- 1Graduate School of Engineering, Osaka Metropolitan University, Osaka, Japan
- 2Japan Aerospace Exploration Agency, Tokyo, Japan
- 3IRIDeS, Tohoku University, Sendai, Japan
- 4Graduate School of Urban Environmental Sciences, Tokyo Metropolitan University, Hachioji, Japan
- 5Graduate School of Engineering, Kyoto University, Kyoto, Japan
- 6College of Engineering, Nihon University, Koriyama, Japan
- 7Faculty of Engineering, Tohoku Gakuin University, Sendai, Japan
- 8IRIDeS, Tohoku University, Sendai, Japan
- 9Graduate School of Agriculture, Kyoto University, Kyoto, Japan
- 10Institute of Engineering, Tribhuvan University, Lalitpur, Nepal
- 11Graduate School of Engineering, Kyoto University, Kyoto, Japan
- 12Faculty of Environmental and Urban Engineering, Kansai University, Suita, Osaka, Japan
This paper studied the feasibility of a sliding base isolation layer to be used in rubble stone masonry buildings in rural areas in the Himalayan Mountain range to provide robust protection to important building like schools against strong earthquakes. In this paper, we carried out on-site investigations, quasi-static tests, and shaking table tests focusing on constructability as well as isolation performance. From the results of the feasibility study, the combination of styrofoam, concrete slab, and grease was found to be the most feasible to be used as the upper element, the lower element, and the lubricant, respectively, in the sliding isolation layer. The key features of the present sliding base isolation layer are: 1) the use of the materials that exist in rural mountain areas or those that can be easily transported from the neighboring towns and cities and 2) ease of construction and minimal change from the current construction practice. From the shaking table tests, we identified the conditions, e.g., grease amount, grease type, normal stress at the interface, and roughness of concrete slab surface, to achieve dynamic friction coefficients ranging from 0.08 to 0.16, suitable for sliding base isolation.
1 Introduction
Rubble stone masonry is one of the most prevalent building types in rural areas in the Himalayan Mountain range. It is known that rubble stone masonry walls, shown in Figure 1A, are prone to strong earthquakes (Ali et al., 2013; Wang et al., 2018). In fact, a number of rubble stone masonry buildings experienced collapse or severe damage in the 2015 Nepal Gorkha earthquake (Goda et al., 2015; Wang et al., 2018). Even after the 2015 Nepal Gorkha earthquake, however, rubble stone masonry is often the only option available in the rural mountain areas (Bothara and Brzev, 2011; Schildkamp and Araki, 2019a; Schildkamp and Araki, 2019b). The main reason for using rubble stone masonry is the limitations of construction materials available in rural areas in the Himalayan Mountain range. The road access to such rural mountain areas is often narrow and bumpy as shown in Figure 1B. This makes it difficult to transport conventional pre-formed masonry elements like bricks and blocks from the nearby towns and cities because they may break during the transportation and because the transportation cost and time are high and long, respectively.
Considerable efforts have been made so far to enhance the seismic resistance of rubble stone masonry buildings. These efforts led to significant amount of literature on seismic design of rubble stone masonry buildings (Bothara and Brzev, 2011; Ali et al., 2013; Wang et al., 2018; Schildkamp and Araki, 2019a; Schildkamp and Araki, 2019b; Schildkamp et al., 2020; Schildkamp et al., 2021; Shrestha, et al., 2020; Parajuli, et al., 2020; Gautam et al., 2022; Khadka et al., 2023). While design and construction in accordance with such literature significantly reduce the risk of collapse or severe damage caused by strong earthquakes, uncertainty remains in their seismic resistance due to inherent variability in construction materials. Variability in skills of masons, who are often residents in rural mountain areas, is also a serious issue from the viewpoint of quality control.
It is well known that base isolation is an effective approach to protect buildings from strong earthquakes (Naeim and Kelly, 1999). Base isolation reduces seismic forces by placing an isolation layer at the interface between the superstructure and its foundation. Base isolation is attractive because it provides robust seismic protection to buildings. Base isolation is attractive also because no or minimal change is required in construction materials and processes of the superstructure. In the last decades, low-cost base isolation has attracted attentions in the earthquake engineering community to protect non-engineered buildings in low to middle-income countries and areas (Qamaruddin et al., 1986; Kelly, 2002; Nanda et al., 2016; Tsiavos et al., 2021a; Tsiavos et al., 2021b; Ali et al., 2022; Galano and Calabrese, 2023; Md at el., 2023). Although such low-cost base isolation is effective in many countries and areas, the materials used at the isolation layer are often locally unavailable in the rural areas in the Himalayan Mountain range, where rubble stone masonry is the most frequently constructed type of buildings. Transportation of such isolation layer materials from the nearby towns and cities is also difficult, if not impossible.
The purpose of this paper is to present a low-cost sliding base isolation for rubble stone masonry buildings in rural areas of the Himalayan Mountain range to protect important buildings like schools from strong earthquakes. To prevent severe damage of collapse, it is most important to follow the guidelines and recommendations (Bothara and Brzev, 2011; Ali et al., 2013; Wang et al., 2018; Schildkamp and Araki, 2019a; Schildkamp and Araki, 2019b; Schildkamp et al., 2020; Schildkamp et al., 2021; Khadka et al., 2023) to construct the superstructure of the rubble stone masonry building, wherein proper reinforcement and use of cement mortar are crucial. In addition to these prerequisites for the superstructure, this paper attempts to provide more robust seismic protection by inserting a sliding isolation layer at the interface between the superstructure and the foundation. The key features of the present low-cost sliding base isolation are: 1) the use of the materials that exist in rural mountain areas or those that can be easily transported from the neighboring towns and cities, and 2) ease of construction and minimal change from the current construction practice.
In this paper, first, we present a prototype building, to which the present sliding base isolation is applied. The design of the prototype building and the sliding isolation layer is based on the results of the on-site investigations in rural mountain areas in Nepal performed by the authors in December 2017. Second, quasi-static tests were performed to determine the reduction of the friction coefficient by using grease as a lubricant for several combinations of materials used in the sliding isolation layer. Third, shaking table tests were conducted to examine the performance of the present low-cost sliding isolation layer. Lastly, conclusions and recommendations for further study are provided.
2 Prototype building
Figure 2 illustrates a prototype school building to which the present low-cost sliding isolation is applied. We selected school as a prototype building because school buildings serve as a central facility to educate children, ensuring whose safety is of most importance, as well as a shelter for the community after strong earthquakes. The prototype school building was designed based on our on-site investigation conducted in December 2017 as well as the experience of designing and constructing 19 schools performed by Thapa and Schildkamp during 2007–2012 in rural mountain areas in the middle west Nepal (Schildkamp and Araki, 2019a; Schildkamp and Araki, 2019b). The mountainous area where Thapa and Schildkamp built the school belongs to the Alpine-Himalayan belt, one of the most earthquake-prone regions in the world. Earthquakes in this area are caused by the movement of the Indian Plate toward the Eurasian Plate (Schildkamp et al., 2020). In the on-site investigation, we scrutinized the damage of school buildings designed and constructed by Thapa and Shildkamp caused by the 2015 Nepal Gorkha Earthquake. Interviews with the residents were also conducted to collect the information on the conventional construction practice of school buildings in rural mountain areas and availability and transportability of construction materials. The results of the on-site investigation and the interviews with residents form the basis for developing the sliding isolation layer presented in this paper.
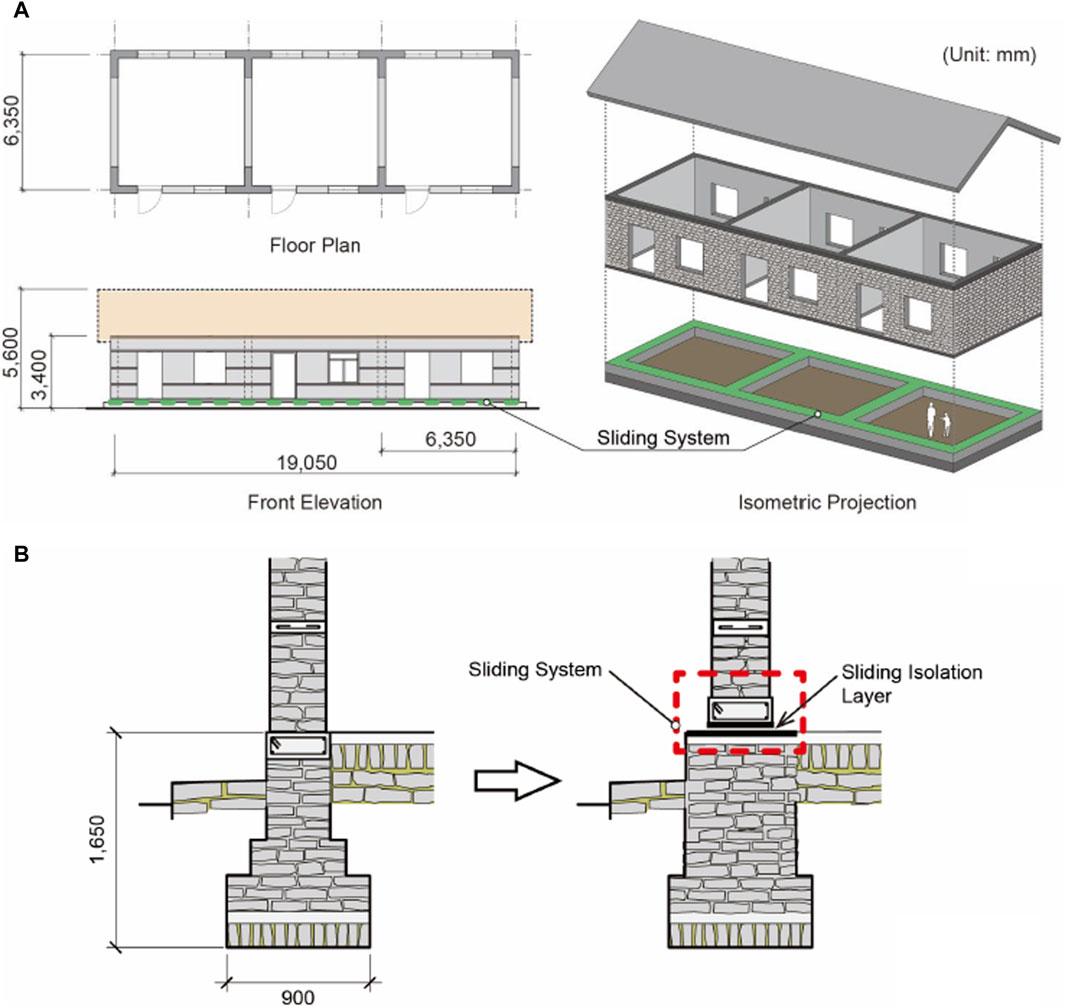
Figure 2. Design of the prototype school building: (A) whole view of the building and (B) the change of the details at the wall base to insert the present sliding isolation layer.
Conventional construction practice obtained through the interviews with the residents can be summarized as follows. Highly inaccessible terrain limits the transportation of construction materials and equipment. Stone masonry buildings built by residents are non-engineered. Large variations in the type and quality of construction exist, with no established norm for training the residents adequately. Furthermore, the stone masonry buildings use rubble stones rather than pre-formed block stones. The mortar in joints is made of mud or non-standardized aggregate, resulting in uncertain seismic strength. Such construction conditions pose serious difficulties in quantitative evaluation of seismic resistance of buildings.
To protect important buildings like schools, it is crucial to follow the guidelines and recommendations for rubble stone masonry buildings in the literature (Bothara and Brzev, 2011; Ali et al., 2013; Wang et al., 2018; Schildkamp and Araki, 2019a; Schildkamp and Araki, 2019b; Schildkamp et al., 2020; Schildkamp et al., 2021; Khadka et al., 2023), where the use of cement mortar and proper reinforcement are strongly recommended. In addition to these prerequisites for the superstructure, this paper attempts to provide more robust seismic protection to rubble stone masonry buildings by inserting a sliding isolation layer at the interface between the superstructure and the foundation as shown in Figures 2A, B. More specifically, the sliding isolation layer is placed at the interface between the reinforced concrete tie beam placed below the bottom end of the masonry wall and the concrete slab placed on the foundation. Large residual displacement induced by a strong ground motion is often a serious issue when sliding isolation is applied. Nevertheless, it was observed in our on-site investigation that rubble stone masonry building in rural mountain areas usually have enough space around the building to move, which allows us to use the building even after large residual displacement takes place. The present low-cost sliding base isolation layer incorporates the following two main features. Firstly, it utilizes materials found in rural mountain areas or readily transportable from nearby towns and cities. Secondly, it prioritizes ease of construction and requires minimal deviation from the conventional building practices.
Figure 3 shows the construction process of the sliding isolation layer. As shown in Figure 3A, concrete slab is placed on the foundation. An interface element with a lubricant is placed on the concrete slab. The interface element serves as the upper sliding surface as well as the bottom formwork for the reinforced concrete tie beam. After placing the side formworks as shown in Figure 3B, concrete is cast into the formwork. After removing the side formwork, rubble stone masonry wall is built on the reinforced concrete tie beam as shown in Figure 3C. It is worth nothing here that the cost increase of introducing this sliding system is roughly estimated as less than 5%–15% of the overall building cost. This estimation is based on the cost study by Schildkamp and Araki (Schildkamp and Araki, 2019b) and on our on-site investigations and interviews.
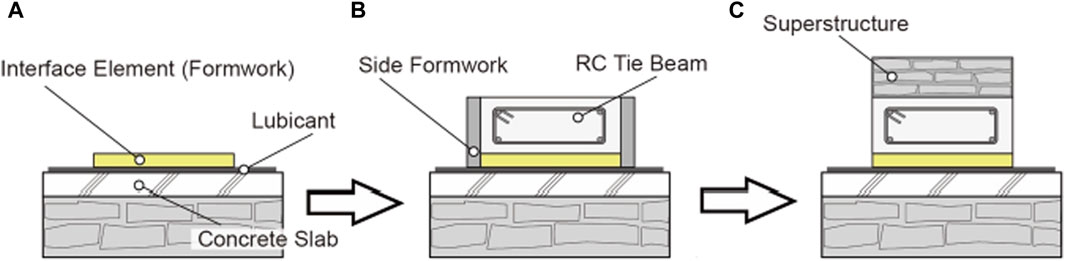
Figure 3. Construction process of the present sliding isolation layer: (A) concrete slab placing, (B) side formwork placing and concrete casting and (C) rubble stone masonry wall construction.
3 QUASI-STATIC tests
3.1 Materials and methods
Quasi-static tests were performed to determine the static friction coefficients of the sliding isolation layer for several combinations of materials used in the sliding isolation layer. Figure 4 shows the experimental setup. The test specimen consists of three portions: a) foundation (concrete slab fixed to the floor), b) sliding isolation layer, and c) superstructure (concrete blocks). During the tests, the lowest temperature was 7°C. Vertical load was applied to the sliding isolation layer by the weight of the superstructure. The weight of the superstructure was 3.05 kN and the vertical compressive stress at the sliding layer was 14.4 kN/m2. This value corresponds to about 1/3 of the vertical compressive stress (49.3 kN/m2) at the sliding layer of the prototype building discussed in Section 2. A block chain was used to apply the horizontal forced displacement to the superstructure manually. As shown in Figure 4, the block chain was fixed to the floor. The end of the steel chain was fixed to the lower portion of the superstructure. Monotonic forced displacement was applied by shortening the steel chain using the chain block. The tensile force applied to the superstructure was measured by the load cell placed at the end of the steel chain. The displacement of the superstructure relative to the concrete slab was measured by laser displacement transducers fixed to the concrete slab.
Styrofoam, marble, pebble stone, and concrete were used in the interface element that provides the upper surface of the sliding isolation layer. These materials are locally available in rural areas in the Himalayan Mountain range or can be transported easily from nearby towns or cities. The nominal material properties for the styrofoam (JIS A 9521 extruded polystyrene foam insulation) are as follows: density is more than 25 kg/m3, thermal conductivity is less than 0.034 W/m K, compressive strength is more than 20 N/cm2, and water absorption rate is less than 0.01 g/100 cm2. The marble used in this test was a serpentine green marble with smooth finish mined in the state of Rajasthan in the Northern India. The diameter of the pebble stones produced in the Philippines ranged from 40 to 60 mm. The design strength of the concrete block and the concrete slab was 24 N/mm2. The grease amount was 1 kg/m2. The nominal material properties of the grease (NIGURUBU MP-DX grease, Japan), called G_JA in this paper, are as follows: drip point is 200°C, viscosity at 25°C is 238, NLGI consistency number is 3, evaporation (99°C 22 h) is 0.20%, oil separate rate (100°C 24 h) is 0.20%, oxidative stability (99°C 100 h) is 25 kPa, water washout (79°C 1 h) is 1.6%, and thickener is lithium soap.
3.2 Results and discussions
Figures 5A, B illustrate the relationship between the horizontal force F and the displacement of the superstructure relative to the concrete slab without and with grease, respectively. The letters in the caption of Figure 5 represent the combination of the materials used at the sliding interface, where M, PE, S, C and G represent marble, pebble stone, styrofoam, concrete, and grease, respectively. As mentioned in Section 3.1, the value of the weight mg, where m is the mass and g is the gravity acceleration, of the superstructure was 3.05 kN. Table 1 summarizes the values of the static friction coefficient μs obtained from the quasi-static friction tests. Here, the static friction coefficient μs is defied by μs = Fmax/mg, where Fmax is the maximum value of F during the tests in for each combination of the materials used in the sliding isolation layer. If grease was not used, the static friction coefficient suitable for sliding isolation can be obtained only when marble was used as the interface material. Note here that 0.6 is often used as the design value for the static friction coefficient between concrete and concrete. In contrast, styrofoam and marble can be used as the interface material if grease was applied.
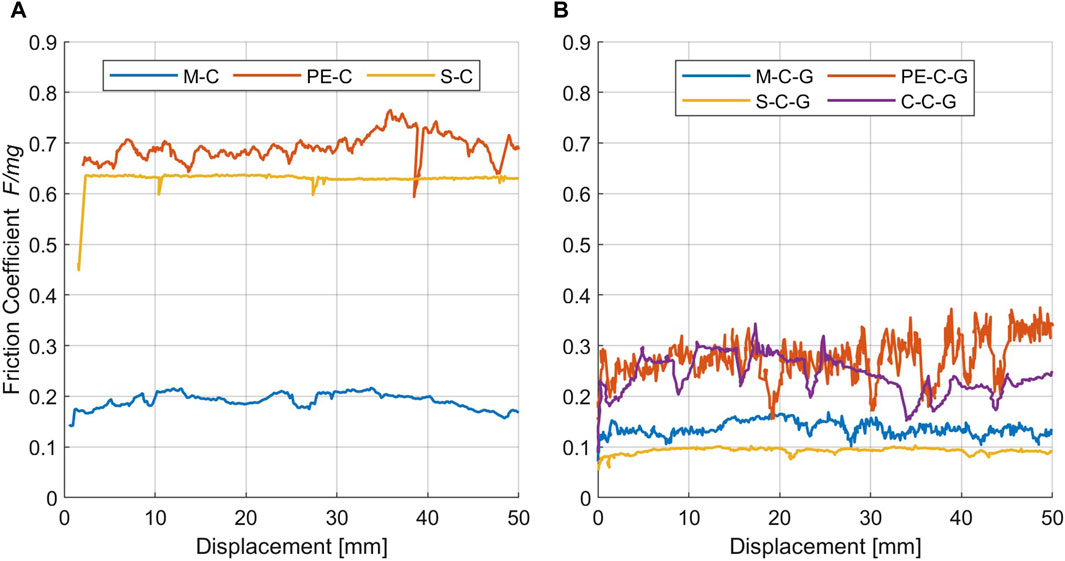
Figure 5. The relationships between the horizontal force and the horizontal displacement for (A) the isolation layer without grease, (B) the isolation layer with grease.
4 Shaking table tests
4.1 Materials and methods
From the quasi-static tests conducted in Section 3, we identified that the static friction coefficient was low enough to be used in sliding isolation layers in the following 2 cases: 1) styrofoam and concrete with grease and 2) marble and concrete with grease. As discussed in Section 2, light materials like styrofoam are highly desirable from the viewpoint of transportability from the nearby towns and cities to the rural mountain areas. In Section 4, therefore, a series of shaking table tests were conducted for the sliding layers consisting of the materials used in the former case, i.e., styrofoam and concrete with grease. In the shaking table tests, first, we determined a baseline condition and examined its isolation performance. Then, we studied the influences of the variations in the materials and loading conditions on the isolation performance.
Figure 6 shows the setup of the shaking table tests. Steel plates were used to change the weight of the superstructure. The superstructure was supported by two interface elements located at both ends of the superstructure. The concrete slab was fixed to the shaking table. Steel guide plates with Teflon sheets were placed at both sides of the superstructure to restrict the direction of motion in one direction. Input base motion was applied to this direction of motion using the shaking table. Accelerometers were placed on the superstructure and the concrete slab. The absolute displacements of the superstructure and the concrete slab were measured using the laser displacement transducers placed on the ground. The relative displacement between the superstructure and the concrete slab was obtained by subtracting these absolute displacements. The lowest temperature during the shaking table tests was 9 °C.
The baseline conditions for the shaking-table tests were determined from the results of the preliminary shaking table tests, the discussions made in Section 2 to design the prototype building, and the results of the quasi-static tests performed in Section 3. As discussed at the first of this section, the combination of the materials used for the interface element and the lubricant were styrofoam and grease, respectively. The baseline value of the grease amount was determined in the preliminary shaking table tests so that sufficient grease remained on the concrete slab surface after applying a couple of harmonic excitations. The results of the preliminary shaking table tests suggested the grease amount of 600 g/m2. For reference, the grease amount applied in the quasi-static tests was 1 kg/m2, which were determined by trial and error. The compressive stress at the sliding isolation layers was set to 53.4 kN/m2 by placing five steel plates to the superstructure. This value is slightly higher than 49.3 kN/m2, which corresponds the compressive stress at the sliding isolation layer of the prototype rubble stone building as discussed in Section 2. The same grease, whose details were given in Section 3, was used as the baseline material for the lubricant. A formwork with a smooth surface was used to cast the concrete slab to obtain very smooth surface at the upper side of the concrete slab. Harmonic excitations of 1.5 Hz were applied as the input base. The frequency of the harmonic excitations was determined from the results of the preliminary tests so that sliding of the specimen was stable up to 1 g excitation. In each case, the acceleration amplitude was increased with an increment of 0.5 m/s2 until the superstructure began to slide.
After conducting the shaking table test using the baseline condition, a series of the shaking table tests were conducted to investigate the influence of the following five factors on the isolation performance: a) the grease amount, b) the grease type, b) the vertical compressive stress, d) the surface conditions of the concrete slab, and e) the types of input base motions. To examine the influence of the grease amount, 5 cases of grease amounts (100 g/m2, 200 g/m2, 300 g/m2, 400 g/m2, 500 g/m2) were applied. To study the influence of the vertical compressive stress at the sliding isolation layer, the number of the steel plate was reduced from five to 3, which corresponds the compressive stresses 34.7 kN/m2, resulted in 35% reduction from the baseline value. To investigate the effect of the grease type, two additional types of grease, produced in India and widely available in Nepal, were used. The physical properties of the Indian greases, called G_IND1 and G_IND2 in this paper, were as follows: drip point was 240 °C and 180 °C, viscosity at 25 °C was 280 and 220–250, NLGI consistency number was 2.5 and 3, and thickener was lithium complex soap and lithium system, respectively. To study the influence of the variations in the roughness of the upper surface of the concrete slab caused by masons’ skills, a relatively rough surface was prepared using a wooden trowel as shown in Figure 7. To examine the influence of input motion, the 1940 EL Centro N–S record was employed as an earthquake ground motion. The ground motion record is most widely available and was selected to use in this paper to obtain the results that can be easily compared with the results of existing and future studies.
4.2 Results and discussions
Figure 8 shows the result of the shaking table test for the baseline specimen S_BL. From Figure 8A, reduction of acceleration can be clearly observed. Define the dynamic friction coefficient μD by μD = aR/g, where aR is the maximum absolute response acceleration of the superstructure and g is the gravitational acceleration. Then, the dynamic friction coefficient μD can be obtained as 0.08 from Figure 8A. The relative displacement of the superstructure to the shaking table accumulated in one direction as shown in Figure 8B. It is well known that such accumulation of relative displacement can be observed in dynamic friction responses under harmonic base excitations.
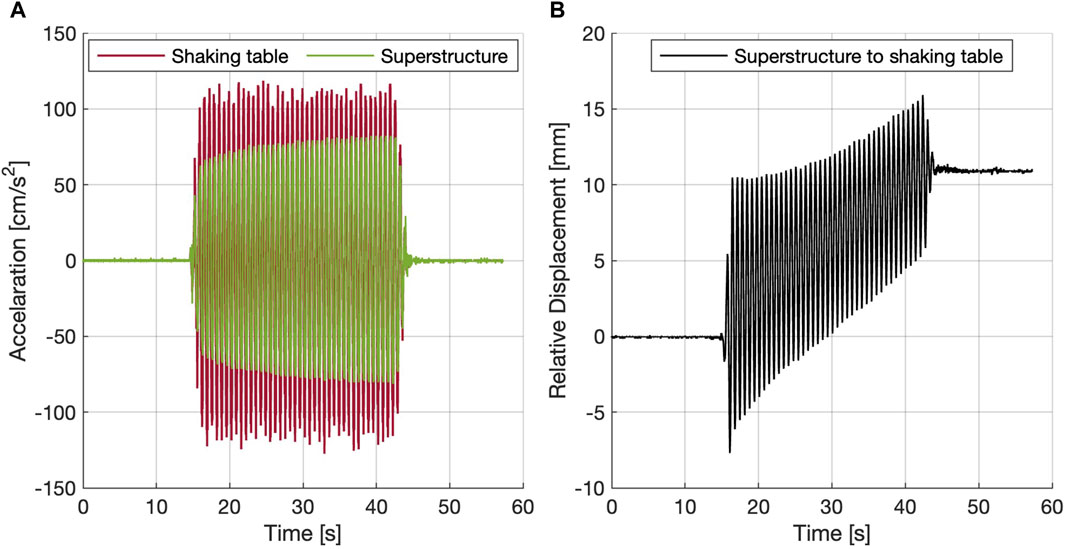
Figure 8. Time histores of the baseline specimen S_BL under a harmonig ground motion: (A) input and response accelerations and (B) response relative displacement.
Table 2 summarizes the dynamic friction coefficient μD obtained from the shaking table tests. The observations and discussions made for each specimen are as follows.
• All specimens: Basically, the acceleration and displacement responses of the specimens were similar to those of Specimen S_BL, the baseline specimen, if sliding took place under harmonic excitations.
• S_GA: Grease amount was changed. It can be identified that 300 g/m2 is the minimum grease amount to obtain enough isolation performance. Increasing the grease amount above this value did not enhance the isolation performance.
• S_GT: Grease type was changed. Relatively larger acceleration was observed for one Indian grease S_GT2 while enough isolation performance comparable to the baseline specimen S_BL was obtained for the other Indian grease S_GT1. It is difficult to explain the difference from the nominal physical properties like viscosity. This result suggests the importance of obtaining dynamic friction coefficients from physical tests before applying grease to practical applications.
• S_VS: Vertical stress at the sliding interface layer was changed. It can be seen that 35% reduction of the vertical stress did not change the isolation performance. This result demonstrated the robustness of the present sliding isolation layer against the variation of the weight of the superstructure.
• S_CSS: It can be observed that relatively rougher concrete slab surface did not change the isolation performance significantly. This suggests that the construction practice available in rural mountain areas can be applied to smoothen the upper side of the concrete slab without compromising isolation performance.
• S_IM: The specimen was subjected to an earthquake ground motion. It was observed that the dynamic coefficient was about 2 times higher than those obtained from the shaking table tests using harmonic excitations. Figures 9A,B show the time histories of the responses. One possible explanation for the larger dynamic coefficient is viscosity of grease. It appears that frequency components of the input motion higher than the frequency of the harmonic excitations resulted in higher frequency response, which led to the increase of the dynamic friction coefficient. It is known that the displacement response has a random nature when sliding isolation layer is inserted (Hu et al., 2020). These results suggest that shaking table tests using a variety of ground motion records and synthetic ground motions, including those with larger peak ground accelerations, are necessary for properly assessing the isolation performance of the sliding isolation layer using grease.
• S_IM_CSS: The specimen with the rougher concrete slab, the same concrete slab surface as S_CSS, was subjected to the same earthquake ground motion as S_IM. The following two observations, which were similar to S_IM and S_CSS, can be made: 1) The relatively rough concrete slab surface did not change the isolation performance significantly. 2) The dynamic coefficient was higher than those obtained from the shaking table tests using harmonic excitations. These results support the discussions made for both S_CSS and S_IM.
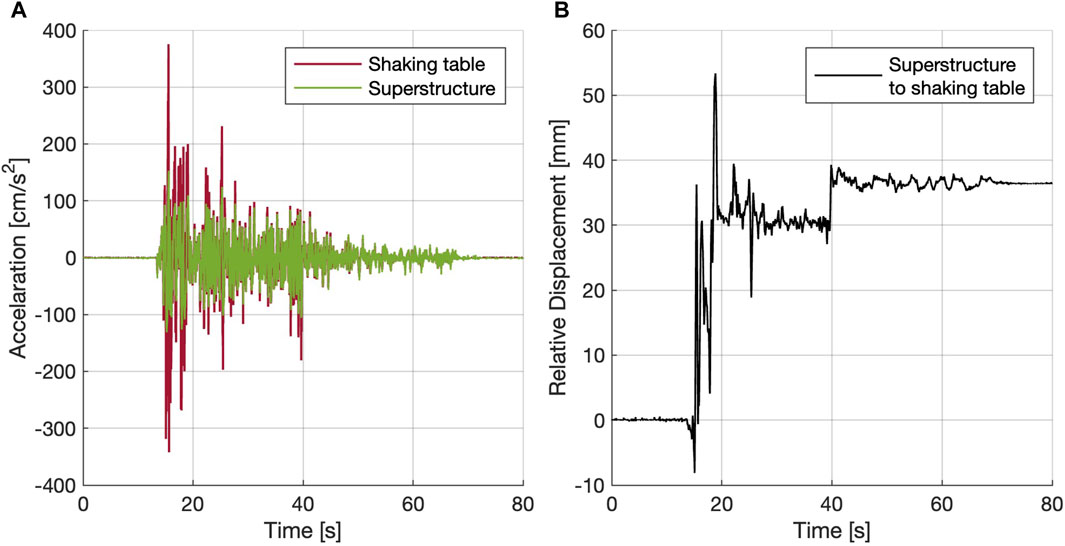
Figure 9. Time histores of the baseline specimen S_BL under an earthquake ground motion: (A) input and response accelerations and (B) response relative displacement.
5 Conclusion
This paper studied the feasibility of sliding isolation layers to be used in rubble stone masonry buildings in rural mountain areas in the Himalayan Mountain range to provide robust protection to important building like schools against strong earthquake ground motions. In the feasibility study, on-site investigations including interview survey to residents, quasi-static tests, and shaking table tests were carried out. From the feasibility study, the combination of styrofoam, concrete slab, and grease as the upper element, the lower element, and lubricant, respectively, was found to be the most feasible to be used in the sliding isolation layer. The key features of the present low-cost sliding base isolation are: 1) the use of materials that exist in rural mountain areas or those that can be easily transported from the neighboring towns and cities and 2) ease of construction and minimal change from the conventional construction practice. From the quasi-static and dynamic friction tests, we identified the conditions, e.g., grease amount, grease type, vertical stress, roughness of concrete slab surface, and input motions, to obtain dynamic friction coefficient ranging from 0.08 to 0.16 to be used in sliding isolation layer. It should be noted that the focus of this paper was placed only on the constructability and the isolation performance at the initial stage during the whole life cycle of the building. Among many factors, durability of grease is an important issue that needs to be addressed. Placing grease exclusively at closed spaces like the sliding interface is anticipated to mitigate degradation risks caused by factors such as exposure to ultraviolet rays from sunlight and contamination by dirt or debris. Investigation by the authors is underway on the long-term performance to address this issue.
Data availability statement
The raw data supporting the conclusions of this article will be made available by the authors, without undue reservation.
Author contributions
YS: Writing–original draft, Writing–review and editing, Investigation. MT: Data curation, Project administration, Writing–review and editing. RE: Data curation, Writing–review and editing, Methodology. JT: Methodology, Writing–review and editing, Conceptualization, Investigation, Project administration. YA: Investigation, Writing–review and editing, Funding acquisition, Writing–original draft. SP: Investigation, Writing–review and editing, Conceptualization, Methodology. SL: Conceptualization, Investigation, Methodology, Writing–review and editing. KI: Conceptualization, Investigation, Methodology, Writing–review and editing. JG: Methodology, Writing–review and editing, Data curation. KS: Methodology, Writing–review and editing, Investigation. IF: Methodology, Writing–review and editing, Data curation. TM: Methodology, Writing–review and editing, Conceptualization, Funding acquisition, Project administration, Supervision.
Funding
The author(s) declare that financial support was received for the research, authorship, and/or publication of this article. This on-site investigation was supported by Grants-in-aid for Scientific Research, Basic Research B (#17H04592), and the quasistatic and shaking table tests were supported by Grants-in-aid for Scientific Research, Fostering Joint International Research B (#20KK0103).
Acknowledgments
The authors are grateful to these financial supports. We would like to express our gratitude to all the students at Tohoku University, Tokyo Metropolitan University, Nihon University, Tohoku-Gakuin University, and Kansai University, who helped to conduct the experiments reported in this paper.
Conflict of interest
The authors declare that the research was conducted in the absence of any commercial or financial relationships that could be construed as a potential conflict of interest.
Publisher’s note
All claims expressed in this article are solely those of the authors and do not necessarily represent those of their affiliated organizations, or those of the publisher, the editors and the reviewers. Any product that may be evaluated in this article, or claim that may be made by its manufacturer, is not guaranteed or endorsed by the publisher.
References
Ali, A., Zhang, C., Bibi, T., Zhu, L., Cao, L., Li, C., et al. (2022). Investigation of five different low-cost locally available isolation layer materials used in sliding base isolation systems. Soil Dyn. Earthq. Eng. 154, 107127. doi:10.1016/j.soildyn.2021.107127
Ali, Q., Khan, A. N., Ashraf, M., Ahmed, A., Alam, B., Ahmad, N., et al. (2013). Seismic performance of stone masonry buildings used in the Himalayan belt. Earthq. Spectra 29 (4), 1159–1181. doi:10.1193/091711eqs228m
Bothara, J., and Brzev, S. (2011). A tutorial: Improving the seismic performance of stone masonry buildings. Oakland, California, USA: Earthquake Engineering Research Institute. Available at: https://www.world-housing.net/tutorials/stone-tutorials (Accessed March 19, 2024).
Galano, S., and Calabrese, A. (2023). State of the Art of seismic protection Technologies for non-engineered buildings (N-EBs) in developing regions of the World. J. Earthq. Eng. 27 (15), 4327–4353. doi:10.1080/13632469.2023.2165579
Gautam, D., Chettri, N., Tempa, K., Rodrigues, H., and Rupakhety, R. (2022). Seismic vulnerability of bhutanese vernacular stone masonry buildings: from damage observation to fragility analysis. Soil Dyn. Earthq. Eng. 160, 107351. doi:10.1016/j.soildyn.2022.107351
Goda, K., Kiyota, T., Pokhrel, R. M., Chiaro, G., Katagiri, T., Sharma, K., et al. (2015). The 2015 Gorkha Nepal earthquake: Insights from earthquake damage survey. Front. Built Environ. 1, 8. doi:10.3389/fbuil.2015.00008
Hu, H.-S., Lin, F., Gao, Y.-C., Guo, Z.-X., and Wang, C. (2020). Maximum superstructure response of sliding-base structures under earthquake excitation. J. Struct. Eng. 146 (7), 04020131. doi:10.1061/(ASCE)ST.1943-541X.0002682
Kelly, J. M. (2002). Seismic isolation systems for developing countries. Earthq. Spectra 18 (3), 385–406. doi:10.1193/1.1503339
Khadka, S. S., Acharya, S., Acharya, A., and Veletzos, M. J. (2023). Enhancement of Himalayan irregular stone masonry buildings for resilient seismic design. Front. Built Environ. 9, 1086008. doi:10.3389/fbuil.2023.1086008
Md, Z. N., Mohan, S. C., and Jyosyula, S. K. R. (2023). Development of low-cost base isolation technique using multi-criteria optimization and its application to masonry building. Soil Dyn. Earthq. Eng. 172, 108204. doi:10.1016/j.soildyn.2023.108024
Naeim, F., and Kelly, J. M. (1999). Design of seismic isolated Structures: from theory to practice. New York: John Wiley and Sons.
Nanda, R. P., Shrikhande, M., and Agarwal, P. (2016). Low-cost base-isolation system for seismic protection of rural buildings. Pract. Periodical Struct. Des. Constr. 21 (1), 04015001. doi:10.1061/(ASCE)SC.1943-5576.0000254
Parajuli, R. R., Furukawa, A., and Gautamu, D. (2020). Experimental characterization of monumental brick masonry in Nepal. Structures 33, 1314–1321. doi:10.1016/j.istruc.2020.09.065
Qamaruddin, M., Rasheeduzzafar, Arya, A. S., and Chandra, B. (1986). Seismic response of masonry buildings with sliding Substructure. J. Struct. Eng. 112 (9), 2001–2011. doi:10.1061/(ASCE)0733-9445(1986)112:9(2001)
Schildkamp, M., and Araki, Y. (2019a). Cost analysis of mountain schools in Nepal: Comparison of earthquake resistant features in rubble stone masonry vs. Concrete block masonry. Front. Built Environ. 5, 55. doi:10.3389/fbuil.2019.00055
Schildkamp, M., and Araki, Y. (2019b). School buildings in rubble stone masonry with cement mortar in seismic areas: literature review of seismic Codes, Technical norms and practical Manuals. Front. Built Environ. 5, 13. doi:10.3389/fbuil.2019.00013
Schildkamp, M., Silvestri, S., and Araki, Y. (2020). Rubble stone masonry buildings with cement mortar: design Specifications in seismic and masonry Codes Worldwide. Front. Built Environ. 6, 590520. doi:10.3389/fbuil.2020.590520
Schildkamp, M., Silvestri, S., and Araki, Y. (2021). Rubble stone masonry buildings with cement mortar: base shear seismic Demand Comparison for selected countries Worldwide. Front. Built Environ. 7, 647815. doi:10.3389/fbuil.2021.647815
Shrestha, J. K., Bhandari, S., Pradhan, S., and Gautam, D. (2020). Simplified frame model for capacity assessment of masonry buildings. Soil Dyn. Earthq. Eng. 131, 106056. doi:10.1016/j.soildyn.2020.106056
Tsiavos, A., Sextos, A., Stavridis, A., Dietz, M., Dihoru, L., and Alexander, N. A. (2021a). Experimental investigation of a highly efficient, low-cost PVC-Rollers Sandwich (PVC-RS) seismic isolation. Structures 33, 1590–1602. doi:10.1016/j.istruc.2021.05.040
Tsiavos, A., Sextos, A., Stavridis, A., Dietz, M., Dihoru, L., Di Michele, F., et al. (2021b). Low-cost hybrid design of masonry structures for developing countries: shaking table tests. Soil Dyn. Earthq. Eng. 146, 106675. doi:10.1016/j.soildyn.2021.106675
Keywords: rubble stone masonry, sliding base isolation, friction coefficient, constructability, transportability, Himalayan Mountain range, rural areas, shaking table tests
Citation: Suzuki Y, Tada M, Enokida R, Takagi J, Araki Y, Pareek S, Lee S, Ikago K, Guo J, Shrestha KC, Fukuda I and Masui T (2024) Feasibility of sliding base isolation for rubble stone masonry buildings in the Himalayan Mountain range. Front. Built Environ. 10:1432912. doi: 10.3389/fbuil.2024.1432912
Received: 14 May 2024; Accepted: 11 July 2024;
Published: 31 July 2024.
Edited by:
Dipendra Gautam, University of Iceland, IcelandReviewed by:
Rishi Ram Parajuli, Arup Group, United KingdomNimesh Chettri, Royal University of Bhutan, Bhutan
Copyright © 2024 Suzuki, Tada, Enokida, Takagi, Araki, Pareek, Lee, Ikago, Guo, Shrestha, Fukuda and Masui. This is an open-access article distributed under the terms of the Creative Commons Attribution License (CC BY). The use, distribution or reproduction in other forums is permitted, provided the original author(s) and the copyright owner(s) are credited and that the original publication in this journal is cited, in accordance with accepted academic practice. No use, distribution or reproduction is permitted which does not comply with these terms.
*Correspondence: Yusuke Suzuki, eS1zdXp1a2lAb211LmFjLmpw