- 1Laboratory of Stem Cell and Neuro-Vascular Biology, Genetics and Developmental Biology Center, National Heart, Lung, and Blood Institute, Bethesda, MD, United States
- 2Robert W. Franz Cancer Center, Providence Portland Medical Center, Earle A. Chiles Research Institute, Portland, OR, United States
Pericytes are mural cells surrounding blood vessels, adjacent to endothelial cells. Pericytes play critical roles in maturation and maintenance of vascular branching morphogenesis. In the central nervous system (CNS), pericytes are necessary for the formation and regulation of the blood-brain barrier (BBB) and pericyte deficiency accompanies CNS diseases including multiple sclerosis, diabetic retinopathy, neonatal intraventricular hemorrhage, and neurodegenerative disorders. Despite the importance of pericytes, their developmental origins and phenotypic diversity remain incompletely understood. Pericytes express multiple markers and the origin of pericytes differs by tissue, which may cause difficulty for the identification and understanding of the ontogeny of pericytes. Also, pericytes have the potential to give rise to different tissues in vitro but this is not clear in vivo. These studies indicate that pericytes are heterogeneous in a tissue- and context- dependent manner. This short review focuses on recent studies about identification of pericytes, heterogeneous origin of pericytes during development and in adults, and the differentiation capacity of pericytes, and pericytes in pathological settings.
Introduction
Pericytes are mural cells surrounding blood vessels and embedded within the basement membrane of the vasculature and adjacent to endothelial cells (1). Pericytes cover microvessels such as arterioles, venules and capillaries, while large-diameter vessels like arteries and veins are covered by vascular smooth muscle cells (VSMCs), the other type of mural cells. Mural cells are known to play fundamental roles in vascular network formation: pericyte coverage is critical for vascular stability and structure. In the central nervous system (CNS), pericytes play an essential role in the maturation and maintenance of the blood brain barrier (BBB) (2–4), which is established by the interaction between the microvessels (endothelial cells and pericytes) and surrounding astrocytes within the neurovascular unit. The BBB is a diffusion barrier which blocks the inflow of various molecules and toxins from blood to brain, but not all CNS vessels equally contribute to the BBB: several areas of the brain including the pituitary gland, pineal gland, subventricular zones and choroid plexus are not protected by the BBB. The diversity of the BBB architecture and function remains largely unexplored. Given that pericyte density, morphology, and function vary in different vascular beds (5), these observations may reflect their heterogeneous characteristics. In this review, we give an overview of pericytes with a focus on their heterogeneity. A more objective definition of different subsets of pericytes may help to shed light on novel therapeutic approaches for neuro-vascular diseases associated with pericyte loss such as stroke and Alzheimer's disease.
Identification of Pericytes
Morphological characteristics of pericytes were defined by electron microscopy: pericytes possess a cell body with a prominent nucleus and contain a small amount of cytoplasm with several long processes covering the endothelial wall. Pericytes are embedded in the basement membrane where they interact with endothelial cells: pericyte-endothelial cell interaction enhances basement membrane assembly (Figure 1).
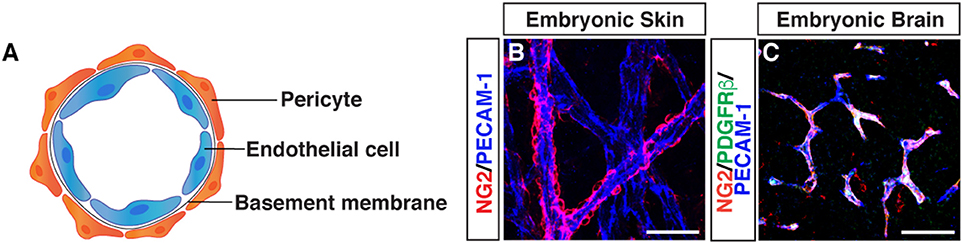
Figure 1. Morphology of pericytes. (A) Schematic image of pericyte-endothelial cell interaction. (B) Whole-mount immunostaining of mouse embryonic skin with antibodies to pericytes (NG2, red) and endothelial cells (PECAM-1, blue) at embryonic day (E) 15.5. Pericytes are semicircular shape and cover blood vessels. (C) Triple immunostaining of E15.5 mouse embryonic brain with antibodies to pericytes (NG2, red; PDGFRβ, green) and endothelial cells (PECAM-1, blue). Scale bars, 50 μm.
Although the ultrastructural characteristics of pericytes has been well studied using electron microscopy, pericytes remain a relatively poorly defined cell type, without highly specific markers available for their identification (6). The following markers have been used as pericyte markers, but no general pan-pericyte molecular marker has been discovered because of their heterogeneous distributions and functions in various tissues (2, 5, 7). We should note that these markers are also expressed by other cell types including perivascular fibroblasts, VSMCs and macrophages. Chondroitin sulfate proteoglycan4/neural glial antigen 2 (NG2) is expressed on the surface of pericytes during angiogenesis (8, 9), but is also expressed on glial precursor O2A cells in the CNS, which generate either oligodendrocytes or astrocytes in vitro. Platelet-derived growth factor receptor beta (PDGFRβ) (10, 11) is one of the most widely studied molecular marker expressed in pericytes. PDGF-B/PDGFRβ signaling is essential for pericyte proliferation and recruitment to blood vessels. Alpha smooth muscle actin (αSMA) (12), desmin (13), and vimentin (14) are contractile filaments. Regulator of G protein signaling 5 (RGS5) (15, 16) is a GTPase activating protein for Giα and Gqα, and the expression pattern of RGS5 overlaps with expression pattern of NG2 and PDGFRβ. CD146, also known as melanoma cell adhesion molecule (MCAM) has been used as a marker for pericytes and VSMCs as well as endothelial cells (17). Recent studies suggest that CD146 regulates PDGFRβ activation and involves in BBB integrity (18) and is essential for pericyte recruitment (19). CD13/aminopeptidase N (APN) (20) is a membrane-bound metalloprotease which was originally identified as a myeloid cell marker (21). CD13 has been used as a surface marker for brain pericytes (22, 23). Overall, current immunohistochemical approaches to identify pericytes use antibodies against these proteins, depending on tissue and microvessel types. The expression of these markers varies depending on developmental stages, organs, pathological situations, and in vitro or in vivo conditions. Therefore, anatomical characteristics combined with at least two molecular markers are important to define pericytes. Indeed, recent studies have revealed that double PDGFRβ-EGFP and NG2-DsRed fluorescence reporter expression patterns are valuable for pericyte/mural cell identification of the CNS tissues (24, 25). Although the expression of αSMA is known as a marker for both pericytes and VSMCs, capillary pericytes do not express αSMA (12): capillary pericytes in embryonic skin express only NG2 and PDGFRβ but not αSMA (26). In contrast, capillary pericytes in tumors do express αSMA (27).
Genetic mouse models including transgenic markers, fluorescent reporters and lineage tracing lines are valuable tools to trace the pericyte lineage during development and in pathological conditions: nuclear β-galactosidase reporter (28) as well as emerging fluorescent reporter and lineage tracing lines using the promoter of PDGFRβ (24, 29), NG2 (24, 30, 31), or Tbx18 (32) are available. Alternatively, a fluorescent Nissl dye specifically labels brain pericytes and enables the imaging in the live mouse (33).
Developmental Origin of Pericytes
The developmental origin of pericytes is heterogeneous, and much remains to be deciphered. Most commonly described, and best understood is their origin from mesenchymal stem cells (34). Chick-quail chimera analysis (35, 36) and genetic lineage tracing experiments using neural crest-specific Cre recombinase lines such as Wnt-1-Cre and Sox10-Cre mice in combination with a Cre-mediated reporter line demonstrate that neural crest contributes to pericytes in the face, brain, and thymus (36–40). Using similar genetic lineage tracing experiments, the origin of pericytes in the gut (41), lung (42), and liver (43) in mice has been traced to the mesothelium, a single layer of squamous epithelium. In the heart, the epicardial mesothelium gives rise to coronary pericytes and VSMCs (44–46). Recent studies have demonstrated that some endocardium also contributes to coronary pericytes (47). These studies clearly indicate that the origin of pericytes is heterogeneous in a tissue- and context- dependent manner.
We have recently revealed that myeloid progenitor cells differentiate into a subset of pericytes in the ectoderm-derived skin and brain during development (Figure 2) (26). Using high-resolution whole-mount imaging and a series of genetic lineage tracing experiments with hematopoietic cell-specific Vav-Cre and myeloid cell-specific CD11b-Cre lines in combination with a Cre-mediated fluorescent reporter line, we found that the developmental sources of pericytes are heterogeneous and some pericytes are derived from myeloid progenitors in the developing skin and brain. Mutant mice lacking myeloid lineage exhibit defective pericyte development. Moreover, TGF-β promotes the differentiation of myeloid progenitors into pericytes in vitro and in vivo. In a similar line of research, some CD31+ F4/80+ macrophages contribute to cerebrovascular pericytes during embryogenesis (48). Insight into the heterogeneous origins of pericytes will have important implications for understanding the establishment of the organ-specific vascular networks during embryonic angiogenesis. Moreover, whether pericytes of different origins have different functions in these tissues remains to be elucidated.
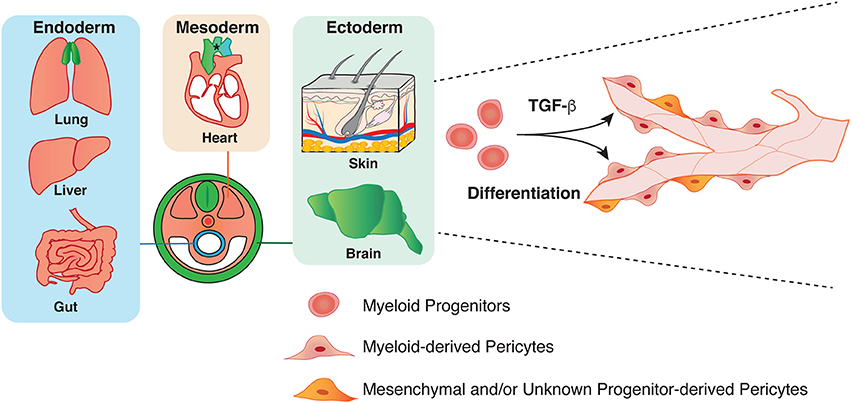
Figure 2. Heterogeneity in the origin of pericytes. Pericytes in both endoderm and mesoderm-derived organs, such as lung, liver, gut, and heart have mesoderm origin (orange). Pericytes in the aorta region have several origins: secondary heart field, neural crest, and somite (asterisk). Previous studies have demonstrated that ectoderm-derived neural crest give rise to pericytes in brain and thymus (green). Our group has recently shown that mesoderm-derived myeloid progenitor cells differentiate into pericytes in ectoderm-derived organs such as skin and brain through TGF-β signaling during development.
It is an intriguing question of whether pericytes of heterogeneous origins at embryonic stages remain in adult: one possible scenario is that a subtype of pericytes may become dominant in adult. Moreover, adult tissue-resident progenitors contribute to pericytes in pathological situations. Indeed, mesenchymal stem cells generate pericytes after radiation therapy (49), while mesenchymal tumors such as bone and soft tissue sarcomas can be derived from pericytes (50). Glioblastoma stem cells generate pericytes to support tumor growth (51). Defining the origin of adult tissue pericytes needs the development of a cell-type specific inducible Cre recombinase line such as CreER. Transient Cre activity produces fluorescent reporter positive cells at a defined developmental time point and then allows for the tracking of their progeny in adults. The inducible lineage tracing experiment also allows us to examine whether pericytes of different origins could differentially contribute to the neovascularization processes in pathological conditions such as tumor angiogenesis and wound healing.
Pericytes in Adult and Diseases
Pericytes have been reported as a component of stem cell niches and mesenchymal stem cells. In the bone marrow, NG2+ pericytes in arterioles promote hematopoietic stem cell (HSC) quiescence and are important for HSC maintenance (52). Likewise, NG2+/Nestin+ pericytes associate with portal blood vessels in fetal liver and are required for the HSC niche (53). In the adult brain, neural stem cells (NSCs) located in the largest germinal region of the forebrain, the ventricular-subventricular zone (V-SVZ). Endothelial cells and pericytes in the V-SVZ form the NSC niche, and V-SVZ pericytes secrete diffusible factors that increase the proliferation and enhance neuronal differentiation (22). In vitro differentiation capacity of pericytes into mesenchymal cell types (e.g., adipocytes, chondrocytes, osteoblasts, fibroblasts, VSMCs) has been proven in a multitude of studies (54–58). Pericytes facilitate repair process after myocardial infarction in the heart (59, 60), while pericytes regenerate injured and dystrophic skeletal muscles (61). In vivo lineage tracing experiments have reported pericytes as progenitors of white adipocytes (62), follicular dendritic cells (63), odontoblasts (mesenchyme-derived dentine producing cells) (64), and skeletal muscle (61). Differentiation capacities of pericytes into neurons, astrocytes, and oligodendrocytes have been also reported (56). In addition, pericytes have been reported to play a major role as fibroblast progenitors in fibrotic responses (65).
Cardiac pericytes have been studied as a therapeutic target after injury. Cardiac pericytes account for up to 5% of the total non-cardiomyocyte cell population (66). Recent studies identified microvascular pericytes in the human ventricular myocardium and demonstrated that human cardiac pericytes express mesenchymal stem/stromal cell markers including CD44, CD73, CD90, and CD105 (58). Indeed, cardiac pericytes have the capacity to differentiate into mesodermal lineage: osteo-, chondro-, and adipogenesis, but no potential for skeletal myogenesis in vitro (58). Many cardiac diseases are associated with fibrosis, an accumulation of fibroblasts and an excess of extracellular matrix proteins which may affect the architecture and function of the organ or the tissue. Pericytes have been shown to contribute to fibrosis organ-dependently (67): NG2+/Nestin− type 1 pericytes are recruited and accumulated in the ischemic interstitial space around fibrotic tissue but do not contribute to fibrosis (67).
However, a series of lineage tracing experiments with Tbx18CreERT2 line, which is selectively expressed by pericytes and VSMCs in multiple adult organs, has revealed that adult pericytes of heart, brain, skeletal muscle and fat depots do not behave as multipotent progenitors in aging and different pathological situations such as a high-fat diet and injury. In addition, pericytes do not contribute to fibroblasts in fibrotic responses (32). These results are in contrast to the previous studies that demonstrated the multipotent potential of pericytes. The discrepancies might be explained by the differing methods used in the studies to identify pericytes as well as the different behavior of in vitro and in vivo pericytes. The population of pericytes which can differentiate into mesenchymal cell types may vary by organ and developmental stage in vivo. It is also possible that pericyte differentiation requires stem cell-like cells of non-pericyte origin (64).
Conclusion
The absence of any single marker to identify pericytes complicates the study of the ontogeny and the differentiation capacity of pericytes. Much remains to be elucidated regarding how and when the origin of pericytes in an organ is determined and whether pericytes have potential for differentiation in vivo. There are questions about how pericytes derived from different origins behave when they co-exist in a tissue: do they show identical gene expression patterning and behavior in adult tissues under normal and pathological conditions? Future studies using pericyte-specific lineage tracing mice and precise gene-expression profiling will pave the way for the understanding of the spatial-temporal pericyte behavior in vivo and may contribute to new therapeutic strategies for diseases associated with pericyte loss and/or dysfunction.
Author Contributions
Both authors listed have made a substantial, direct and intellectual contribution to the work, and approved it for publication.
Funding
YM is supported by the Intramural Research Program of the National Heart, Lung, and Blood Institute (HL005702-11). TY was supported by the Japan Society for the Promotion of Science (JSPS) NIH-KAITOKU.
Conflict of Interest Statement
The authors declare that the research was conducted in the absence of any commercial or financial relationships that could be construed as a potential conflict of interest.
Acknowledgments
We thank K. Gill for editorial help and laboratory management, and R. Reed for administrative assistance.
References
1. Armulik A, Abramsson A, Betsholtz C. Endothelial/pericyte interactions. Circ Res. (2005) 97:512–23. doi: 10.1161/01.RES.0000182903.16652.d7
2. Armulik A, Genove G, Mae M, Nisancioglu MH, Wallgard E, Niaudet C, et al. Pericytes regulate the blood-brain barrier. Nature (2010) 468:557–61. doi: 10.1038/nature09522
3. Daneman R, Zhou L, Kebede AA, Barres BA. Pericytes are required for blood-brain barrier integrity during embryogenesis. Nature (2010) 468:562–6. doi: 10.1038/nature09513
4. Bell RD, Winkler EA, Sagare AP, Singh I, LaRue B, Deane R. Pericytes control key neurovascular functions and neuronal phenotype in the adult brain and during brain aging. Neuron (2010) 68:409–27. doi: 10.1016/j.neuron.2010.09.043
5. Bergers G, Song S. The role of pericytes in blood-vessel formation and maintenance. Neuro Oncol. (2005) 7:452–64. doi: 10.1215/S1152851705000232
6. Armulik A, Genove G, Betsholtz C. Pericytes: developmental, physiological, and pathological perspectives, problems, and promises. Dev Cell. (2011) 21:193–215. doi: 10.1016/j.devcel.2011.07.001
7. Trost A, Lange S, Schroedl F, Bruckner D, Motloch KA, Bogner B, et al. Brain and retinal pericytes: origin, function and role. Front Cell Neurosci. (2016) 10:20. doi: 10.3389/fncel.2016.00020
8. Ozerdem U, Grako KA, Dahlin-Huppe K, Monosov E, Stallcup WB. NG2 proteoglycan is expressed exclusively by mural cells during vascular morphogenesis. Dev Dyn. (2001) 222:218–27. doi: 10.1002/dvdy.1200
9. Stallcup WB. The NG2 proteoglycan: past insights and future prospects. J Neurocytol. (2002) 31:423–35. doi: 10.1023/A:1025731428581
10. Lindahl P, Johansson BR, Leveen P, Betsholtz C. Pericyte loss and microaneurysm formation in PDGF-B-deficient mice. Science (1997) 277:242–5. doi: 10.1126/science.277.5323.242
11. Winkler EA, Bell RD, Zlokovic BV. Pericyte-specific expression of PDGF beta receptor in mouse models with normal and deficient PDGF beta receptor signaling. Mol Neurodegener (2010) 5:32. doi: 10.1186/1750-1326-5-32
12. Nehls V, Drenckhahn D. Heterogeneity of microvascular pericytes for smooth muscle type alpha-actin. J Cell Biol. (1991) 113:147–54. doi: 10.1083/jcb.113.1.147
13. Nehls V, Denzer K, Drenckhahn D. Pericyte involvement in capillary sprouting during angiogenesis in situ. Cell Tissue Res. (1992) 270:469–74. doi: 10.1007/BF00645048
14. Bandopadhyay R, Orte C, Lawrenson JG, Reid AR, De Silva S, Allt G. Contractile proteins in pericytes at the blood-brain and blood-retinal barriers. J Neurocytol. (2001) 30:35–44. doi: 10.1023/A:1011965307612
15. Cho H, Kozasa T, Bondjers C, Betsholtz C, Kehrl JH. Pericyte-specific expression of Rgs5: implications for PDGF and EDG receptor signaling during vascular maturation. FASEB J. (2003) 17:440–2. doi: 10.1096/fj.02-0340fje
16. Mitchell TS, Bradley J, Robinson GS, Shima DT, Ng YS. RGS5 expression is a quantitative measure of pericyte coverage of blood vessels. Angiogenesis (2008) 11:141–51. doi: 10.1007/s10456-007-9085-x
17. Crisan M, Yap S, Casteilla L, Chen CW, Corselli M, Park TS, et al. A perivascular origin for mesenchymal stem cells in multiple human organs. Cell Stem Cell (2008) 3:301–13. doi: 10.1016/j.stem.2008.07.003
18. Chen J, Luo Y, Hui H, Cai T, Huang H, Yang F, et al. CD146 coordinates brain endothelial cell-pericyte communication for blood-brain barrier development. Proc Natl Acad Sci USA. (2017) 114:E7622–31. doi: 10.1073/pnas.1710848114
19. Chen J, Luo Y, Huang H, Wu S, Feng J, Zhang J, et al. CD146 is essential for PDGFRbeta-induced pericyte recruitment. Protein Cell (2017). doi: 10.1007/s13238-017-0484-5. [Epub ahead of print].
20. Alliot F, Rutin J, Leenen PJ, Pessac B. Pericytes and periendothelial cells of brain parenchyma vessels co-express aminopeptidase N, aminopeptidase A, and nestin. J Neurosci Res. (1999) 58:367–78. doi: 10.1002/(SICI)1097-4547(19991101)58:3<367::AID-JNR2>3.0.CO;2-T
21. Favaloro EJ, Bradstock KF, Kabral A, Grimsley P, Zowtyj H, Zola H. Further characterization of human myeloid antigens (gp160,95; gp150; gp67): investigation of epitopic heterogeneity and non-haemopoietic distribution using panels of monoclonal antibodies belonging to CD-11b, CD-13 and CD-33. Br J Haematol. (1988) 69:163–71. doi: 10.1111/j.1365-2141.1988.tb07618.x
22. Crouch EE, Liu C, Silva-Vargas V, Doetsch F. Regional and stage-specific effects of prospectively purified vascular cells on the adult V-SVZ neural stem cell lineage. J Neurosci. (2015) 35:4528–39. doi: 10.1523/JNEUROSCI.1188-14.2015
23. Crouch EE, Doetsch F. FACS isolation of endothelial cells and pericytes from mouse brain microregions. Nat Protoc. (2018) 13:738–51. doi: 10.1038/nprot.2017.158
24. Jung B, Arnold TD, Raschperger E, Gaengel K, Betsholtz C. Visualization of vascular mural cells in developing brain using genetically labeled transgenic reporter mice. J Cereb Blood Flow Metab. (2018) 38:456–68. doi: 10.1177/0271678X17697720
25. Vanlandewijck M, He L, Mae MA, Andrae J, Ando K, Del Gaudio F. A molecular atlas of cell types and zonation in the brain vasculature. Nature (2018) 554:475–80. doi: 10.1038/nature25739
26. Yamazaki T, Nalbandian A, Uchida Y, Li W, Arnold TD, Kubota Y, et al. Tissue myeloid progenitors differentiate into pericytes through TGF-beta signaling in developing skin vasculature. Cell Rep. (2017) 18:2991–3004. doi: 10.1016/j.celrep.2017.02.069
27. Morikawa S, Baluk P, Kaidoh T, Haskell A, Jain RK, McDonald DM. Abnormalities in pericytes on blood vessels and endothelial sprouts in tumors. Am J Pathol. (2002) 160:985–1000. doi: 10.1016/S0002-9440(10)64920-6
28. Tidhar A, Reichenstein M, Cohen D, Faerman A, Copeland NG, Gilbert DJ, et al. A novel transgenic marker for migrating limb muscle precursors and for vascular smooth muscle cells. Dev Dyn. (2001) 220:60–73. doi: 10.1002/1097-0177(2000)9999:9999<::AID-DVDY1089>3.0.CO;2-X
29. Cuervo H, Pereira B, Nadeem T, Lin M, Lee F, Kitajewski J, et al. PDGFRbeta-P2A-CreERT2 mice: a genetic tool to target pericytes in angiogenesis. Angiogenesis (2017) 20:655–62. doi: 10.1007/s10456-017-9570-9
30. Zhu X, Hill RA, Nishiyama A. NG2 cells generate oligodendrocytes and gray matter astrocytes in the spinal cord. Neuron Glia Biol. (2008) 4:19–26. doi: 10.1017/S1740925X09000015
31. Hill RA, Patel KD, Goncalves CM. Grutzendler J, Nishiyama A, Modulation of oligodendrocyte generation during a critical temporal window after NG2 cell division. Nat Neurosci. (2014) 17:1518–27. doi: 10.1038/nn.3815
32. Guimaraes-Camboa N, Cattaneo P, Sun Y, Moore-Morris T, Gu Y, et al. Pericytes of multiple organs do not behave as mesenchymal stem cells in vivo. Cell Stem Cell (2017) 20:345.e5–359e5. doi: 10.1016/j.stem.2016.12.006
33. Damisah EC, Hill RA, Tong L, Murray KN, Grutzendler J. A fluoro-Nissl dye identifies pericytes as distinct vascular mural cells during in vivo brain imaging. Nat Neurosci. (2017) 20:1023–32. doi: 10.1038/nn.4564
34. Creazzo TL, Godt RE, Leatherbury L, Conway SJ, Kirby ML. Role of cardiac neural crest cells in cardiovascular development. Annu Rev Physiol. (1998) 60:267–86. doi: 10.1146/annurev.physiol.60.1.267
35. Etchevers HC, Vincent C, Le Douarin NM, Couly GF. The cephalic neural crest provides pericytes and smooth muscle cells to all blood vessels of the face and forebrain. Development (2001) 128:1059–68.
36. Korn J, Christ B, Kurz H. Neuroectodermal origin of brain pericytes and vascular smooth muscle cells. J Comp Neurol. (2002) 442:78–88. doi: 10.1002/cne.1423
37. Muller SM, Stolt CC, Terszowski G, Blum C, Amagai T, Kessaris N, et al. Neural crest origin of perivascular mesenchyme in the adult thymus. J Immunol. (2008) 180:5344–51. doi: 10.4049/jimmunol.180.8.5344
38. Foster K, Sheridan J, Veiga-Fernandes H, Roderick K, Pachnis V, Adams R, et al. Contribution of neural crest-derived cells in the embryonic and adult thymus. J Immunol. (2008) 180:3183–9. doi: 10.4049/jimmunol.180.5.3183
39. Reyahi A, Nik AM, Ghiami M, Gritli-Linde A, Ponten F, Johansson BR, et al. Foxf2 is required for brain pericyte differentiation and development and maintenance of the blood-brain barrier. Dev Cell (2015) 34:19–32. doi: 10.1016/j.devcel.2015.05.008
40. Yamanishi E, Takahashi M, Saga Y, Osumi N. Penetration and differentiation of cephalic neural crest-derived cells in the developing mouse telencephalon. Dev Growth Differ. (2012) 54:785–800. doi: 10.1111/dgd.12007
41. Wilm B, penberg A, Hastie ND, Burch JB, Bader DM. The serosal mesothelium is a major source of smooth muscle cells of the gut vasculature. Development (2005) 132:5317–28. doi: 10.1242/dev.02141
42. Que J, Wilm B, Hasegawa H, Wang F, Bader D, Hogan BL. Mesothelium contributes to vascular smooth muscle and mesenchyme during lung development. Proc Natl Acad Sci USA. (2008) 105:16626–30. doi: 10.1073/pnas.0808649105
43. Asahina K, Zhou B, Pu WT, Tsukamoto H. Septum transversum-derived mesothelium gives rise to hepatic stellate cells and perivascular mesenchymal cells in developing mouse liver. Hepatology (2011) 53:983–95. doi: 10.1002/hep.24119
44. Dettman RW, Denetclaw W Jr. Ordahl CP, Bristow J. Common epicardial origin of coronary vascular smooth muscle, perivascular fibroblasts, and intermyocardial fibroblasts in the avian heart. Dev Biol. (1998) 193:169–81. doi: 10.1006/dbio.1997.8801
45. Mikawa T, Gourdie RG. Pericardial mesoderm generates a population of coronary smooth muscle cells migrating into the heart along with ingrowth of the epicardial organ. Dev Biol. (1996) 174:221–32. doi: 10.1006/dbio.1996.0068
46. Zhou B, Ma Q, Rajagopal S, Wu SM, Domian I, Rivera-Feliciano J, et al. Epicardial progenitors contribute to the cardiomyocyte lineage in the developing heart. Nature (2008) 454:109–13. doi: 10.1038/nature07060
47. Chen Q, Zhang H, Liu Y, Adams S, Eilken H, Stehling M, et al. Endothelial cells are progenitors of cardiac pericytes and vascular smooth muscle cells. Nat Commun. (2016) 7:12422. doi: 10.1038/ncomms12422
48. Yamamoto S, Muramatsu M, Azuma E, Ikutani M, Nagai Y, Sagara H, et al. A subset of cerebrovascular pericytes originates from mature macrophages in the very early phase of vascular development in CNS. Sci Rep. (2017) 7:3855. doi: 10.1038/s41598-017-03994-1
49. Wang HH, Cui YL, Zaorsky NG, Lan J, Deng L, Zeng XL, et al. Mesenchymal stem cells generate pericytes to promote tumor recurrence via vasculogenesis after stereotactic body radiation therapy. Cancer Lett. (2016) 375:349–59. doi: 10.1016/j.canlet.2016.02.033
50. Sato S, Tang YJ, Wei Q, Hirata M, Weng A, Han I, et al. Mesenchymal tumors can derive from Ng2/Cspg4-expressing pericytes with beta-catenin modulating the neoplastic phenotype. Cell Rep. (2016) 16:917–27. doi: 10.1016/j.celrep.2016.06.058
51. Cheng L, Huang Z, Zhou W, Wu Q, Donnola S, Liu JK, et al. Glioblastoma stem cells generate vascular pericytes to support vessel function and tumor growth. Cell (2013) 153:139–52. doi: 10.1016/j.cell.2013.02.021
52. Kunisaki Y, Bruns I, Scheiermann C, Ahmed J, Pinho S, Zhang D, et al. Arteriolar niches maintain haematopoietic stem cell quiescence. Nature (2013) 502:637–43. doi: 10.1038/nature12612
53. Khan JA, Mendelson A, Kunisaki Y, Birbrair A, Kou Y, Arnal-Estape A, et al. Fetal liver hematopoietic stem cell niches associate with portal vessels. Science (2016) 351:176–80. doi: 10.1126/science.aad0084
54. Farrington-Rock C, Crofts NJ, Doherty MJ, Ashton BA, Griffin-Jones C, Canfield AE. Chondrogenic and adipogenic potential of microvascular pericytes. Circulation (2004) 110:2226–32. doi: 10.1161/01.CIR.0000144457.55518.E5
55. Collett G, Wood A, Alexander MY, Varnum BC, Boot-Handford RP, Ohanian V, et al. Receptor tyrosine kinase Axl modulates the osteogenic differentiation of pericytes. Circ Res. (2003) 92:1123–9. doi: 10.1161/01.RES.0000074881.56564.46
56. Dellavalle A, Sampaolesi M, Tonlorenzi R, Tagliafico E, Sacchetti B, Perani L, et al. Pericytes of human skeletal muscle are myogenic precursors distinct from satellite cells. Nat Cell Biol. (2007) 9:255–67. doi: 10.1038/ncb1542
57. Doherty MJ, Ashton BA, Walsh S, Beresford JN, Grant ME, Canfield AE. Vascular pericytes express osteogenic potential in vitro and in vivo. J Bone Miner Res. (1998) 13:828–38. doi: 10.1359/jbmr.1998.13.5.828
58. Chen WC, Baily JE, Corselli M, Diaz ME, Sun B, Xiang G, et al. Human myocardial pericytes: multipotent mesodermal precursors exhibiting cardiac specificity. Stem Cells (2015) 33:557–73. doi: 10.1002/stem.1868
59. Katare R, Riu F, Mitchell K, Gubernator M, Campagnolo P, Cui Y, et al. Transplantation of human pericyte progenitor cells improves the repair of infarcted heart through activation of an angiogenic program involving micro-RNA-132. Circ Res. (2011) 109:894–906. doi: 10.1161/CIRCRESAHA.111.251546
60. Chen CW, Okada M, Proto JD, Gao X, Sekiya N, Beckman SA, et al. Human pericytes for ischemic heart repair. Stem Cells (2013) 31:305–16. doi: 10.1002/stem.1285
61. Dellavalle A, Maroli G, Covarello D, Azzoni E, Innocenzi A, Perani L, et al. Pericytes resident in postnatal skeletal muscle differentiate into muscle fibres and generate satellite cells. Nat Commun. (2011) 2:499. doi: 10.1038/ncomms1508
62. Tang W, Zeve D, Suh JM, Bosnakovski D, Kyba M, Hammer RE, et al. White fat progenitor cells reside in the adipose vasculature. Science (2008) 322:583–6. doi: 10.1126/science.1156232
63. Krautler NJ, Kana V, Kranich J, Tian Y, Perera D, Lemm D, et al. Follicular dendritic cells emerge from ubiquitous perivascular precursors. Cell (2012) 150:194–206. doi: 10.1016/j.cell.2012.05.032
64. Feng J, Mantesso A, De Bari C, Nishiyama A, Sharpe PT. Dual origin of mesenchymal stem cells contributing to organ growth and repair. Proc Natl Acad Sci USA. (2011) 108:6503–8. doi: 10.1073/pnas.1015449108
65. Goritz C, Dias DO, Tomilin N, Barbacid M, Shupliakov O, Frisen J. A pericyte origin of spinal cord scar tissue. Science (2011) 333:238–42. doi: 10.1126/science.1203165
66. Pinto AR, Ilinykh A, Ivey MJ, Kuwabara JT, D'Antoni ML, Debuque R, et al. Revisiting cardiac cellular composition. Circ Res. (2016) 118:400–9. doi: 10.1161/CIRCRESAHA.115.307778
Keywords: pericyte, development, differentiation capacity, origin, heterogeneity
Citation: Yamazaki T and Mukouyama Y (2018) Tissue Specific Origin, Development, and Pathological Perspectives of Pericytes. Front. Cardiovasc. Med. 5:78. doi: 10.3389/fcvm.2018.00078
Received: 14 April 2018; Accepted: 07 June 2018;
Published: 27 June 2018.
Edited by:
Masanori Aikawa, Harvard Medical School, United StatesReviewed by:
Xin Zhang, University of Oklahoma Health Sciences Center, United StatesJames B. Hoying, Cardiovascular Innovation Institute (CII), United States
Copyright © 2018 Yamazaki and Mukouyama. This is an open-access article distributed under the terms of the Creative Commons Attribution License (CC BY). The use, distribution or reproduction in other forums is permitted, provided the original author(s) and the copyright owner are credited and that the original publication in this journal is cited, in accordance with accepted academic practice. No use, distribution or reproduction is permitted which does not comply with these terms.
*Correspondence: Yoh-suke Mukouyama, bXVrb3lhbWF5QG1haWwubmloLmdvdg==