- 1Department of Theoretical and Applied Sciences, University of Insubria, Varese, Italy
- 2Department of Civil Engineering and Architecture, University of Pavia, Pavia, Italy
- 3Department of Civil, Environmental, Architectural Engineering and Mathematics, University of Brescia, Brescia, Italy
- 4Department of Building Engineering and Energetics, Institute of Technology, MATE, Gödöllő, Hungary
- 5Interdepartmental Centre for Water Research, University of Pavia, Pavia, Italy
Coronavirus disease (COVID-19) is caused by severe acute respiratory syndrome coronavirus 2 (SARS-CoV-2). In indoor environments, a structured strategy is needed to reduce the risk of infection. In addition to maintaining proper ventilation and wearing face masks, the development of effective technologies for limiting the transmission of SARS-CoV-2 through infectious respiratory particles (IRPs) has been studied. UV-C devices have already proved effective against other types of microorganisms and have also been investigated for their potential in inactivating SARS-CoV-2. This work aims to review and discuss these findings while also presenting recommendations for future research. Based on the available data, UV-C proved to be effective in the inactivation of airborne SARS-CoV-2 or its surrogates. The main gaps in this research have also been highlighted, and some outlooks for future studies have been suggested. In some studies, the use of surrogates with higher susceptibility to UV-C than airborne SARS-CoV-2 or the consideration of total bacterial counts alone may have led to misleading results. The question “how much variables affect the inactivation rate of airborne SARS-CoV-2 by UV-C in indoor environments?” is still unsolved, considering that the number of studies on the inactivation of this virus in real indoor environments is quite limited. The outcomes of this study can be useful for the scientific community, the technical stakeholders (e.g., managers in the healthcare and transport sectors), and the common people, providing important information about the performance of these technologies to improve the quality of air in indoor environments.
1 Introduction
Considering that, in industrialized countries, several studies estimate that people spend more than 80%–90% of their time in indoor environments, there has been increasing concern in recent years about the effect of the indoor air quality (IAQ) on human health (Leung, 2015; Spiru and Simona, 2017; Cincinelli and Martellini, 2017). In indoor environments, even the position of furniture can impact the IAQ by affecting the ventilation efficiency (Hormigos-Jimenez et al., 2019).
In the literature, to avoid or reduce the impact of bad IAQ on human health, three approaches are generally followed: (i) reducing the number of sources of emissions, (ii) reducing the amount of pollutants emitted, and (iii) improving the distribution of flows in indoor environments (Batault et al., 2017; González-Martín et al., 2021; Ahmadzadeh et al., 2021; Zuazua-Ros et al., 2023). In this last case, CO2 could be a valid indicator of the bioeffluents released by the occupants of the indoor environment and, therefore, can highlight when the air exchange rate is not adequate (Settimo et al., 2020; Settimo and Avino, 2021; Settimo et al., 2023). However, these strategies are not always feasible or effective and can depend on several factors, such as the pollutants, the characteristics of the indoor environment, and the interactions with the outdoor environment, and may generate additional energy consumption (Batault et al., 2017).
Among the pollutants, the control of airborne microorganisms in indoor environments, especially pathogenic microorganisms, represents a priority. They can be released by infected people or entered through natural and mechanical ventilation systems and transported by dust and particulate matter (PM) (Kumar et al., 2022). Several types of pathogenic microorganisms can be present in the indoor air with potential adverse impacts on human health, such as bacteria (e.g., Escherichia coli, Pseudomonas fluorescens, Legionella pneumophila, and Mycobacterium), viruses (e.g., MS2, Norovirus, and adenovirus), and fungi (e.g., Aspergillus versicolor and Aspergillus niger) (Song et al., 2022).
Several types of treatments for indoor air pollution have been investigated for their effectiveness in removing microorganisms (e.g., fibrous filters) or inactivating them (e.g., ultraviolet (UV) lamps and plasma devices) (Feng et al., 2021; Assadi et al., 2022). Filtration technologies can remove up to 99% of microorganisms, but if proper maintenance is neglected, the possibility of potential re-emission of fungi or bacteria is significant (Mata et al., 2022). Plasma devices seem to be promising in the inactivation of pathogens with an inactivation efficiency of more than 99% for total microbial counts and total fungal counts (Jangra et al., 2023). Furthermore, the use of UV irradiation proved to be an effective approach for air disinfection (Blatchley et al., 2023). UV-A (315–400 nm) and UV-B (280–315 nm) wavelengths are very dangerous for humans and animals because they can penetrate the skin and cause skin cancer and immune system disruption (Memarzadeh, 2021). For this reason, they are generally not used for indoor air disinfection.
UV-C technologies exploit the spectrum of wavelengths from 200 to 280 nm to damage the microorganisms affecting the replication of DNA and RNA (Szczotko et al., 2022). This does not directly kill the microorganism but has an inactivation effect, disrupting its replication and viability (Szczotko et al., 2022; Nguyen et al., 2022a). Generally, UV-C can be produced by (i) low-pressure mercury vapor lamps, (ii) excimer lamps, (iii) light-emitting diodes (LEDs), (iv) pulsed-xenon, or (v) special lamps specifically designed to emit the desired wavelength of UV light, such as far UV-C lamps (emission at 200–230 nm wavelength) (Memarzadeh, 2021; Demeersseman et al., 2023).
The application of UV-C radiation is a well-known strategy for the disinfection of indoor air, with several examples of application, especially in healthcare facilities and indoor public spaces (Atci et al., 2021; Nguyen et al., 2022b; Pereira et al., 2023). Generally, the UV-C lamps can be placed (i) directly in the room which requires air disinfection, irradiating the upper air or the entire room (in this case, in the absence of people), or (ii) within enclosed air systems that recirculate the air in the room (Corrêa et al., 2021).
Despite the absence of the need for chemical reagents, some drawbacks of UV-C disinfection have been highlighted. For instance, in the case of human overexposure, conventional UV-C lamps emitting at 254 nm can determine the effects on skin and eyes. To overcome this drawback, far UV-C lamps that emit at wavelengths less than 230 nm have been investigated (Görlitz et al., 2024). The limited penetration depth in the human skin reduces the potential damage to human health, but they could generate ozone as a byproduct due to the interaction of UV rays with oxygen in the air, and if inhaled, it can cause adverse effects on human health (Görlitz et al., 2024; SCHEER, 2017). Graeffe et al. (2023) discussed the unwanted indoor air quality effects, highlighting that commercial high-intensity UV-C lamps can determine a significant increase in gas concentration and particle numbers with potentially adverse effects on human health. Recently, the scientific community has also focused on the use of LEDs as UV-C sources, given the high power density, the low energy consumption, and the absence of production of ozone (Palma et al., 2024).
In any case, this technology remains promising for the disinfection of indoor environments. For instance, Pseudomonas aeruginosa was effectively inactivated by UV-C (emission at 265 nm) up to 90% with a dose equal to 984 μJ cm−2 (Nguyen et al., 2022a). UV-C devices were also investigated for their effectiveness in healthcare facilities, showing encouraging results for the inactivation of Gram-negative bacilli (de Souza et al., 2022). This approach also proved to be useful for removing the vaccinia virus (as a surrogate for the smallpox virus) from the air of a simulated hospital scenario in order to reduce the risk of infection in indoor environments (McDevitt et al., 2008).
The recent COVID-19 outbreak posed a challenge to the scientific community in finding solutions to limit the spread of the pandemic. COVID-19 is caused by infection with severe acute respiratory syndrome coronavirus 2 (SARS-CoV-2) (Al-Aly et al., 2022). Studies have confirmed that the disease can also be transmitted through airborne particles by asymptomatic infected people, making it difficult to contain the spread of the virus (Wang et al., 2020). For this reason, many governments imposed severe lockdowns in order to limit social activities and contact between people (Lai et al., 2020; Collivignarelli et al., 2021b; Wang H. et al., 2022). In some countries, the use of face masks was also imposed both in indoor and outdoor environments to prevent the release of infectious respiratory particles (IRPs) and the subsequent inhalation by non-infected people (this last protection is not provided with surgical masks) (Wang et al., 2021; Ma et al., 2022).
Another study showed that SARS-CoV-2 remains viable in the air for up to 3 h (Wang et al., 2020), where it can be transported and accumulate on surfaces, persisting for more than 1 day on materials such as plastic and steel (van Doremalen et al., 2020). Although the chronic role of air pollution in increasing the severity of the disease among exposed people has been confirmed, studies on the acute effect of PM on SARS-CoV-2 transmission outdoors are conflicting due to the potential rapid inactivation of the adsorbed virus (Travaglio et al., 2021; Belosi et al., 2021; Paital and Agrawal, 2021; Collivignarelli et al., 2021a; 2023; Pivato et al., 2021). In indoor environments, the role of PM in the spread of SARS-CoV-2 is not completely clear, with studies presenting conflicting results regarding the presence of viral RNA adsorbed onto particles (Santurtún et al., 2022).
To date, it is not clear not how much the different environmental variables affect the rate of inactivation of SARS-CoV-2 in indoor air as this process generally depends on a combination of factors rather than a single variable. In any case, SARS-CoV-2 is very sensitive to high temperatures and sunlight (Jarvis, 2020), so it is plausible to think that the virus could potentially easily spread in indoor environments (e.g., hospitals, transports, and offices), where these conditions are not always granted. Specifically, it has been proved that high-intensity sunlight (such as in summer) can reduce the infectious concentration by 90% in the IRPs, three times faster with respect to low-intensity sunlight conditions such as in winter (Azuma et al., 2020). In general, the distribution and spread of infectious respiratory particles can be influenced by several factors such as the type of pathogen, particle size, health status of the host, mode of expulsion, and environmental conditions (WHO, 2024). In addition, proper ventilation of the environment (artificial or natural) helps reduce the risk of SARS-CoV-2 airborne transmission by reducing the IRPs in the indoor environment (Chen et al., 2021). However, proper ventilation is not always granted (Chen et al., 2021; Ding et al., 2022), and wearing masks can significantly reduce the risk of being infected in an indoor environment by at least two orders of magnitude compared to the “no-mask” scenario (Liu and Qian, 2022). However, this cannot be assumed as a resolutive control measure if not properly coupled with other preventive strategies, such as air disinfection. For instance, even with mask protection, the airborne SARS-CoV-2 in the hospital environment is reduced but not completely avoided (odds ratio of 0.41 vs. 2.56 in case masks are not worn) (Ribaric et al., 2022). In another study, Andrejko et al. showed that wearing an N95/KN95 mask reduces the odds ratio of testing positive after indoor events by 83%, but complete protection (100%) against infection is not provided (Andrejko et al., 2022).
As reported by Niazi et al. (2021), IRPs with dimensions smaller than 2.5 µm can remain suspended in the air for hours. In this case, the use of an adequate face mask, along with other measures, such as maintaining distance, may not be sufficient to reduce the risk of infection with airborne SARS-CoV-2 due to the ability of these IRPs to penetrate/circumnavigate the masks (Niazi et al., 2021).
For this reason, the use of adequate devices for removing or inactivating SARS-CoV-2 from indoor air environments is necessary. Several approaches have been tested, such as filtration, ionization, non-thermal plasma, reactive oxygen species, use of chemical aerosol disinfectants, and heat (Berry et al., 2022; Dowell et al., 2022). However, these studies were mainly carried out in laboratory conditions, especially for chemical and physico-chemical disinfection, with few data on the potential production of polluting and reactive byproducts—potentially harmful to human health. In this field, UV-C devices were also investigated to prevent the spread of the disease in indoor environments, but, to date, the number of reviews on the application of this type of device is strictly limited. For instance, Pertegal et al. (2023) reviewed possible technologies for the inactivation of pathogenic microorganisms in indoor environments, but they did not focus specifically on UV-C (including also, for instance, electrochemical technologies, filtration membranes, and irradiation systems) and SARS-CoV-2. Blatchley et al. (2023) discussed the advantage of using these systems for pandemic control but focused only on far UV-C devices (without considering the effectiveness of emissions at higher wavelengths). García de Abajo et al. (2020) mainly focused on the different configurations of UV-C systems and integration with heating, ventilation, and air-conditioning systems for preventing COVID-19 spread, but they did not provide details on the results of the previous tests conducted on SARS-CoV-2 or its surrogates. Memarzadeh (2021) discussed the potential application of UV for indoor disinfection but without focusing on the application of UV-C devices for SARS-CoV-2 inactivation in the IRPs.
Bhardwaj et al. (2021) reviewed the application of UV-C radiation for indoor air disinfection from coronaviruses while also reporting details regarding the performance of these devices in the inactivation of SARS-CoV-2. However, this last work dates back to the end of 2021, and according to our bibliometric analysis in Scopus® (see Section 2 for search criteria), half of the documents on this topic have been published since 2022.
This work aims to review and discuss the effectiveness of UV-C treatment for SARS-CoV-2 inactivation in IRPs, highlighting the current gaps in the research and suggesting some outlooks for the new studies.
The outcomes of this study can be useful for (i) the scientific community, suggesting tips for future studies according to the main gaps in the research; (ii) the technical stakeholders (e.g., managers in the healthcare and transport sectors), discussing the main application of UV-C for controlling the spread of SARS-CoV-2, other coronaviruses, and pathogenic microorganisms in indoor environments; and (iii) the common people, providing important information about the performance of these technologies to improve the quality of air in common spaces (e.g., houses and offices).
2 Methodology
This work aims to present and discuss the results of the application of UV-C as disinfection technologies for the inactivation of SARS-CoV-2 in indoor air. The paper wants to determine if UV-C could be considered a tool for preventing COVID-19 spread in indoor environments.
Considering the aim of this work, the literature was screened following the preferred reporting items for systematic reviews and meta-analysis (PRISMA) guidelines (Page et al., 2021). In order to avoid overlooking relevant documents, the search was restricted to only articles dealing with SARS-CoV-2 during a second manual screening.
Therefore, in the first screening, the equation “(UV-C OR UVC) AND (virus OR microorganisms) AND indoor” in the “abstract, title, and keywords” field has been used. Scopus® was chosen as the database in order to avoid non-peer-reviewed documents. To discuss novel results, only research articles were considered, excluding review papers, conference proceedings, and books. Documents not written in English were also excluded. After this first screening, 59 documents were selected (Figure 1).
The documents were subjected to a second screening in order to retain only papers directly related to the topic. For instance, documents (i) not referring to SARS-CoV-2, (ii) only based on data estimated by models, (iii) referring to UV-C application for outdoor environments, (iv) reporting only aspects such as built criteria and energy optimization, or (v) presenting data on the effectiveness of UV-C only on surfaces were excluded. Papers focused on UV-C applications for air disinfection of other coronaviruses were taken into account, considering the possible similarities with SARS-CoV-2 (Xu et al., 2020; Bassetti et al., 2020; Zhou et al., 2021). In total, 12 articles were reviewed and included in the manuscript.
The number of cited references in this work is higher because the documents related to aspects such as (i) the concept of indoor air quality, (ii) the general characteristics of UV-C systems, (iii) the effectiveness of UV-C devices on other microorganisms, and (iv) the spread of SARS-CoV-2 and health issues have been retrieved using specific keywords (e.g., “indoor air quality,” “UV-C AND air,” and “(COVID-19 OR SARS-CoV-2) AND human AND health”) and/or referring to the gray literature (e.g., reports and working papers of official agencies).
3 UV-C devices for indoor air disinfection against SARS-CoV-2
Several authors proposed exploiting the effectiveness of UV-C devices in microorganism inactivation in order to prevent infections from SARS-CoV-2 in indoor environments.
Wells–Riley (W-R) is a well-known model to estimate the probability of infection by COVID-19 in an indoor environment using ventilation and quanta generation rates (a representation of infectious doses) (Feng et al., 2024; Riley et al., 1978). This model has the great advantage of not needing unknown viral infectivity parameters, but it assumes that the air in indoor environments is well-mixed. However, when the geometric layout is complex, W-R is often coupled with computational fluid dynamics (CFD) simulations to overcome this limitation (Wang Z. et al., 2022).
CFD models were generally applied to estimate the impact of this technology on SARS-CoV-2 inactivation. For instance, Won et al. (2023) investigated the performance of an upper-room UV-C system emitting at 254 nm wavelength. Their model highlighted that the effectiveness of this device was affected by the recirculation rate and ventilation strategy (Won et al., 2023). Srivastava et al. (2021) used the CFD model to prove that it is possible to reduce the infection risk by adopting limited changes in the existing ventilation systems of an office building. The use of 100% outside air combined with a UV-C device represented the best option that allows reducing the infection risk in the office from 27% (10% of outdoor air and without UV-C treatment) to less than 2% (Srivastava et al., 2021).
Coupling the W-R model with CFD overcomes the main limitation of Riley’s model and provides a better estimate of the breathing and coughing effects of infected people in indoor environments. For instance, Wang Z. et al. (2022) highlighted that the distribution of IRPs in indoor environments is non-uniform and dependent on the type of ventilation, suggesting that a non-coupled approach could lead to the misinterpretation of the infection probability.
The main disadvantage of this combined approach is the need for a robust set of real infection data. However, models were often applied without referring to a previous structured campaign of field tests for estimating the influence of each factor on SARS-CoV-2 inactivation but mainly based on limited literature data on its resistance in indoor environments and potential inhibition factors. Although the number of studies that propose UV-C devices through the application of CFD models is relevant, to date, the documents studying the effectiveness of this technology on SARS-CoV-2 are quite limited and mainly based on simulated environments or model organisms (Table 1).
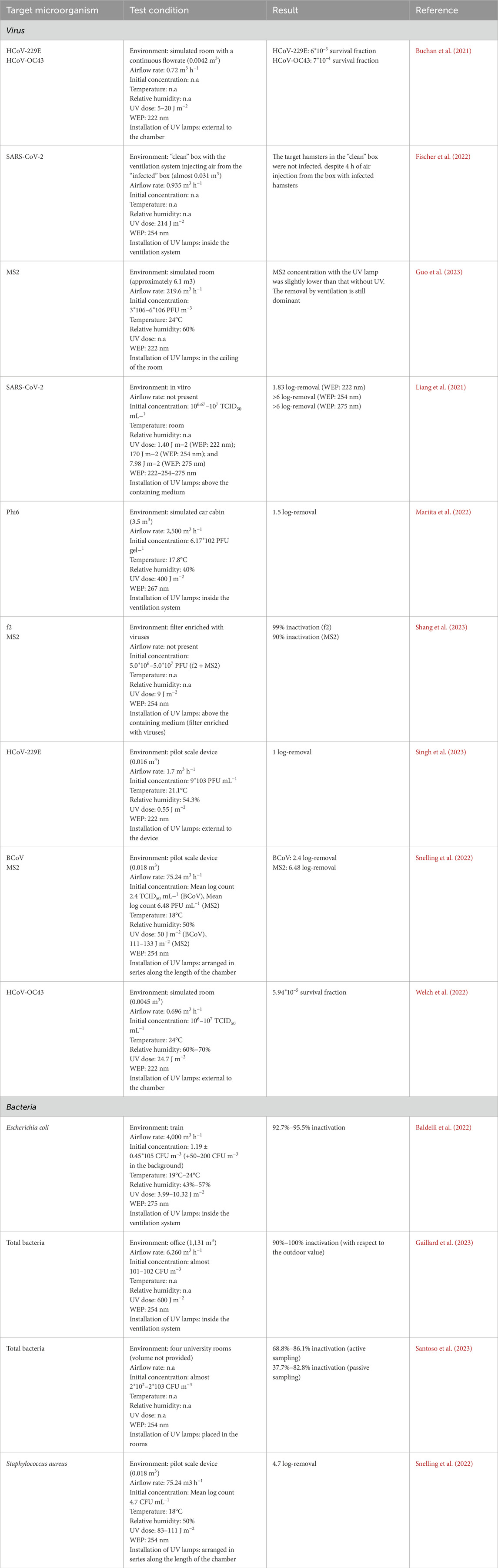
Table 1. Results of UV-C disinfection for SARS-CoV-2 removal from indoor air. n.a, not available; WEP, wavelength of the emission peak; TCID50, 50% tissue culture infectious dose; BCoV, bovine coronavirus.
One of the first studies on this topic was conducted by Fischer et al. (2022), who used model animals. They studied the response of the “target” hamster placed in a box that was forcibly ventilated with air (flow rate: 934.5 L h−1; duration: 4 h) from a “contaminated” box with a hamster infected with SARS-CoV-2. Their results proved that treating the “infected” air with UV-C (particularly with a germicide wavelength of approximately 254 nm) completely prevented infection in the “target” hamsters (Fischer et al., 2022). A surrogate of SARS-CoV-2 (HCoV-229E) was inactivated up to 90% using UV-C (emission at 222 nm wavelength) with a 55 μJ cm−2 radiation dose (Singh et al., 2023). The same surrogate virus, HCoV-229E, along with HCoV-OC43, was used by Buchan et al. (2021) to estimate the influence of far UV-C (emission at 222 nm) on SARS-CoV-2, highlighting the high sensitivity of these viruses to radiation at this wavelength. The high sensibility of HCoV-OC43 to far UV-C emissions was also confirmed by Welch et al. (2022), who evaluated a susceptibility constant of 12.4 cm2 mJ−1.
Shang et al. (2023) tested the effect of a combined filtration and UV-C device of f2 and MS2 phages, discovering that MS2 phages were less sensitive to ultraviolet radiation than f2 phages due to their more resistant protein capsid. After 30 min of UV-C irradiation at a dosage of 9.0 × 103 mJ cm−2, more than 90% and 99% of MS2 and f2 phages retained by the filter, respectively, were inactivated (Shang et al., 2023).
MS2 and bovine coronavirus (BCoV) were effectively inactivated after only a single pass in the UV chamber equipped with lamps emitting at 254 nm. MS2 needed a higher UV dose for complete inactivation than BCoV (11.1 mJ cm−2 vs. 5 mJ cm−2) (Snelling et al., 2022). Bacteriophage Phi6 sensibility to UV-C LED was investigated by Mariita et al. (2022) in order to test the feasibility of this technology for the disinfection of cabin air. They found that 1 log-reduction value was obtained after only 1 min of treatment, which is equivalent to more than 3.5 log-reduction for SARS-CoV-2, being more sensitive to UV-C radiation (Mariita et al., 2022). The Escherichia coli bacteriophage MS2 was used by Guo et al. (2023) to validate a CFD model in which far UV-C devices were used to estimate the disinfection of SARS-CoV-2 in dental clinics and offices. They found that this device can reduce the risk of being infected but not completely. After 10 min of treatment, UV-C can inactivate the virus up to 39% in offices and up to 52% after 20 min of treatment in the clinic with a significant residual amount of virus that can be present in the air (Guo et al., 2023).
In any case, the type of UV source seems to impact the performance against SARS-CoV-2. The in vitro study by Liang et al. (2021) found that UV-C LEDs (emission at 275 nm wavelength) were more effective than a mercury lamp (254 nm) with a log-reduction value higher than 6 after 10 s and 20 s of exposure, respectively, while the excimer lamp (222 nm) was quite ineffective on SARS-CoV-2, showing a log-reduction value lower than 2 after 40 s.
In other studies, model airborne bacteria, which were generally more sensitive than airborne viruses to UV-C effects, were used (Guridi et al., 2019). Santoso et al. (2023) tested a commercial UV-C lamp emitting at 254 nm wavelength (100 mJ cm−2) in several university classrooms and laboratories to evaluate its effect on total bacteria as a predictor of the effect on SARS-CoV-2. They found that UV-C did not remove all bacteria (from 38% to 86%), with the results significantly affected by factors such as air circulation and the equipment placed in the rooms (Santoso et al., 2023).
Baldelli et al. (2022) investigated the effectiveness of UV-C LEDs (emitting at 275 nm wavelength) for SARS-CoV-2 disinfection in a train using E. coli as a model bacterium. They found that more than 90% of bacteria were effectively inactivated, suggesting promising results on human coronaviruses (Baldelli et al., 2022). Gaillard et al. (2023) evaluated the impact of the integration of UV-C technology in the heating, ventilation, and air-conditioning systems of an open-space office. Analyzing bacteria in the indoor air, IAQ is significantly improved, and they assumed that the level of active SARS-CoV-2 could also be strongly reduced by increasing the UV-C intensity (600 J m−2), which is higher than the level required for 90% virus inactivation (Gaillard et al., 2023).
4 Discussion
Providing a single range of effectiveness remains difficult as it is strongly variable depending on the conditions of tests, type of UV lamp, and “target” microorganism. For instance, to date, it is difficult to say which is the best wavelength for SARS-CoV-2 inactivation, given that each study differs for at least one condition (e.g., surrogate, UV dose, and configuration of the UV system). Only in one study, Liang et al. (2021) compared the results obtained in similar conditions except from three different wavelength emissions (222, 254, and 275 nm) and highlighted that treatment with UV-C at 254 nm and 275 nm allowed to obtain comparable results, much higher than those obtained with far-UV-C. However, this study was carried out in vitro, therefore simulating conditions far from the real conditions.
In any case, based on the analysis of the literature, UV-C proved to be effective in the inactivation of SARS-CoV-2 or its surrogates. In 2 out of 12 studies, UV-C rays were tested for preventing the spread of airborne SARS-CoV-2. In one study, model animals were used (Fischer et al., 2022), while in the other, the tests for evaluating the resistance of the virus were carried out in in vitro conditions (Liang et al., 2021). In four studies, the effectiveness against SARS-CoV-2 has been proved by studying model bacteria or evaluating the change in the total bacteria count (Baldelli et al., 2022; Snelling et al., 2022; Santoso et al., 2023; Gaillard et al., 2023), while 6 studies out of 12 reported on the performance of UV-C devices on other viruses, mainly coronaviruses (HCoV-229E, HCoV-OC43, and BCoV) and phages (Buchan et al., 2021; Welch et al., 2022; Snelling et al., 2022; Mariita et al., 2022; Singh et al., 2023; Shang et al., 2023; Guo et al., 2023) (Figure 2).
The choice of using surrogates in previous studies is due to the need to respect stringent laboratory protocols in the case of direct SARS-CoV-2 testing. Therefore, the phase of selection of proper surrogate(s) became crucial (Abkar et al., 2022). Generally, airborne viruses are more sensitive to UV-C rays than airborne bacteria (Guridi et al., 2019), but susceptibility strongly depends on the species, and this assumption cannot always be valid (Zhang and Lai, 2022). There are several reasons for the different inactivation rates among pathogens, such as (i) the dimension of the genome, (ii) the different proteins composing the capsid in viruses, and (iii) the presence of a wall in bacteria (Abkar et al., 2022). Beggs and Avital (2020) suggested that the inactivation rate of airborne SARS-CoV-2 with UV-C is in the range of 0.038–0.059 cm2 mJ−1.
In future studies evaluating the effectiveness of UV-C devices on airborne SARS-CoV-2, the choice of the “target” should focus on an appropriate surrogate, avoiding, for instance, the sole consideration of the variation in the total bacteria count. Furthermore, other bacteria, such as Escherichia coli, may not be a valid choice, given their higher inactivation rate in the case of UV-C exposure compared to SARS-CoV-2 (Abkar et al., 2022).
Most of the studies also avoid considering the influence of variables such as the airflow rate (1 study did not report this information but 11 studies did), initial concentration (2 vs. 10), air temperature (5 vs. 7), relative humidity (6 vs. 6), UV dose (2 vs. 10), and interactions of the virus with other pollutants that can strongly affect the performance of UV-C devices (Elsaid and Ahmed, 2021; Piscitelli et al., 2022). In this last case, the selected studies did not investigate this aspect. This raises the following question: how much do these variables affect the inactivation rate of airborne SARS-CoV-2 by UV-C in indoor environments?
The question is still unsolved, considering that the number of studies regarding the inactivation of this virus in real indoor environments is quite limited and that each study differs for at least one condition. Some studies tried to answer this question with the application of mathematical models or focusing on in vitro response, but the number of studies on real indoor environments is scarce (four vs. eight). This represents an essential point for obtaining reliable and comparable results. Moreover, it is not clear how much the infiltration rate can potentially affect the performance of this technology against SARS-CoV-2 since the influence of this variable has not yet been investigated in depth.
To date, 5 of the 12 examined studies showed the high efficacy of far UV-C lamps in inactivating the target microorganisms, but only 1 of these has evaluated the potential release of ozone, highlighting ozone concentrations below the detection limits of 0.01 ppm even in the presence of a functioning UV lamp (Singh et al., 2023). In any case, in-depth studies on the release of potentially toxic byproducts are still necessary to carry out a complete risk/benefit assessment and evaluate the correct procedure for using far UV-C lamps.
The study and development of effective technologies in the inactivation of pathogens in the air in indoor environments are of fundamental importance in order to quickly limit the spread of future pandemics. Furthermore, the development of technologies that are effective in preventing the spread of pathogenic viruses in indoor environments can potentially present a greater benefit-cost ratio compared to pharmaceutical post-treatment of infected patients (Fischer et al., 2022). Recent studies also highlighted that UV irradiation modules added to the artificial ventilation system were very efficient in the inactivation of pathogens but can also lead to a reduction in the energy required for the ventilation, with a strong energy saving, if coupled with a better air distribution strategy (Gaillard et al., 2023). Moreover, for these reasons, the study on the applications of UV-C disinfection systems as a technology to reduce the infectious risk of SARS-CoV-2 in indoor environments should be stimulated.
5 Conclusion
In this work, the effectiveness of UV-C technologies against airborne SARS-CoV-2 in indoor environments has been reviewed. The following aspects should be taken into account:
• Based on the analysis of the literature, UV-C proved to be effective in the inactivation of SARS-CoV-2 or its surrogates. However, given the different conditions in which the tests were performed, providing a single range of performances (e.g., in terms of log-removal values) remains difficult.
• In some studies, the incorrect selection of the surrogate with a higher susceptibility to UV-C than airborne SARS-CoV-2 (e.g., E. coli) or the evaluation of the total bacteria count could have led to misleading results. In this regard, the selection of a proper surrogate is suggested for future studies.
To date, the question “how much do variables, such as the type of ventilation, air temperature, humidity, and interactions with other pollutants, affect the inactivation rate of airborne SARS-CoV-2 in indoor environments?” is mainly answered by the application of mathematical models, but the number of studies about the inactivation of this virus in real indoor environments is quite limited. Further studies are also needed to accurately define the potential ozone release due to far UV-C disinfection although preliminary data have shown that the ozone concentration in the vicinity of operating UV-C devices is not significantly different from that in the environment.
Author contributions
ECR: validation and writing–review and editing. CV: investigation, visualization, and writing–original draft. SB: visualization and writing–original draft. MCM: conceptualization, investigation, methodology, validation, and writing–review and editing. AA: validation, visualization, and writing–original draft. MS: visualization and writing–review and editing. VT: conceptualization, supervision, validation, and writing–review and editing. MCC: conceptualization, supervision, validation, and writing–review and editing.
Funding
The author(s) declare that no financial support was received for the research, authorship, and/or publication of this article. This research did not receive external funding.
Conflict of interest
The authors declare that the research was conducted in the absence of any commercial or financial relationships that could be construed as a potential conflict of interest.
The author(s) declared that they were an editorial board member of Frontiers, at the time of submission. This had no impact on the peer review process and the final decision.
Generative AI statement
The author(s) declare that no Generative AI was used in the creation of this manuscript.
Publisher’s note
All claims expressed in this article are solely those of the authors and do not necessarily represent those of their affiliated organizations, or those of the publisher, the editors and the reviewers. Any product that may be evaluated in this article, or claim that may be made by its manufacturer, is not guaranteed or endorsed by the publisher.
References
Abkar, L., Zimmermann, K., Dixit, F., Kheyrandish, A., and Mohseni, M. (2022). CoViD-19 pandemic lesson learned-critical parameters and research needs for UVC inactivation of viral aerosols. J. Hazard. Mater. Adv. 8, 100183. doi:10.1016/j.hazadv.2022.100183
Ahmadzadeh, M., Farokhi, E., and Shams, M. (2021). Investigating the effect of air conditioning on the distribution and transmission of CoViD-19 virus particles. J. Clean. Prod. 316, 128147. doi:10.1016/j.jclepro.2021.128147
Al-Aly, Z., Bowe, B., and Xie, Y. (2022). Long COVID after breakthrough SARS-CoV-2 infection. Nat. Med. 28, 1461–1467. doi:10.1038/s41591-022-01840-0
Andrejko, K. L., Pry, J. M., Myers, J. F., Fukui, N., DeGuzman, J. L., Openshaw, J., et al. (2022). Effectiveness of face mask or respirator use in indoor public settings for prevention of SARS-CoV-2 infection — California, february–december 2021. MMWR Morb. Mortal. Wkly. Rep. 71, 212–216. doi:10.15585/mmwr.mm7106e1
Assadi, I., Guesmi, A., Baaloudj, O., Zeghioud, H., Elfalleh, W., Benhammadi, N., et al. (2022). Review on inactivation of airborne viruses using non-thermal plasma technologies: from MS2 to coronavirus. Environ. Sci. Pollut. Res. 29, 4880–4892. doi:10.1007/s11356-021-17486-3
Atci, F., Cetin, Y. E., Avci, M., and Aydin, O. (2021). Evaluation of in-duct UV-C lamp array on air disinfection: a numerical analysis. Sci. Technol. Built Environ. 27, 98–108. doi:10.1080/23744731.2020.1776549
Azuma, K., Yanagi, U., Kagi, N., Kim, H., Ogata, M., and Hayashi, M. (2020). Environmental factors involved in SARS-CoV-2 transmission: effect and role of indoor environmental quality in the strategy for COVID-19 infection control. Environ. health Prev. Med. 25, 66–16. doi:10.1186/s12199-020-00904-2
Baldelli, G., Aliano, M. P., Amagliani, G., Magnani, M., Brandi, G., Pennino, C., et al. (2022). Airborne microorganism inactivation by a UV-C LED and ionizer-based continuous sanitation air (CSA) system in train environments. Int. J. Environ. Res. Public Health 19, 1559. doi:10.3390/ijerph19031559
Bassetti, M., Vena, A., and Giacobbe, D. R. (2020). The novel Chinese coronavirus (2019-nCoV) infections: challenges for fighting the storm. Eur. J. Clin. Invest. 50, e13209. doi:10.1111/eci.13209
Batault, F., Héquet, V., Raillard, C., Thévenet, F., Locoge, N., and Le Coq, L. (2017). How chemical and physical mechanisms enable the influence of the operating conditions in a photocatalytic indoor air treatment device to be modeled. Chem. Eng. J. 307, 766–775. doi:10.1016/j.cej.2016.08.118
Beggs, C. B., and Avital, E. J. (2020). Upper-room ultraviolet air disinfection might help to reduce COVID-19 transmission in buildings: a feasibility study. PeerJ 8, e10196. doi:10.7717/peerj.10196
Belosi, F., Conte, M., Gianelle, V., Santachiara, G., and Contini, D. (2021). On the concentration of SARS-CoV-2 in outdoor air and the interaction with pre-existing atmospheric particles. Environ. Res. 193, 110603. doi:10.1016/j.envres.2020.110603
Berry, G., Parsons, A., Morgan, M., Rickert, J., and Cho, H. (2022). A review of methods to reduce the probability of the airborne spread of COVID-19 in ventilation systems and enclosed spaces. Environ. Res. 203, 111765. doi:10.1016/j.envres.2021.111765
Bhardwaj, S. K., Singh, H., Deep, A., Khatri, M., Bhaumik, J., Kim, K. H., et al. (2021). UVC-based photoinactivation as an efficient tool to control the transmission of coronaviruses. Sci. Total Environ. 792, 148548. doi:10.1016/j.scitotenv.2021.148548
Blatchley, E. R., Brenner, D. J., Claus, H., Cowan, T. E., Linden, K. G., Liu, Y., et al. (2023). Far UV-C radiation: an emerging tool for pandemic control. Crit. Rev. Environ. Sci. Technol. 53, 733–753. doi:10.1080/10643389.2022.2084315
Buchan, A. G., Yang, L., Welch, D., Brenner, D. J., and Atkinson, K. D. (2021). Improved estimates of 222 nm far-UVC susceptibility for aerosolized human coronavirus via a validated high-fidelity coupled radiation-CFD code. Sci. Rep. 11, 19930. doi:10.1038/s41598-021-99204-0
Chen, B., Jia, P., and Han, J. (2021). Role of indoor aerosols for COVID-19 viral transmission: a review. Environ. Chem. Lett. 19, 1953–1970. doi:10.1007/s10311-020-01174-8
Cincinelli, A., and Martellini, T. (2017). Indoor air quality and health. Int. J. Environ. Res. Public Health 14, 1286. doi:10.3390/ijerph14111286
Collivignarelli, M. C., Abbà, A., Caccamo, F. M., Bertanza, G., Pedrazzani, R., Baldi, M., et al. (2021a). Can particulate matter be identified as the primary cause of the rapid spread of CoViD-19 in some areas of Northern Italy? Environ. Sci. Pollut. Res. 28, 33120–33132. doi:10.1007/s11356-021-12735-x
Collivignarelli, M. C., Bellazzi, S., Caccamo, F. M., and Carnevale Miino, M. (2023). Discussion about the latest findings on the possible relation between air particulate matter and COVID-19. Int. J. Environ. Res. Public Health 20, 5132. doi:10.3390/ijerph20065132
Collivignarelli, M. C., De Rose, C., Abbà, A., Baldi, M., Bertanza, G., Pedrazzani, R., et al. (2021b). Analysis of lockdown for CoViD-19 impact on NO2 in London, Milan and Paris: what lesson can be learnt? Process Saf. Environ. Prot. 146, 952–960. doi:10.1016/j.psep.2020.12.029
Corrêa, T. Q., Blanco, K. C., Vollet-Filho, J. D., Morais, V., Trevelin, W., Pratavieira, S., et al. (2021). Efficiency of an air circulation decontamination device for micro-organisms using ultraviolet radiation. J. Hosp. Infect. 115, 32–43. doi:10.1016/j.jhin.2021.06.002
Demeersseman, N., Saegeman, V., Cossey, V., Devriese, H., and Schuermans, A. (2023). Shedding a light on ultraviolet-C technologies in the hospital environment. J. Hosp. Infect. 132, 85–92. doi:10.1016/j.jhin.2022.12.009
de Souza, S. O., Cardoso, Jr A. A., Sarmento, A. S. C., and d’Errico, F. (2022). Effectiveness of a UVC air disinfection system for the HVAC of an ICU. Eur. Phys. J. Plus 137, 37. doi:10.1140/epjp/s13360-021-02240-y
Ding, E., Zhang, D., and Bluyssen, P. M. (2022). Ventilation regimes of school classrooms against airborne transmission of infectious respiratory droplets: a review. Build. Environ. 207, 108484. doi:10.1016/j.buildenv.2021.108484
Dowell, D., Lindsley, W. G., and Brooks, J. T. (2022). Reducing SARS-CoV-2 in shared indoor air. Jama 328 (2), 141–142. doi:10.1001/jama.2022.9970
Elsaid, A. M., and Ahmed, M. S. (2021). Indoor air quality strategies for air-conditioning and ventilation systems with the spread of the global coronavirus (COVID-19) epidemic: improvements and recommendations. Environ. Res. 199, 111314. doi:10.1016/j.envres.2021.111314
Feng, Y., Fan, Y., Luo, X., and Ge, J. (2024). A Wells-Riley based COVID-19 infectious risk assessment model combining both short range and room scale effects. In, Building simulation (Vol. 17, No. 1, pp. 93–111). doi:10.1007/s12273-023-1060-yBeijing: Tsinghua University Press.
Feng, Z., Wei, F., Li, H., and Yu, C. W. (2021). Evaluation of indoor disinfection technologies for airborne disease control in hospital. Indoor Built Environ. 30, 727–731. doi:10.1177/1420326X211002948
Fischer, R. J., Port, J. R., Holbrook, M. G., Yinda, K. C., Creusen, M., ter Stege, J., et al. (2022). UV-C light completely blocks aerosol transmission of highly contagious SARS-CoV-2 variants WA1 and delta in hamsters. Environ. Sci. Technol. 56, 12424–12430. doi:10.1021/acs.est.2c02822
Gaillard, A., Lohse, D., Bonn, D., and Yigit, F. (2023). Reconciling airborne disease transmission concerns with energy saving requirements: the potential of UV-C pathogen deactivation and air distribution optimization. Indoor Air 2023, 1–12. doi:10.1155/2023/3927171
García de Abajo, F. J., Hernández, R. J., Kaminer, I., Meyerhans, A., Rosell-Llompart, J., and Sanchez-Elsner, T. (2020). Back to normal: an old physics route to reduce SARS-CoV-2 transmission in indoor spaces. ACS Nano 14, 7704–7713. doi:10.1021/acsnano.0c04596
González-Martín, J., Kraakman, N. J. R., Pérez, C., Lebrero, R., and Muñoz, R. (2021). A state–of–the-art review on indoor air pollution and strategies for indoor air pollution control. Chemosphere 262, 128376. doi:10.1016/j.chemosphere.2020.128376
Görlitz, M., Justen, L., Rochette, P. J., Buonanno, M., Welch, D., Kleiman, N. J., et al. (2024). Assessing the safety of new germicidal far-UVC technologies. Photochem. Photobiol. 100 (3), 501–520. doi:10.1111/php.13866
Graeffe, F., Luo, Y., Guo, Y., and Ehn, M. (2023). Unwanted indoor air quality effects from using ultraviolet C lamps for disinfection. Environ. Sci. Technol. Lett. 10, 172–178. doi:10.1021/acs.estlett.2c00807
Guo, K., Pan, Y., Chan, H. F. R., Ho, K. F., and Chen, C. (2023). Far-UVC disinfection of airborne and surface virus in indoor environments: laboratory experiments and numerical simulations. Build. Environ. 245, 110900. doi:10.1016/j.buildenv.2023.110900
Guridi, A., Sevillano, E., de la Fuente, I., Mateo, E., Eraso, E., and Quindós, G. (2019). Disinfectant activity of A portable ultraviolet C equipment. Int. J. Environ. Res. Public Health 16, 4747. doi:10.3390/ijerph16234747
Hormigos-Jimenez, S., Padilla-Marcos, M. Á., Meiss, A., Gonzalez-Lezcano, R. A., and Feijó-Muñoz, J. (2019). Assessment of the ventilation efficiency in the breathing zone during sleep through computational fluid dynamics techniques. J. Build. Phys. 42 (4), 458–483. doi:10.1177/1744259118771314
Jangra, R., Ahlawat, K., Dixit, A., and Prakash, R. (2023). Efficient deactivation of aerosolized pathogens using a dielectric barrier discharge based cold-plasma detergent in environment device for good indoor air quality. Sci. Rep. 13 (1), 10295. doi:10.1038/s41598-023-37014-2
Jarvis, M. C. (2020). Aerosol transmission of SARS-CoV-2: physical principles and implications. Front. Public Health 8, 590041. doi:10.3389/fpubh.2020.590041
Kumar, P., Singh, A. B., and Singh, R. (2022). Comprehensive health risk assessment of microbial indoor air quality in microenvironments. PLoS One 17, e0264226. doi:10.1371/journal.pone.0264226
Lai, S., Ruktanonchai, N. W., Zhou, L., Prosper, O., Luo, W., Floyd, J. R., et al. (2020). Effect of non-pharmaceutical interventions to contain COVID-19 in China. Nature 585, 410–413. doi:10.1038/s41586-020-2293-x
Leung, D. Y. C. (2015). Outdoor-indoor air pollution in urban environment: challenges and opportunity. Front. Environ. Sci. 2. doi:10.3389/fenvs.2014.00069
Liang, J.-J., Liao, C.-C., Chang, C.-S., Lee, C. Y., Chen, S. Y., Huang, S. B., et al. (2021). The effectiveness of far-ultraviolet (UVC) light prototype devices with different wavelengths on disinfecting SARS-CoV-2. Appl. Sci. 11, 10661. doi:10.3390/app112210661
Liu, F., and Qian, H. (2022). Uncertainty analysis of facemasks in mitigating SARS-CoV-2 transmission. Environ. Pollut. 303, 119167. doi:10.1016/j.envpol.2022.119167
Ma, X., Wu, D., Wang, J., Sun, F., Li, Z., Zhang, L., et al. (2022). Make the invisible visible: valuation of the hidden cost of particulate-filtering facemask use against air pollution in China. J. Clean. Prod. 372, 133667. doi:10.1016/j.jclepro.2022.133667
Mariita, R. M., Davis, J. H., Lottridge, M. M., Randive, R. V., Witting, H., and Yu, J. (2022). Towards a healthy car: UVC LEDs in an automobile’s HVAC demonstrates effective disinfection of cabin air. Atmos. (Basel) 13, 1926. doi:10.3390/atmos13111926
Mata, T. M., Martins, A. A., Calheiros, C. S., Villanueva, F., Alonso-Cuevilla, N. P., Gabriel, M. F., et al. (2022). Indoor air quality: a review of cleaning technologies. Environments 9 (9), 118. doi:10.3390/environments9090118
McDevitt, J. J., Milton, D. K., Rudnick, S. N., and First, M. W. (2008). Inactivation of poxviruses by upper-room UVC light in a simulated hospital room environment. PLoS One 3, e3186. doi:10.1371/journal.pone.0003186
Memarzadeh, F. (2021). A review of recent evidence for utilizing ultraviolet irradiation technology to disinfect both indoor air and surfaces. Appl. Biosaf. 26, 52–56. doi:10.1089/apb.20.0056
Nguyen, T. T., He, C., Carter, R., Ballard, E. L., Smith, K., Groth, R., et al. (2022a). The effectiveness of ultraviolet-C (UV-C) irradiation on the viability of airborne Pseudomonas aeruginosa. Int. J. Environ. Res. Public Health 19, 13706. doi:10.3390/ijerph192013706
Nguyen, T. T., Johnson, G. R., Bell, S. C., and Knibbs, L. D. (2022b). A systematic literature review of indoor air disinfection techniques for airborne bacterial respiratory pathogens. Int. J. Environ. Res. Public Health 19, 1197. doi:10.3390/ijerph19031197
Niazi, S., Groth, R., Spann, K., and Johnson, G. R. (2021). The role of respiratory droplet physicochemistry in limiting and promoting the airborne transmission of human coronaviruses: a critical review. Environ. Pollut. 276, 115767. doi:10.1016/j.envpol.2020.115767
Page, M. J., McKenzie, J. E., Bossuyt, P. M., Boutron, I., Hoffmann, T. C., Mulrow, C. D., et al. (2021). The PRISMA 2020 statement: an updated guideline for reporting systematic reviews. Int. J. Surg. 88, 105906. doi:10.1016/j.ijsu.2021.105906
Paital, B., and Agrawal, P. K. (2021). Air pollution by NO2 and PM2.5 explains COVID-19 infection severity by overexpression of angiotensin-converting enzyme 2 in respiratory cells: a review. Environ. Chem. Lett. 19, 25–42. doi:10.1007/s10311-020-01091-w
Palma, F., Baldelli, G., Amagliani, G., Aliano, M. P., Magnani, M., Brandi, G., et al. (2024). Assessment of a new UV-C light-emitting diode–based technology for air sanitization in indoor sports environments. Indoor Built Environ. 33 (2), 237–249. doi:10.1177/1420326x231193597
Pereira, A. R., Braga, D. F. O., Vassal, M., Gomes, I. B., and Simões, M. (2023). Ultraviolet C irradiation: a promising approach for the disinfection of public spaces? Sci. Total Environ. 879, 163007. doi:10.1016/j.scitotenv.2023.163007
Pertegal, V., Riquelme, E., Lozano-Serra, J., Cañizares, P., Rodrigo, M. A., Sáez, C., et al. (2023). Cleaning technologies integrated in duct flows for the inactivation of pathogenic microorganisms in indoor environments: a critical review of recent innovations and future challenges. J. Environ. Manage 345, 118798. doi:10.1016/j.jenvman.2023.118798
Piscitelli, P., Miani, A., Setti, L., De Gennaro, G., Rodo, X., Artinano, B., et al. (2022). The role of outdoor and indoor air quality in the spread of SARS-CoV-2: overview and recommendations by the research group on COVID-19 and particulate matter (RESCOP commission). Environ. Res. 211, 113038. doi:10.1016/j.envres.2022.113038
Pivato, A., Amoruso, I., Formenton, G., Di Maria, F., Bonato, T., Vanin, S., et al. (2021). Evaluating the presence of SARS-CoV-2 RNA in the particulate matters during the peak of COVID-19 in Padua, northern Italy. Sci. Total Environ. 784, 147129. doi:10.1016/j.scitotenv.2021.147129
Ribaric, N. L., Vincent, C., Jonitz, G., Hellinger, A., and Ribaric, G. (2022). Hidden hazards of SARS-CoV-2 transmission in hospitals: a systematic review. Indoor Air 32, e12968. doi:10.1111/ina.12968
Riley, E. C., Murphy, G., and Riley, R. L. (1978). Airborne spread of measles in a suburban elementary school. Am. J. Epidemiol. 107 (5), 421–432. doi:10.1093/oxfordjournals.aje.a112560
Santoso, I., Fadhilah, Q. G., Abdullah, S., Tamrela, H., and Sugiyanto, A. (2023). Microbial air pollutant control using commercial UV-C lamp for preparing Re-opening class activities at universitas Indonesia. Int. J. Adv. Sci. Eng. Inf. Technol. 13, 276–282. doi:10.18517/ijaseit.13.1.17474
Santurtún, A., Colom, M. L., Fdez-Arroyabe, P., Real, Á. d., Fernández-Olmo, I., and Zarrabeitia, M. T. (2022). Exposure to particulate matter: direct and indirect role in the COVID-19 pandemic. Environ. Res. 206, 112261. doi:10.1016/j.envres.2021.112261
SCHEER (2017) Biological effects of UV-C radiation relevant to health with particular reference to UV-C lamps
Settimo, G., and Avino, P. (2021). The dichotomy between indoor air quality and energy efficiency in light of the onset of the COVID-19 pandemic. Atmosphere 12 (6), 791. doi:10.3390/atmos12060791
Settimo, G., Gola, M., and Capolongo, S. (2020). The relevance of indoor air quality in hospital settings: from an exclusively biological issue to a global approach in the Italian context. Atmosphere 11 (4), 361. doi:10.3390/atmos11040361
Settimo, G., Yu, Y., Gola, M., Buffoli, M., and Capolongo, S. (2023). Challenges in IAQ for indoor spaces: a comparison of the reference guideline values of indoor air pollutants from the governments and international institutions. Atmosphere 14 (4), 633. doi:10.3390/atmos14040633
Shang, M., Kong, Y., Yang, Z., Cheng, R., Zheng, X., Liu, Y., et al. (2023). Removal of virus aerosols by the combination of filtration and UV-C irradiation. Front. Environ. Sci. Eng. 17, 27. doi:10.1007/s11783-023-1627-y
Singh, D., Soorneedi, A. R., Vaze, N., Domitrovic, R., Sharp, F., Lindsey, D., et al. (2023). Assessment of SARS-CoV-2 surrogate inactivation on surfaces and in air using UV and blue light-based intervention technologies. J. Air Waste Manage Assoc. 73, 200–211. doi:10.1080/10962247.2022.2157907
Snelling, W. J., Afkhami, A., Turkington, H. L., Carlisle, C., Cosby, S. L., Hamilton, J. W., et al. (2022). Efficacy of single pass UVC air treatment for the inactivation of coronavirus, MS2 coliphage and Staphylococcus aureus bioaerosols. J. Aerosol Sci. 164, 106003. doi:10.1016/j.jaerosci.2022.106003
Song, L., Zhou, J., Wang, C., Meng, G., Li, Y., Jarin, M., et al. (2022). Airborne pathogenic microorganisms and air cleaning technology development: a review. J. Hazard Mater 424, 127429. doi:10.1016/j.jhazmat.2021.127429
Spiru, P., and Simona, P. L. (2017). A review on interactions between energy performance of the buildings, outdoor air pollution and the indoor air quality. Energy Procedia 128, 179–186. doi:10.1016/j.egypro.2017.09.039
Srivastava, S., Zhao, X., Manay, A., and Chen, Q. (2021). Effective ventilation and air disinfection system for reducing coronavirus disease 2019 (COVID-19) infection risk in office buildings. Sustain Cities Soc. 75, 103408. doi:10.1016/j.scs.2021.103408
Szczotko, M., Orych, I., Mąka, Ł., and Solecka, J. (2022). A review of selected types of indoor air purifiers in terms of microbial air contamination reduction. Atmos. (Basel) 13, 800. doi:10.3390/atmos13050800
Travaglio, M., Yu, Y., Popovic, R., Selley, L., Leal, N. S., and Martins, L. M. (2021). Links between air pollution and COVID-19 in England. Environ. Pollut. 268, 115859. doi:10.1016/j.envpol.2020.115859
van Doremalen, N., Bushmaker, T., Morris, D. H., Holbrook, M. G., Gamble, A., Williamson, B. N., et al. (2020). Aerosol and surface stability of SARS-CoV-2 as compared with SARS-CoV-1. N. Engl. J. Med. 382, 1564–1567. doi:10.1056/NEJMc2004973
Wang, H., Zheng, Y., de Jonge, M. I., Wang, R., Verhagen, L. M., Chen, Y., et al. (2022). Lockdown measures during the COVID-19 pandemic strongly impacted the circulation of respiratory pathogens in Southern China. Sci. Rep. 12, 16926. doi:10.1038/s41598-022-21430-x
Wang, J., Pan, L., Tang, S., Ji, J. S., and Shi, X. (2020). Mask use during COVID-19: a risk adjusted strategy. Environ. Pollut. 266, 115099. doi:10.1016/j.envpol.2020.115099
Wang, Y., Deng, Z., and Shi, D. (2021). How effective is a mask in preventing COVID-19 infection? Med. Devices Sens. 4, e10163. doi:10.1002/mds3.10163
Wang, Z., Galea, E. R., Grandison, A., Ewer, J., and Jia, F. (2022). A coupled Computational Fluid Dynamics and Wells-Riley model to predict COVID-19 infection probability for passengers on long-distance trains. Saf. Sci. 147, 105572. doi:10.1016/j.ssci.2021.105572
Welch, D., Buonanno, M., Buchan, A. G., Yang, L., Atkinson, K. D., Shuryak, I., et al. (2022). Inactivation rates for airborne human coronavirus by low doses of 222 nm far-UVC radiation. Viruses 14, 684. doi:10.3390/v14040684
WHO (2024). Global technical consultation report on proposed terminology for pathogens that transmit through the air. Geneva, Switzerland: World Health Organization. Available at: https://cdn.who.int/media/docs/default-source/documents/emergencies/global-technical-consultation-report-on-proposed-terminology-for-pathogens-that-transmit-through-the-air.pdf (Accessed July 15, 2024).
Won, Y., Rim, D., Mistrick, R., and Bahnfleth, W. (2023). CFD modeling of room airflow effects on inactivation of aerosol SARS-CoV-2 by an upper-room ultraviolet germicidal irradiation (UVGI) system. Sci. Technol. Built Environ. 29, 719–729. doi:10.1080/23744731.2023.2247947
Xu, J., Zhao, S., Teng, T., Abdalla, A. E., Zhu, W., Xie, L., et al. (2020). Systematic comparison of two animal-to-human transmitted human coronaviruses: SARS-CoV-2 and SARS-CoV. Viruses 12, 244. doi:10.3390/v12020244
Zhang, H., and Lai, A. C. K. (2022). Evaluation of single-pass disinfection performance of far-UVC light on airborne microorganisms in duct flows. Environ. Sci. Technol. 56, 17849–17857. doi:10.1021/acs.est.2c04861
Zhou, H., Yang, J., Zhou, C., Chen, B., Fang, H., Chen, S., et al. (2021). A review of SARS-CoV2: compared with SARS-CoV and MERS-CoV. Front. Med. (Lausanne) 8, 628370. doi:10.3389/fmed.2021.628370
Keywords: COVID-19, pandemic, indoor pollutants, airborne virus, pathogens, UV disinfection
Citation: Rada EC, Vignali C, Bellazzi S, Carnevale Miino M, Abbà A, Szabó M, Torretta V and Collivignarelli MC (2025) Disinfection of indoor air for the inactivation of SARS-CoV-2: a review of the effectiveness of UV-C technology and gaps in research. Front. Built Environ. 10:1523055. doi: 10.3389/fbuil.2024.1523055
Received: 06 November 2024; Accepted: 09 December 2024;
Published: 03 January 2025.
Edited by:
Hasim Altan, Prince Mohammad bin Fahd University, Saudi ArabiaReviewed by:
Roberto Alonso González-Lezcano, CEU San Pablo University, SpainGaetano Settimo, Istituto Superiore di Sanità, Italy
Copyright © 2025 Rada, Vignali, Bellazzi, Carnevale Miino, Abbà, Szabó, Torretta and Collivignarelli. This is an open-access article distributed under the terms of the Creative Commons Attribution License (CC BY). The use, distribution or reproduction in other forums is permitted, provided the original author(s) and the copyright owner(s) are credited and that the original publication in this journal is cited, in accordance with accepted academic practice. No use, distribution or reproduction is permitted which does not comply with these terms.
*Correspondence: Elena Cristina Rada, ZWxlbmEucmFkYUB1bmluc3VicmlhLml0