- Universidade Federal do Paraná (UFPR), Chemistry Departament, Curitiba, Brazil
Graphene oxide (GO) has attracted significant attention from the scientific community due to its mechanical, optical, electrical, and chemical properties. This review outlines synthetic methods for GO functionalization, including those involving covalent and noncovalent bonds with organic molecules. In a novel contribution to this field, particular emphasis is placed on functionalization via epoxy ring opening, a poorly studied and understood topic. We first provide an overview of the basic structure and properties of graphene oxide. We then explore the various methods employed to functionalize graphene oxide, noting the complexity of these reactions, which sometimes occur in a non-specific manner. However, there are some strategies for targeted functionalization. Furthermore, we present a critical analysis of the covalent functionalization through epoxy groups, demonstrating important aspects to be considered when choosing the reaction medium. An alkaline environment seems to favor this reaction, and there is no consensus regarding the advantages and disadvantages of using basic pH in functionalization reactions. We also demonstrate some challenges involving the characterization and confirmation of the functionalization, mainly in the basal plane, and we show advances in characterization techniques that can be explored in future studies. Finally, some current challenges and future research directions are presented to contribute to the advancement of the field.
1 Introduction
Graphene oxide (GO) is a remarkable material derived from graphene, featuring a two-dimensional arrangement of carbon atoms bonded in hexagons along with oxygen functional groups (Brisebois and Siaj, 2020; Vacchi et al., 2020; Guo et al., 2022; Donato et al., 2023). This unique structure enables GO to disperse in aqueous solutions and serve as a support for various functional groups, enabling a multitude of applications. These applications range from sensors (Shahdost-fard and Roushani, 2017; Devi et al., 2018; Jayaraman et al., 2022; Alves et al., 2023) and catalysts (Xue et al., 2015; Flores et al., 2020; Hao et al., 2020; Hostert et al., 2020; Santos et al., 2022; Santos et al., 2023; Xie et al., 2023) to membranes (Kumar et al., 2012; Nair et al., 2012; Zhang et al., 2015; Feng et al., 2018; Meng et al., 2019; Tan et al., 2022), solar cells (Liu et al., 2012; Lima et al., 2016; Gao et al., 2022), energy storage (Georgakilas et al., 2016; Zhang et al., 2016; Payami et al., 2020; Tian et al., 2021), and even biomedical therapies (Mirzaie et al., 2019; Monteiro et al., 2020; Ranishenka et al., 2021).
One of the most utilized techniques for obtaining GO involves the chemical oxidation of graphite, followed by exfoliation. However, this process introduces structural defects in the sp2 network due to the oxidative cutting of graphene sheets (Komeily-Nia et al., 2021). Although the oxygenated groups are spread throughout the whole structure, the defect regions present a different prioritization of groups (Feicht and Eigler, 2018). Epoxides, a functional group found in GO, are formed during the initial stages of oxidation due to their kinetic favorability (Pancharatna et al., 2020). Apart from epoxides, GO also contains other oxygenated groups such as carboxyl, hydroxyl, and carbonyl groups, which can be found in the edges and defects in the structure. The composition of these groups varies depending on the preparation method and the extent of oxidation (Komeily-Nia et al., 2021). Consequently, GO is not a material with a fixed composition and structure but rather a material with significant variation in size and oxidation degree (Donato et al., 2023).
It is crucial to acknowledge the structural complexity when studying the functionalization and application of GO in various fields. Several models have been proposed for GO structure, three of which are highlighted: the L-K model introduced by Lerf and Klinowski (Figure 1A) (Lerf et al., 1998), the two-component structure model (Rourke et al., 2011), and the Dynamic Structure Model (DSM) (Dimiev, 2016) (Figure 1B).
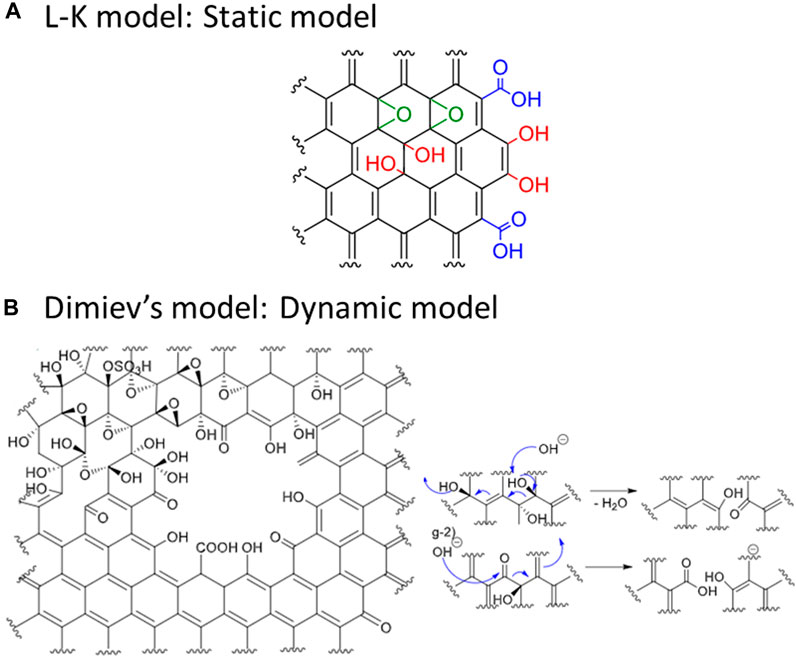
Figure 1. Structure models of GO. (A) LK model, static model (Kasprzak et al., 2018). (B) Dimiev’s model, dynamic model (Monteiro et al., 2020). Reprinted with permission from Beilstein-Institut (2018) and Wiley-VCH (2020).
These models provide valuable insights into the structure of GO and its properties. For example, the L-K model suggests that the epoxy and hydroxyl functional groups are attached to the basal plane of GO, while the carboxyl functional groups are linked to the edges. This model has been updated to explain the high acidity, defects, and partial heterogeneity of GO. Among these updates, Rourke et al. (2011) have suggested the partial heterogeneity of GO obtained by the Hummers method. Rourke’s “two-component structural model” divides GO into bwGO (base-washed graphene oxide) and OD (oxygenated debris), relating its properties based on these two components and their interaction. ODs are small fragments that can be separated from GO by alkaline washing, accounting for about one-third of its total mass. In neutral or acidic environments, ODs are tightly bonded to GO, while in alkaline environments, the deprotonation of functional groups in OD detaches it from bwGO. This process cannot be reversed once the components are separated. Dimiev (2016) proposed a dynamic structure model (DSM) for GO based on a study of the origin and structural evolution of its acidity. This model attributes the acidity of GO to its enol groups and suggests that the structure of GO is constantly changing in the presence of water. The study also reported a significant change between the freshly prepared GO and the same GO after 2 months.
An increasing number of researchers are now exploring the anchoring of various chemical species in GO, thus creating novel composites with unique chemical and physical properties and expanding their applications (Yu et al., 2020). This can be achieved through both non-covalent (involving intermolecular interactions) and covalent functionalization involving reactions between carboxylic acids, hydroxyls, or epoxides of graphene oxide with other molecules (Yu et al., 2020). A known challenge in this area is corroborating the intended modification with different characterization techniques and answering questions such as where the modification was made, what group was anchored, and what the degree of functionalization is (Kasprzak et al., 2018; Vacchi et al., 2020).
The covalent functionalization approach, which will be the main scope of this review, utilizes a range of classic organic reactions, including carboxylic acids, alcohols, and epoxides (Kasprzak et al., 2018). This field is still very challenging since it is difficult to extend organic reactions to complex structures such as GO (Kasprzak et al., 2018). For example, shifts in reactivity sometimes require changes to the reaction conditions, such as solvent and temperature. Derivatization in carboxylic acid groups via amidation or esterification is a popular choice for the chemical modification of GO—extensively discussed in Georgakilas et al. (2012), Yu et al. (2020), Guo et al. (2022), and Liu et al. (2022) —and offers the benefits of mild pH and temperature conditions (Vacchi et al., 2020). However, this approach is limited by the low quantity of carboxylic acid groups due to their location on the GO sheets’ edges (Guo et al., 2020a).
On the other hand, the functionalization of basal groups, particularly epoxides, has been significantly overlooked, as Kumar et al. (2022) have highlighted. Both hydroxyls and epoxides can be functionalized, but epoxides are more susceptible to nucleophilic attack and will be the focus of this review. Nucleophilic attack promotes epoxide ring opening, with the nucleophile becoming covalently attached to the GO (Thomas et al., 2014; Taniguchi et al., 2015; Ranishenka et al., 2021; Kumar et al., 2022). Researchers have highlighted several advantages of this method, including the abundance of epoxides and the absence of coupling reagents commonly used in functionalization via carboxylic acids (Ranishenka et al., 2021; Kumar et al., 2022). Furthermore, the opening of epoxides generates additional hydroxyls that can be functionalized in subsequent reactions (Khan et al., 2021). Also, the modification of basal oxygen groups can yield materials with specific properties distinct from those functionalized at the edges; it is important to explore these differences thoroughly. However, there are also some drawbacks and limitations associated with this approach. First, the characterization and quantification of epoxide groups in GO are challenging as they are in a region where there are many steric hindrances (Piñeiro-García and Semetey, 2023); thus, differentiating hydroxyls and epoxides using spectroscopic methods is difficult (Rabchinskii et al., 2022). Second, there is a preference for alkaline media in these reactions (Taniguchi et al., 2015; Fallah-Mehrjardi et al., 2018; Piñeiro-García and Semetey, 2023), although certain studies caution against functionalization under alkaline conditions due to the potential reduction and destabilization of the GO colloidal system (Guo et al., 2020a; Guo et al., 2020b; Vacchi et al., 2020; Guo et al., 2022). It is important to highlight that some researchers seek out the effects evoked by functionalization via epoxide in an alkaline medium and do not view it as a drawback (Gao et al., 2010; Thomas et al., 2013; Thomas et al., 2014; Kumar et al., 2022). This will be evident when we discuss the pH effects on these reactions.
In fact, the functionalization route via GO epoxides is very promising, but there are still several crucial aspects that require thorough analysis and discussion. We have thus conducted a comprehensive review, compiling studies that have employed this route, showing the proposed mechanisms, and highlighting the main characterization techniques as well as the difficulties associated with them. We expect that this research will contribute to the advancement of studies on the functionalization of GO and provide clarity for future investigations in this field.
2 Strategies for functionalizing GO
Two primary approaches exist in the field of GO functionalization: covalent and non-covalent (Yu et al., 2020; Liu et al., 2022). In this section, we will discuss some general aspects regarding these two types of functionalization, placing particular emphasis on covalent functionalization targeting carbon atoms or oxygen functional groups of GO. This excludes the discussion of functionalization through epoxy groups, which will be detailed in subsequent sections. Additionally, we will explore cases where multiple molecules are incorporated into distinct functional sites of GO, resulting in multifunctionalization.
Non-covalent functionalization is the simplest way of functionalizing; (Table 1) it involves the incorporation of molecules, ions, atoms, or particles of interest into GO through diverse intermolecular interactions (Georgakilas et al., 2012; Georgakilas et al., 2016). For example, molecules that possess a π-conjugate system can be introduced to GO via π–π stacking interaction with the aromatic rings of the graphene plane. The success of this interaction is influenced by factors such as the molecule’s ability to donate or withdraw electrons, its planarity, and its molecular size (Georgakilas et al., 2016). The surface of graphene can also interact with molecules or polymers with high hydrophobic characteristics through London scattering forces. On the other hand, the oxygenated functions present on the surface of GO allow Keesom (permanent dipole–permanent dipole) and Debye (permanent dipole–induced dipole) interactions, hydrogen bonds, and ion–dipole interactions (Georgakilas et al., 2012; Georgakilas et al., 2016). Figure 2 illustrates non-covalent interactions between graphene oxide and pharmaceutical molecules (PhAs).
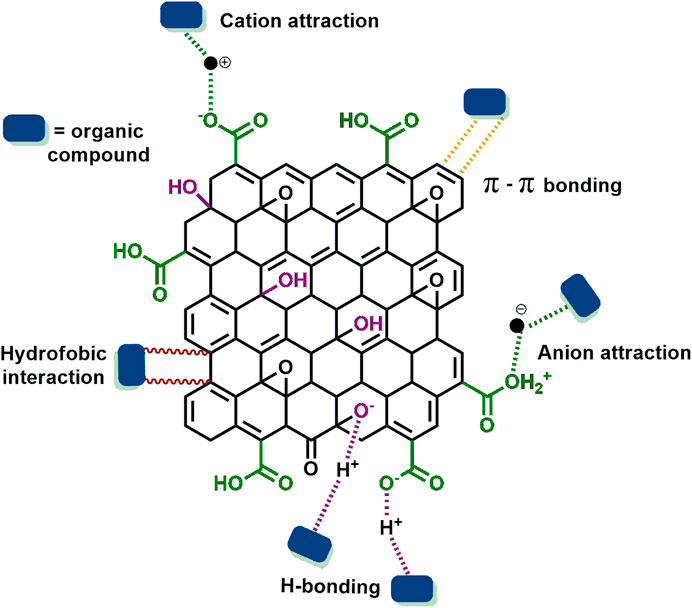
Figure 2. Non-covalent interaction between graphene oxide and pharmaceuticals (PhAs). See Khan et al. (2017).
Georgakilas et al. (2012) comprehensively reviewed the non-covalent functionalization of graphene oxide and demonstrated the diverse range of molecules that can be incorporated based on specific interaction types. Polymers and carbon allotropes (such as fullerenes) via π–π interactions can be added for application in solar cells and the formation of gels and thin films, respectively. Furthermore, the incorporation of medicines and biomolecules through hydrogen bonds with oxygenated functional groups can enable biomedical applications.
Despite the simplicity and maintenance of the chemical structure of graphene oxide, there is a drawback when it comes to materials obtained by non-covalent functionalization: the weakly bound molecules can be easily lixiviated (Georgakilas et al., 2012; Lopez and Liu, 2020). Covalent functionalization addresses this drawback by creating stronger attachments between the molecules and the GO structure (Georgakilas et al., 2012; Lopez and Liu, 2020), which is crucial for applications that require material durability over multiple cycles, such as catalysis (Orth et al., 2013; Silvestri et al., 2022). The main reactions of covalent functionalization will be discussed below (see also Figure 3).
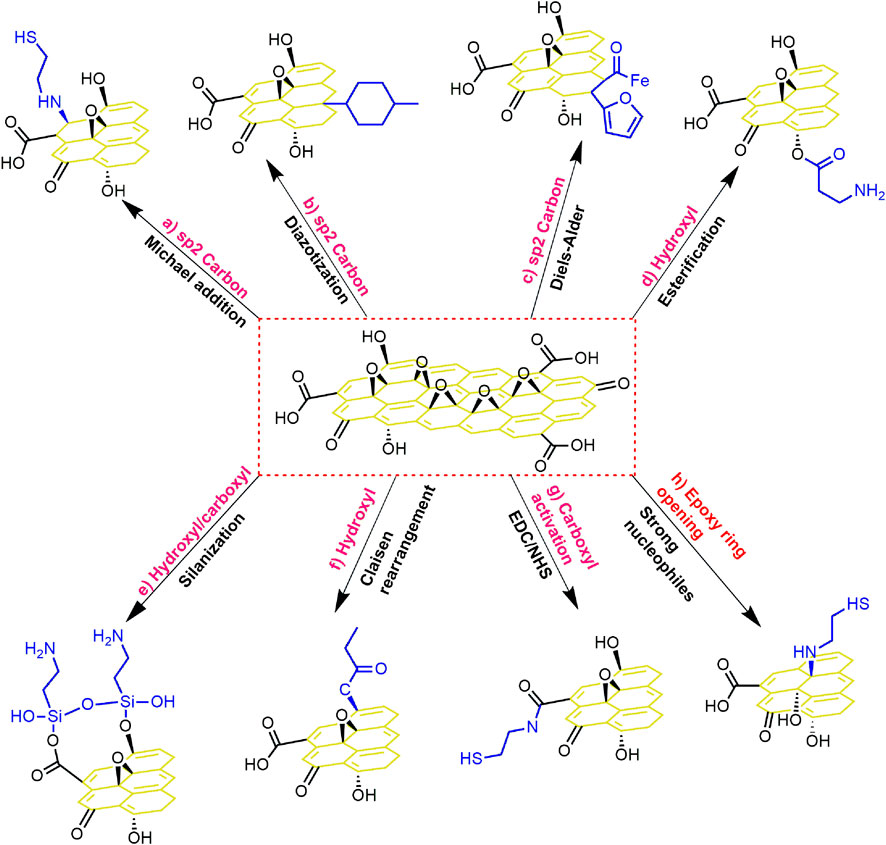
Figure 3. Examples of covalent functionalization through different routes in GO. (A) Michael addition (Piñeiro-García et al., 2021). (B) Diazotization (Ossonon and Bélanger, 2017). (C) Diels–Alder (Rahimpour et al., 2022). (D) Esterification (Zhang et al., 2011). (E) Silanization (Serodre et al., 2019). (F) Claisen rearrangement (Collins et al., 2011a), (G) Q31 Carboxyl activation with EDC/NHS (Santos et al., 2022). (H) Epoxy-ring opening (Santos et al., 2023).
When covalent functionalization is employed, reactions can occur directly in the sp2 carbon network or in the oxygenated functional groups of the graphene oxide (Yu et al., 2020; Liu et al., 2022). Some methodologies that primarily focus on functionalizing the carbon plane are thiol-ene reactions (including the Michael addition) (Piñeiro-García et al., 2021; Piñeiro-García and Semetey, 2023) (Figures 3A), diazotization (Ossonon and Bélanger, 2017) (Figures 3B), and Diels–Alder reactions (Tang et al., 2017) (Figures 3C). The thiol-ene process involves the addition of a thiol compound to an alkene in an anti-Markovnikov orientation (Piñeiro-García et al., 2021; Piñeiro-García et al., 2022; Piñeiro-García and Semetey, 2023); it encompasses thiol-ene radical addition and thiol-ene Michael addition, initiated by radical or base catalysts, respectively (Piñeiro-García and Semetey, 2023). Thiol-ene radical addition occurs in three stages. Firstly, an initiating radical interacts with a thiol molecule, forming thiyl radicals. These then react with a carbon–carbon double bond, creating a central radical in the adjacent carbon. Subsequently, the newly formed central carbon radical can stabilize by extracting a hydrogen atom from another thiol molecule, generating a fresh thiyl radical that perpetuates the reaction (Piñeiro-García et al., 2021).
A thiol-ene Michael addition (Figures 4A,B) involves adding a nucleophile to the β carbon of an α,β-unsaturated carbonyl or carboxyl acid (Guo et al., 2020b; Piñeiro-García et al., 2021; Piñeiro-García and Semetey, 2023). This reaction can serve as a method for functionalizing GO alkenes (acting as electrophiles–Michael acceptors) and hydroxyls (acting as nucleophiles–Michael donors). In the first method, a nucleophile attacks GO’s active C=C bonds, which are the double bonds in the beta position of ketones or carboxylic acids. This functionalization can occur under conditions similar to nucleophilic substitution reactions via open epoxide rings, which require nucleophilic attack and employ basic catalysts. Hence, it is highly likely that the nucleophilic attack occurs on both the Michael acceptors and the epoxides (Figure 4A), although the epoxides are more abundant. Therefore, to favor the Michael reaction, the use of reduced graphene oxide (rGO; containing fewer epoxide groups) is recommended (Piñeiro-García et al., 2021) (Figure 4B). The second method, where GO acts as a Michael donor, will be discussed with other functionalization methods involving hydroxyls.
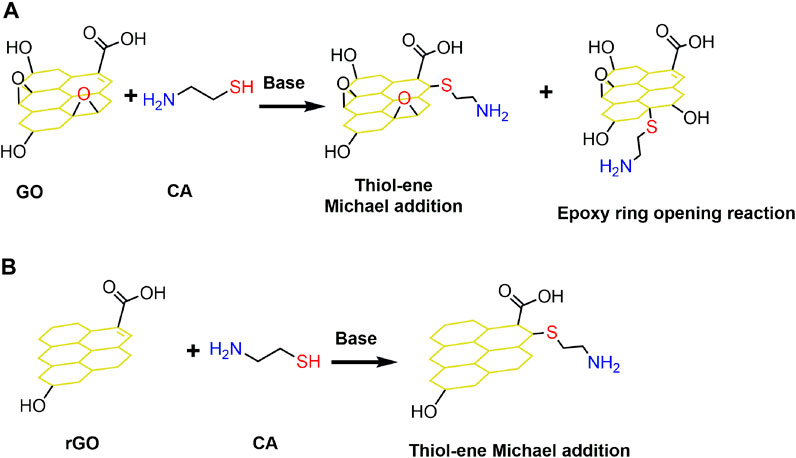
Figure 4. (A) Functionalization of GO with cysteamine – CA, using a base catalyst where two possible products are shown: Michael addition and epoxy ring opening reaction by SH. (B) Functionalization of rGO with CA by Michael addition. See Piñeiro-García et al. (2021).
Another common method for functionalizing C=C bonds is diazotization (Figure 3B). It involves a cycloaddition between, which employs aryl diazonium salts (ArN2+, Ar=aryl). In this method, electron transfer from the carbon substrate to the ion reduces diazonium ions to aryl diazonium radicals and enables their addition to the C=C bonds of GO and the formation of a C–C covalent bond between the carbon and the aryl group (Ossonon and Bélanger, 2017; Monteiro et al., 2020; Yu et al., 2020; Guo et al., 2022). This reaction is compatible with different groups (Guo et al., 2022) and is usually performed in organic solvents to avoid collateral reactions with water (Monteiro et al., 2020).
Finally, the Diels–Alder reaction is a significant method for functionalizing C=C bonds (Figures 3C). It involves a cycloaddition between a diene (an electron-rich alkene) and a dienophile (an electron-poor alkene). The conjugated double bonds of GO can act as both a diene and a dienophile and result in the saturation of conjugated sp2 hybridized carbon atoms. A carbonyl group adds an extra electron to the graphene π-system, while an epoxy group removes two π-electrons from neighboring sites in the sublattice (Donato et al., 2023). Therefore, the dienophilic nature of the GO surface prevails over its diene behavior due to the large amount of epoxides and hydroxyls (Tang et al., 2017). The main reactions that will be discussed below are illustrated in Figure 3.
The most widely explored functionalization methods have focused on the oxygen functional groups of GO, and we will didactically show the reactions separated by oxygenated functions, starting with hydroxyls, although some reactions are not selective and occur in different groups. Michael additions can ensue using hydroxyl groups, but unlike the process mentioned above, the GO hydroxyls in this case act as Michael donors, attacking other α,β-unsaturated carbonyl molecules. For example, Guo et al (2020b) added benzoquinone (a Michael acceptor) to GO hydroxyls using Michael addition. Hydroxyl groups can also be modified to produce ester (Yu et al., 2020) or carbodiimide (Zhang et al., 2011) (Figures 3D). These reactions offer a great opportunity to customize GO and the attachment of different chemical species, thereby enhancing its properties. For example, esterification with biocompatible polymers greatly improves the dispersibility of GO in physiological solutions (Monteiro et al., 2020). Another approach is to condense GO hydroxyl groups with organosilanes to produce silica-decorated graphene oxide (Figures 3E) (Ma et al., 2016; Serodre et al., 2019). Another functionalization method that initially leverages the reactivity of hydroxyl groups involves the reaction of N, N-dimethylacetamide dimethyl acetal (DMDA) with GO. In this case, the hydroxyl group condenses with DMDA to form a vinyl ether intermediate, which undergoes a Claisen sigmatropic rearrangement with the carbon surface, resulting in the formation of a new C–C bond (Figures 3F) (Sydlik and Swager, 2013).
The carboxyl functionalization of GO, which is the most applied method, requires an activation step followed by anchoring of the group of interest to form an ester or amide bond (Figures 3G). Reagents such as thionyl chloride (SOCl2), N,N-dicyclohexylcarbodiimide (DCC), and 1-ethyl-3-(3-dimethylaminopropyl)-carbodiimide (EDC) are commonly used to activate the carboxyl (Yu et al., 2020). These reagents react with the hydroxyl groups of the carboxylic acid of GO, resulting in an intermediate called o-acylurea, which is more susceptible to nucleophilic attack. However, in some reactions, a direct attack on o-acylurea is not favored, resulting in slow reactions. To overcome this issue, a step before the attack is performed by adding reagents that increase the stability of the intermediate, such as N-hydroxysuccinimide (NHS) reagent (Kasprzak et al., 2018). This methodology is used to add nucleophiles such as cysteamine (Orth et al., 2014; Santos et al., 2023), hydroxamic acid (Hostert et al., 2020), and ethylene diamine (Maslekar et al., 2020) but is not restricted to small nucleophiles since enzymes (Paz-Cedeno et al., 2021) and polymeric materials such as polyethylene glycol (Hao et al., 2020), polyethyleneimine (Oh et al., 2021), alginate (Golkar et al., 2023), and chitosan (Rana et al., 2011; Mirzaie et al., 2019) can also be attached. Table 2 provides a summary of some covalent functionalization on the surface of GOs, taking into consideration different methods and applications.
In addition to the research mentioned in Table 2, other studies have demonstrated the applications of covalently functionalized GO in fields such as desalination (Nair et al., 2012), healthcare products (Liu et al., 2008; Kumar et al., 2019; Shetti et al., 2019), oil–water separation (Feng et al., 2018), solar cells (Liu et al., 2008; Xue et al., 2015), energy storage (Cakici et al., 2017; Tian et al., 2021), and coatings (Ma et al., 2016). Some other studies have discussed the influence of the neighboring effect on functionalized materials, further emphasizing the importance of controlling selectivity in the diverse applications of functionalized GO (Santos et al., 2022; Santos et al., 2023).
Bifunctionalization or multifunctionalization of GO is also an interesting approach to producing advanced materials and is an underexplored subject. Many approaches exist, such as combining epoxy ring opening with Michael addition on hydroxyl groups (Guo et al., 2020b), amidation of carboxyl groups combined with epoxy ring opening (Santos et al., 2022), and epoxy ring opening combined with Williamson reaction on hydroxyl groups (Vacchi et al., 2020). These examples are illustrated in Figure 5.
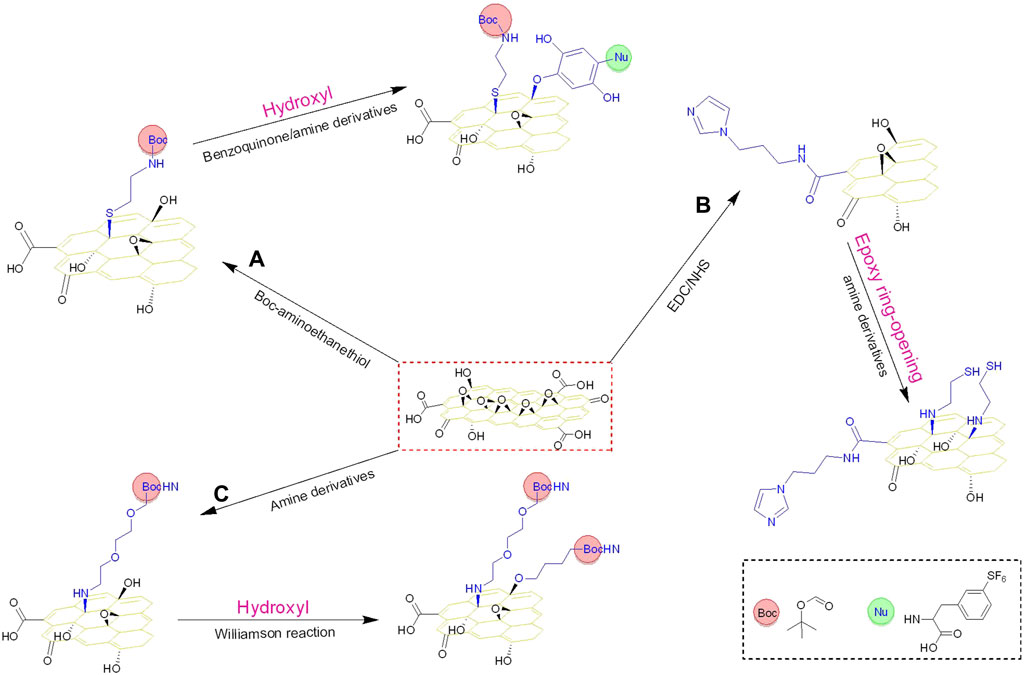
Figure 5. Examples of covalent bifunctionalization through different routes in GO. Path (A) epoxy-ring opening + Michael addition (See Guo et al., 2020b). Path (B) Carboxyl activation + epoxy-ring opening (See Santos et al., 2022). Path (C) epoxy-ring opening + Williamson reaction (See Vacchi et al., 2020).
Path a) is appropriate for introducing different functional groups, considering that benzoquinone is widely used as a coupling agent and can also react with azides, phosphines, and thiol groups. Conjugation takes place through a Michael addition between benzoquinone and the amine or hydroxyl groups, with this method being performed in mild conditions without any heating. Path b) was surprisingly studied for application in catalysis—modified GO with the lowest degree of functionalization had the best performance in catalytic performance. This was attributed to a dependence on the neighboring groups of GO due to steric effects or non-ideal positioning of the groups. Considering this, the application of bifunctionalized GO in catalysis still lacks a full understanding of its reactions and mechanisms. Path c) utilizes two orthogonally protected amino chains to allow selective cleavage of these protecting groups, allowing further derivatization (such as amidation) with molecules of interest under mild conditions and thus preserving the structure of GO. The Williamson reaction forms an ether bond between an organohalide and deprotonated alcohol via a substitution reaction. This functionalized GO has a high potential in nanomedicine for therapeutic and imaging applications.
Some other underexplored studies show free-radical covalent functionalization on the carbonaceous surface with two different groups, thereby increasing structure complexity (Pennetreau et al., 2014). Another study explored the multifunctionalization of GO with both -NH2 and -NH3+ groups, achieving bifunctional graphene oxide through a microwave-assisted method. In this research, GO was functionalized using N-isopropylidene–3-aminopropyl–triethoxysilane (ITES), with this nanocomposite being utilized in epoxide resin coatings and demonstrating exceptional hydrophobic behavior and dual corrosion protection for mild steel in a sodium chloride solution (Ramirez-Soria et al., 2021).
However, studies have reported that when the epoxy ring opening and carboxylation/amidation were combined, the partial reduction of GO led to a lower overall functionalization efficiency, thereby limiting the viability of this method for future applications. Therefore, in this epoxy ring functionalization, pre-treatment of GO with m-chloroperoxibenzoic acid served to increase the number of carbon atoms with this functional group on its surface (Collins et al., 2011b).
3 Functionalization via epoxide ring opening
A variety of functionalization routes toward epoxides have been reported, mostly involving a nucleophilic attack on one of the carbon atoms composing the epoxide ring (Khanra et al., 2012; Thomas et al., 2014; Alzate-Carvajal et al., 2018; Fallah-Mehrjardi et al., 2018; Kasprzak et al., 2018; Elsie et al., 2019; Jiang et al., 2019; Price et al., 2019; Javidparvar et al., 2020; Piñeiro-García et al., 2021; Guo et al., 2022; Kumar et al., 2022; Meng et al., 2022; Sainz-Urruela et al., 2022; Surabhi et al., 2022; Tan et al., 2022; Singh and Kar, 2023). The nucleophilic attack leads to the opening of the epoxide ring, thus binding the nucleophile to the GO (Guo et al., 2020a; Martin-Gullon et al., 2020; Kumar et al., 2022). The attached nucleophile can also be linked to other molecules or atoms, thus increasing the complexity (Kasprzak et al., 2018; Kumar et al., 2022). Some examples of nucleophiles that can be used in this reaction are amines (Li et al., 2020), thiols (Thomas et al., 2014), hydrazine (Gao et al., 2010), and sulfide ions (Kumar et al., 2022).
Applications greatly depend on the molecule to be added to the GO; this route proves to be interesting for the synthesis of catalysts. Santos et al. (2023) carried out a comparative study of the performance of catalysts functionalized with thiol or imidazole at different GO sites—epoxide and carboxylic acid. They thoroughly demonstrated that materials functionalized through epoxide groups were more effective in catalyzing organophosphate neutralization reactions than those functionalized through carboxylic acids. One of the main factors justifying the superior performance of catalysts functionalized via epoxide is the cooperative effect between the added nucleophile (thiol or imidazole) and neighboring groups, such as carboxylic acids present at the edges of graphene oxide. Surabhi et al. (2022) performed the functionalization with imidazole groups, followed by decoration with palladium nanoparticles. The material was used to catalyze the production of amides from aldehydes and amines. This study highlighted the importance of the imidazole groups that were functionalized via epoxide ring opening for the uniform distribution of the metal nanoparticles, avoiding their agglomeration.
In some studies, the application was not experimentally evaluated, even though researchers suggested some possibilities for future use of the material. For example, Guo et al. (2020b) and Vacchi et al. (2020) performed functionalization with biomolecules and proposed future use for multifunctional applications in therapy, biosensing, and bioimaging. Some functionalizations were also made for GO reduction (Khanra et al., 2012; Price et al., 2019; Javidparvar et al., 2020). Table 3 contains studies in which functionalization via epoxide was carried out, along with both tested and suggested applications.
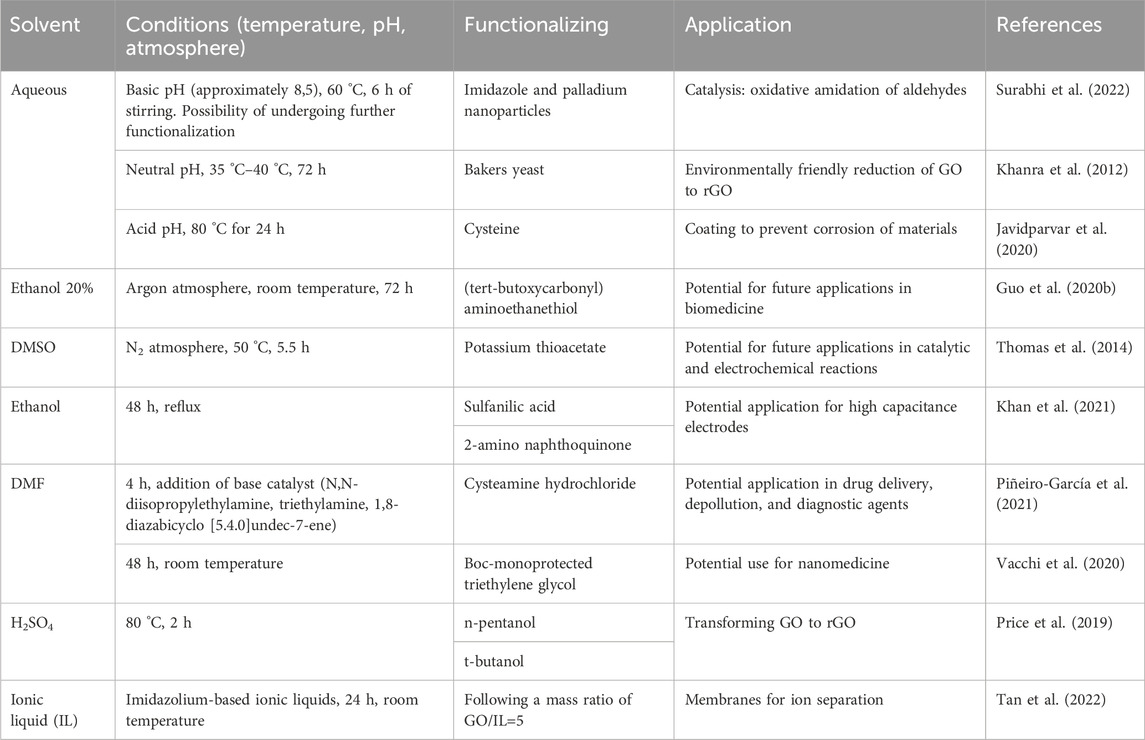
Table 3. Conditions and applications of some routes of functionalization of GO via the epoxide ring.
This section will cover the main approaches used in functionalizing the epoxide groups, discussing the influence of the medium, such as the solvent and pH.
3.1 Epoxide functionalization conditions
Some factors that can influence functionalization are the temperature, solvent, and pH of the reaction. Although the defects of the GO structure are a factor that can be considered, due to the positioning of the groups, there are not enough studies on how the defects can influence the functionalization outcome.
Functionalization via the epoxide ring is usually carried out at room temperature and at temperatures up to 90 °C (see conditions in Table 3). Higher temperatures can reduce the GO, so they are usually avoided. At 100 °C for 30 min, GO already loses a large portion of its oxygenated groups, leading to the production of rGO; at 200 °C, practically all GO is converted to rGO (Kumar et al., 2021).
In the majority of studies, the effects of temperature are not discussed, but we can highlight two studies in which the effects of temperature during functionalization via epoxide were evaluated. One evaluated the epoxide opening by amine at room temperature and at 90 °C to investigate the impact of temperature on the reduction process. The results showed that treatment at room temperature did not lead to a reduction while heating to 90 °C resulted in a partial reduction of GO (Ranishenka et al., 2021). Sun et al. (2018) conducted a study of reaction temperature in which GO was modified with p-phenylenediamine (PPD) in aqueous media for 24 h. There was no pH adjustment, and different samples were obtained by controlling the reaction temperature to 10, 30, 50, 70, and 90 °C. Based on the characterizations, the authors concluded that when the reaction temperature was 10–70 °C, GO was linked with PPD through non-covalent interactions such as ionic interactions (–COO- − H3+N–R) and hydrogen bonds (C–OH…H2N–X). When the reaction temperature reached 90 °C, GO was functionalized with PPD through covalent C–N bonds, which they attributed to the attack of PPD on the carboxyl and epoxy groups of GO. In this study, a higher temperature was necessary to form the covalent bond. However, the effects of temperature are expected to vary for each grafting group and for each type of mechanism. A change in the pH of the medium could also modify the formation of the covalent bond at lower temperatures.
According to Le et al. (2018), the atmosphere in which a reaction occurs can also influence it; reactions at high temperatures in the air lead to the reaction of the carbonaceous skeleton with oxygen, whereas, in non-oxidative atmospheres and at high temperatures, it leads to the loss of functional groups, maintaining the carbonaceous structure.
Functionalization via epoxides can be conducted in solvents such as water, dimethylformamide (DMF) (Piñeiro-García et al., 2021), tetrahydrofuran (THF) (Collins et al., 2011b; Ha et al., 2016), and dimethyl sulfoxide (DMSO) (Thomas et al., 2014). In choosing the solvent, it is important to consider some points, the first being the solubility of the GO in the chosen solvent. GO can be dispersed in water and even interact with polar solvents. This is determined by the quantity of oxygenated groups in the structure; GO can thus be partially soluble in high-polar solvents such as DMSO.
The dispersibility of GO in polar solvents is related to the dipole moment and the superficial tension of the solvent; solvents that have high dipole moments, like NMP, propanol, and water, can be used to achieve GO dispersion, changing the stability of the dispersion depending on the solvent used (Paredes et al., 2008; Sainz-Urruela et al., 2022). With the dispersion of GO, it is possible to functionalize the structure. In addition to temperature, atmosphere and solvent, the pH is another factors that can influence the functionalization outcome. In changing the pH, the mechanism for functionalization can change, and although they are not well-established, much of the discussion is usually based on classic epoxide ring-opening reactions (i.e., without the GO structure).
Functionalization with polymers such as polyaniline (PANI) and poly 3, 4-ethylenedioxythiophene (PEDOT) shows great results and can be made in aqueous and non-aqueous media. With further study, it is possible to relate the pH effect on polymers as well as functionalization, as this effect is not well established in functionalization with polymers (Kuilla et al., 2010). Some functionalization reactions and the necessary conditions are shown in Table 3.
In aqueous media, the main factor that can influence the reaction is the pH; different ranges create different conditions, changing the temperature at which the reaction can occur and limiting the reagents that can be used under determined conditions (Kasprzak et al., 2018; Price et al., 2019; Guo et al., 2022; Sainz-Urruela et al., 2022). The use of non-aqueous media can produce harsher conditions than with aqueous media, with higher temperatures and longer reactions; however, the use of different solvents means that functionalization might not happen in an aqueous medium. Figure 6 shows some functionalizations and the conditions of each reaction.
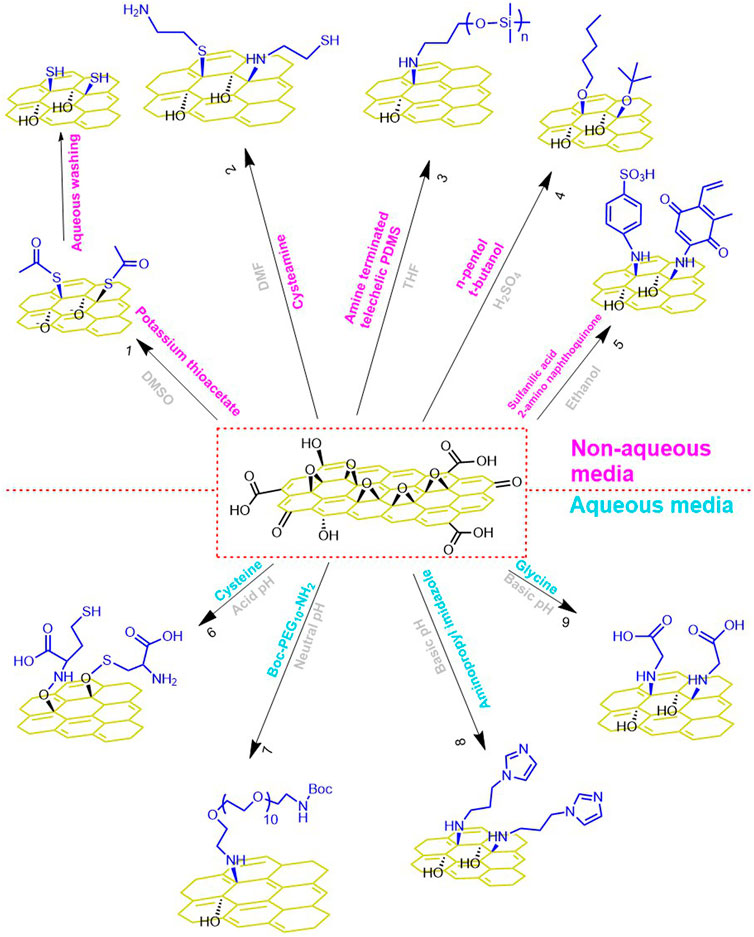
Figure 6. General schematics of some reported functionalization routes via epoxy-ring opening in aqueous and non-aqueous media.
3.2 pH effects
Functionalizing the epoxide group in water offers better environmental conditions and often does not need a coupling reagent for the attachment of new nucleophilic groups (Kasprzak et al., 2018). The functionalization reaction will occur with the epoxide ring opening, with pH being the main factor affecting the reaction (Thomas et al., 2014; Fallah-Mehrjardi et al., 2018; Guo et al., 2020a). The use of a basic solution is one of the main methods of opening the epoxide ring, following an SN2 mechanism, with a nucleophilic attack on the electrophilic carbon from the epoxide (SN2) (Figures 7–I) or the carbon double bond which is adjacent to the epoxy moiety (SN2’) (Figure 7-I’) (Thomas et al., 2014; Fallah-Mehrjardi et al., 2018). This modification of GO is possible due to the high nucleophilicity of the ligand, making it possible to perform an attack at 180° on the carbon in an SN2 reaction, breaking the C–O bond and generating a GO–Nu bond (Kumar et al., 2022) (Figures 7–II). If the attack occurs in the electrophilic carbon, the nucleophilic group has to make the interaction in the opposite face; otherwise, if the attack occurs in the double-bound carbon, the approach can be in the same face or the opposite, creating a hydroxyl group (Thomas et al., 2014; Fallah-Mehrjardi et al., 2018). With the opening of the epoxide ring, it is possible to functionalize with a nucleophilic group, with multiple reported cases of functionalization with basic solutions and the use of nucleophilic groups (Thomas et al., 2014; Fallah-Mehrjardi et al., 2018).
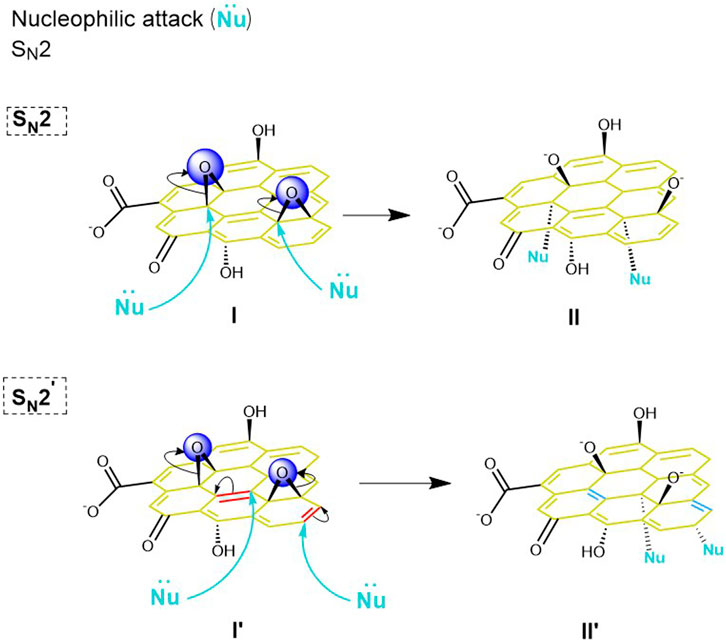
Figure 7. General mechanism of functionalization via the epoxide route in a basic medium. See Thomas et al., 2014; Fallah-Mehrjardi et al., 2018.
There is evidence that a high pH can cause the reduction of GO (Guo et al., 2022), and it is possible to observe a minimum effect on pH 8,5. However, with the increase in pH, the reduction of GO is intensified, leading the GO to have fewer oxygenated groups and more defects on its basal plan, affecting functionalization (Guo et al., 2020a; Guo et al., 2022). Research is still conflicting regarding the pH range in which the reduction will be harsh on the GO structure. Some studies indicate that the reduction of GO at pH levels as high as 13 is not sufficient to reduce its structure (Guo et al., 2020a), and others indicate that it is possible to observe low levels of reduction with basic treatment with sodium hydroxide because GO is unstable after treatment with strong bases (Guo et al., 2020a; Guo et al., 2022). The reduction of GO must be avoided in functionalization reactions since reduction makes GO lose most of its oxygenated groups, leaving fewer active sites for functionalization (Guo et al., 2020a). After alkaline treatment, GO becomes much more sensitive to temperature influence; at approximately 40 °C GO becomes unstable, leading to the rupture of its carbon backbone. If the epoxide ring opens at a higher temperature (70 °C), the reaction becomes irreversible. These conditions can cause the reduction of GO, creating defects and the eventual disintegration of the flakes (Guo et al., 2022).
Surabhi et al. (2022) performed the functionalization with 1-(3-aminopropyl) imidazole on the GO structure via an epoxide ring opening made with KOH in aqueous media; see Figure 6.8 for a general schematic. It was further functionalized with palladium nanoparticles (Pd NP) for nanocatalysis of oxidative amidation of aldehydes. In this synthesis, a harsher condition was used in comparison to other functionalizations on the epoxide ring, but these conditions were not enough to cause reduction and any kind of defects in the GO structure (Guo et al., 2022; Surabhi et al., 2022). However, at this temperature (60 °C) the reaction will be irreversible (Guo et al., 2022).
Guo et al. (2020a) studied different types of functionalizations O-(2-aminoethyl)-O0 -[2-(Boc-amino)ethyl]decaethylene glycol (BocNH-PEG10-NH2). In this study, the functionalization of the epoxide ring was made at a neutral pH (Figure 6.7). Other types of functionalization besides epoxide functionalization were compared: other functionalizations with carboxylic acids were made, making use of activating agents, and hydroxyl functionalization to increase the amount of carboxylic acid in the structure. The number of amino-PEG groups in the GO structure was quantified with the Kaiser test. The study showed that after further functionalization of the GO structure, part of the functionalized groups was partially removed from the structure. This was probably because the reduction of the GO structure, caused by the amount of NaOH used, created a highly alkaline solution that reduced GO. The reaction was repeated with a lower concentration of NaOH, achieving pH ranges of 9–13. It was observed that solutions with a higher pH had suffered a higher reduction. XPS analysis did not show the expected increase in the amount of carboxyl groups, and epoxy groups also suffered a reduction. Even though the results showed that GO had been reduced, this was not severe, so it was possible to make further functionalizations in the epoxy groups at a neutral pH for 3 days. It was possible for Guo et al. (2020a) to see the benefits of the epoxide ring opening without any further functionalization and how strong basic conditions affect GO structure.
Khanra et al. (2012) conducted a study to develop an eco-friendly and cost-effective method for functionalizing and reducing GO. The functionalization was achieved at neutral pH using baker’s yeast to utilize the enzyme nicotinamide adenine dinucleotide phosphate (NADPH) as a reducing agent, thereby facilitating the GO reduction. The functionalization was carried out under mild conditions, resulting in the expected GO reduction.
Acid pH can also be used to functionalize the epoxide ring, although the mechanism is not yet well established for the GO structure. In general, the mechanism in epoxides will be an SN1, with the attack occurring at the more substituted carbon, but research is necessary to establish this type of mechanism for GO (Fallah-Mehrjardi et al., 2018). Tanighuti et al. (2015) studied the influence of pH on the opening of GO epoxide rings and proposed that in an acidic environment, the reaction occurs through a mechanism involving the formation of carbocations and hydroxyl. The reaction of the hydroxyl group with the carbocation occurs preferentially, generating a highly reactive protonated epoxy group that can be cleaved by weak nucleophiles such as water, ultimately generating two hydroxyls. Furthermore, the delocalization of positive charges leads to lower reactivity of the protonated epoxy groups in the GO plane. As a result, much less epoxy ring opening reaction in GO occurs at a neutral or acidic pH.
Javidparvar et al. (2020) carried out functionalization with cysteine on the GO structure (Figure 6.6). This study used a sustainable nanocarrier with the capacity to absorb cerium ions to improve its anti-corrosion properties. To functionalize GO, a pH range of 2–3 was used (Javidparvar et al., 2020). Functionalization in acidic pH is not usual but there are some reports benefiting from this condition. In this range of pH, the reduction of the GO is avoided; however, temperatures as high as 80 °C as used in the study can cause a reduction.
Functionalization in aqueous media is possible in different ranges of pH but is easier with basic pH and is consequently a faster way of achieving functionalization in aqueous media (Khanra et al., 2012; Kasprzak et al., 2018; Price et al., 2019; Javidparvar et al., 2020; Piñeiro-García et al., 2021; Guo et al., 2022; Surabhi et al., 2022; Singh and Kar, 2023). Moreover, functionalization in neutral or acidic pH can occur at higher temperatures, and those conditions can also change depending on the stability of the functionalization and GO (Khanra et al., 2012; Guo et al., 2020a; Guo et al., 2022; Surabhi et al., 2022; Singh and Kar, 2023). The majority of studies do not explain the use of some pH ranges, temperatures, and mechanisms involving reactions, so it is not possible to draw general conclusions on which pH range to use at certain types of functionalization on the epoxide groups. However, it is possible to observe that the main influences on functionalization in aqueous media are pH, temperature, and time of reaction. Controlling these conditions allows new functionalization reactions in the epoxide ring that would normally not be possible without a basic or neutral medium.
3.3 Functionalization in a non-aqueous solvent
The functionalization reaction by epoxide ring opening can be performed in non-aqueous media if necessary. GO has different solubility and dispersion behavior depending on the solvent used. GO can be dispersed in some solvents like ethanol and dimethyl sulfoxide (DMSO), but the dispersion is not stable, precipitating in a matter of hours or days. Other solvents like tetrahydrofuran (THF), dimethylformamide (DMF), ethylene glycol, n-methyl-2-pyrrolidone (NMP), and water can form stable dispersions that do not precipitate (Paredes et al., 2008). Therefore, some factors that affect the planning of GO functionalization on non-aqueous media are the behavior of GO in the medium and the solubility of the functionalization in the medium. Other decisive factors in the choice of the solvent can be the use of catalysts (Ramírez-Jiménez et al., 2018; Piñeiro-García et al., 2021) or synergistic effects between the solvent and reagents. Using aprotic polar solvents such as DMF can facilitate Sn2 reactions, which include the epoxide ring-opening reaction because these solvents can solvate positively charged ions while not interacting as well with anions, therefore increasing their reactivity as nucleophiles and thus enhancing reaction rates (Clayden et al., 2012; Solomons et al., 2016). Therefore, when using anionic nucleophile reagents, the utilization of polar aprotic solvents can be considered. Epoxide ring opening functionalization has been reported in many solvents, like DMF (Shim et al., 2012; Vacchi et al., 2016; Ramírez-Jiménez et al., 2018; Vacchi et al., 2020; Piñeiro-García et al., 2021), DMSO (Thomas et al., 2014), ethanol (Lin et al., 2010; Guo et al., 2020b; Khan et al., 2021), sulfuric acid (Price et al., 2019), and THF (Collins et al., 2011b; Ha et al., 2016).
Polymer/GO composite synthesis is done in aqueous and non-aqueous media (Kuilla et al., 2010; Shim et al., 2012; Lowe, 2014; Gao, 2015; Ha et al., 2016). For elastomers produced with poly (dimethylsiloxane) (PDMS) and GO, THF was used because of its ability to solubilize PDMS. The objective of this study was to create a facile method for producing elastomers with polymers and GO in which the GO showed capabilities of crosslinking and filling the polymer to form a solid product with the added function of acting as a gas barrier. The authors used functional telechelic PDMS, functionalized at both ends with primary amines, which were dissolved in THF; GO was then added and dispersed by sonication in an ice bath. After drying in a Teflon dish for 3 days (2 days normal atmosphere, 1 day vacuum) at room temperature, a liquid solution of uncrosslinked PDMS/GO was obtained, which was then heated to 160 °C in a vacuum oven for 24 h to form the solid crosslinked PDMS/GO elastomer. This temperature is known to thermally reduce GO (Jung et al., 2008; Gao, 2015), which the authors did not acknowledge. The elastomer formed was mechanically resistant, as verified by rheological testing, and showed a reduction in single gas permeability compared to the neat PDMS, which was attributed to the gas adsorption in the GO dispersed phase. FTIR and XPS were used to characterize the polymer composite (Ha et al., 2016).
Functionalization via epoxide ring opening proved to be useful when multi-functionalizing because it is done mostly under moderate conditions without modifying other oxygenated groups of GO while also increasing hydroxyl moieties as a by-product of epoxide ring opening. The study by Khan et al. (2021) is an example, where many bifunctionalized GOs were synthesized to endow the composites with redox and acidic properties and to develop proton conductive electrodes and high capacitance electrodes. They utilized an epoxide ring opening followed by a Michael addition reaction with α, and β-unsaturated carbonyl compounds as a method of double functionalization with the synergistic approach mentioned previously. This study synthesized eleven different functionalized GOs, six of which were monofunctionalized to serve as a comparison. Khan et al. (2021) made use of three different solvents—water, ethanol, and toluene. For the sake of this review and the topic to be discussed, only the functionalization with ethanol as a solvent will be addressed since aqueous media was already explored in the previous sections and toluene is utilized as a solvent for the Michael addition reaction step.
The epoxide ring opening reaction utilized two compounds with a primary amine, which proved to be a good nucleophile in this regard—the first was sulfanilic acid for its proton conductivity function, and the second compound was 2-amino naphthoquinone for its redox-active function. The primary amine compounds were dissolved in ethanol and added to a GO aqueous dispersion. The mixture was sonicated and then refluxed for 48 h, followed by purification via centrifugation and washing. All the functionalized GO thus obtained were characterized via FTIR and XPS, confirming the nature of the GO composites. The double-functionalized samples showed an increment in properties (proton conductivity and capacitance) compared to monofunctionalized samples; the results confirm the efficiency of the double-functionalization method proposed and highlight the capabilities of modulating the physical and chemical properties of GO in mild conditions (Khan et al., 2021).
The epoxide ring opening reaction needs a nucleophilic attack to open the epoxide ring; a strong nucleophile is the thiolate anion that is obtained by removing the hydrogen proton from a thiol moiety. A good source of thiolate anion is potassium thioacetate, used by Thomas et al. (2014) to functionalize GO. The authors proposed a facile method for epoxide ring opening functionalization in DMSO. First, the GO was base washed to remove oxidative debris, leading to bwGO that was dispersed in DMSO via sonication. The dispersion was submitted to an N2 atmosphere, followed by the addition of potassium thioacetate. When the synthesis was completed, the material was washed multiple times. The aqueous washing was expected to result in the hydrolysis of the thioacetate, leaving surface-bound thiol groups. The material obtained was named GO–SH and was characterized by FTIR, XPS, EDX, solid-state RMN, TEM, and TGA, confirming the study’s propositions. Thiol is a hanging group on the surface plane of GO, which can lead to multiple new syntheses and applications. Thomas et al. (2014) anchored gold nanoparticles (AuNP) due to the strong interaction between sulfur and gold, as well as the ability to add a butane moiety to the thiol, demonstrating the diversity of possibilities of the material. Some of the applications suggested were in catalysis and electrochemistry due to the selectivity and reactivity of AuNP, as well as grafting polymers via thiol-ene “click” reactions (Lowe, 2014; Thomas et al., 2014; Piñeiro-García and Semetey, 2023).
Base catalysts, also known as non-nucleophilic bases, can be used to enhance the nucleophilicity of compounds by deprotonation of acidic hydrogen; one example was in the functionalization of GO with cysteamine by Piñeiro-García et al. (2021). They utilized three different base catalysts—N,N-diisopropylethylamine (DIPEA), triethylamine (NEt3), and 1,8-diazabicyclo [5.4.0]undec-7-ene (DBU) —to generate the thiolate anion on the cysteamine molecule and thus improve its nucleophilicity. Four materials were produced in THF as a solvent, three with the addition of the different base catalysts, and one for control. Samples characterizations were made with XPS and STEM, while a colorimetric titration was performed to quantify the primary amine moieties after GO functionalization. Cysteamine has two different nucleophilic groups, an amine (-NH2>) and a thiol (-SH). Due to the lower pKa of the thiol group than the amine group in this molecule, the thiolate anion was formed when base catalysts were added. This is a stronger nucleophile than the amine, which means that secondary amine moieties were not found on the functionalized GO samples, which is confirmed by the XPS’s N1s deconvolution analysis. Therefore, the addition of base catalysts to polar aprotic solvents can determine which of the nucleophiles in cysteamine will react, thus breeding a selective medium. This study was done to learn about the competing reactions of epoxide ring opening and thiol-ene Michael addition, concluding that both reactions happen simultaneously on the GO sample (Piñeiro-García et al., 2021).
Epoxide ring opening reactions may also occur in non-aqueous acidic conditions, such as when utilizing sulfuric acid as a solvent. GO was functionalized by Price et al. (2019) with n-pentanol and t-butanol, named GO-p and GO-tb, respectively, with the purpose of studying the wettability and dispersibility of the modified GO. The functionalized GO was prepared with sulfuric acid as a solvent, then extracted with hexane and washed with HCl and ethanol. The samples were characterized via TGA, FTIR, Raman spectroscopy, XPS, and DRX. The researchers concluded that the functionalization was successful and that the samples suffered a mild reduction due to the temperature and extremely acidic medium. Epoxide ring opening was not the only pathway of functionalization in this case—it is possible that a Fischer esterification occurred simultaneously. The addition of the alcohols and the reduction of the GO modified its hydrophilicity, turning the material hydrophobic and changing its dispersibility in apolar organic solvents like hexane. Since the reaction is not selective and the conditions are considerably harsh, this functionalization methodology deviates from those typically done via epoxide ring opening, offering a different approach for GO functionalization (Price et al., 2019).
In conclusion, the use of non-aqueous solvents offers a good alternative in cases where water is impractical. It also has synergistic effects when the nucleophile utilized is anionic, thus facilitating the Sn2 epoxide ring opening reaction (Clayden et al., 2012; Solomons et al., 2016).
4 Characterization techniques
Good characterization of GO and its modifications can lead to a better understanding of the chemoselectivity and regioselectivity of each route of GO modification, making correctly characterizing GO and its modifications a difficult and necessary challenge. Due to its various forms of storage and its highly complex structure, several interferences and limitations can occur; some of them are due to the overlap of data in the same analysis or to insufficient or hard-to-optimize instrumental equipment (Guo et al., 2022).
The characterization of functionalized GO aims to understand its morphology, possible uses, and the extent of the nanomaterial’s successful functionalization. To achieve this, techniques such as FTIR spectroscopy (Acik et al., 2011), X-ray photoelectron spectroscopy (XPS) (Yang et al., 2009), solid-state NMR spectroscopy (SSNMR) (Casabianca et al., 2010), electrochemical methods (Chua and Pumera, 2013), and thermogravimetric analysis (TGA) are utilized (Sharma et al., 2013; Rani et al., 2014). Particle size may also be characterized in solution using DLS and other laser scattering methods (Hong et al., 2012) or solid-state techniques such as TEM (Kumar et al., 2012) or AFM (Lu et al., 2010). Techniques can also characterize the surface area, such as BET methods (Brunauer et al., 1938), and the interlayer spacing in the powdered GO material can be determined via powder X-ray diffraction (Mu et al., 2013). The extent of defects in the lattice as well as the number of layers in the stacked structure can be measured using Raman spectroscopy (Kudin et al., 2008). Other advanced techniques have also been reported, such as integrated differential phase contrast scanning transmission electron microscopy (iDPC-STEM) (Wu et al., 2023), thermogravimetry-Fourier transform infrared spectroscopy-mass spectrometry (TG-IR-MS) (Liu et al., 2023), X-ray absorption spectroscopy (XAS), and X-ray absorption near edge structure (XANES) (Han et al., 2020).
Some characterization techniques are summarized in Table 4, and this section will cover the techniques usually used for GO modified via epoxy ring opening. It will consist of a brief explanation of the data that each technique provides and then an explanation, if necessary, to detect the functionalization of GO samples.
4.1 Fourier transform infrared (FTIR)
FTIR spectroscopy makes it possible to easily determine and identify functional groups grafted to GO using the vibrational modulus of the bonds formed between the grafted species and GO (Pintus et al., 2023). The experimental modes that are most used are the attenuated total reflection (ATR) and the use of KBr pellets. These are differentiated mainly in the sample preparation, which may be necessary as a function of how it was stored (powder, film, or dispersion) or responds to the experiment (Trikkaliotis et al., 2021; Pintus et al., 2023).
GO characterization using FTIR data has been widely reported (Khanra et al., 2012; Thomas et al., 2014; Zhang et al., 2015; Zhang et al., 2016; Javidparvar et al., 2020; Piñeiro-García et al., 2021; Trikkaliotis et al., 2021; Guo et al., 2022; Surabhi et al., 2022). The analysis of both the non-functionalized GO and the functionalized spectrums—for example, the immobilization of imidazolium-based ionic liquids on GO—was supported in Zheng Y. et al. (2021) and Tan et al. (2022) with the appearance of N–H and C–N stretching. The new modulus is thus identified and attributed to the GO–ligand bond, evidencing functionalization (Payami et al., 2020; Zheng Y. et al., 2021; Khan et al., 2021). It is also possible to assign the epoxide band in the pure GO sample and compare its intensity in the functionalized one; there is expected to be a decrease in this band after functionalization (Ha et al., 2016). One good example was reported by Zheng Y. et al., 2021, where the authors functionalized rGO with octylamine, decylamine, and dodecylamine via epoxide ring opening. Comparing all the functionalized spectra with those from the precursor, the decrease in epoxide band intensity is clearly evident (Ha et al., 2016; Zheng A. et al., 2021).
However, FTIR encounters limitations in determining bands in samples with low degrees of functionalization and in identifying a wide range of functional groups due to their similarity to GO’s background and fingerprint region (Pintus et al., 2023). Identifying and quantifying hydroxyls and epoxides in GO for confirmation of functionalization presents an even greater challenge. The assignment of epoxide bands in GO using FTIR often leads to conflicting outcomes.
Advancements in characterizing groups within basal planes using polarized ATR-IR have emerged as a solution. Polarization techniques offer a means of differentiating the contributions of various molecular orientations in the IR spectrum, yielding insights into the organization of molecules on the sample surface. This approach has been successfully applied to a variety of materials, including polymers (Hikima et al., 2019; Perret et al., 2021), molybdate composites (Davantès and Lefèvre, 2016; Botella and Lefèvre, 2022), proteins (Ausili et al., 2011), and graphene derivatives (Wang et al., 2016; Botella et al., 2022). Botella et al. (2022) demonstrated the potential of polarized ATR-IR spectroscopy in the characterization of graphene oxide (GO). By applying polarization angles of 0° and 90°, the researchers were able to analyze GO layers aligned along the x–y plane, identifying differences in both x–y (parallel components) and z (perpendicular component) orientations. Additional investigations within the 1,500–1,000 cm−1 range helped elucidate specific band assignments, revealing disparities in epoxide vibrations between the edge and basal plane groups. For instance, GO shows signals at 1,152 cm−1 for epoxides on the basal plane and 1,053 cm−1 for those at the edges (Botella et al., 2022).
4.2 Raman spectroscopy
Raman spectroscopy is often used since conjugated and double carbon–carbon bonds lead to high Raman intensities (Kudin et al., 2008). This method of characterization provides a simple structural characterization of graphene derivatives by the appearance of bands typical of these nanomaterials (Kuila et al., 2012). Bands typical of GO consist of the D and G bands, where the D band, present at 1,356 cm−1, is related to defects in its surface, and the G band, present at 1,583 cm−1, corresponds to plane vibrations of sp2 carbon (Kudin et al., 2008).
Utilizing the measurement of the band area in the obtained spectra can extract the ID/IG ratio values, which offer insights into the level of disorder present in the structure. This disorder can occur due to the interaction between oxygenated and functionalizing groups. Therefore, a greater ID/IG predicts a higher disorder in the functionalized GO structure, indicating more groups on its surface, although there is debate about this (Monteiro et al., 2020).
At around 2,650 cm−1, we have a band known as the 2D band, which is related to the structural organization of GO sheets, indicating the number of layers (Kuila et al., 2012). Considering this, it has been deduced that Raman spectroscopy could clearly distinguish between a single layer, a bilayer, and several layers, given that the number of layers alters the shape of the band (Monteiro et al., 2020). Other bands are observed at 2,930 and 3,170 cm−1, corresponding to the D+G and 2G bands, respectively, which are also characteristic of these materials (Kaniyoor and Ramaprabhu, 2012).
The information from Raman analysis can produce evidence of functionalization, but cannot prove it. Once the epoxy ring opens on a basic medium, it can lead to the formation of new defects or even to a reduction of the carbon structure (Guo et al., 2022). Moreover, it is important for reliable data to have many regions analyzed.
4.3 Microscopic techniques
Atomic force microscopy (AFM), transmission electron microscopy (TEM), and scanning electron microscopy (SEM) can be all used to characterize GO’s morphology and structure (Zhang et al., 2016; Chau et al., 2017; Alzate-Carvajal et al., 2018; Jiang et al., 2019; Kang et al., 2019; Meng et al., 2019; Payami et al., 2020; Shi et al., 2020; Zheng A. et al., 2021; Ranishenka et al., 2021), thickness (Alzate-Carvajal et al., 2018; Jiang et al., 2019; Meng et al., 2019; Meng et al., 2022), and porosity (Zheng A. et al., 2021). These microscopy techniques are commonly used together: AFM mainly generates the topographic and mechanical proprieties map, while SEM and TEM work mostly to visualize the GO sheets. A comparison of differences between non-functionalized and functionalized can elucidate some properties. Shi et al. (2020) used AFM and SEM to justify the increase in mechanical stability after the 1-octadecylamine and 1,12-diaminododecane were grafted, with the images showing that the functionalized sample became relatively more ordered than GO (Alzate-Carvajal et al., 2018; Meng et al., 2022). Meng et al. (2022) used these three techniques to measure GO and grafted GO: the thickness was determined with AFM measurements, while SEM and TEM were used to better understand the surface roughness and how the addition of a group affected the interaction between GO sheets and the interlayer spacing.
These characterizations can detect some evidence of chemical modification of GO with changes in morphology or roughness, although microscopy is the least reliable for confirming functionalization because the conditions of the reactions can cause changes in morphology and roughness even if the functionalization does not occur. It is important to analyze these data, knowing that it is a complementary analysis of GO functionalization.
Although microscopy techniques alone do not produce much information about functionalization, when combining AFM with spectroscopic techniques, it is possible to map the functional groups (Bartlam et al., 2018; Liu et al., 2018; Liu et al., 2019; Pérez et al., 2019). The outstanding combinations are AFM coupled with FTIR (AFM-IR) (Bartlam et al., 2018; Liu et al., 2018; Liu et al., 2019) and Raman (AFM-Raman) (Pérez et al., 2019). These techniques’ main disadvantages are high equipment costs and difficult sample preparation. However, their main advantage is that they can give valuable information on the nature of the functional groups and their distribution.
Using AFM-IR, Liu et al. (2019) mapped the functional groups on GO sheets, demonstrating that different groups can be found on the basal plane and borders. They also successively characterized GO groups in a way that is usually difficult, such as differentiating ketones from the carbonyl groups in the infrared spectra. Therefore, a spectrum of specific regions of a modified GO can be used to prove not just the modification but whether it occurred on the basal plane or border (Bartlam et al., 2018; Liu et al., 2018; Liu et al., 2019).
Experiments such as AFM-Raman yield important information about defects in structure and how the intensity of the D and G bands is distributed throughout the sample. This makes it possible to map the ID/IG distribution, as reported by Pérez et al. (2019), who mapped the D and ID/IG. With this map, they could understand which regions have more oxygenated groups and where it was modified most.
4.4 X-ray photoelectron spectroscopy (XPS)
XPS usually is used to obtain the chemical composition of nanomaterials and information about surface functionalization (Zhang et al., 2015; Chau et al., 2017; Zhu et al., 2018; Krishna and Philip, 2022; Ding et al., 2023). It can also be a tool for characterizing layer thickness, adsorbates, and even nanoparticle size (Krishna and Philip, 2022). Through XPS, it is possible to demonstrate the modification with different groups and calculate the ratio between the abundance of certain atoms in the sample (Chau et al., 2017; Zhu et al., 2018; Ding et al., 2023). Analyzing the changes between the non-functionalized and functionalized GO makes it easy to confirm the grafting of different ligands (Zhang et al., 2015; Ding et al., 2023).
Estimating the presence of hydroxyls and epoxides poses a challenge because of the subtle variations in binding energies within the X-ray photoelectron spectra (XPS) of C 1s and O 1s. Rabchinskii et al. (2022) employed a technique involving the analysis of XPS spectra alongside data from NMR and infrared (IR) spectroscopy. By utilizing density functional theory calculations, they successfully pinpointed and characterized distinct spectral components linked to epoxide and hydroxyl groups within the C 1s spectra.
The decrease of C 1s peaks associated with epoxy and hydroxyl groups, Surabhi et al. (2022) was assigned to the functionalization via epoxy ring opening in the reaction between GO and 1-(3-aminopropyl) imidazole. They also identified another peak appearing after the functionalization attributed to N 1s, corresponding to the imidazole nitrogen. This process is widely used; another example is Khanra et al. (2012), who functionalized it with baker’s yeast and reduced GO. Both events were visible in XPS, in which the decrease in intensity in the oxygenated groups is relatable to the reduction and new amine groups appearing from the functionalization with amine groups from baker’s yeast.
In the various techniques, XPS stands out for confirming functionalization. As it gives precise and quantitative information about the bonds, it is easy to identify the formation of new bonds and the decrease of the bond in the exact position where the new ligands were grafted. XPS also provides quantitative information about bonds, enabling the identification of new bond formations and reductions in bond intensity at the precise positions where new ligands were grafted (Meng et al., 2022). It proves to be a valuable tool for identifying new bond formations even at low degrees of functionalization (Krishna and Philip, 2022). Santos et al. (2022) confirmed bi-functionalization in carboxylic acid groups by distinguishing bands related to the cysteamine and imidazole derivatives anchored on graphene oxide and estimating the percentage of these groups. They also observed that signals corresponding to amides closely resembled the quantity of added groups.
4.5 Nuclear magnetic resonance (NMR)
Materials coming from graphite are often heterogeneous, noncrystalline, and insoluble, so obtaining structural data from them has been a difficult task. However, NMR can provide this crucial information about GO and modified GO, which is why solid-state NMR (SSNMR) has been employed (Casabianca et al., 2010; Vacchi et al., 2016).
Casabianca et al. (2010) studied the modeling of functionalized graphene from the analysis of GO. They showed that the combination of multidimensional experiments and chemical shift calculations is a great tool for constructing a model capable of reproducing the chemical structure. Vacchi et al. (2016) also used magic angle spinning (MAS) SSNMR, but this time aimed to elucidate the chemical reactivity of GO toward amine derivatives once it has a high reactivity due to the variety of oxygenated moieties, which can lead to many simultaneous reactions. They were able to determine that amines preferably react with the epoxy groups, not with carboxylic acids (Vacchi et al., 2016). NMR is a powerful technique for calculating the percentage of each functional group in the GO sheet; other techniques cannot be that exact (Vacchi et al., 2020).
SSNMR analysis enables the structural characterization of GO and derivatives (Casabianca et al., 2010; Vacchi et al., 2016) and the control of their derivatization by understanding the information of chemical reactivity (Vacchi et al., 2016) and estimating the constituent functional groups in different GO samples (Vacchi et al., 2020). Although this has great advantages, SSNMR does entail difficulties. It is hard to obtain a usable spectrum due to the higher number of interactions in the sample, the low resolution and intensity of signals, the need to strongly optimize the analyses, and the time for it—the experiment usually takes days to perform.
4.6 Thermogravimetric analysis
TGA analysis is usually performed at approximately 100 °C in an inert atmosphere (nitrogen or argonium). With this analysis, the curve shows the weight loss in each temperature range, making it possible to take the first derivative to obtain the differential thermal analysis (TGA-DTA), which helps elucidate the TGA curves (Alzate-Carvajal et al., 2018; Jiang et al., 2019). All GO samples must be analyzed in this way, making it possible to understand whether there was a bond between the GO and the target group. This is done by analyzing whether there is new weight loss at a certain temperature. Many studies use TGA analysis to confirm functionalization (Alzate-Carvajal et al., 2018; Elsie et al., 2019; Jiang et al., 2019) and to estimate an approximate degree of chemical modification (Alzate-Carvajal et al., 2018; Zhu et al., 2018; Elsie et al., 2019; Jiang et al., 2019; Ding et al., 2023).
Alzate-Carvajal et al. (2018) used such data to support the functionalization of GO with a group of various amines. The first and second weight loss (between 100 °C and approximately 230 °C) was attributed to the loss of oxygenated groups, and it reported a different weight loss, which was attributed to functionalization (Khanra et al., 2012; Javidparvar et al., 2020; Piñeiro-García et al., 2021). As shown above, Guo et al. (2020a) studied the functionalization of GO with O-(2-aminoethyl)-O0 -[2-(Boc-amino)ethyl]decaethylene glycol (BocNH-PEG10-NH2). They used TGA to demonstrate functionalization, showing a new weight loss of approximately 200 °C–400 °C after the modification of GO.
TGA characterization provides important information about samples, giving clues as to whether functionalization occurred. It is possible with a control sample to estimate the number of molecules grafted on GO (Alzate-Carvajal et al., 2018; Elsie et al., 2019; Jiang et al., 2019). The method cannot identify which group was grafted, so it is usually accompanied by methods such as FTIR or XPS.
4.7 X-ray diffraction (XRD)
The suspended GO sheets tend to undergo a re-stacking process after solvent removal or due to some kind of crosslinking due to functionalization. The resulting re-stacked solid can be analyzed by X-ray diffractometry. The crystallographic analysis can provide excellent information about the interlayer distance between sheets, how the ligands are arranged between sheets, and how the functionalization process affects crystallography (Xue et al., 2015; Ding et al., 2023). Knowing the initial basal reflections of GO enables an understanding of how the new ligands are intercalated in the basal planes by calculating the shifting of the peaks (Xue et al., 2015; Payami et al., 2020). With this information, the stitching of GO with functional groups, such as ethylenediamine, can be evidenced (Xue et al., 2015; Zhang et al., 2016; Ranishenka et al., 2021), making it possible to notice that with the increase in EDA ethylenediamine, there is an increase of the peak at 26,6° with a shifting of the 12,3° peak to lower values.
The XRD peak will appear even if the bonding between GO and the functionalizing group is not a covalent bond or if it is just adsorbed on the GO surface, although the interplanar distance may change, leading to a shifting of the GO natural peaks (Xue et al., 2015). XRD also cannot precisely identify what group was added via epoxy ring opening—other techniques are required.
4.8 Brunauer–Emmett–Teller (BET) isotherms
The absorption-desorption of N2 on GO sheets is used to estimate the surface area and pore diameter (Payami et al., 2020; Zheng A. et al., 2021). For this, the Brunauer–Emmett–Teller (BET) isotherms (Brunauer et al., 1938) are usually used for the surface area and Barrett–Joyner–Halenda (BJH) for pore size distribution (Zheng A. et al., 2021). Related to this is that the functionalization of GO can lead to an increase in the interlayer spacing (Zheng A. et al., 2021). The BET isotherms clarify the porous structures of GO and GO derivatives in their powder or colloidal forms.
Surabhi et al. (2022) used BET analysis to demonstrate that the material has a large superficial area, making it possible to incorporate Pd NP (Surabhi et al., 2022). As noted above, another use of the technique is to estimate the pore size and diameters of alkylamines in rGO hydrogels acting as spacers that lead to an increase in interlayer spacing (Zheng A. et al., 2021).
The change in surface area or pore size distribution indicates that there was probably a modification, but this is far from an accurate result. The synthesis conditions can lead to a rearrangement of the material or changing the carbon structure (Zheng A. et al., 2021), leading to modifications in the surface area.
5 Summary and future perspectives
We here present a critical analysis of the covalent functionalization of graphene oxide via epoxy groups. This is an under-explored method with great potential and stands out due to the large number of epoxide groups present in graphene oxide and the absence of coupling reagents. These generally make functionalization more expensive. Indeed, the functionalization process using epoxides can be conducted in different solvents. When selecting a solvent, it is crucial to consider the solubility of both the GO and the molecule being added. However, opting for aqueous media not only offers a more environmentally friendly approach but also ensures excellent GO dispersibility, so the use of aqueous media is recommended whenever possible. In addition, the success of the functionalization reaction depends on the pH of the medium, which has a significant impact on the nucleophilic attack. The preference for alkaline media in this reaction has been identified by different researchers. Although some discourage functionalization in alkaline media to avoid the reduction of graphene oxide, others highlight its advantages. For example, an alkaline wash prior to epoxide functionalization can eliminate oxidative debris and facilitate nucleophilic attack on graphene oxide sheets. Furthermore, the possibility of producing materials with properties that differ from those functionalized primarily at the edges is yet another reason why we believe this route to be worth further exploration. Two major challenges were highlighted in this review: the development of controllable methodologies and characterization to confirm the modified groups. The majority of studies focus only on confirming the occurrence of functionalization without worrying about distinguishing whether more than one group was modified. However, in studies seeking controlled functionalization, some techniques adopted include pH control, the use of coupling reagents, and the reduction of graphene oxide, which may favor one synthesis route over another.
Another challenge addressed in this review is characterization. Identifying and quantifying hydroxyls and epoxides in graphene oxide (GO) and confirming their functionalization is a known challenge for all routes, but its challenge is even greater in epoxide functionalization. A specific issue arises with the assignment of epoxide bands in GO using FTIR, as different studies often produce conflicting results. Recently, advances in the characterization of groups in basal planes have been made using polarized FTIR. Furthermore, we explained that estimating the presence of hydroxyls and epoxides is made difficult by the small differences in binding energies in the XPS of C 1s and O 1s. The recent progress in XPS and theoretical studies has allowed the differentiation and characterization of spectral components associated with epoxide and hydroxyl groups in C 1s spectra. These developments contribute to ongoing research for more accurate characterization, and successful implementation of these characterization methods is essential in the epoxide or hydroxyl functionalization of materials.
The improvement of functionalization methods and the development of characterization techniques are very important for fundamental studies and for the practical use of graphene oxide materials. Strategic functionalization can broaden and improve applications in fields including catalysis, energy, and the environment. Our aspiration is that this review plays a significant role in understanding the functionalization of graphene oxide, contributing to future exploration in this field.
Author contributions
MG: Conceptualization, Formal Analysis, Visualization, Writing–original draft, Writing–review and editing, Investigation, Methodology. VC: Conceptualization, Investigation, Methodology, Visualization, Writing–original draft, Writing–review and editing. AM: Investigation, Visualization, Writing–original draft, Writing–review and editing. BR: Investigation, Visualization, Writing–original draft, Writing–review and editing, Formal Analysis. GG: Formal Analysis, Investigation, Visualization, Writing–original draft, Writing–review and editing. AZ: Formal Analysis, Investigation, Writing–original draft, Writing–review and editing, Funding acquisition, Methodology, Resources, Validation. EO: Formal Analysis, Funding acquisition, Resources, Writing–original draft, Writing–review and editing, Conceptualization, Project administration, Supervision, Visualization.
Funding
The authors declare that financial support was received for the research, authorship, and/or publication of this article. This work was supported by UFPR, CNPq, CAPES, L’Oréal-UNESCO-ABC, PhosAgro/UNESCO/IUPAC, the National Institute of Science and Technology of Nanomaterials for Life (INCTNanovida), the National Institute of Science and Technology of Carbon Nanomaterials (INCTNanocarbon), and the Academic Cooperation Program in Public Security and Forensic Sciences-CAPES.
Conflict of interest
The authors declare that the research was conducted in the absence of any commercial or financial relationships that could be construed as a potential conflict of interest.
Publisher’s note
All claims expressed in this article are solely those of the authors and do not necessarily represent those of their affiliated organizations, or those of the publisher, the editors and the reviewers. Any product that may be evaluated in this article, or claim that may be made by its manufacturer, is not guaranteed or endorsed by the publisher.
References
Acik, M., Lee, G., Mattevi, C., Pirkle, A., Wallace, R. M., Chhowalla, M., et al. (2011). The role of oxygen during thermal reduction of graphene oxide studied by infrared absorption spectroscopy. J. Phys. Chem. C 115 (40), 19761–19781. doi:10.1021/jp2052618
Alves, L. S. M., Neves, M. F. F. D., Benatto, L., Ramos, M. K., Eising, M., de Oliveira, CKBQM, et al. (2023). Influence of nanostructuring sensors based on graphene oxide and PEDOT:PSS for methanol detection. IEEE Sensors J. 23 (3), 1845–1853. doi:10.1109/jsen.2022.3228954
Alzate-Carvajal, N., Acevedo-Guzmán, D. A., Meza-Laguna, V., Farías, M. H., Pérez-Rey, L. A., Abarca-Morales, E., et al. (2018). One-step nondestructive functionalization of graphene oxide paper with amines. RSC Adv. 8, 15253–15265. doi:10.1039/c8ra00986d
Ausili, A., Corbalán-García, S., Gómez-Fernández, J. C., and Marsh, D. (2011). Membrane docking of the C2 domain from protein kinase Cα as seen by polarized ATR-IR. The role of PIP2. Biochimica Biophysica Acta (BBA) - Biomembr. 1808 (3), 684–695. doi:10.1016/j.bbamem.2010.11.035
Bartlam, C., Morsch, S., Heard, K. W. J., Quayle, P., Yeates, S. G., and Vijayaraghavan, A. (2018). Nanoscale infrared identification and mapping of chemical functional groups on graphene. Carbon 139, 317–324. doi:10.1016/j.carbon.2018.06.061
Botella, R., and Lefèvre, G. (2022). A deep look into the diverse surface speciation of the mono-molybdate/lepidocrocite system by ATR-IR and polarized ATR-IR spectroscopy. Colloids Surfaces A Physicochem. Eng. Aspects 647, 129065. doi:10.1016/j.colsurfa.2022.129065
Botella, R., Piñeiro-García, A., Semetey, V., and Lefèvre, G. (2022). Polarized ATR-IR spectroscopy for the identification of material structure: the case of graphene oxide. Mater. Lett. 320, 132352. doi:10.1016/j.matlet.2022.132352
Brisebois, P. P., and Siaj, M. (2020). Harvesting graphene oxide – years 1859 to 2019: a review of its structure, synthesis, properties and exfoliation. J. Mater. Chem. C 8 (5), 1517–1547. doi:10.1039/c9tc03251g
Brunauer, S., Emmett, P. H., and Teller, E. (1938). Adsorption of gases in multimolecular layers. J. Am. Chem. Soc. 60 (2), 309–319. doi:10.1021/ja01269a023
Cakici, M., Kakarla, R. R., and Alonso-Marroquin, F. (2017). Advanced electrochemical energy storage supercapacitors based on the flexible carbon fiber fabric-coated with uniform coral-like MnO2 structured electrodes. Chem. Eng. J. 309, 151–158. doi:10.1016/j.cej.2016.10.012
Casabianca, L. B., Shaibat, M. A., Cai, W. W., Park, S., Piner, R., Ruoff, R. S., et al. (2010). NMR-based structural modeling of graphite oxide using multidimensional 13C solid-state NMR and ab initio chemical shift calculations. J. Am. Chem. Soc. 132 (16), 5672–5676. doi:10.1021/ja9030243
Chai, N., Zeng, J., Zhou, K., Xie, Y., Wang, H., Zhang, H., et al. (2013). Free-radical-promoted conversion of graphite oxide into chemically modified graphene. Chem. – A Eur. J. 19 (19), 5948–5954. doi:10.1002/chem.201203819
Chau, N. D. Q., Reina, G., Raya, J., Vacchi, I. A., Ménard-Moyon, C., Nishina, Y., et al. (2017). Elucidation of siRNA complexation efficiency by graphene oxide and reduced graphene oxide. Carbon 122, 643–652. doi:10.1016/j.carbon.2017.07.016
Chua, C. K., and Pumera, M. (2013). Covalent chemistry on graphene. Chem. Soc. Rev. 42 (8), 3222–3233. doi:10.1039/c2cs35474h
Collins, W. R., Lewandowski, W., Schmois, E., Walish, J., and Swager, T. M. (2011a). Claisen rearrangement of graphite oxide: a route to covalently functionalized graphenes. Angew. Chem. Int. Ed. 50 (38), 8848–8852. doi:10.1002/anie.201101371
Collins, W. R., Schmois, E., and Swager, T. M. (2011b). Graphene oxide as an electrophile for carbon nucleophiles. Chem. Commun. 47 (31), 8790–8792. doi:10.1039/c1cc12829a
Davantès, A., and Lefèvre, G. (2016). Molecular orientation of molybdate ions adsorbed on goethite nanoparticles revealed by polarized in situ ATR-IR spectroscopy. Surf. Sci. 653, 88–91. doi:10.1016/j.susc.2016.06.007
Dehsari, H. S., Shalamzari, E. K., Gavgani, J. N., Taromi, F. A., and Ghanbary, S. (2014). Efficient preparation of ultralarge graphene oxide using a PEDOT:PSS/GO composite layer as hole transport layer in polymer-based optoelectronic devices. RSC Adv. 4 (98), 55067–55076. doi:10.1039/c4ra09474c
Devi, N. R., Sasidharan, M., and Sundramoorthy, A. K. (2018). Gold nanoparticles-thiol-functionalized reduced graphene oxide coated electrochemical sensor system for selective detection of mercury ion. J. Electrochem Soc. 165 (8), B3046–B3053. doi:10.1149/2.0081808jes
Dimiev, A. M. (2016). “Mechanism of formation and chemical structure of graphene oxide,” in Graphene oxide (Chichester, UK: John Wiley & Sons, Ltd), 36–84.
Ding, L., Li, S., Yuan, X., Chang, W., Li, C., and Zheng, X. (2023). Preparation and tribological properties of Phenyl-Functionalized thermally reduced GrapheneOxide. Chem. Eng. Sci. 278, 118869. doi:10.1016/j.ces.2023.118869
Donato, K. Z., Tan, H. L., Marangoni, V. S., Martins, M. V. S., Ng, P. R., Costa, M. C. F., et al. (2023). Graphene oxide classification and standardization. Sci. Rep. 13 (1), 6064. doi:10.1038/s41598-023-33350-5
Elsie, S., Green, A., Rubavathi, D., Angamuthu, A., Gopal, B., and Bhagavathsingh, J. (2019). Tris-(2-aminoethyl)amine-Intercalated graphene oxide as an efficient 2D material for cerium-ion fluorescent sensor applications. ACS Omega 4, 22431–22437. doi:10.1021/acsomega.9b03089
Fallah-Mehrjardi, M., Kiasat, A. R., and Niknam, K. (2018). Nucleophilic ring-opening of epoxides: trends in β-substituted alcohols synthesis. J. Iran. Chem. Soc. 15, 2033–2081. doi:10.1007/s13738-018-1400-5
Feicht, P., and Eigler, S. (2018). Defects in graphene oxide as structural motifs. ChemNanoMat 4 (3), 244–252. doi:10.1002/cnma.201700357
Feng, Y., Wang, Z., Zhang, R., Lu, Y., Huang, Y., Shen, H., et al. (2018). Anti-fouling graphene oxide based nanocomposites membrane for oil-water emulsion separation. Water Sci. Technol. 77 (5), 1179–1185. doi:10.2166/wst.2017.634
Flores, K., Valdes, C., Ramirez, D., Eubanks, T. M., Lopez, J., Hernandez, C., et al. (2020). The effect of hybrid zinc oxide/graphene oxide (ZnO/GO) nano-catalysts on the photocatalytic degradation of simazine. Chemosphere 259, 127414. doi:10.1016/j.chemosphere.2020.127414
Gao, Q., Yan, J., Wan, L., Zhang, C., Wen, Z., Zhou, X., et al. (2022). High-efficiency graphene-oxide/silicon solar cells with an organic-passivated interface. Adv. Mater. Interfaces 9 (24), 2201221. doi:10.1002/admi.202201221
Gao, W. (2015). Graphene oxide: reduction recipes, spectroscopy, and applications. Graphene oxide: reduction recipes. Spectrosc. Appl., 1–147. doi:10.1007/978-3-319-15500-5
Gao, X., Jang, J., and Nagase, S. (2010). Hydrazine and thermal reduction of graphene oxide: reaction mechanisms, product structures, and reaction design. J. Phys. Chem. C 114 (2), 832–842. doi:10.1021/jp909284g
Ge, X., Li, H., Wu, L., Li, P., Mu, X., and Jiang, Y. (2017). Improved mechanical and barrier properties of starch film with reduced graphene oxide modified by <scp>SDBS</scp>. J. Appl. Polym. Sci. 134 (22). doi:10.1002/app.44910
Georgakilas, V., Demeslis, A., Ntararas, E., Kouloumpis, A., Dimos, K., Gournis, D., et al. (2015). Hydrophilic nanotube supported graphene–water dispersible carbon superstructure with excellent conductivity. Adv. Funct. Mater. 25 (10), 1481–1487. doi:10.1002/adfm.201403801
Georgakilas, V., Otyepka, M., Bourlinos, A. B., Chandra, V., Kim, N., Kemp, K. C., et al. (2012). Functionalization of graphene: covalent and non-covalent approaches, derivatives and applications. Chem. Rev. 112 (11), 6156–6214. doi:10.1021/cr3000412
Georgakilas, V., Tiwari, J. N., Kemp, K. C., Perman, J. A., Bourlinos, A. B., Kim, K. S., et al. (2016). Noncovalent functionalization of graphene and graphene oxide for energy materials, biosensing, catalytic, and biomedical applications. Chem. Rev. 116, 5464–5519. doi:10.1021/acs.chemrev.5b00620
Golkar, N., Sarikhani, Z., Aghaei, R., Heidari, R., Amini, A., and Gholami, A. (2023). An oral nanoformulation of insulin: development and characterization of human insulin loaded graphene oxide-sodium alginate-gold nanocomposite in an animal model. J. Drug Deliv. Sci. Technol. 82, 104309. doi:10.1016/j.jddst.2023.104309
Guo, S., Garaj, S., Bianco, A., and Ménard-Moyon, C. (2022). Controlling covalent chemistry on graphene oxide. Nat. Rev. Phys. 4, 247–262. doi:10.1038/s42254-022-00422-w
Guo, S., Nishina, Y., Bianco, A., Ménard-Moyon, C., and Ménard, C. (2020b). A flexible method for covalent double functionalization of graphene oxide. Angew. Chem. Int. Ed. 59 (4), 1542–1547. doi:10.1002/anie.201913461
Guo, S., Raya, J., Ji, D., Nishina, Y., Ménard-Moyon, C., and Bianco, A. (2020a). Is carboxylation an efficient method for graphene oxide functionalization? Nanoscale Adv. 2 (9), 4085–4092. doi:10.1039/d0na00561d
Ha, H., Park, J., Ha, K. R., Freeman, B. D., and Ellison, C. J. (2016). Synthesis and gas permeability of highly elastic poly(dimethylsiloxane)/graphene oxide composite elastomers using telechelic polymers. Polym. Guildf. 93, 53–60. doi:10.1016/j.polymer.2016.04.016
Han, G. F., Li, F., Zou, W., Karamad, M., Jeon, J. P., Kim, S. W., et al. (2020). Building and identifying highly active oxygenated groups in carbon materials for oxygen reduction to H2O2. Nat. Commun. 11 (1), 2209. doi:10.1038/s41467-020-15782-z
Hao, B., Lu, G., Zhang, S., Li, Y., Ding, A., and Huang, X. (2020). Gold nanoparticles standing on PEG/PAMAM/thiol-functionalized nanographene oxide as aqueous catalysts. Polym. Chem. 11 (25), 4094–4104. doi:10.1039/d0py00471e
He, M., Zhang, R., Zhang, K., Liu, Y., Su, Y., and Jiang, Z. (2019). Reduced graphene oxide aerogel membranes fabricated through hydrogen bond mediation for highly efficient oil/water separation. J. Mater. Chem. A 7 (18), 11468–11477. doi:10.1039/c9ta01700c
Hikima, Y., Morikawa, J., and Kazarian, S. G. (2019). Analysis of molecular orientation in polymeric spherulite using polarized micro attenuated total reflection Fourier transform infrared (ATR-FTIR) spectroscopic imaging. Anal. Chim. Acta 1065, 79–89. doi:10.1016/j.aca.2019.02.017
Hong, B. J., Compton, O. C., An, Z., Eryazici, I., and Nguyen, S. T. (2012). Successful stabilization of graphene oxide in electrolyte solutions: enhancement of biofunctionalization and cellular uptake. ACS Nano 6 (1), 63–73. doi:10.1021/nn202355p
Hostert, L., Blaskievicz, S. F., Fonsaca, J. E. S., Domingues, S. H., Zarbin, A. J. G., and Orth, E. S. (2017). Imidazole-derived graphene nanocatalysts for organophosphate destruction: powder and thin film heterogeneous reactions. J. Catal. 356, 75–84. doi:10.1016/j.jcat.2017.10.008
Hostert, L., Zarbin, A. J. G., and Orth, E. S. (2020). Hydroxamic acid-functionalized graphene thin films as nanocatalysts towards organophosphate degradation. J. Phys. Mater. 3 (3), 034003. doi:10.1088/2515-7639/ab854c
Javidparvar, A. A., Naderi, R., and Ramezanzadeh, B. (2020). L-cysteine reduced/functionalized graphene oxide application as a smart/control release nanocarrier of sustainable cerium ions for epoxy coating anti-corrosion properties improvement. J. Hazard. Mater. 389, 122135. doi:10.1016/j.jhazmat.2020.122135
Jayaraman, N., Palani, Y., Jonnalagadda, R. R., and Shanmugam, E. (2022). Covalently dual functionalized graphene oxide-based multiplex electrochemical sensor for Hg(II) and Cr(VI) detection. Sensors Actuators B Chem. 367, 132165. doi:10.1016/j.snb.2022.132165
Jiang, X., Lu, G., Huang, X., Li, Y., Cao, F., Chen, H., et al. (2019). Thermo-responsive graphene oxide/poly(ethyl ethylene phosphate) nanocomposite via ring opening polymerization. Nanomaterials 9, 207. doi:10.3390/nano9020207
Jung, I., Dikin, D. A., Piner, R. D., and Ruoff, R. S. (2008). Tunable electrical conductivity of individual graphene oxide sheets reduced at “low” temperatures. Nano Lett. 8 (12), 4283–4287. doi:10.1021/nl8019938
Kang, S., Qiao, S., Hu, Z., Yu, J., Wang, Y., and Zhu, J. (2019). Interfacial polymerized reduced graphene oxide covalently grafted polyaniline nanocomposites for high-performance electromagnetic wave absorber. J. Mater Sci. 54, 6410–6424. doi:10.1007/s10853-019-03347-5
Kaniyoor, A., and Ramaprabhu, S. (2012). A Raman spectroscopic investigation of graphite oxide derived graphene. AIP Adv. 2 (3). doi:10.1063/1.4756995
Kasprzak, A., Zuchowska, A., and Poplawska, M. (2018). Functionalization of graphene: does the organic chemistry matter? Beilstein J. Org. Chem. 14, 2018–2026. doi:10.3762/bjoc.14.177
Khan, A., Wang, J., Li, J., Wang, X., Chen, Z., Alsaedi, A., et al. (2017). The role of graphene oxide and graphene oxide-based nanomaterials in the removal of pharmaceuticals from aqueous media: a review. Environ. Sci. Pollut. Res. 24 (9), 7938–7958. doi:10.1007/s11356-017-8388-8
Khan, R., Miyagawa, K., Bianco, A., and Nishina, Y. (2021). Covalent double functionalization of graphene oxide for proton conductive and redox-active functions. Appl. Mater. Today 24, 101120. doi:10.1016/j.apmt.2021.101120
Khanra, P., Kuila, T., Kim, N. H., Bae, S. H., Yu, D. sheng, and Lee, J. H. (2012). Simultaneous bio-functionalization and reduction of graphene oxide by baker’s yeast. Chem. Eng. J. 183, 526–533. doi:10.1016/j.cej.2011.12.075
Komeily-Nia, Z., Qu, L. T., and Li, J. L. (2021). Progress in the understanding and applications of the intrinsic reactivity of graphene-based materials. Small Sci. 1 (2), 2000026. doi:10.1002/smsc.202000026
Kong, D., He, H., Song, Q., Wang, B., Lv, W., Yang, Q. H., et al. (2014). Rational design of MoS 2 @graphene nanocables: towards high performance electrode materials for lithium ion batteries. Energy Environ. Sci. 7 (10), 3320–3325. doi:10.1039/c4ee02211d
Krishna, D. N. G., and Philip, J. (2022). Review on surface-characterization applications of X-ray photoelectron spectroscopy (XPS): recent developments and challenges. Appl. Surf. Sci. Adv. 12, 100332. doi:10.1016/j.apsadv.2022.100332
Kudin, K. N., Ozbas, B., Schniepp, H. C., Prud’Homme, R. K., Aksay, I. A., and Car, R. (2008). Raman spectra of graphite oxide and functionalized graphene sheets. Nano Lett. 8 (1), 36–41. doi:10.1021/nl071822y
Kuila, T., Bose, S., Mishra, A. K., Khanra, P., Kim, N. H., and Lee, J. H. (2012). Chemical functionalization of graphene and its applications. Prog. Mater. Sci. 57 (7), 1061–1105. doi:10.1016/j.pmatsci.2012.03.002
Kuilla, T., Bhadra, S., Yao, D., Kim, N. H., Bose, S., and Lee, J. H. (2010). Recent advances in graphene based polymer composites. Prog. Polym. Sci. 35 (11), 1350–1375. doi:10.1016/j.progpolymsci.2010.07.005
Kumar, P., Bajpai, H., Gopinath, C. S., and Luwang, M. N. (2022). Sulfur functionalization via epoxide ring opening on a reduced graphene oxide surface to form metal (II) organo-bis-[1,2]-oxathiin. Inorg. Chem. 61 (1), 279–286. doi:10.1021/acs.inorgchem.1c02819
Kumar, P., Divya, N., and Ratan, J. K. (2021). Study on the physico-chemical properties of reduced graphene oxide with different degrees of reduction temperature. J. Iran. Chem. Soc. 18 (1), 201–211. doi:10.1007/s13738-020-02014-w
Kumar, R., Xu, C., and Scott, K. (2012). Graphite oxide/Nafion composite membranes for polymer electrolyte fuel cells. RSC Adv. 2 (23), 8777–8782. doi:10.1039/c2ra20225e
Kumar, S., Bukkitgar, S. D., Singh, S., Pratibha, M., Singh, V., Reddy, K. R., et al. (2019). Electrochemical sensors and biosensors based on graphene functionalized with metal oxide nanostructures for healthcare applications. ChemistrySelect 4 (18), 5322–5337. doi:10.1002/slct.201803871
Le, G. T. T., Manyam, J., Opaprakasit, P., Chanlek, N., Grisdanurak, N., and Sreearunothai, P. (2018). Divergent mechanisms for thermal reduction of graphene oxide and their highly different ion affinities. Diam. Relat. Mater. 89, 246–256. doi:10.1016/j.diamond.2018.09.006
Lerf, A., He, H., Forster, M., and Klinowski, J. (1998). Structure of graphite oxide revisited. J. Phys. Chem. B 102 (23), 4477–4482. doi:10.1021/jp9731821
Li, D., Wang, J., Yu, S., Ye, S., Zou, W., Zhang, H., et al. (2020). Highly regioselective ring-opening of epoxides with amines: a metal- and solvent-free protocol for the synthesis of β-amino alcohols. Chem. Commun. 56 (15), 2256–2259. doi:10.1039/c9cc09048g
Lima, L. F., Matos, C. F., Gonçalves, L. C., Salvatierra, R. V., Cava, C. E., Zarbin, A. J. G., et al. (2016). Water based, solution-processable, transparent and flexible graphene oxide composite as electrodes in organic solar cell application. J. Phys. D. Appl. Phys. 49 (10), 105106. doi:10.1088/0022-3727/49/10/105106
Lin, Z., Liu, Y., and Wong, C. P. (2010). Facile fabrication of superhydrophobic octadecylamine-functionalized graphite oxide film. Langmuir 26 (20), 16110–16114. doi:10.1021/la102619n
Liu, J., Chen, S., Liu, Y., and Zhao, B. (2022). Progress in preparation, characterization, surface functional modification of graphene oxide: a review. J. Saudi Chem. Soc. 26, 101560. doi:10.1016/j.jscs.2022.101560
Liu, J., Jiang, X., Xu, L., Wang, X., Hennink, W. E., and Zhuo, R. (2010). Novel reduction-responsive cross-linked polyethylenimine derivatives by click chemistry for nonviral gene delivery. Bioconjugate Chem. 21 (10), 1827–1835. doi:10.1021/bc100191r
Liu, J., Xue, Y., Gao, Y., Yu, D., Durstock, M., Dai, L., et al. (2012). Hole and electron extraction layers based on graphene oxide derivatives for high-performance bulk heterojunction solar cells. Adv. Mater. 24 (17), 2228–2233. doi:10.1002/adma.201104945
Liu, J., Yu, H., Wang, L., Vatsadze, S. Z., Chen, D., Wu, X., et al. (2023). Preparation of graphene oxides functionalized with ferrocene for the thermal decomposition of ammonium perchlorate. Mol. Catal. 550, 113572. doi:10.1016/j.mcat.2023.113572
Liu, Y., Jiang, X., Li, B., Zhang, X., Liu, T., Yan, X., et al. (2014). Halloysite nanotubes@reduced graphene oxide composite for removal of dyes from water and as supercapacitors. J. Mater. Chem. A 2 (12), 4264. doi:10.1039/c3ta14594h
Liu, Z., Nørgaard, K., Overgaard, M. H., Ceccato, M., Mackenzie, D. M. A., Stenger, N., et al. (2018). Direct observation of oxygen configuration on individual graphene oxide sheets. Carbon 127, 141–148. doi:10.1016/j.carbon.2017.10.100
Liu, Z., Rios-Carvajal, T., Ceccato, M., and Hassenkam, T. (2019). Nanoscale chemical mapping of oxygen functional groups on graphene oxide using atomic force microscopy-coupled infrared spectroscopy. J. Colloid Interface Sci. 556, 458–465. doi:10.1016/j.jcis.2019.08.089
Liu, Z., Robinson, J. T., Sun, X., and Dai, H. (2008). PEGylated nanographene oxide for delivery of water-insoluble cancer drugs. J. Am. Chem. Soc. 130 (33), 10876–10877. doi:10.1021/ja803688x
Lopez, A., and Liu, J. (2020). Covalent and noncovalent functionalization of graphene oxide with DNA for smart sensing. Adv. Intell. Syst. 2 (11). doi:10.1002/aisy.202000123
Lowe, A. B. (2014). Thiol–ene “click” reactions and recent applications in polymer and materials synthesis: a first update. Polym. Chem. 5 (17), 4820–4870. doi:10.1039/c4py00339j
Lu, G., Zhou, X., Li, H., Yin, Z., Li, B., Huang, L., et al. (2010). Nanolithography of single-layer graphene oxide films by atomic force microscopy. Langmuir 26 (9), 6164–6166. doi:10.1021/la101077t
Ma, Y., Di, H., Yu, Z., Liang, L., Lv, L., Pan, Y., et al. (2016). Fabrication of silica-decorated graphene oxide nanohybrids and the properties of composite epoxy coatings research. Appl. Surf. Sci. 360, 936–945. doi:10.1016/j.apsusc.2015.11.088
Martin-Gullon, I., Pérez, J. M., Domene, D., Salgado-Casanova, A. J. A., and Radovic, L. R. (2020). New insights into oxygen surface coverage and the resulting two-component structure of graphene oxide. Carbon 158, 406–417. doi:10.1016/j.carbon.2019.11.003
Maslekar, N., Mat Noor, R. A., Kuchel, R. P., Yao, Y., Zetterlund, P. B., and Agarwal, V. (2020). Synthesis of diamine functionalised graphene oxide and its application in the fabrication of electrically conducting reduced graphene oxide/polymer nanocomposite films. Nanoscale Adv. 2 (10), 4702–4712. doi:10.1039/d0na00534g
Meng, C., Chen, Q., Li, X., and Liu, H. (2019). Controlling covalent functionalization of graphene oxide membranes to improve enantioseparation performances. J. Membr. Sci. 582, 83–90. doi:10.1016/j.memsci.2019.03.087
Meng, Z., Lu, S., Zhang, D., Liu, Q., Chen, X., Liu, W., et al. (2022). Grafting macromolecular chains on the surface of graphene oxide through crosslinker for antistatic and thermally stable polyethylene terephthalate nanocomposites. RSC Adv. 12, 33329–33339. doi:10.1039/d2ra06725k
Mirzaie, A., Hasanzadeh, M., and Jouyban, A. (2019). Cross-linked chitosan/thiolated graphene quantum dots as a biocompatible polysaccharide towards aptamer immobilization. Int. J. Biol. Macromol. 123, 1091–1105. doi:10.1016/j.ijbiomac.2018.11.139
Monteiro, A. R., Neves, MGPMS, and Trindade, T. (2020). Functionalization of graphene oxide with porphyrins: synthetic routes and biological applications. ChemPlusChem 85, 1857–1880. doi:10.1002/cplu.202000455
Mu, S. J., Su, Y. C., Xiao, L. H., Liu, S. D., Hu, T., Tang, H. B., et al. (2013). X-ray difraction pattern of graphite oxide. Chin. Phys. Lett. 30 (9), 096101. doi:10.1088/0256-307x/30/9/096101
Nair, R. R., Wu, H. A., Jayaram, P. N., Grigorieva, I. V., and Geim, A. K. (2012). Unimpeded permeation of water through helium-leak–tight graphene-based membranes. Science 335 (6067), 442–444. doi:10.1126/science.1211694
Oh, J. S., Park, J. S., and Lee, E. J. (2021). Enhanced effect of polyethyleneimine-modified graphene oxide and simvastatin on osteogenic differentiation of murine bone marrow-derived mesenchymal stem cells. Biomedicines 9 (5), 501. doi:10.3390/biomedicines9050501
Orth, E. S., Fonsaca, J. E. S., Almeida, T. G., Domingues, S. H., Ferreira, J. G. L., and Zarbin, A. J. G. (2014). Functionalized graphene oxide as a nanocatalyst in dephosphorylation reactions: pursuing artificial enzymes. Chem. Commun. 50 (69), 9891–9894. doi:10.1039/c4cc03262d
Orth, E. S., Fonsaca, J. E. S., Domingues, S. H., Mehl, H., Oliveira, M. M., and Zarbin, A. J. G. (2013). Targeted thiolation of graphene oxide and its utilization as precursor for graphene/silver nanoparticles composites. Carbon 61, 543–550. doi:10.1016/j.carbon.2013.05.032
Ossonon, B. D., and Bélanger, D. (2017). Functionalization of graphene sheets by the diazonium chemistry during electrochemical exfoliation of graphite. Carbon 111, 83–93. doi:10.1016/j.carbon.2016.09.063
Pancharatna, P. D., Jhaa, G., and Balakrishnarajan, M. M. (2020). Nature of interactions between epoxides in graphene oxide. J. Phys. Chem. C 124 (2), 1695–1703. doi:10.1021/acs.jpcc.9b10262
Paredes, J. I., Villar-Rodil, S., Martínez-Alonso, A., and Tascón, J. M. D. (2008). Graphene oxide dispersions in organic solvents. Langmuir 24, 10560–10564. doi:10.1021/la801744a
Payami, E., Teimuri-Mofrad, R., Ahadzadeh, I., and Mohammadi, R. (2020). A novel composite electrode material derived from bisferrocenyl-functionalized GO and PANI for high performance supercapacitor. Electrochimica Acta 354, 136712. doi:10.1016/j.electacta.2020.136712
Paz-Cedeno, F. R., Carceller, J. M., Iborra, S., Donato, R. K., Godoy, A. P., Veloso de Paula, A., et al. (2021). Magnetic graphene oxide as a platform for the immobilization of cellulases and xylanases: ultrastructural characterization and assessment of lignocellulosic biomass hydrolysis. Renew. Energy 164, 491–501. doi:10.1016/j.renene.2020.09.059
Pennetreau, F., Riant, O., and Hermans, S. (2014). One-step double covalent functionalization of reduced graphene oxide with xanthates and peroxides. Chem. – A Eur. J. 20 (46), 15009–15012. doi:10.1002/chem.201405148
Pérez, L. A., Bajales, N., and Lacconi, G. I. (2019). Raman spectroscopy coupled with AFM scan head: a versatile combination for tailoring graphene oxide/reduced graphene oxide hybrid materials. Appl. Surf. Sci. 495, 143539. doi:10.1016/j.apsusc.2019.143539
Perret, E., Sharma, K., Tritsch, S., and Hufenus, R. (2021). Reversible mesophase in stress-annealed poly(3-hydroxybutyrate) fibers: a synchrotron x-ray and polarized ATR-FTIR study. Polym. Guildf. 231, 124141. doi:10.1016/j.polymer.2021.124141
Piñeiro-García, A., and Semetey, V. (2023). The “how” and “where” behind the functionalization of graphene oxide by thiol-ene “click” chemistry. Chem. Weinheim der Bergstrasse, Ger. 29, e202301604. doi:10.1002/chem.202301604
Piñeiro-García, A., Vega-Díaz, S. M., Tristán, F., Meneses-Rodríguez, D., Labrada-Delgado, G. J., and Semetey, V. (2021). New insights in the chemical functionalization of graphene oxide by thiol-ene Michael addition reaction. FlatChem 26, 100230. doi:10.1016/j.flatc.2021.100230
Piñeiro-García, A., Vega-Díaz, S. M., Tristan, F., Meneses-Rodríguez, D., and Semetey, V. (2022). Functionalization and soft photoreduction of graphene oxide triggered by the photoinitiator during thiol-ene radical addition. FlatChem 33, 100349. doi:10.1016/j.flatc.2022.100349
Pintus, A., Mantovani, S., Kovtun, A., Bertuzzi, G., Melucci, M., and Bandini, M. (2023). Recyclable GO-arginine hybrids for CO2 fixation into cyclic carbonates. Chem. Weinheim der Bergstrasse, Ger. 29, e202202440. doi:10.1002/chem.202202440
Price, E. K., Bansala, T., Achee, T. C., Sun, W., and Green, M. J. (2019). Tunable dispersibility and wettability of graphene oxide through one-pot functionalization and reduction. J. Colloid Interface Sci. 552, 771–780. doi:10.1016/j.jcis.2019.05.097
Rabchinskii, M. K., Shnitov, V. V., Brzhezinskaya, M., Baidakova, M. V., Stolyarova, D. Y., Ryzhkov, S. A., et al. (2022). Manifesting epoxide and hydroxyl groups in XPS spectra and valence band of graphene derivatives. Nanomaterials 13 (1), 23. doi:10.3390/nano13010023
Rahimpour, S., Luo, L., and Teimuri-Mofrad, R. (2022). Preparation of ferrocenyl-furan modified graphene oxide via Diels-Alder click reaction and using of its polypyrrole nanocomposites as supercapacitor electrode material. Electrochimica Acta 416, 140285. doi:10.1016/j.electacta.2022.140285
Ramírez-Jiménez, R., Franco, M., Rodrigo, E., Sainz, R., Ferritto, R., Lamsabhi, A. M., et al. (2018). Unexpected reactivity of graphene oxide with DBU and DMF. J. Mater. Chem. A 6, 12637–12646. doi:10.1039/c8ta03529f
Ramirez-Soria, E. H., León-Silva, U., Lara-Ceniceros, T. E., Bazán-Díaz, L., Advíncula, R. C., and Bonilla-Cruz, J. (2021). Graphene oxide bifunctionalized with NH2/NH3+ and their outstanding-performance against corrosion. Appl. Surf. Sci. 561, 150048. doi:10.1016/j.apsusc.2021.150048
Rana, V. K., Choi, M., Kong, J., Kim, G. Y., Kim, M. J., Kim, S., et al. (2011). Synthesis and drug-delivery behavior of chitosan-functionalized graphene oxide hybrid nanosheets. Macromol. Mater. Eng. 296 (2), 131–140. doi:10.1002/mame.201000307
Rani, S., Kumar, M., Kumar, R., Kumar, D., Sharma, S., and Singh, G. (2014). Characterization and dispersibility of improved thermally stable amide functionalized graphene oxide. Mater. Res. Bull. 60, 143–149. doi:10.1016/j.materresbull.2014.07.019
Ranishenka, B., Ulashchik, E., Tatulchenkov, M., Sharko, O., Panarin, A., Dremova, N., et al. (2021). Graphene oxide functionalization via epoxide ring opening in bioconjugation compatible conditions. FlatChem 27, 100235. doi:10.1016/j.flatc.2021.100235
Rourke, J. P., Pandey, P. A., Moore, J. J., Bates, M., Kinloch, I. A., Young, R. J., et al. (2011). The real graphene oxide revealed: stripping the oxidative debris from the graphene-like sheets. Angew. Chem. Int. Ed. 50 (14), 3173–3177. doi:10.1002/anie.201007520
Sainz-Urruela, C., Vera-López, S., Paz San Andrés, M., and Díez-Pascual, A. M. (2022). Surface functionalization of graphene oxide with tannic acid: covalent vs non-covalent approaches. J. Mol. Liq. 357, 119104. doi:10.1016/j.molliq.2022.119104
Santos, Y. H., Martinez, A. H. G., Veiga, A. G., Rocco, M. L. M., Zarbin, A. J. G., and Orth, E. S. (2022). Neighboring effects on the selective bifunctionalization of graphene oxide for nanocatalytic organophosphate neutralization. ACS Appl. Nano Mater. 5, 6001–6012. doi:10.1021/acsanm.2c00832
Santos, Y. H., Martinez, A. H. G., Veiga, A. G., Rocco, M. L. M., Zarbin, A. J. G., and Orth, E. S. (2023). Site-selective mono- and bifunctionalization of graphene oxide: screening nanocatalysts for organophosphate degradation. ChemCatChem 16. doi:10.1002/cctc.202301440
Serodre, T., Oliveira, N., Miquita, D., Ferreira, M., Santos, A., Resende, V., et al. (2019). Surface silanization of graphene oxide under mild reaction conditions. J. Braz Chem. Soc. doi:10.21577/0103-5053.20190167
Shahdost-fard, F., and Roushani, M. (2017). Designing an ultra-sensitive aptasensor based on an AgNPs/thiol-GQD nanocomposite for TNT detection at femtomolar levels using the electrochemical oxidation of Rutin as a redox probe. Biosens. Bioelectron. 87, 724–731. doi:10.1016/j.bios.2016.09.048
Sharma, P., Tuteja, S. K., Bhalla, V., Shekhawat, G., Dravid, V. P., Suri, C. R., et al. (2013). Bio-functionalized graphene–graphene oxide nanocomposite based electrochemical immunosensing. Biosens. Bioelectron. 39 (1), 99–105. doi:10.1016/j.bios.2012.06.061
Shetti, N. P., Malode, S. J., Nayak, D. S., Bagihalli, G. B., Reddy, K. R., Ravindranadh, K., et al. (2019). A novel biosensor based on graphene oxide-nanoclay hybrid electrode for the detection of Theophylline for healthcare applications. Microchem. J. 149, 103985. doi:10.1016/j.microc.2019.103985
Shi, L., Wang, Z., Yang, G., Yang, H., and Zhao, F. (2020). A novel electrochemical immunosensor for aflatoxin B1 based on Au nanoparticles-poly 4-aminobenzoic acid supported graphene. Appl. Surf. Sci. 527, 146934. doi:10.1016/j.apsusc.2020.146934
Shim, S. H., Kim, K. T., Lee, J. U., and Jo, W. H. (2012). Facile method to functionalize graphene oxide and its application to poly(ethylene terephthalate)/graphene composite. ACS Appl. Mater Interfaces 4 (8), 4184–4191. doi:10.1021/am300906z
Silvestri, S., and Gonçalves, M. G. (2022). “Carbon composites and catalysts for decomposition of organic pollutants,” in Carbon composite catalysts. Editors M. Jawaid, and A. Khan (Springer Nature), 337–369.
Singh, R., and Kar, R. K. (2023). Dynamic properties of graphene oxide functionalized with l-cysteine. J. Phys. Chem. C 127, 17438–17453. doi:10.1021/acs.jpcc.3c04469
Sun, H. J., Liu, B., Peng, T. J., and Zhao, X. L. (2018). Effect of reaction temperature on structure, appearance and bonding type of functionalized graphene oxide modified P-phenylene diamine. Materials. 11 (4), 647. doi:10.3390/ma11040647
Surabhi, S. D., Sah, D., Shabir, J., Gupta, P., and Mozumdar, S. (2022). Imidazole-functionalized porous graphene oxide nanosheets loaded with palladium nanoparticles for the oxidative amidation of aldehydes. ACS Appl. Nano Mater 5, 5776–5792. doi:10.1021/acsanm.2c00859
Sydlik, S. A., and Swager, T. M. (2013). Functional graphenic materials via a Johnson− Claisen rearrangement. Adv. Funct. Mater. 23 (15), 1873–1882. doi:10.1002/adfm.201201954
Tan, S., Zhang, D., Nguyen, M. T., Shutthanandan, V., Varga, T., Rousseau, R., et al. (2022). Tuning the charge and hydrophobicity of graphene oxide membranes by functionalization with ionic liquids at epoxide sites. ACS Appl. Mater Interfaces 14 (16), 19031–19042. doi:10.1021/acsami.2c02366
Tang, S., Wu, W., Liu, L., Cao, Z., Wei, X., and Chen, Z. (2017). Diels-Alder reactions of graphene oxides: greatly enhanced chemical reactivity by oxygen-containing groups. Phys. Chem. Chem. Phys. 19 (18), 11142–11151. doi:10.1039/c7cp01086a
Taniguchi, T., Kurihara, S., Tateishi, H., Hatakeyama, K., Koinuma, M., Yokoi, H., et al. (2015). PH-driven, reversible epoxy ring opening/closing in graphene oxide. Carbon 84 (1), 560–566. doi:10.1016/j.carbon.2014.12.054
Thomas, H. R., Day, S. P., Woodruff, W. E., Vallés, C., Young, R. J., Kinloch, I. A., et al. (2013). Deoxygenation of graphene oxide: reduction or cleaning? Chem. Mater. 25 (18), 3580–3588. doi:10.1021/cm401922e
Thomas, H. R., Marsden, A. J., Walker, M., Wilson, N. R., and Rourke, J. P. (2014). Sulfur-functionalized graphene oxide by epoxide ring-opening. Angew. Chem. - Int. Ed. 53 (29), 7613–7618. doi:10.1002/anie.201404002
Tian, Y., Yu, Z., Cao, L., Zhang, X. L., Sun, C., and Wang, D. W. (2021). Graphene oxide: an emerging electromaterial for energy storage and conversion. J. Energy Chem. 55, 323–344. doi:10.1016/j.jechem.2020.07.006
Trikkaliotis, D. G., Christoforidis, A. K., Mitropoulos, A. C., and Kyzas, G. Z. (2021). Graphene oxide synthesis, properties and characterization techniques: a comprehensive review. ChemEngineering 5, 64. doi:10.3390/chemengineering5030064
Vacchi, I. A., Guo, S., Raya, J., Bianco, A., and Ménard-Moyon, C. (2020). Strategies for the controlled covalent double functionalization of graphene oxide. Chem. – A Eur. J. 26 (29), 6591–6598. doi:10.1002/chem.201905785
Vacchi, I. A., Spinato, C., Raya, J., Bianco, A., and Ménard-Moyon, C. (2016). Chemical reactivity of graphene oxide towards amines elucidated by solid-state NMR. Nanoscale 8 (28), 13714–13721. doi:10.1039/c6nr03846h
Wang, H., Bi, S. G., Ye, Y. S., Xue, Y., Xie, X. L., and Mai, Y. W. (2015). An effective non-covalent grafting approach to functionalize individually dispersed reduced graphene oxide sheets with high grafting density, solubility and electrical conductivity. Nanoscale 7 (8), 3548–3557. doi:10.1039/c4nr06710j
Wang, J. T. W., Ball, J. M., Barea, E. M., Abate, A., Alexander-Webber, J. A., Huang, J., et al. (2014). Low-temperature processed electron collection layers of graphene/TiO 2 nanocomposites in thin film perovskite solar cells. Nano Lett. 14 (2), 724–730. doi:10.1021/nl403997a
Wang, X., Wang, W., Liu, Y., Ren, M., Xiao, H., and Liu, X. (2016). Characterization of conformation and locations of C–F bonds in graphene derivative by polarized ATR-FTIR. Anal. Chem. 88 (7), 3926–3934. doi:10.1021/acs.analchem.6b00115
Wang, Z., Hu, G., Liu, J., Liu, W., Zhang, H., and Wang, B. (2015). Coordinated assembly of a new 3D mesoporous Fe 3 O 4 @Cu 2 O–graphene oxide framework as a highly efficient and reusable catalyst for the synthesis of quinoxalines. Chem. Commun. 51 (24), 5069–5072. doi:10.1039/c5cc00250h
Wu, Q., Zou, H., Mao, X., He, J., Shi, Y., Chen, S., et al. (2023). Unveiling the dynamic active site of defective carbon-based electrocatalysts for hydrogen peroxide production. Nat. Commun. 14 (1), 6275. doi:10.1038/s41467-023-41947-7
Xie, Z., Sun, B., Lin, H., Fu, M., Zhang, Y., Ma, Z., et al. (2023). Bifunctional reduced graphene oxide/polyelectrolyte/NiFe layered double hydroxide composites for efficient catalyzed dephosphorylation and 4-nitrophenol reduction. J. Water Process Eng. 1, 53. doi:10.1016/j.jwpe.2023.103624
Xue, B., Zhu, J., Liu, N., and Li, Y. (2015). Facile functionalization of graphene oxide with ethylenediamine as a solid base catalyst for Knoevenagel condensation reaction. Catal. Commun. 64, 105–109. doi:10.1016/j.catcom.2015.02.003
Yang, D., Velamakanni, A., Bozoklu, G., Park, S., Stoller, M., Piner, R. D., et al. (2009). Chemical analysis of graphene oxide films after heat and chemical treatments by X-ray photoelectron and Micro-Raman spectroscopy. Carbon 47 (1), 145–152. doi:10.1016/j.carbon.2008.09.045
Yang, S., Cao, C., Huang, P., Peng, L., Sun, Y., Wei, F., et al. (2015). Sandwich-like porous TiO 2/reduced graphene oxide (rGO) for high-performance lithium-ion batteries. J. Mater. Chem. A 3 (16), 8701–8705. doi:10.1039/c5ta01744k
Yu, W., Sisi, L., Haiyan, Y., and Jie, L. (2020). Progress in the functional modification of graphene/graphene oxide: a review. RSC Adv. 10, 15328–15345. doi:10.1039/d0ra01068e
Zhang, B., Chen, Y., Xu, L., Zeng, L., He, Y., Kang, E. T., et al. (2011). Growing poly(N -vinylcarbazole) from the surface of graphene oxide via RAFT polymerization. J. Polym. Sci. A Polym. Chem. 49 (9), 2043–2050. doi:10.1002/pola.24633
Zhang, J., Gao, J., Song, Q., Guo, Z., Chen, A., Chen, G., et al. (2016). N-Substituted carboxyl polyaniline covalent grafting reduced graphene oxide nanocomposites and its application in supercapacitor. Electrochimica Acta 199, 70–79. doi:10.1016/j.electacta.2016.03.003
Zhang, Z. B., Wu, J. J., Su, Y., Zhou, J., Gao, Y., Yu, H. Y., et al. (2015). Layer-by-layer assembly of graphene oxide on polypropylene macroporous membranes via click chemistry to improve antibacterial and antifouling performance. Appl. Surf. Sci. 332, 300–307. doi:10.1016/j.apsusc.2015.01.193
Zheng, A. L. T., Boonyuen, S., Li, G. Y., Ngee, L. H., and Andou, Y. (2021). Design of reduced graphene hydrogel with alkylamine surface functionalization through immersion/agitation method and its adsorption mechanism. J. Mol. Struct. 1245, 131008. doi:10.1016/j.molstruc.2021.131008
Zheng, Y., Li, S., Han, D., Kong, L., Wang, J., Zhao, M., et al. (2021). Eco-friendly preparation of epoxy-rich graphene oxide for wound healing. ACS Biomater. Sci. Eng. 7 (2), 752–763. doi:10.1021/acsbiomaterials.0c01598
Keywords: functionalized graphene, epoxy groups, covalent derivatization, epoxide opening, nucleophilic reactions
Citation: Gonçalves MG, Costa VO, Martinez AHG, Régnier BM, Gomes GCB, Zarbin AJG and Orth ES (2024) Functionalization of graphene oxide via epoxide groups: a comprehensive review of synthetic routes and challenges. Front. Carbon 3:1393077. doi: 10.3389/frcrb.2024.1393077
Received: 28 February 2024; Accepted: 23 May 2024;
Published: 25 July 2024.
Edited by:
Derek Hao, RMIT University, AustraliaReviewed by:
Peng Li, RMIT University, AustraliaZijun Yong, RMIT University, Australia
Qilong Wu, University of Wollongong, Australia
Copyright © 2024 Gonçalves, Costa, Martinez, Régnier, Gomes, Zarbin and Orth. This is an open-access article distributed under the terms of the Creative Commons Attribution License (CC BY). The use, distribution or reproduction in other forums is permitted, provided the original author(s) and the copyright owner(s) are credited and that the original publication in this journal is cited, in accordance with accepted academic practice. No use, distribution or reproduction is permitted which does not comply with these terms.
*Correspondence: Elisa S. Orth, ZWxpc2FvcnRoQHVmcHIuYnI=