- 1Engineering Department, Changde Renhe Construction Co., Ltd, Changde, China
- 2School of Civil and Environmental Engineering, and Construction Management, University of Texas at San Antonio, San Antonio, TX, United States
Since Artificial ground Freezing (AGF) appeared in the 1880s in the mining sector in Europe, it has been used for various construction applications worldwide. In recent years, it has been increasingly popular in urban projects due to its versatility and applicability to complicated site conditions. So far, it has been used to stabilize substrata to nearly 1,000 m below the ground surface, which is considered not possible for many other ground improvement technologies. Due to the growth in field applications, the practice and theories related to AGF have become more mature in the most recent two decades. The improvement in understanding of this topic is a result of lessons that have been learned through numerous projects, as well as a variety of comprehensive studies that have been completed. This paper reviews the existing practice, the recent development on AGF and the challenges of AGF.
1 Introduction
Artificial ground freezing (AGF) is a soil stabilization technique, which involves removing heat from the ground through circulation of a chilled liquid to convert pore water into ice (Harris, 1995; Jones et al., 1982; Schmall and Braun, 2006). The ice in the pores of soil mass bonds soil particles to form a soil-ice composite that temporarily stabilizes soil, cuts off water flow, and provides support for excavations (Hashemi and Sliepcevich, 1973; Lacy and Floess, 1988; Sanger, 1968). The impervious nature of ice also makes AGF suitable for temporarily immobilization of various species of contaminants, including radioactive materials (International Atomic Energy Agency, 2006). In summary, AGF is a very useful technology for many applications even though it is not among the most used ground improvement technologies. The first reported use of Artificial Ground Freezing (AGF) for underground construction occurred in 1862 in Swansea, South Wales, where a mineshaft was constructed by circulating chilled brine through tubing sunk into the ground (Harris, 1995). This concept was later patented in 1883 by German engineer F. H. Poetsch to prevent water ingress in Belgian coal mines (Harris, 1995; Schäfers and Hab, 2005). The principles outlined in Poetsch’s patent remain relevant to modern projects. Since the 1880s, AGF technology has been implemented at increasing depths in various countries. Germany achieved a penetration depth of approximately 600 m, Canada reached 900 m in the 1950s, and England reached 975 m in the 1970s (Harris, 1995; Hass, 2006). China has also reported multiple AGF projects with depths exceeding 800 m, with the deepest being 990 m in Shanxi Province (Wu et al., 2020; Zhang et al., 2012). These deep applications are primarily used for sinking mining shafts and open pits. In China alone, the total length of shafts constructed using AGF reached 227 km by 2010 (Zhou et al., 2022). The first urban underground application of AGF was in 1886 in Sweden for a pedestrian tunnel (Kinoshita, 1982). Since then, AGF has been widely used in urban transportation systems, especially in densely populated coastal cities. In recent years, China has extensively employed AGF for its inner-city transportation projects, initially as a supplement to jet grouting and then increasingly as the preferred method. By 2016, more than 700 urban underground AGF projects had been completed in China, mostly in large coastal cities (Zhou et al., 2022).
2 Principles and practice
The working principle of AGF is relatively simple. The heat energy of underground soil and water is extracted by circulating cooler liquid so that ice is formed to create frozen soil. The most important component of AGF is the freezing system, which circulates low temperature liquid to exchange heat with subsurface soil. The original patented prototype was a refrigerant-brine system, which includes two closed loops: cooling and circulation, which work independently (Alzoubi et al., 2020). The cooling system functions like a refrigerator, which lowers the temperature of the brine (typically calcium chloride). Then the brine is circulated through buried tubes to cool the soil. The heat exchange of two loops is achieved to lower the brine temperature after it returns from circulation. For the cooling system, the used refrigerant includes Freon, ammonia, R22 and fluorine (Zhou et al., 2022; Zhou et al., 1997). So far, the refrigerant-brine system can lower the brine temperature to −22°C to −35°C (Harris, 1995; Zhou et al., 2022). In contrast, an open loop cooling system uses liquid nitrogen (LN) to extract heat from soil (Alzoubi et al., 2020). The returning nitrogen gas is released to the air. Such a system can lower the soil temperature to approximately −100°C, which turns pore water into ice immediately. The idea of LN for AGF came out around 1960s and soon rapidly became an alternative cooling choice (Jessberger and Jagow, 1988; Zhou et al., 2022). Compared with refrigerant-brine cooling system, LN system is safer because nitrogen is harmless. For both cooling systems, once frozen soil mass is formed it is typical to maintain the temperature between −30°C to −15°C to save energy (Chang and Lacy, 2008).
Theoretically, this method should be applicable to all soils and rocks as long as there is sufficient water and water flow is insignificant (Harris, 1995). According to Harris (1995), Thorburn and Littlejohn (1992), and Karol (1990), the AGF is probably the only ground improvement method that can be used for any type of soil and rock. As a matter of fact, AGF has been successfully used from very soft clay to rock (Russo et al., 2015; Vitel et al., 2016b; Zhou and Tang, 2018). Another outstanding advantage of AGF is great treatment depth, which is typically a few hundred meters and can be as deep as 1,000 m (Harris, 1995; Zhou et al., 2022). The deepest jet grouting and deep soil mixing can reach 150 m, while stone columns and rammed aggregate columns typically cannot be more than 20 m (Schaefer et al., 2017). The disadvantage of AGF lies in two aspects: temporary and costly. The soil will thaw shortly after the circulation pauses. The cost of AGF includes drilling, installation, freezing and maintaining temperature, which could be significantly higher than other methods.
3 Practice, research and development
AGF was initiated from mining excavation and construction, which was then used in many other underground and above ground construction. Particularly, after horizontal drilling and inclined drilling become possible, AGF gained significant attention in tunneling, open excavation, underpinning, gap filling, slope stabilization, cofferdam, and permafrost preservation (Harris, 1995; Jones et al., 1982; Thorburn and Littlejohn, 1992; Viggiani and Casini, 2015). In 1997, AGF was used with the assistance of horizontal drilling to build a tunnel of metro line in Beijing (Qiao et al., 2004). In that project, horizontal tubes were installed to create a vaulted portion to prevent the saturated running sands from collapsing. Li (2007) reported extra-long horizontal freezing of 140 m when building metro line in Guangzhou, China. A few years later, Su and Su (2014) reported an inclination angle of 14o of AGF in a coal mining application. The field success and advance in construction techniques have greatly promoted the usage of AGF. In addition to the advance in construction technology, there is a noticeable advance in materials. For example, non-metal pipes has been developed and used in AGF so they can be left in place when tunneling machine cutting through frozen soils (Zhou et al., 2022).
Alongside advancements in construction, extensive efforts have been made to understand the basic phenomena and fundamental theories underlying AGF. Initially, AGF practice was based primarily on freezing kinetics, including thermal conduction, diffusion, and phase change. However, it gradually became clear that AGF involves more complex physical processes, such as nucleation and crystal growth, pore water movement, and soil-water interactions. Shackelford (2000) described that the initiation of freezing begins with unstable clusters of water molecules when the temperature drops below the freezing point, Tf. For pure water, Tf is approximately −40°C according to (Fletcher, 1970). However, in natural environments, the presence of foreign surfaces, particles, and ions causes the freezing temperature, Tf, to be around 0°C. In soil, pore water is bound to surfaces of soil particles by chemical bonds and electrostatic forces, with bonding strength decreasing as the distance from the particle surface increases. Consequently, the freezing of pore water starts from the center of the pores and gradually progresses to soil particle surface (Lackner et al., 2005). Willams (1988) studied ice formation and the percentage of unfrozen water in total pore water at different temperatures, summarizing the results into non-linear relationships depending on soil types, as shown in Figure 1. The study found that only 1% of pore water in sand remains unfrozen when the temperature drops below 0°C, but about 5% of water in silt and 8%–20% in clay remains unfrozen at −5°C (Harris, 1995; Willams, 1988). These findings provide fundamental theories to design and analyze the thermal problems related to AGF (Harris, 1995; Lackner et al., 2005; Lackner et al., 2008).
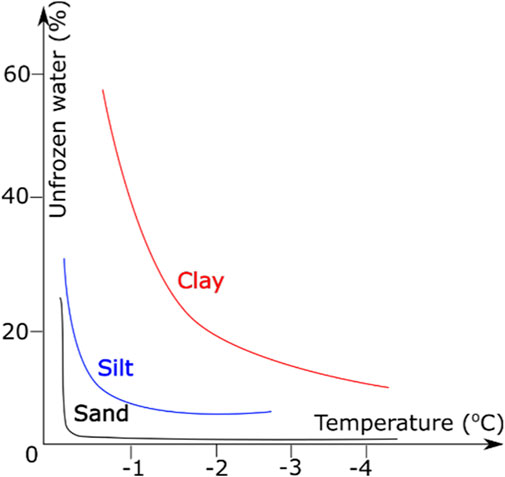
Figure 1. Unfrozen water content as a function of temperature for different types of soil [modified from Willams (1988)].
When supporting excavation, the strength of the formed soil-ice composite is a crucial design parameter. It has long been established that the strength of frozen soil is a function of temperature. Generally, compressive strength increases as temperature decreases, and clay soils are more affected by temperature changes than sandy soils (Haynes and Karalius, 1977; Shastri et al., 2021; Tsytovich, 1975). The creep behavior of frozen soil became a focus for engineers with the study of bearing capacity foundations on frozen ground (Parameswaran, 1987). Extensive analytical and experimental research has been conducted to understand this behavior (Fish, 1980; Ladanyi, 1972; Ladanyi, 1983). Early studies indicated that the creep behavior of frozen soil is similar to that of other materials like polymers and metals, with primary, secondary, and tertiary phases based on strain rate (Ladanyi, 1972; Zhu and Carbee, 1983). However, further research revealed that frozen soil might experience strength attenuation under loads, differentiating its creep behavior from other materials (Wang et al., 2019; Yao et al., 2017). Wang et al. (2019) proposed a binary model (Figure 2) to describe the creep behavior of frozen soil, accounting for both non-decay and decay scenarios. Considering strength decay significantly alters the secondary creep phase, impacting AGF applications.
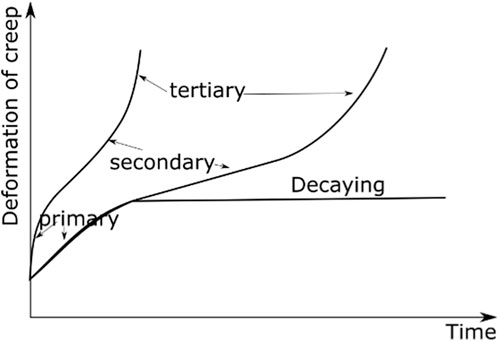
Figure 2. Binary model for frozen soil creep [modified from Wang et al. (2019)].
Other significant developments include thermal models accounting for high-velocity water flow, failure criteria of frozen soil considering creep and temperature, constitutive models for frozen soil, temperature distribution in frozen soil, ice lens and soil interaction, and the impact of ice formation on soil structure (Huang et al., 2018; Lackner et al., 2005; Lackner et al., 2008; Liang et al., 2022; Marwan et al., 2016; Vitel et al., 2016a; Vitel et al., 2016b; Xie et al., 2015; Zhao et al., 2023). Advancements in understanding over the past few decades have not only made designs more reliable and construction safer but also resulted in significant energy savings.
4 Conclusion
As AGF becomes more and more popular, it has been used in more and more applications; consequently, more challenges emerge (Harris, 1995; Zhou et al., 2022). Some of the challenges lie in lacking of a full understanding of the problem, for example, frozen soil behavior under cyclic or impact loads, and consolidation induced by soil frozen. Whereas others are due to the complexity of the site that makes uncertainty unknown (Liu et al., 2015; Zhou et al., 2022). Therefore, failure of AGF is not uncommon (Li et al., 2015; Zhou et al., 2022). Shuster (2000) reported more than one hundred problems that have plagued AGF projects, which are related to design, construction, installation, maintenance. Therefore, it can be concluded that AGF has become more mature nowaday but further studies are needed to improve this technology, particularly, improve the understanding of the uncertainty under complicated, large-scale projects.
Author contributions
HM: Conceptualization, Resources, Writing–original draft, Writing–review and editing. JH: Conceptualization, Writing–review and editing. DJ: Conceptualization, Writing–review and editing.
Funding
The author(s) declare that no financial support was received for the research, authorship, and/or publication of this article.
Conflict of interest
Author HM was employed by Changde Renhe Construction Co., Ltd.
The remaining authors declare that the research was conducted in the absence of any commercial or financial relationships that could be construed as a potential conflict of interest.
The author(s) declared that they were an editorial board member of Frontiers, at the time of submission. This had no impact on the peer review process and the final decision.
Publisher’s note
All claims expressed in this article are solely those of the authors and do not necessarily represent those of their affiliated organizations, or those of the publisher, the editors and the reviewers. Any product that may be evaluated in this article, or claim that may be made by its manufacturer, is not guaranteed or endorsed by the publisher.
References
Alzoubi, M. A., Xu, M., Hassani, F. P., Poncet, S., and Sasmito, A. P. (2020). Artificial ground freezing: a review of thermal and hydraulic aspects. Tunn. Undergr. Space Technol. 104, 103534. doi:10.1016/j.tust.2020.103534
Chang, D. K., and Lacy, H. S. (2008). “Artificial ground freezing in geotechnical engineering,” in International Conference on case Histories in geotechnical Engineering, Arlington, VA.
Fish, A. M. (1980). “Kinetic nature of the long-term strength of frozen soils,” in 2nd international symposium on ground freezing. Editors P. E. Frivik, N. Janbu, R. Saetersdal, and L. I. Finborud (Trondheim, Norway), 95–108.
Hashemi, H. T., and Sliepcevich, C. M. (1973). Effect of seepage stream on artificial soil freezing. J. Soil Mech. Found. Div. 99 (SM3), 267–289. doi:10.1061/jsfeaq.0001861
Haynes, F. D., and Karalius, J. A. (1977). Effect of temperature on the strength of frozen silt, 33. Hanover, New Hampshire: Cold Regions Research and Engineering Laboratory Hanover, New Hampshire.
Huang, S., Guo, Y., Liu, Y., Ke, L., Liu, G., and Chen, C. (2018). Study on the influence of water flow on temperature around freeze pipes and its distribution optimization during artificial ground freezing. Appl. Therm. Eng. 135, 435–445. doi:10.1016/j.applthermaleng.2018.02.090
International Atomic Energy Agency (2006). Remediation of sites with mixed contamination of radioactive and other hazardous substances. Vienna, Austria, 232.
Jessberger, H. L., and Jagow, R. (1988). “Thermal design of a frozen soil structure for stabilization of the soil on top of two parallel metro tunnels,” in 5th int. Symp. On ground freezing. Nottingham, Uk: Balkema, 349–356.
Jones, . J. S. (1982). State-of-the-Art report – engineering practice in artificial ground freezing. Dev. Geotechnical Eng. 28, 313–326. doi:10.1016/b978-0-444-42010-7.50034-7
Lackner, R., Amon, A., and Lagger, H. (2005). Artificial ground freezing of fully saturated soil: thermal problem. J. Eng. Mech. 131 (2), 211–220. doi:10.1061/(asce)0733-9399(2005)131:2(211)
Lackner, R., Pichler, C., and Kloiber, A. (2008). Artificial ground freezing of fully saturated soil: viscoelastic behavior. J. Eng. Mech. 134 (1), 1–11. doi:10.1061/(asce)0733-9399(2008)134:1(1)
Lacy, H. S., and Floess, C. H. (1988). Minimum requirements for temporary support with artificially frozen ground. Transp. Res. Rec. 1190, 46–56.
Ladanyi, B. (1972). An engineering theory of creep of frozen soils. Can. Geotech. J. 9 (1), 63–80. doi:10.1139/t72-005
Ladanyi, B. (1983). Shallow foundations on frozen soil: creep settlement. J. Geotech. Eng. 109 (11), 1434–1448. doi:10.1061/(asce)0733-9410(1983)109:11(1434)
Li, D. J. (2007). Extra-long horizontal freezing adopted in Guangzhou subway construction. Urban Rapid Rail Transit 20, 55–59.
Li, F. Z., Luo, F. R., and Han, Y. F. (2015). Cause analysis and countermeasure research for unclosed freezing wall of subway cross passage under complex conditions. Ind. Constr. 45, 187–190.
Liang, J., Shen, W., Lu, D., and Qi, J. (2022). A three-stage strength criterion for frozen soils. Cold Regions Sci. Technol. 201, 103597. doi:10.1016/j.coldregions.2022.103597
Liu, Y., Li, K.-Q., Dian-Qing Li, X.-S. T. S.-X. G., Tang, X. S., and Gu, S. X. (2015). Coupled thermal–hydraulic modeling of artificial ground freezing with uncertainties in pipe inclination and thermal conductivity. Acta Geotech. 17, 257–274. doi:10.1007/s11440-021-01221-w
Marwan, A., Zhou, M.-M., Abdelrehim, M. Z., and Meschke, G. (2016). Optimization of artificial ground freezing in tunneling in the presence of seepage flow. Comput. Geotechnics 75, 112–125. doi:10.1016/j.compgeo.2016.01.004
Parameswaran, V. R. (1987). Extended failure time in the creep of frozen soils. Mech. Mater. 6, 233–243. doi:10.1016/0167-6636(87)90014-7
Qiao, J., Tao, L., and Mo, S. (2004). Simulation study on deformation characteristics of ground surface in horizontal freezing construction of metro tunnel. Chin. J. Rock Mech. Eng. 23 (15), 2643–2646.
Russo, G., Corbo, A., Cavuoto, F., and Autuori, S. (2015). Artificial Ground Freezing to excavate a tunnel in sandy soil - measurements and back analysis. Tunn. Undergr. Space Technol. 50, 226–238. doi:10.1016/j.tust.2015.07.008
Sanger, F. J. (1968). Ground freezing in construction. J. Soil Mech. Found. Div. 94 (SM!), 131–158. doi:10.1061/jsfeaq.0001080
Schaefer, V. R., Berg, R. R., Collin, J. G., Christopher, B. R., DiMaggio, J. A., Filz, G. M., et al. (2017). Geotechnical engineering circular No. 13 ground modification methods - reference manual. Washington, DC: FHWA, 542.
Schäfers, P., and Hab, H. (2005). “Application of ground freezing for underground construction in soft ground,” in Proceedings of the 5th international conference of TC28 of the ISSMGEAmsterdam (Netherlands), 405–412.
Schmall, P. C., and Braun, B. (2006). “Ground freezing — a viable and versatile construction technique,” in 13th international conference on cold regions engineering 2006. Editors M. Davies, and J. E. Zufelt (Orono, Maine, USA: ASCE), 1–11.
Shastri, A., Sanchez, M., Gai, X., Lee, M. Y., and Dewers, T. (2021). Mechanical behavior of frozen soils: experimental investigation and numerical modeling. Comput. Geotechnics 138, 104361. doi:10.1016/j.compgeo.2021.104361
Shuster, J. A. (2000). “Ground freezing failures: causes and preventions,” in The international symposium on ground freezing and frost action in soils. Editor J. F. Thimus (Louvain-la-Neuve, Belgium: CRC Press), 6.
Su, W. D., and Su, H. B. (2014). Inclined shaft hole freezing construction technology. Coal Chem. Ind. 37 (6), 109–111.
Viggiani, G. M. B., and Casini, F. (2015). “Artificial Ground Freezing: from applications and case studies to fundamental research,” in The XVI ECSMGE geotechnical engineering for infrastructure and development. Editors M. G. Winter, D. M. Smith, P. J. L. Eldred, and D. G. Toll (Edinburgh, United Kingdom: ICE Publishing), 65–92.
Vitel, M., Rouabhi, A., Tijani, M., and Guérin, F. (2016a). Modeling heat and mass transfer during ground freezing subjected to high seepage velocities. Comput. Geotechnics 73, 1–15. doi:10.1016/j.compgeo.2015.11.014
Vitel, M., Rouabhi, A., Tijani, M., and Guérin, F. (2016b). Thermo-hydraulic modeling of artificial ground freezing: application to an underground mine in fractured sandstone. Comput. Geotechnics 75, 80–92. doi:10.1016/j.compgeo.2016.01.024
Wang, P., Liu, E., Song, B., Liu, X., Zhang, G., and Zhang, D. (2019). Binary medium creep constitutive model for frozen soils based on homogenization theory. Cold Regions Sci. Technol. 162, 35–42. doi:10.1016/j.coldregions.2019.03.019
Willams, P. (1988). “Thermodynamic of mechanical conditions within frozen soil and their effects,” in 5th int. Conf. On PermafrostTrondheim (Norway), 493–498.
Wu, T., Zhou, X., Liu, S., and Zhang, L. (2020). Longitudinal temperature measurement for precise positioning of weak point of frozen wall. Coal Eng. 52, 51–54.
Xie, S.-b., Qu, J.-j., Lai, Y.-m., Zhou, Z.-w., and Xu, X.-t. (2015). Effects of freeze-thaw cycles on soil mechanical and physical properties in the Qinghai-Tibet Plateau. J. Mt. Sci. 12, 999–1009. doi:10.1007/s11629-014-3384-7
Yao, X., Qi, J., Liu, M., and Yu, F. (2017). A frozen soil creep model with strength attenuation. Acta Geotech. 12, 1385–1393. doi:10.1007/s11440-017-0554-0
Zhang, Y., Liu, Z., and Duan, B. (2012). “Achievements of China’s coal mine construction in recent years,” in 3rd international conference on shaft design and construction (London), 127–130.
Zhao, Y., Zhang, M., and Gao, J. (2023). Research progress of constitutive models of frozen soils: a review. Cold Regions Sci. Technol. 206, 103720. doi:10.1016/j.coldregions.2022.103720
Zhou, J., and Tang, Y. (2018). Practical model of deformation prediction in soft clay after artificial ground freezing under subway low-level cyclic loading. Tunn. Undergr. Space Technol. incorporating Trenchless Technol. Res. 76, 30–42. doi:10.1016/j.tust.2018.03.003
Zhou, X.-m., Jiang, G., Li, F., Gao, W., Han, Y., Wu, T., et al. (2022). Comprehensive review of artificial ground freezing applications to urban tunnel and underground space engineering in China in the last 20 years. J. Cold Regions Eng. 36 (3), 04022002. doi:10.1061/(asce)cr.1943-5495.0000273
Zhou, X. M., Su, L. F., Yunli, Z., Binzhuang, X., and Genda, L. (1997). “Application of ground freezing method to brace a foundation pit,” in The int. Symp. On ground Freezing and frost Action in soils (Rotterdam, Netherlands), 537–540.
Keywords: artificial ground freezing (AGF), soil improvement, excavation, underground construction, tunnel
Citation: Mao H, Huang J and Johnson DW (2024) Artificial ground freezing for underground construction – a brief review of the theory, practice and challenge. Front. Built Environ. 10:1453407. doi: 10.3389/fbuil.2024.1453407
Received: 23 June 2024; Accepted: 30 September 2024;
Published: 14 October 2024.
Edited by:
Min Zhou, North University of China, ChinaReviewed by:
Xuefeng Yan, China University of Geosciences Wuhan, ChinaCopyright © 2024 Mao, Huang and Johnson. This is an open-access article distributed under the terms of the Creative Commons Attribution License (CC BY). The use, distribution or reproduction in other forums is permitted, provided the original author(s) and the copyright owner(s) are credited and that the original publication in this journal is cited, in accordance with accepted academic practice. No use, distribution or reproduction is permitted which does not comply with these terms.
*Correspondence: Huan Mao, aHVhbm1hbzE5OTAwOTE4QGhvdG1haWwuY29t