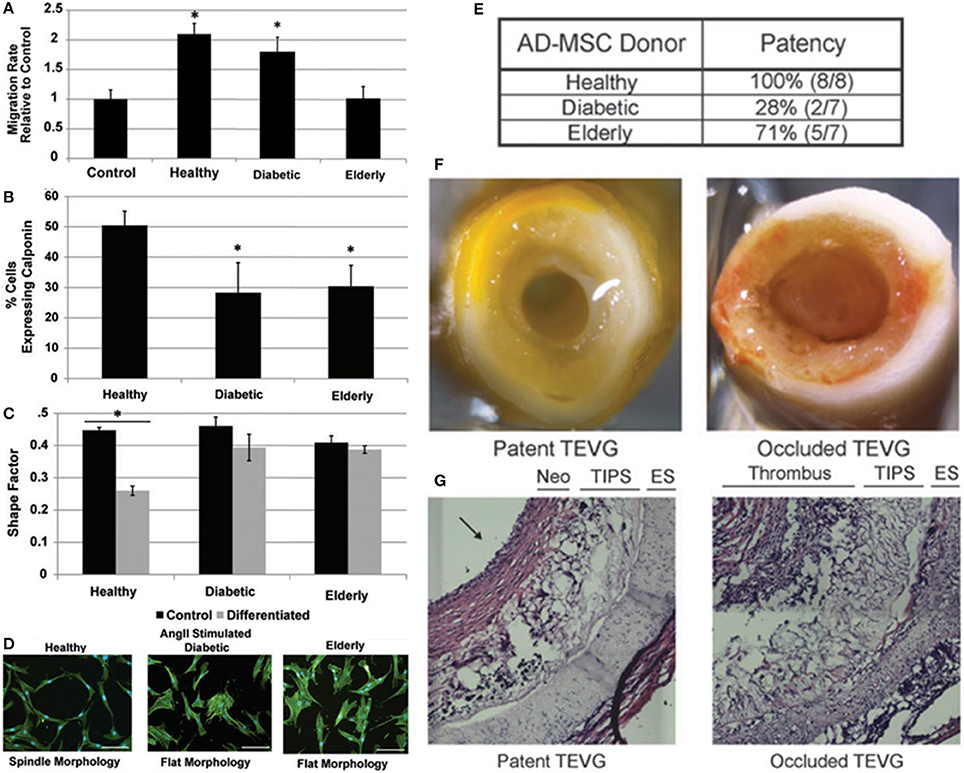
Figure 2. Combination of in vitro and in vivo studies that demonstrate the diminished regenerative potential of stem cells in vascular tissue engineering when harvested from elderly or diabetic patients. (A–D) The ability of ADMSCs from elderly or diabetic patients to encourage smooth muscle cell migration and secrete factors that promote fibrinolysis is decreased (48, 59). (E–G) The ability of ADMSCs to prevent acute thrombosis and encourage remodeling when seeded on a PEUU scaffold implanted in a murine model is reduced when harvested from elderly or diabetic patient groups (48). Adapted from Krawiec et al. (48, 59) with permission from Mary Ann Liebert, Inc.
Allogeneic MSCs
MSCs are an appropriate candidate for allogeneic stem cell therapies as they are immune evasive. MSC immune evasion can be partially attributed to their low expression of major histocompatibility complex (MHC) class I antigens and freedom from expression of MHC class II antigens which are both associated with immune rejection (62, 63). Similarly, the MSC secretome has been shown to suppress immune response by inhibiting T cell proliferation and monocyte maturation and also by promoting regulatory T cells and M2 macrophages (64). Although allogeneic MSCs have not yet been investigated as a cellular base for TEVGs, the use of allogeneic fibroblasts for generating vascular grafts by self-assembly has been proven safe for use in humans as arteriovenous fistulas following devitalization (12). The use of allogeneic fibroblasts highlights the premise of employing allogeneic cells in vascular tissue engineering. Furthermore, other fields of tissue engineering have employed allogeneic MSCs to safely and effectively regenerate bone (65–67), cartilage (68–70), skin (71, 72), and nerve (73).
Clinical studies of allogeneic MSCs in the broader field of cardiovascular disease have also presented convincing evidence to suggest that allogeneic MSCs are minimally immunogenic and induce an equivalent therapeutic response when compared to autologous MSCs. The POSEIDON randomized trial compared the safety and efficacy of allogeneic MSCs to autologous MSCs in patients with ischemic cardiomyopathy. The trial results found that transendocardial delivery of allogeneic MSCs did not stimulate significant donor-specific immune reactions and was also associated with a reduction in left ventricle volume and an increase in ejection fraction comparable to treatment with autologous MSCs (74). It has also been demonstrated that transendocardial injections of allogeneic MSCs produce a dose dependent reduction in major adverse events in chronic heart failure patients (75). Furthermore, adventitial administration of commercially available allogeneic MSCs to the coronary arteries of myocardial infarction (MI) patients showed that allogeneic MSCs were well tolerated, with no serious adverse events, and significantly increased both ejection fraction and ventricular stroke volume (76). The safety and therapeutic efficacy of administering allogeneic MSCs to treat MI has also been demonstrated separately in both large (77) and small animal preclinical models (78). Combined, the preceding evidence supports the premise of employing allogeneic vascular cells as a cellular base for developing TEVGs and also the safety and efficacy of administering allogeneic MSCs to treat cardiovascular conditions. The use of allogeneic MSCs is therefore one potential future direction for stem cell based TEVGs that has yet to be fully investigated.
Remodeling Process of Stem Cell Seeded Vascular Scaffolds
Despite the great success of directly incorporating stem cells in vascular tissue engineered scaffolds, evidence supporting a paracrine mechanism as the main effector of stem cell therapy indicates the potential of employing stem cell secreted products as a more straightforward, cell-free therapeutic base for tissue engineering (Figure 1). Compelling evidence for the paracrine effect of stem cells in vascular tissue engineering is that remodeling of implanted vascular scaffolds is mediated by an inflammatory process (26, 27, 42), and that seeded stem cells signal the recruitment and moderation of the immune cells that trigger the required inflammatory process in a paracrine manner (26, 27, 35).
The role of inflammation in vascular graft remodeling was initially demonstrated through observations that host monocyte and macrophage infiltration precedes the repopulation of scaffolds with vascular cells (26). Subsequently, it was demonstrated that peak macrophage infiltration coincides with the formation of functional vascular tissue and that depleting the host of macrophages completely inhibits the formation of neo-tissue (27). Furthermore, the role of modulating host immune cells was demonstrated by preventing host monocytes from secreting pro-inflammatory factors, through inhibition of TGF-β receptor 1, which significantly increased unseeded scaffold patency relative to untreated controls (79). Functionalization of vascular scaffolds to locally release TGF-β1 inhibitor was proven to be as effective as seeding BM-MNCs in promoting graft patency (41). Therefore, both recruitment and modulation of host immune cells are required to ensure the formation of a functional neo-vessel.
Evidence for the paracrine role of seeded stem cells in vascular scaffold remodeling is that seeded BM-MNCs reside in the scaffold for <7 days in vivo and are not incorporated into the developing neo-vessel (26, 35). Rather, the transient presence of BM-MNCs significantly increases the recruitment of host immune cells (monocytes and macrophages) compared to unseeded controls, partially through the secretion of MCP-1 (26). Subsequently, functionalization of vascular scaffolds to locally release MCP-1 was proven to be significantly more effective than seeded BM-MNCs in recruiting host monocytes (26). Furthermore, BM-MNCs have been shown to suppress the expression of M1 macrophage phenotype, the presence of which has been shown to decrease graft patency and remodeling (27). Seeded stem cells therefore modulate both the infiltration and phenotype of the host immune cells that mediate the vascular remodeling process in a paracrine manner through the secretion of bioactive products.
The concept of moving toward a cell-free approach by employing stem cell secreted products is to preserve the formation of neo-tissue while also removing the potential safety, regulatory, and practicality issues of cellular incorporation such as the use of stem cells with damaged/mutated DNA, undesirable trans-differentiation of persistent stem cells, and micro-vessel clotting in the case of stem cell relocation (80). It is important to note that TEVGs formed using cell secreted products may be better described as Tissue Regenerative Vascular Grafts to reflect the departure from the traditional cell based paradigm.
MSC Secreted Factors/Conditioned Media
Stem cells, particularly MSCs, secrete a range of bioactive products that have an indirect or trophic effect on surrounding cells (81) and it has been proposed that these MSC-secreted bioactive products could replace MSCs as a therapeutic base for cell-free vascular tissue engineering (26, 41). One such manner of moving toward a cell-free approach is to utilize MSC conditioned media (MSC-CM) as it has been frequently demonstrated that MSCs secrete trophic factors into their surrounding media. However, the use of MSC-CM in vascular tissue engineering has demonstrated poor initial results. Scaffolds incubated over-night in BM-MNC-CM, following over-night incubation of the cells in serum-free media at 5% O2, exhibited poor patency rates in vivo which were comparable to PBS incubated scaffolds (patent: 2/10 vs. 6/25) (43).
Despite the discouraging results observed by Best et al, free injections of MSC-CM to treat MI have exhibited pre-clinical success. Intracardial administration of CM from BM-MSCs, under hypoxic conditions, and overexpressing the survival gene Akt1, significantly decreased infarct size and apoptosis in a murine model of MI (82). Intravenous and intracoronary administration of CM harvested from human embryonic stem cell (hESC) derived MSC was associated with a 60% reduction in infarct size, improvements in systolic and diastolic cardiac performance and increased capillary density in an infarct porcine model (83, 84). Furthermore, co-administration of MSC-CM and the parent ADMSCs synergistically increased neovascularization of infarcted myocardium compared to saline control in a porcine model of MI (77).
Culture conditions of MSCs can greatly alter the content of MSC-CM (85), therefore optimization of culture conditions could generate a more effective therapeutic. Exposing BM-MSCs to hypoxic conditions has been shown to induce a >1.5 fold increase in an array of angiogenic/arteriogeneic cytokine genes; furthermore, administering the same MSC-CM to a murine model of hind limb ischemia enhanced collateral flow, improved limb function, reduced auto-amputation, and attenuated muscle atrophy when compared with control media (86). Forming spheroids of BM-MSCs (25k cells) using the hanging drop method has been shown to increase the production of anti-inflammatory agents TSG-6 and PGE2 compared to dissociated MSCs, and the administration of the resulting MSC-CM attenuated macrophage phenotype in vitro and significantly lowered inflammation in a mouse model of peritonitis (87, 88). Exposing MSC spheroids to hypoxic conditions and inflammatory stimuli further enhanced the secretion of PGE2 and VEGF (85) and encapsulating MSC spheroids in unmodified or RGD modified fibrin gels has also been shown to increase MSC secretion of VEGF and PGE2 (89–91). However, recent findings suggest that MSCs seeded onto macroporous scaffolds secrete significantly higher levels of pro-angiogenic factors compared to MSCs encapsulated in fibrin gels (92). Furthermore, culturing ADMSCs on electrospun fibers produces significantly higher levels of anti-inflammatory and pro-angiogenic cytokines compared to those cultured on plates (93). Combined, the preceding evidence suggests that culturing MSC spheroids in 3D hypoxic environments, and exposing cells to an inflammatory stimulus, enhances the anti-inflammatory and pro-angiogenic potential of the resulting MSC-CM.
Providing a therapeutic effect using MSC secreted factors or MSC-CM is limited by the difficulties in delivering these products to the intended cell type and also by their short residence time in vivo which necessitates high initial concentrations. These limitations can largely be overcome by employing only the extracellular vesicles (EVs) secreted by stem cells. EVs offer many benefits as a therapeutic base for functionalizing vascular scaffolds such as cell specific targeting via the presentation of surface/membrane proteins (94, 95), physiological delivery of cargo to target cells (96, 97), reduced immunogenicity and stability under physiological conditions including protection of cargo from enzymatic degradation (98) and bypass of compliment activation (95, 99, 100). The potential of employing EVs as a therapeutic base for functionalizing vascular scaffolds is explored in the following section.
MSC Derived Extracellular Vesicles
EVs are cell-derived phospholipid membrane based nano-particles that present with functional surface/membrane proteins and contain protein and RNA species that dynamically reflect the state of the parent cell and tissue (101). EVs are produced by most cells in the body (102, 103) and serve to transmit biological signals, transfer proteins/nucleic acids, and induce biological effects on target cells via surface receptor interactions, membrane fusion or endocytosis of the EVs by the target cell (96, 97). EVs can be categorized into three classes based on their cellular origins. Exosomes (30–200 nm) are released by cells when intracellular multi-vesicle bodies form via invagination of the cell membrane and are selectively loaded with endosomes containing protein, mRNA and miRNA. Fusion of the multi-vesicle body with the cell membrane releases these endosomes as exosomes (104). Micro-vesicles (200–1,000 nm) are released via direct outward budding of the cell membrane and contain protein, mRNA, and miRNA (Figure 3). The loading of microvesicle cargo is less selective than exosomes and membrane proteins are more reflective of the parent cell membrane due to direct budding (103). Apoptotic bodies (1,000–5,000 nm) are released by cells upon fragmentation of the plasma membrane during apoptosis (105). The term EV is used here to refer exclusively to exosomes and microvesicles as apoptotic bodies are distinct in activity and content (106).
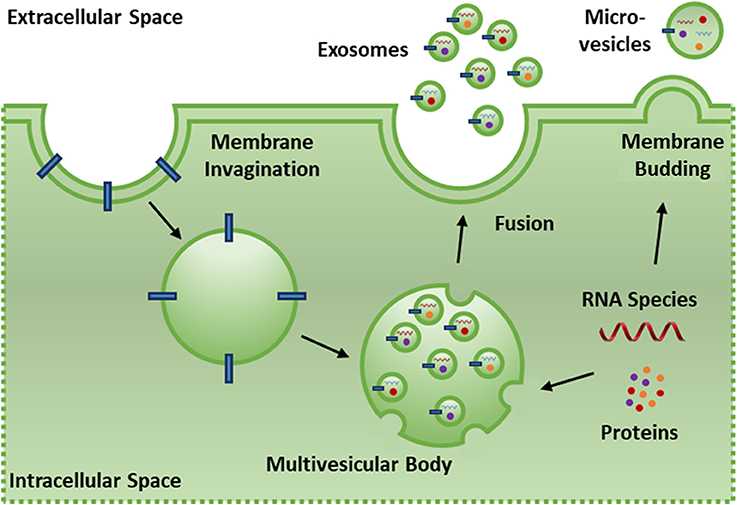
Figure 3. Exosomes (30–200 nm) are released by cells when intracellular multi-vesicle bodies form via invaginations of the cell membrane and are selectively loaded with endosomes containing protein, mRNA and miRNA. Fusion of the multi-vesicle body with the cell membrane releases these endosomes as exosomes. Micro-vesicles (200–1,000 nm) are released via direct outward budding of the cell membrane and contain protein, mRNA and miRNA. The loading of microvesicle cargo is less selective than exosomes and membrane proteins are more reflective of the parent cell membrane due to direct budding.
EVs can be isolated from cell culture supernatant via density-based, size-based, precipitation, immunoaffinity, and microfluidic based techniques [as reviewed in Li et al. (107)]. Although ultracentrifugation remains the gold standard, each technique has inherent advantages and limitations regarding process speed/cost and EV yield/functionality. Once isolated, guidelines have been published by the International Society for EVs regarding minimum standards for EV characterization. These guidelines require that EV size, concentration and morphology be determined in addition to screening for EV enriched markers and quantifying the co-precipitating protein levels to assess the purity of the EV isolate (108).
MSC-EVs have already shown regenerative potential and have also been credited with many of the therapeutic effects seen during the treatment of cells and tissues with MSC-CM (109, 110). Furthermore, MSCs are regarded as the optimal source for obtaining therapeutic EVs due to their immunomodulatory properties (111), their high expansion capacity/potential for immortalization (112) and the large numbers of EVs that they secrete relative to other cells (100). Although not yet employed in the field of vascular tissue engineering, other areas of tissue engineering have begun to utilize EVs such as bone regeneration (113), adipose tissue regeneration (114), and wound healing (115, 116). Furthermore, the functional relevance of EVs in regenerative medicine (such as promoting cell viability, angiogenesis, extracellular matrix interactions, and immunomodulation) has already been highlighted [as reviewed in De Jong et al. (117)].
Numerous studies have examined the effects of free EV injections in other areas of cardiovascular research such as vein grafting, angiogenesis and MI. Liu et al. demonstrated that the degree of intimal hyperplasia was significantly decreased following vein graft implantation in a murine model with multiple intraperitoneal injections of human ADMSC-EVs. Macrophage presence was also found to be reduced and significantly decreased expression levels of IL-6 and MCP-1 were found in ADMSC-EV treated mice compared to controls (118). MSC derived exosomes have been shown to induce angiogenesis in vitro through increased endothelial cell migration and tube formation (119, 120), and also in vivo through increased vessel formation in murine Matrigel plugs, corneal assays, and cerebral artery occlusion relative to controls (119, 121–124). Furthermore, administration of hESC derived exosomes (125), hESC-MSCs derived exosomes (109, 112, 126), BM-MSC derived EVs (127), and BM-MSC derived exosomes (128–130) have all been shown to significantly reduce infarct size in murine models of MI compared to controls (Figure 4). Interestingly, only intact and not lysed exosomes demonstrated a therapeutic effect, therefore suggesting that both exosome mediated delivery, in addition to exosome cargo, are required to successfully treat cardiovascular conditions (126).
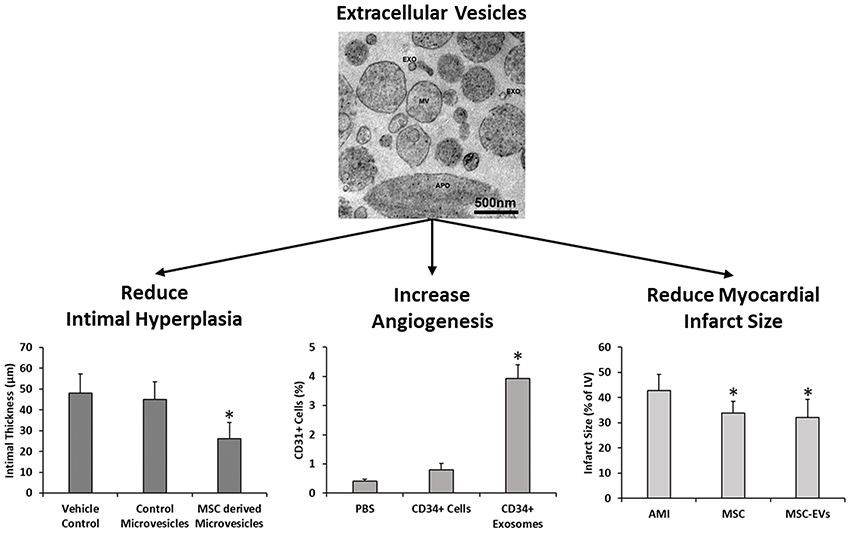
Figure 4. MSC derived extracellular vesicles have been previously shown to reduce intimal hyperplasia in murine models of vein grafting relative to vehicle and fibroblast microvesicle controls (118), increase angiogenesis in murine matriplug models relative to vehicle control and parent cells (119), and also to reduce infarct size in murine models of MI in a manner similar to the parent cells (124). EXO, exosomes; MV, microvesicles and APO, apoptotic bodies. Images and data adapted from Liu et al. (118), Sahoo et al. (119), Bian et al. (127), and Osteikoetxea et al. (131) with permissions. *denotes statistically significant differences at p < 0.05.
In an attempt to elucidate the therapeutic mechanism of MSC-EVs, extensive transcriptomic and proteomic characterization of ADMSC-EVs has been performed and the results compared to those obtained from the parent MSCs. It has been shown that ADMSC-EVs contain a similar yet distinct protein, miRNA and mRNA cargo compared to their parent cells. Specifically, ADMSC-EVs are enriched for the mi-RNAs miR-183, miR-378, miR-140, and miR-222; for 255 genes including TRPS1, ELK4, KLF7, and NRIP1; and for 277 proteins that play important biological roles including glycoproteins, extracellular matrix remodeling, blood coagulation, inflammatory response, TGF-B signaling pathway, and angiogenic proteins. The ADMSC-EV cargo is therefore enriched to support a range of functions important to vascular tissue engineering including extracellular matrix remodeling, angiogenesis, inflammation, blood coagulation, and apoptosis (132–134). Consequently, MSC-derived EVs are worthy of future research/therapeutic focus in this context.
Future of MSC-EVs in Vascular Tissue Engineering
The role of MSC-EVs in vascular tissue engineering is particularly exciting due to the need for a cell-free therapeutic base that can be incorporated into a scaffold and signal to cells in a paracrine manner to prevent acute thrombosis and encourage appropriate remodeling. However, a number of points must be addressed prior to the effective translation of TEVG technologies that incorporate MSC-EVs:
1. The optimal culture conditions for parent MSCs must be identified to ensure that the optimal yield of EVs is being obtained in a safe and repeatable manner. Although this has been studied extensively for MSC-CM (see section MSC Derived Extracellular Vesicles), the therapeutic effects of free factors and EVs in MSC-CM must be de-coupled and culture conditions optimized to specifically increase the therapeutic efficacy of isolated EVs.
2. A cheap, reliable and EV friendly method of isolating MSC-EVs must be identified and implemented to ensure that the optimal yield of EVs is being obtained in a safe and repeatable manner. Ultimately, a preferred method of isolating intact EVs must be identified and scaled so that EV based TEVGs can be developed into a clinically viable therapy.
3. The optimal method of delivering and retaining MSC-EVs into a tissue engineered vascular scaffold must be identified to ensure that MSC-EVs are present in sufficient numbers and remain intact. Encouraging research has demonstrated that directly incorporating EVs into a decalcified bone matrix scaffold is possible and elicits an equivalent neo-vessel formation response compared to incorporating MSCs alone following subcutaneous murine implants (113). Furthermore, it has been shown that cardiosphere derived EVs remain stable at −80°C for up to 90 days and that both in vitro and in vivo bioactivity is preserved following lyophilisation (135). This suggests that EVs can be directly incorporated into many forms of scaffold production and therefore exhibit potential as a therapeutic source in off-the-shelf vascular graft applications.
4. The in vivo remodeling potential of MSC-EV seeded vascular scaffolds must be assessed using established small and large animal preclinical models to determine if they elicit an appropriate TEVG host remodeling response.
Summary
One approach to vascular tissue engineering is to implant biodegradable tubular scaffolds, seeded with autologous stem cells that trigger the development of functional native-like vascular replacements. However, stem cells harvested from elderly or diabetic patients have diminished regenerative potential in vascular tissue engineering. The inherent limitations of an autologous stem cell approach can be addressed using allogeneic MSCs. However, potential safety, regulatory, and practicality issues of cellular incorporation suggest that a cell-free approach may be more prudent. MSC-EVs present as one such cell-free approach and offer many benefits as a therapeutic base for functionalizing vascular scaffolds such as cell specific targeting, physiological delivery of cargo to target cells, reduced immunogenicity, and stability under physiological conditions. Despite promising findings of EV therapy in the broader field of cardiovascular research, further work is required to explore the full potential of this promising therapeutic in vascular tissue engineering.
Author Contributions
EC: concept generation, literature review, manuscript writing, critical review of manuscript; JW, FO and DV: concept generation, manuscript writing, critical review of manuscript.
Funding
This work was funded by the European Union's Horizon 2020 research and innovation program under the Marie Sklodowska-Curie grant agreement No 708867 awarded to EC, the Competitive Medical Research Fund, University of Pittsburgh awarded to JW and the National Institute of Health under grant agreement No R01HL130077 awarded to DV.
Conflict of Interest Statement
The authors declare that the research was conducted in the absence of any commercial or financial relationships that could be construed as a potential conflict of interest.
References
1. Kaushal S, Amiel GE, Guleserian KJ, Shapira OM, Perry T, Sutherland FW, et al. Functional small-diameter neovessels created using endothelial progenitor cells expanded ex vivo. Nat Med. (2001) 7:1035–40. doi: 10.1038/nm0901-1035
2. He H, Shirota T, Yasui H, Matsuda T. Canine endothelial progenitor cell-lined hybrid vascular graft with nonthrombogenic potential. J Thorac Cardiovasc Surg. (2003) 126:455–64. doi: 10.1016/S0022-5223(02)73264-9
3. Zhu C, Ying D, Mi J, Li L, Zeng W, Hou C, et al. Development of anti-atherosclerotic tissue-engineered blood vessel by A20-regulated endothelial progenitor cells seeding decellularized vascular matrix. Biomaterials (2008) 29:2628–36. doi: 10.1016/j.biomaterials.2008.03.005
4. Neff LP, Tillman BW, Yazdani SK, MacHingal MA, Yoo JJ, Soker S, et al. Vascular smooth muscle enhances functionality of tissue-engineered blood vessels in vivo. J Vasc Surg. (2011) 53:426–34. doi: 10.1016/j.jvs.2010.07.054
5. Quint C, Kondo Y, Manson RJ, Lawson JH, Dardik A, Niklason LE. Decellularized tissue-engineered blood vessel as an arterial conduit. Proc Natl Acad Sci USA. (2011) 108:9214–9. doi: 10.1073/pnas.1019506108
6. L'Heureux N, Pâquet S, Labbé R, Germain L, Auger FA. A completely biological tissue-engineered human blood vessel. FASEB J. (1998) 12:47–56. doi: 10.892-6638/97/0012-0047
7. L'Heureux N, Dusserre N, Konig G, Victor B, Keire P, Wight TN, et al. Human tissue-engineered blood vessels for adult arterial revascularization. Nat Med. (2006) 12:361–5. doi: 10.1038/nm1364
8. L'Heureux N, McAllister TN, de la Fuente LM. Tissue-engineered blood vessel for adult arterial revascularization. N Engl J Med. (2007) 357:1451–3. doi: 10.1056/NEJMc071536
9. McAllister TN, Maruszewski M, Garrido SA, Wystrychowski W, Dusserre N, Marini A, et al. Effectiveness of haemodialysis access with an autologous tissue-engineered vascular graft: a multicentre cohort study. Lancet (2009) 373:1440–6. doi: 10.1016/S0140-6736(09)60248-8
10. Dahl SLM, Kypson AP, Lawson JH, Blum JL, Strader JT, Li Y, et al. Readily available tissue-engineered vascular grafts. Sci Trans Med. (2011) 3:68ra9. doi: 10.1126/scitranslmed.3001426
11. Wystrychowski W, Cierpka L, Zagalski K, Garrido S, Dusserre N, Radochonski S, et al. Case study: first implantation of a frozen, devitalized tissueengineered vascular graft for urgent hemodialysis access. J Vasc Access (2011) 12:67–70. doi: 10.5301/JVA.2011.6360
12. Wystrychowski W, McAllister TN, Zagalski K, Dusserre N, Cierpka L, L'Heureux N. First human use of an allogeneic tissue-engineered vascular graft for hemodialysis access. J Vasc Surg. (2014) 60:1353–7. doi: 10.1016/j.jvs.2013.08.018
13. Shin'oka T, Shum-Tim D, Ma PX, Tanel RE, Isogai N, Langer R, et al. Creation of viable pulmonary artery autografts through tissue engineering. J Thorac Cardiovasc Surg. (1998) 115:536–46. doi: 10.1016/S0022-5223(98)70315-0
14. Niklason LE, Gao J, Abbott WM, Hirschi KK, Houser S, Marini R, et al. Functional arteries grown in vitro. Science (1999) 284:489–93. doi: 10.1126/science.284.5413.489
15. Shin'oka T, Imai Y, Ikada Y. Transplantation of a tissue-engineered pulmonary artery. N Engl J Med. (2001) 344:532–3. doi: 10.1056/NEJM200102153440717
16. Naito Y, Imai Y, Shin'oka T, Kashiwagi J, Aoki M, Watanabe M, et al. Successful clinical application of tissue-engineered graft for extracardiac Fontan operation. J Thorac Cardiovasc Surg. (2003) 125:419–420. doi: 10.1067/mtc.2003.134
17. Cho SW, Lim SH, Kim IK, Hong YS, Kim SS, Yoo KJ, et al. Small-diameter blood vessels engineered with bone marrow-derived cells. Ann Surg. (2005) 241:506–15. doi: 10.1097/01.sla.0000154268.12239.ed
18. Cho SW, Lim JE, Chu HS, Hyun HJ, Choi CY, Hwang KC, et al. Enhancement of in vivo endothelialization of tissue-engineered vascular grafts by granulocyte colony-stimulating factor. J Biomed Mater Res. (2006) 76:252–263. doi: 10.1002/jbm.a.30535
19. Cho SW, Kim IK, Kang JM, Song KW, Kim HS, Park CH, et al. Evidence for in vivo growth potential and vascular remodeling of tissue-engineered artery. Tissue Eng A. (2009) 15:901–912. doi: 10.1089/ten.tea.2008.0172
20. Roh JD, Brennan MP, Lopez-Soler RI, Fong PM, Goyal A, Dardik A, et al. Construction of an autologous tissue-engineered venous conduit from bone marrow-derived vascular cells: optimization of cell harvest and seeding techniques. J Pediatr Surg. (2007) 42:198–202. doi: 10.1016/j.jpedsurg.2006.09.054
21. Lim SH, Cho SW, Park JC, Jeon O, Lim JM, Kim SS, et al. Tissue-engineered blood vessels with endothelial nitric oxide synthase activity. J Biomed Mater Res. (2008) 85B:537–46. doi: 10.1002/jbm.b.309770
22. Zhao Y, Zhang S, Zhou J, Wang J, Zhen M, Liu Y, et al. The development of a tissue-engineered artery using decellularized scaffold and autologous ovine mesenchymal stem cells. Biomaterials (2010) 31:296–307. doi: 10.1016/j.biomaterials.2009.09.049
23. Maul TM, Nieponice A, Vorp DA. Chapter 9: Vascular differentiation of stem cells by mechanical forces. Hemodynamics Mechanobiol Endothelium. (2010) 247–9. doi: 10.1142/9789814280426_0009
24. Gui L, Dash BC, Luo J, Qin L, Zhao L, Yamamoto K, et al. Implantable tissue-engineered blood vessels from human induced pluripotent stem cells. Biomaterials (2016) 102:120–9. doi: 10.1016/j.biomaterials.2016.06.010
25. Luo J, Qin L, Kural MH, Schwan J, Li X, Bartulos O, et al. Vascular smooth muscle cells derived from inbred swine induced pluripotent stem cells for vascular tissue engineering. Biomaterials (2017) 147:116–32. doi: 10.1016/j.biomaterials.2017.09.019
26. Roh JD, Sawh-Martinez R, Brennan MP, Jay SM, Devine L, Rao DA, et al. Tissue-engineered vascular grafts transform into mature blood vessels via an inflammation-mediated process of vascular remodeling. Proc Natl Acad Sci USA. (2010) 107:4669–74. doi: 10.1073/pnas.0911465107
27. Hibino N, Yi T, Duncan DR, Rathore A, Dean E, Naito Y, et al. A critical role for macrophages in neovessel formation and the development of stenosis in tissue-engineered vascular grafts. Faseb J. (2011) 25:4253–63. doi: 10.1096/fj.11-186585
28. Matsumura G, Miyagawa-Tomita S, Shin'Oka T, Ikada Y, Kurosawa H. First evidence that bone marrow cells contribute to the construction of tissue-engineered vascular autografts in vivo. Circulation (2003) 108:1729–34. doi: 10.1161/01.CIR.0000092165.32213.61
29. Hibino N, Shin'oka T, Matsumura G, Ikada Y, Kurosawa H. The tissue-engineered vascular graft using bone marrow without culture. J Thorac Cardiovasc Surg. (2005) 129:1064–70. doi: 10.1016/j.jtcvs.2004.10.030
30. Matsumura G, Ishihara Y, Miyagawa-Tomita S, Ikada Y, Matsuda S, Kurosawa H, et al. Evaluation of tissue-engineered vascular autografts. Tissue Eng. (2006) 12:3075–83. doi: 10.1089/ten.2006.12.3075
31. Brennan MP, Dardik A, Hibino N, Roh JD, Nelson GN, Papademitris X, et al. Tissue-engineered vascular grafts demonstrate evidence of growth and development when implanted in a Juvenile animal model. Trans Meet Am Surg Assoc. (2008) 126:20–7. doi: 10.1097/SLA.0b013e318184dcbd
32. Hibino N, McGillicuddy E, Matsumura G, Ichihara Y, Naito Y, Breuer C, et al. Late-term results of tissue-engineered vascular grafts in humans. J Thorac Cardiovasc Surg. (2010) 139:431–6, 436.e1–2. doi: 10.1016/j.jtcvs.2009.09.057
33. Mirensky TL, Hibino N, Sawh-Martinez RF, Yi T, Villalona G, Shinoka T, et al. Tissue-engineered vascular grafts: does cell seeding matter? J Pediatric Surg. (2010) 45:1299–305. doi: 10.1016/j.jpedsurg.2010.02.102
34. Hibino N, Nalbandian A, Devine L, Martinez RS, McGillicuddy E, Yi T, et al. Comparison of human bone marrow mononuclear cell isolation methods for creating tissue-engineered vascular grafts: novel filter system versus traditional density centrifugation method. Tissue Eng. Part C Methods (2011) 17:933–8. doi: 10.1089/ten.tec.2011.0110
35. Hibino N, Villalona G, Pietris N, Duncan DR, Schoffner A, Roh JD, et al. Tissue-engineered vascular grafts form neovessels that arise from regeneration of the adjacent blood vessel. FASEB J. (2011) 25:2731–9. doi: 10.1096/fj.11-182246
36. Naito Y, Williams-Fritze M, Duncan DR, Church SN, Hibino N, Madri JA, et al. Characterization of the natural history of extracellular matrix production in tissue-engineered vascular grafts during neovessel formation. Cells Tissues Organs (2011) 195:60–72. doi: 10.1159/000331405
37. Udelsman BV, Khosravi R, Miller KS, Dean EW, Bersi MR, Rocco K, et al. Characterization of evolving biomechanical properties of tissue engineered vascular grafts in the arterial circulation. J Biomech. (2014) 47:2070–9. doi: 10.1016/j.jbiomech.2014.03.011
38. Stacy MR, Naito Y, Maxfield MW, Kurobe H, Tara S, Chan C, et al. Targeted imaging of matrix metalloproteinase activity in the evaluation of remodeling tissue-engineered vascular grafts implanted in a growing lamb model. J Thorac Cardiovasc Surg. (2014) 148:2227–33. doi: 10.1016/j.jtcvs.2014.05.037
39. Kurobe H, Maxfield MW, Naito Y, Cleary M, Stacy MR, Solomon D, et al. Comparison of a closed system to a standard open technique for preparing tissue-engineered vascular grafts. Tissue Eng. Part C Methods (2015) 21:88–93. doi: 10.1089/ten.tec.2014.0160
40. Kurobe H, Tara S, Maxfield MW, Rocco KA, Bagi PS, Yi T, et al. Comparison of the biological equivalence of two methods for isolating bone marrow mononuclear cells for fabricating tissue-engineered vascular grafts. Tissue Eng. Part C Methods (2015) 21:597–604. doi: 10.1089/ten.tec.2014.0442
41. Duncan DR, Chen PY, Patterson JT, Lee YU, Hibino N, Cleary M, et al. TGFβR1 inhibition blocks the formation of stenosis in tissue-engineered vascular grafts. J Am College Cardiol. (2015) 512–4. doi: 10.1016/j.jacc.2014.08.057
42. Lee YU, Mahler N, Best CA, Tara S, Sugiura T, Lee AY, et al. Rational design of an improved tissue-engineered vascular graft: determining the optimal cell dose and incubation time. Regener Med. (2016) 11:159–67. doi: 10.2217/rme.15.85
43. Best C, Tara S, Wiet M, Reinhardt J, Pepper V, Ball M, et al. Deconstructing the Tissue engineered vascular graft: evaluating scaffold pre-wetting, conditioned media incubation, and determining the optimal mononuclear cell source. ACS Biomater Sci Eng. (2017) 3:1972–9. doi: 10.1021/acsbiomaterials.6b00123
44. Pepper VK, Clark ES, Best CA, Onwuka EA, Sugiura T, Heuer ED, et al. Intravascular Ultrasound characterization of a tissue-engineered vascular graft in an ovine model. J Cardiovasc Trans Res. (2017) 10:128–38. doi: 10.1007/s12265-016-9725-x
45. Zhang L, Zhou J, Lu Q, Wei Y, Hu S. A novel small-diameter vascular graft: in vivo behavior of biodegradable three-layered tubular scaffolds. Biotechnol Bioeng. (2008) 99:1007–15. doi: 10.1002/bit.21629
46. Hjortnaes J, Gottlieb D, Figueiredo JL, Melero-Martin J, Kohler RH, Bischoff J, et al. Intravital molecular imaging of small-diameter tissue-engineered vascular grafts in mice: a feasibility study. Tissue Eng. (2010) 16:597–607. doi: 10.1089/ten.tec.2009.0466
47. Hashi CK, Zhu Y, Yang GY, Young WL, Hsiao BS, Wang K, et al. Antithrombogenic property of bone marrow mesenchymal stem cells in nanofibrous vascular grafts. Proc Natl Acad Sci USA. (2007) 104:11915–20. doi: 10.1073/pnas.0704581104
48. Krawiec JT, Weinbaum JS, Liao HT, Ramaswamy AK, Pezzone DJ, Josowitz AD, et al. In vivo functional evaluation of tissue-engineered vascular grafts fabricated using human adipose-derived stem cells from high cardiovascular risk populations. Tissue Eng. (2016) 22:765–75. doi: 10.1089/ten.tea.2015.0379
49. Nieponice A, Soletti L, Guan J, Deasy BM, Huard J, Wagner WR, et al. Development of a tissue-engineered vascular graft combining a biodegradable scaffold, muscle-derived stem cells and a rotational vacuum seeding technique. Biomaterials (2008) 29:825–33. doi: 10.1016/j.biomaterials.2007.10.044
50. He W, Nieponice A, Soletti L, Hong Y, Gharaibeh B, Crisan M, et al. Pericyte-based human tissue engineered vascular grafts. Biomaterials (2010) 31:8235–44. doi: 10.1016/j.biomaterials.2010.07.034
51. Shin'oka T, Matsumura G, Hibino N, Naito Y, Watanabe M, Konuma T, et al. Midterm clinical result of tissue-engineered vascular autografts seeded with autologous bone marrow cells. J Thorac Cardiovasc Surg. (2005) 129:1330–8. doi: 10.1016/j.jtcvs.2004.12.047
52. Shin'oka T, Breuer C. Tissue-engineered blood vessels in pediatric cardiac surgery. Yale J Biol Med. (2008) 81:161–6.
53. Huang NF, Li S. Mesenchymal stem cells for vascular regeneration. Regener Med. (2008) 3:877–92. doi: 10.2217/17460751.3.6.877
54. Caplan AI. Mesenchymal stem cells: time to change the name!. Stem Cells Trans Med. (2017) 6:1445–51. doi: 10.1002/sctm.17-0051
55. Krawiec JT, Liao HT, Kwan LY, D'Amore A, Weinbaum JS, Rubin JP, et al. Evaluation of the stromal vascular fraction of adipose tissue as the basis for a stem cell-based tissue-engineered vascular graft. J Vasc Surg. (2017) 66.883e1–90.e1. doi: 10.1016/j.jvs.2016.09.034
56. Nieponice A, Soletti L, Guan J, Hong Y, Gharaibeh B, Maul TM, et al. In vivo assessment of a tissue-engineered vascular graft combining a biodegradable elastomeric scaffold and muscle-derived stem cells in a rat model. Tissue Eng. (2010) 16:1215–23. doi: 10.1089/ten.TEA.2009.0427
57. Crisan M, Corselli M, Chen WCW, Péault B. Perivascular cells for regenerative medicine. J Cell Mol Med. (2012) 16:2851–60. doi: 10.1111/j.1582-4934.2012.01617.x
58. Matsumura G, Hibino N, Ikada Y, Kurosawa H, Shin'oka T. Successful application of tissue engineered vascular autografts: clinical experience. Biomaterials (2003) 24:2303–8. doi: 10.1016/S0142-9612(03)00043-7
59. Krawiec JT, Weinbaum JS, St Croix CM, Phillippi JA, Watkins SC, Peter Rubin J, et al. A cautionary tale for autologous vascular tissue engineering: impact of human demographics on the ability of adipose-derived mesenchymal stem cells to recruit and differentiate into smooth muscle cells. Tissue Eng. (2015) 21:426–37. doi: 10.1089/ten.tea.2014.0208
60. Soletti L, Nieponice A, Guan J, Stankus JJ, Wagner WR, Vorp DA. A seeding device for tissue engineered tubular structures. Biomaterials (2006) 27:4863–70. doi: 10.1016/j.biomaterials.2006.04.042
61. Soletti L, Hong Y, Guan J, Stankus JJ, El-Kurdi MS, Wagner WR, et al. A bilayered elastomeric scaffold for tissue engineering of small diameter vascular grafts. Acta Biomater. (2010) 6:110–22. doi: 10.1016/j.actbio.2009.06.026
62. Le Blanc K, Tammik C, Rosendahl K, Zetterberg E, Ringdén O. HLA expression and immunologic properties of differentiated and undifferentiated mesenchymal stem cells. Exp Hematol. (2003) 31:890–6. doi: 10.1016/S0301-472X(03)00110-3
63. Le Blanc K, Tammik L, Sundberg B, Haynesworth SE, Ringdén O. Mesenchymal stem cells inhibit and stimulate mixed lymphocyte cultures and mitogenic responses independently of the major histocompatibility complex. Scand. J Immunol. (2003) 57:11–20. doi: 10.1046/j.1365-3083.2003.01176.x
64. François M, Romieu-Mourez R, Li M, Galipeau J. Human MSC suppression correlates with cytokine induction of indoleamine 2,3-dioxygenase and bystander m2 macrophage differentiation. Mol Ther. (2012) 20:187–95. doi: 10.1038/mt.2011.189
65. Tsuchida H, Hashimoto J, Crawford E, Manske P, Lou J, Louis S, et al. Engineered allogeneic mesenchymal stem cells repair femoral segmental defect in rats.pdf. J Orthopaedic Res. (2003) 21:44–53. doi: 10.1016/S0736-0266(02)00108-0
66. Liu G, Zhang Y, Liu B, Sun J, Li W, Cui L. Bone regeneration in a canine cranial model using allogeneic adipose derived stem cells and coral scaffold. Biomaterials (2013) 34:2655–64. doi: 10.1016/j.biomaterials.2013.01.004
67. Wu J, Wang Q, Fu X, Wu X, Gu C, Bi J, et al. Influence of immunogenicity of allogeneic bone marrow mesenchymal stem cells on bone tissue engineering. Cell Trans. (2016) 25:229–42. doi: 10.3727/096368915X687967
68. Vega A, Martín-Ferrero MA, Del Canto F, Alberca M, García V, Munar A, et al. Treatment of knee osteoarthritis with allogeneic bone marrow mesenchymal stem cells. Transplantation (2015) 99:1681–90. doi: 10.1097/TP.0000000000000678
69. Harman R, Carlson K, Gaynor J, Gustafson S, Dhupa S, Clement K, et al. A prospective, randomized, masked, and placebo-controlled efficacy study of intraarticular allogeneic adipose stem cells for the treatment of osteoarthritis in dogs. Front Vet Sci. (2016) 3:81. doi: 10.3389/fvets.2016.00081
70. Feng C, Luo X, He N, Xia H, Lv X, Zhang X, et al. Efficacy and persistence of allogeneic adipose-derived mesenchymal stem cells combined with hyaluronic acid in osteoarthritis after intra-articular injection in a sheep model. Tissue Eng. (2017) 24:219–33. doi: 10.1089/ten.tea.2017.0039
71. Chen L, Tredget EE, Liu C, Wu Y. Analysis of allogenicity of mesenchymal stem cells in engraftment and wound healing in mice. PLoS ONE (2009) 4: e7119. doi: 10.1371/journal.pone.0007119
72. Hanson SE, Kleinbeck KR, Cantu D, Kim J, Bentz ML, Faucher LD, et al. Local delivery of allogeneic bone marrow and adipose tissue-derived mesenchymal stromal cells for cutaneous wound healing in a porcine model. J Tissue Eng Regen Med. (2016) 10:E90–E100. doi: 10.1002/term.1700
73. Ryu HH, Lim JH, Byeon YE, Park JR, Seo MS, Lee YW, et al. Functional recovery and neural differentiation after transplantation of allogenic adipose-derived stem cells in a canine model of acute spinal cord injury. J Vet Sci. (2009) 10:273–84. doi: 10.4142/jvs.2009.10.4.273
74. Hare JM, Fishman JE, Gerstenblith G, DiFede Velazquez DL, Zambrano JP, Suncion VY, et al. Comparison of allogeneic vs autologous bone marrow–derived mesenchymal stem cells delivered by transendocardial injection in patients with ischemic cardiomyopathy. JAMA (2012) 308:2369. doi: 10.1001/jama.2012.25321
75. Perin EC, Borow KM, Silva GV, DeMaria AN, Marroquin OC, Huang PP, et al. A Phase II dose-escalation study of allogeneic mesenchymal precursor cells in patients with ischemic or nonischemic heart failure. Circ Res. (2015) 117:576–84. doi: 10.1161/CIRCRESAHA.115.306332
76. Penn MS, Ellis S, Gandhi S, Greenbaum A, Hodes Z, Mendelsohn FO, et al. Adventitial delivery of an allogeneic bone marrow-derived adherent stem cell in acute myocardial infarction: phase i clinical study. Circ Res. (2012) 110:304–11. doi: 10.1161/CIRCRESAHA.111.253427
77. Vilahur G, Oñate B, Cubedo J, Béjar MT, Arderiu G, Peña E, et al. Allogenic adipose-derived stem cell therapy overcomes ischemia-induced microvessel rarefaction in the myocardium: systems biology study. Stem Cell Res Ther. (2017) 8:52. doi: 10.1186/s13287-017-0509-2
78. Huang XP, Sun Z, Miyagi Y, Kinkaid HM, Zhang L, Weisel RD, et al. Differentiation of allogeneic mesenchymal stem cells induces immunogenicity and limits their long-term benefits for myocardial repair. Circulation (2010) 122:2419–29. doi: 10.1161/CIRCULATIONAHA.110.955971
79. Lee YU, De Dios Ruiz-Rosado J, Mahler N, Best CA, Tara S, Yi T, et al. TGF-β receptor 1 inhibition prevents stenosis of tissue-engineered vascular grafts by reducing host mononuclear phagocyte activation. FASEB J. (2016) 30:2627–36. doi: 10.1096/fj.201500179R
80. Phinney DG, Pittenger MF. Concise review: MSC-derived exosomes for cell-free therapy. Stem Cells (2017) 35:851–8. doi: 10.1002/stem.2575
81. Caplan AI, Dennis JE. Mesenchymal stem cells as trophic mediators. J Cell Biochem. (2006) 98:1076–84. doi: 10.1002/jcb.20886
82. Gnecchi M, He H, Liang OD, Melo LG, Morello F, Mu H, et al. Paracrine action accounts for marked protection of ischemic heart by Akt-modified mesenchymal stem cells. Nat Med. (2005) 11:367–8. doi: 10.1038/nm0405-367
83. Timmers L, Lim SK, Arslan F, Armstrong JS, Hoefer IE, Doevendans PA, et al. Reduction of myocardial infarct size by human mesenchymal stem cell conditioned medium. Stem Cell Res. (2008) 1:129–37. doi: 10.1016/j.scr.2008.02.002
84. Timmers L, Lim SK, Hoefer IE, Arslan F, Lai RC, van Oorschot AAM, et al. Human mesenchymal stem cell-conditioned medium improves cardiac function following myocardial infarction. Stem Cell Res. (2011) 6:206–14. doi: 10.1016/j.scr.2011.01.001
85. Murphy KC, Whitehead J, Falahee PC, Zhou D, Simon SI, Leach JK. Multifactorial experimental design to optimize the anti-inflammatory and proangiogenic potential of mesenchymal stem cell spheroids. Stem Cells (2017) 35:1493–504. doi: 10.1002/stem.2606
86. Kinnaird T, Stabile E, Burnett MS, Lee CW, Barr S, Fuchs S, et al. Marrow-derived stromal cells express genes encoding a broad spectrum of arteriogenic cytokines and promote in vitro and in vivo arteriogenesis through paracrine mechanisms. Circ Res. (2004) 94:678–85. doi: 10.1161/01.RES.0000118601.37875.AC
87. Bartosh TJ, Ylöstalo JH, Mohammadipoor A, Bazhanov N, Coble K, Claypool K, et al. Aggregation of human mesenchymal stromal cells (MSCs) into 3D spheroids enhances their antiin fl ammatory properties. Proc Natl Acad Sci USA. (2010) 107:13724–9. doi: 10.1073/pnas.1008117107
88. Ylöstalo JH, Bartosh TJ, Coble K, Prockop DJ. Human mesenchymal stem/stromal cells cultured as spheroids are self-activated to produce prostaglandin E2 that directs stimulated macrophages into an anti-inflammatory phenotype. Stem Cells (2012) 30:2283–96. doi: 10.1002/stem.1191
89. Murphy KC, Fang SY, Leach JK. Human mesenchymal stem cell spheroids in fibrin hydrogels exhibit improved cell survival and potential for bone healing. Cell Tissue Res. (2014) 357:91–9. doi: 10.1007/s00441-014-1830-z
90. Ho SS, Murphy KC, Binder BYK, Vissers CB, Leach JK. Increased survival and function of mesenchymal stem cell spheroids entrapped in instructive alginate hydrogels. Stem Cells Trans Med. (2016) 5:773–81. doi: 10.5966/sctm.2015-0211
91. Murphy KC, Whitehead J, Zhou D, Ho SS, Leach JK. Engineering fibrin hydrogels to promote the wound healing potential of mesenchymal stem cell spheroids. Acta Biomater. (2017) 64:176–186. doi: 10.1016/j.actbio.2017.10.007
92. Qazi TH, Mooney DJ, Duda GN, Geissler S. Biomaterials that promote cell-cell interactions enhance the paracrine function of MSCs. Biomaterials (2017) 140:103–14. doi: 10.1016/j.biomaterials.2017.06.019
93. Su N, Gao PL, Wang K, Wang JY, Zhong Y, Luo Y. Fibrous scaffolds potentiate the paracrine function of mesenchymal stem cells: a new dimension in cell-material interaction. Biomaterials (2017) 141:74–85. doi: 10.1016/j.biomaterials.2017.06.028
94. Mittelbrunn M, Gutiérrez-Vázquez C, Villarroya-Beltri C, González S, Sánchez-Cabo F, González MÁ, et al. Unidirectional transfer of microRNA-loaded exosomes from T cells to antigen-presenting cells. Nat Commun. (2011) 2:282. doi: 10.1038/ncomms1285
95. Kooijmans SAA, Vader P, van Dommelen SM, van Solinge WW, Schiffelers RM. Exosome mimetics: a novel class of drug delivery systems. Int J Nanomed. (2012) 7:1525–41. doi: 10.2147/IJN.S29661
96. Théry C, Zitvogel L, Amigorena S. Exosomes: composition, biogenesis and function. Nat Rev Immunol. (2002) 2:569–79. doi: 10.1038/nri855
97. Valadi H, Ekström K, Bossios A, Sjöstrand M, Lee JJ, Lötvall JO. Exosome-mediated transfer of mRNAs and microRNAs is a novel mechanism of genetic exchange between cells. Nat Cell Biol. (2007) 9:654–9. doi: 10.1038/ncb1596
98. Biancone L, Bruno S, Deregibus MC, Tetta C, Camussi G. Therapeutic potential of mesenchymal stem cell-derived microvesicles. Nephrol Dialysis Trans. (2012) 27:3037–42. doi: 10.1093/ndt/gfs168
99. Clayton A, Harris CL, Court J, Mason MD, Morgan BP. Antigen-presenting cell exosomes are protected from complement-mediated lysis by expression of CD55 and CD59. Eur J Immunol. (2003) 33:522–31. doi: 10.1002/immu.200310028
100. Yeo RWY, Lai RC, Zhang B, Tan SS, Yin Y, Teh BJ, et al. Mesenchymal stem cell: An efficient mass producer of exosomes for drug delivery. Adv Drug Deliv Rev. (2013) 65:336–341. doi: 10.1016/j.addr.2012.07.001
101. Van Dommelen SM, Vader P, Lakhal S, Kooijmans SAA, Van Solinge WW, Wood MJA, et al. Microvesicles and exosomes: opportunities for cell-derived membrane vesicles in drug delivery. J Control Release (2012) 161:635–44. doi: 10.1016/j.jconrel.2011.11.021
102. Théry C, Ostrowski M, Segura E. Membrane vesicles as conveyors of immune responses. Nat Reviews Immunol. (2009) 9:581–93. doi: 10.1038/nri2567
103. El Andaloussi S, Mäger I, Breakefield XO, Wood MJA. Extracellular vesicles: biology and emerging therapeutic opportunities. Nat Rev Drug Discov. (2013) 12:347–57. doi: 10.1038/nrd3978
104. Mathivanan S, Ji H, Simpson RJ. Exosomes: extracellular organelles important in intercellular communication. J Proteom. (2010) 73:1907–20. doi: 10.1016/j.jprot.2010.06.006
105. Akers JC, Gonda D, Kim R, Carter BS, Chen CC. Biogenesis of extracellular vesicles (EV): exosomes, microvesicles, retrovirus-like vesicles, and apoptotic bodies. J Neuro-oncol. (2013) 113:1–11. doi: 10.1007/s11060-013-1084-8
106. Bruno S, Porta S, Bussolati B. Extracellular vesicles in renal tissue damage and regeneration. Eur J Pharmacol. (2016) 790:83–91. doi: 10.1016/j.ejphar.2016.06.058
107. Li P, Kaslan M, Lee SH, Yao J, Gao Z. Progress in exosome isolation techniques. Theranostics (2017) 7:789–804. doi: 10.7150/thno.18133
108. Lotvall J, Hill AF, Hochberg F, Buzas EI, Vizio D, Di G, Thery C, et al. Minimal experimental requirements for definition of extracellular vesicles and their functions: a position statement from the International Society for extracellular vesicles. J Extracell Vesicles (2014) 3:26913. doi: 10.3402/jev.v3.26913
109. Lai RC, Arslan F, Lee MM, Sze NSK, Choo A, Chen TS, et al. Exosome secreted by MSC reduces myocardial ischemia/reperfusion injury. Stem Cell Res. (2010) 4:214–22. doi: 10.1016/j.scr.2009.12.003
110. Wang K, Jiang Z, Webster K, Chen J, Hu H, Zhou Y, et al. Enhanced cardioprotection by human endometrium mesenchymal stem cells driven by exosomal MicroRNA-21. Stem Cells Trans Med. (2016) 6:209–22. doi: 10.5966/sctm.2015-0023
111. Puissant B, Barreau C, Bourin P, Clavel C, Corre J, Bousquet C, et al. Immunomodulatory effect of human adipose tissue-derived adult stem cells: comparison with bone marrow mesenchymal stem cells. Br J Haematol. (2005) 129:118–29. doi: 10.1111/j.1365-2141.2005.05409.x
112. Chen T, Arslan F, Yin Y, Tan S, Lai R, Choo A, et al. Enabling a robust scalable manufacturing process for therapeutic exosomes through oncogenic immortalization of human ESC-derived MSCs. J Trans Med. (2011) 9:47. doi: 10.1186/1479-5876-9-47
113. Xie H, Wang Z, Zhang L, Lei Q, Zhao A, Wang H, et al. Extracellular vesicle-functionalized decalcified bone matrix scaffolds with enhanced pro-angiogenic and pro-bone regeneration activities. Sci Rep. (2017) 7:1–13. doi: 10.1038/srep45622
114. Dai M, Yu M, Zhang Y, Tian W. Exosome-like vesicles derived from adipose tissue provide biochemical cues for adipose tissue regeneration. Tissue Eng. (2017) 23: 1221–30. doi: 10.1089/ten.tea.2017.0045
115. Hu L, Wang J, Zhou X, Xiong Z, Zhao J, Yu R, et al. Exosomes derived from human adipose mensenchymal stem cells accelerates cutaneous wound healing via optimizing the characteristics of fibroblasts. Sci Rep. (2016) 6:32993. doi: 10.1038/srep32993
116. Wang L, Hu L, Zhou X, Xiong Z, Zhang C, Shehada HMA, et al. Exosomes secreted by human adipose mesenchymal stem cells promote scarless cutaneous repair by regulating extracellular matrix remodelling. Sci Rep. (2017) 7:13321. doi: 10.1038/s41598-017-12919-x
117. De Jong OG, Van Balkom BWM, Schiffelers RM, Bouten CVC, Verhaar MC. Extracellular vesicles: potential roles in regenerative medicine. Front Immunol. (2014) 5:608. doi: 10.3389/fimmu.2014.00608
118. Liu R, Shen H, Ma J, Sun L, Wei M. Extracellular vesicles derived from adipose mesenchymal stem cells regulate the phenotype of smooth muscle cells to limit intimal hyperplasia. Cardiovasc Drugs Ther. (2016) 30:111–8. doi: 10.1007/s10557-015-6630-5
119. Sahoo S, Klychko E, Thorne T, Misener S, Schultz KM, Millay M, et al. Exosomes from human CD34+ stem cells mediate their proangiogenic paracrine activity. Circ Res. (2011) 109:724–28. doi: 10.1161/CIRCRESAHA.111.253286
120. Salomon C, Ryan J, Sobrevia L, Kobayashi M, Ashman K, Mitchell M, et al. Exosomal signaling during hypoxia mediates microvascular endothelial cell migration and vasculogenesis. PLoS ONE (2013) 8:e68451. doi: 10.1371/journal.pone.0068451
121. Zhang PF, Su HJ, Zhang M, Li JF, Liu CX, Ding SF, et al. Atherosclerotic plaque components characterization and macrophage infiltration identification by intravascular ultrasound elastography based on b-mode analysis: validation in vivo. Int J Cardiovasc Imaging (2011) 27:39–49. doi: 10.1007/s10554-010-9659-3
122. Xin H, Li Y, Cui Y, Yang JJ, Zhang ZG, Chopp M. Systemic administration of exosomes released from mesenchymal stromal cells promote functional recovery and neurovascular plasticity after stroke in rats. J Cerebral Blood Flow Metab. (2013) 33:1711–5. doi: 10.1038/jcbfm.2013.152
123. Doeppner TR, Herz J, Gorgens A, Schlechter J, Ludwig AK, Radtke S, et al. Extracellular vesicles improve post-stroke neuroregeneration and prevent postischemic immunosuppression. Stem Cells Trans Med. (2015) 4:1131–43. doi: 10.5966/sctm.2013-0118
124. Liang X, Zhang L, Wang S, Han Q, Zhao RC. Exosomes secreted by mesenchymal stem cells promote endothelial cell angiogenesis by transferring miR-125a. J Cell Sci. (2016) 129:2182–9. doi: 10.1242/jcs.170373
125. Khan M, Nickoloff E, Abramova T, Johnson J, Verma SK, Krishnamurthy P, et al. Embryonic stem cell-derived exosomes promote endogenous repair mechanisms and enhance cardiac function following myocardial infarction. Circ Res. (2015) 117:52–64. doi: 10.1161/CIRCRESAHA.117.305990
126. Arslan F, Lai RC, Smeets MB, Akeroyd L, Choo A, Aguor ENE, et al. Mesenchymal stem cell-derived exosomes increase ATP levels, decrease oxidative stress and activate PI3K/Akt pathway to enhance myocardial viability and prevent adverse remodeling after myocardial ischemia/reperfusion injury. Stem Cell Res. (2013) 10:301–12. doi: 10.1016/j.scr.2013.01.002
127. Bian S, Zhang L, Duan L, Wang X, Min Y, Yu H. Extracellular vesicles derived from human bone marrow mesenchymal stem cells promote angiogenesis in a rat myocardial infarction model. J Mol Med. (2014) 92:387–97. doi: 10.1007/s00109-013-1110-5
128. Feng Y, Huang W, Wani M, Yu X, Ashraf M. Ischemic preconditioning potentiates the protective effect of stem cells through secretion of exosomes by targeting Mecp2 via miR-22. PLoS ONE (2014) 9:e0088685. doi: 10.1371/journal.pone.0088685
129. Teng X, Chen L, Chen W, Yang J, Yang Z, Shen Z. Mesenchymal stem cell-derived exosomes improve the microenvironment of infarcted myocardium contributing to angiogenesis and anti-inflammation. Cell Physiol Biochem. (2015) 37:2415–24. doi: 10.1159/000438594
130. Yu B, Kim HW, Gong M, Wang J, Millard RW, Wang Y, et al. Exosomes secreted from GATA-4 overexpressing mesenchymal stem cells serve as a reservoir of anti-apoptotic microRNAs for cardioprotection. Int J Cardiol. (2015) 182:349–360. doi: 10.1016/j.ijcard.2014.12.043
131. Osteikoetxea X, Sódar B, Németh A, Szabó-Taylor K, Pálóczi K, Vukman KV, et al. Differential detergent sensitivity of extracellular vesicle subpopulations. Org Biomol Chem R Soc Chem. (2015) 13:9775–82. doi: 10.1039/C5OB01451D
132. Eirin A, Riester SM, Zhu XY, Tang H, Evans JM, O'Brien D, et al. MicroRNA and mRNA cargo of extracellular vesicles from porcine adipose tissue-derived mesenchymal stem cells. Gene (2014) 551:55–64. doi: 10.1016/j.gene.2014.08.041
133. Eirin A, Zhu XY, Puranik AS, Woollard JR, Tang H, Dasari S, et al. Comparative proteomic analysis of extracellular vesicles isolated from porcine adipose tissue-derived mesenchymal stem/stromal cells. Sci Rep. (2016) 6:36120. doi: 10.1038/srep36120
134. Eirin A, Zhu XY, Puranik AS, Woollard JR, Tang H, Dasari S, et al. Integrated transcriptomic and proteomic analysis of the molecular cargo of extracellular vesicles derived from porcine adipose tissue-derived mesenchymal stem cells. Plos ONE (2017) 12:e0174303. doi: 10.1371/journal.pone.0174303
Keywords: tissue engineered vascular grafts, stem cells, autologous, allogeneic, conditioned media, extracellular vesicles, exosomes
Citation: Cunnane EM, Weinbaum JS, O'Brien FJ and Vorp DA (2018) Future Perspectives on the Role of Stem Cells and Extracellular Vesicles in Vascular Tissue Regeneration. Front. Cardiovasc. Med. 5:86. doi: 10.3389/fcvm.2018.00086
Received: 25 April 2018; Accepted: 13 June 2018;
Published: 03 July 2018.
Edited by:
Joshua D. Hutcheson, Florida International University, United StatesReviewed by:
Chris A. Bashur, Florida Institute of Technology, United StatesWilli Jahnen-Dechent, RWTH Aachen Universität, Germany
Copyright © 2018 Cunnane, Weinbaum, O'Brien and Vorp. This is an openaccess article distributed under the terms of the Creative Commons Attribution License (CC BY). The use, distribution or reproduction in other forums is permitted, provided the original author(s) and the copyright owner(s) are credited and that the original publication in this journal is cited, in accordance with accepted academic practice. No use, distribution or reproduction is permitted which does not comply with these terms.
*Correspondence: David A. Vorp, dm9ycEBwaXR0LmVkdQ==