- 1SMAGRA d.o.o., Zagreb, Croatia
- 2University of Zagreb, Faculty of Civil Engineering, Zagreb, Croatia
Analysing the entire project life cycle is a new trend in sustainability assessment in the construction sector. Research on sustainability issues in the construction sector started with issues related to buildings, while research for other structures such as infrastructure projects and bridges was not originally an integral part of the research. Therefore, the findings, knowledge and practical applications for buildings are more comprehensive than for infrastructure projects. This paper deals with the assessment of the sustainability of bridges throughout their life cycle and emphasises the importance of life cycle analysis of bridges in the early stages of planning and design. The study provides an overview of existing life cycle sustainability assessment (LCSA) methodologies for environmental (Life Cycle Assessment - LCA), economic (Life Cycle Cost - LCC) and social (Social - Life Cycle Assessment – S-LCA) aspects. This paper provides a critical overview of the current state of the art in the field of LCSA of bridges. To identify knowledge gaps, a review of the literature on LCSA of bridges, which performs a comparative life cycle analysis of different variants or components of the load-bearing structure of bridges is provided. The main objective of the paper is to provide recommendations for conducting LCSA of bridges and to identify research directions for design criteria for sustainable bridges of the future.
1 Introduction
Sustainable development according to its most famous definition: “Development that meets the needs of the present without compromising the ability of future generations to meet their own needs” (WCED, 2022), implies thinking about long-term circumstances, future generations and future demands, as well as the impact of today’s decisions and actions on the future. The issues of sustainable development and the impetus to develop its unique guidelines are motivated by the changes that have been taking place at the global level for several decades. Some of them have already expanded and we are feeling the consequences, while some changes and their consequences are only warned about because they are obvious by analysing their key indicators in the past and today, such are environmental changes. In addition to the changes that are already clearly noticeable, such as sudden urbanisation, the awareness of the finite nature of resources promoted by the industrial revolution, climate change, i.e., the impact of humans and their activities on the world around them, are central themes when it comes to thinking about current activities and their impact on the future. Reflections on the causes that have led to the above changes, and on their current and future consequences if not mitigated or prevented, have been at the centre of research directions in recent decades, guided by global strategic goals such as the Global Goals for Sustainable Development (Transforming our world, 2022). In the European Union there are some guidelines such as Political Guidelines for the next European Commission for the period 2019–2024 (Political Guidelines, 2019) and the established The European Green Deal (The European Green Deal, 2019). In the fight against climate change, the EU has also developed a strategy for a climate-resilient Europe - the new EU Strategy on Adaptation to Climate Change (Forging a climate-resilient Europe, 2021), and the framework for achieving climate neutrality, known as the European Climate Law (European Climate Law, 2018). There are also policy guidelines with an EU-wide assessment of National Energy And Climate Plans (An EU-wide assessment, 2020), European action for sustainability (Next steps for a sustainable European future, 2016) and A new Circular Economy Action Plan (A new Circular Economy, 2020).
This article presents the different stages of the life cycle according to the standardised stages for civil engineering works and emphasises the importance of the non-construction stages that precede them. It also presents different methods for assessing the sustainability of bridges during their life cycle or at a specific stage of the life cycle, taking into account the environmental (LCA), economic (LCC) and social (S-LCA) aspects. An overview of the literature on comparative life cycle analyses from a structural perspective of different bridge types and comparative analyses of different bridge elements is given, as well as a critical review of these analyses. An attempt is made to argue why, in line with the principles of sustainable development, it is necessary to analyse the entire life cycle of bridges in the planning and early design of new bridges, identifying the changing parameters and future requirements as well as the critical points by life cycle stage for sustainability outcomes. The paper tries to justify why due to a large number of parameters that depend on a specific case it is important to carry out an analysis of the different options and define the boundaries properly, as the choices made in planning and design can drastically steer the processes towards more sustainable approaches and determine the sustainability of the whole system during its lifetime. Furthermore, the paper aims to find a trade-off that can be identified in the comparative Life cycle sustainability assessment - LCSA of load-bearing elements of bridges, e.g., the trade-off between higher initial costs for high performance material or its greater environmental impact in the manufacturing process, but highest durability and lower maintenance needs during the service life. The literature review conducted in this paper, which compares different variants of load-bearing bridge structures during their life cycle using the LCSA method, aims to determine whether these gaps in knowledge can be identified using comparative LCSA and what they are. It also aims to identify uncertainties and key points that have the greatest impact on the sustainability of bridges, as well as possible research directions for the design of more sustainable bridges.
In the next chapter, the stages of the life cycle, i.e., the stages of bridges according to the corresponding standardized norm, are presented first. Then the existing methodologies for LCA, LCC and S-LCA are presented and discussed, corresponding standards for those that are standardized, examples of applicable software, databases and certain assessment methods that can be used in life cycle analysis. Finally, an overview of the literature on the comparative LCSA of load-bearing elements of bridges is given, followed by a critical review. Following the discussion and conclusion of the literature review, recommendations for conducting LCSA analyses of bridges and research directions for the design of sustainable bridges of the future are outlined.
2 Life cycle stages in life cycle analysis
Bridges are often projects of great strategic importance and financial necessity. As essential components of transport infrastructure, they play a key role in accessibility, have an impact on the lives of the local population and consume many natural resources, making them particularly important for efforts to find more sustainable solutions in the construction sector. The issue of sustainability generally requires consideration of the three fundamental pillars of sustainability, and discussions about achieving sustainability usually satisfy a holistic, integrated assessment of those three fundamental pillars of sustainability: environmental, economic and social. There are various life cycle assessments that aim to quantify and measure sustainability using a specific methodology and accurate data. Section 2.1 explains the life cycle of a product, taking into account the construction product and its environmental assessment procedures, documentation and life cycle stages according to the specific standard. The sustainability analysis in the construction sector needs to be much more comprehensive and consider the life cycle of a whole building or infrastructure project. In section 2.2, the life cycle stages of construction works are described according to the specific standard and the life cycle of bridges is polemized accordingly in section 2.3. Details and examples of LCSA methods, including LCA, LCC and S-LCA for sustainability assessment, are presented in Section 3.
2.1 Life cycle stages of products
The Environmental Product Declaration (EPD) is a document containing verified and comparable data on the environmental impact of a construction product during its life cycle. It is defined according to the HRN EN 15804:2019 standard (HRN EN 15804, 2019), and the life cycle stages of the product for LCA analysis is also defined according to this standard, as shown in Figure 1. The product life cycle is defined by four stages: A1–A3 product stage, A4–A5 construction process stage, B1–B7 use stage, C1–C4 end of life stage. Module D is also defined for additional product information beyond construction works life cycle. The aim is to obtain data on the environmental impact of products during their life cycle, based on clear guidelines, so that they can be compared in order to determine which products are more environmentally friendly, i.e., which have less negative impact on the environment.
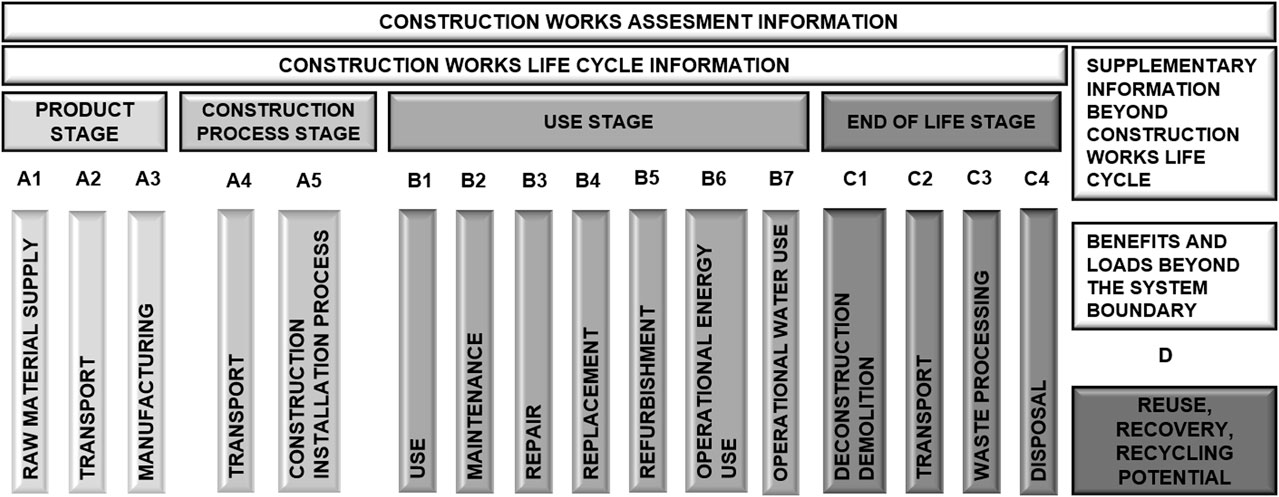
Figure 1. Stages of the product life cycle according to HRN EN 15804 (HRN EN 15804, 2019).
2.2 Life cycle stages of civil engineering works
The stages of the life cycle of civil engineering works are defined in HRN EN 17472:2022 (HRN EN 17472, 2022) and are shown in the diagram in Figure 2. There are different stages associated with the life cycle and construction of bridges, i.e., construction activities associated with the life cycle of bridges. The life cycle of civil engineering works assessment is divided into the following stages according to the standard: A0 - pre-construction stage related to land and associated fees, A1–A3 product stage, which is divided into raw material supply, transport and manufacturing, A4–A5 construction process stage, which includes transport and all processes of construction/installation of the facility. The next stage is the use stage, which is divided into eight sub-stages B1–B8, namely: use, maintenance, repairs, replacement, refurbishment, operational energy use, operational water use and user’s utilisation. The end of life stage C1–C4 is divided into the following sub-stages: deconstruction, transport, waste processing for reuse, recycling and/or energy recovery and the final sub-stage, disposal. Additional information beyond the life cycle and system boundaries, such as reuse, recycling, energy recovery or other recovery and exported utilities is also considered.
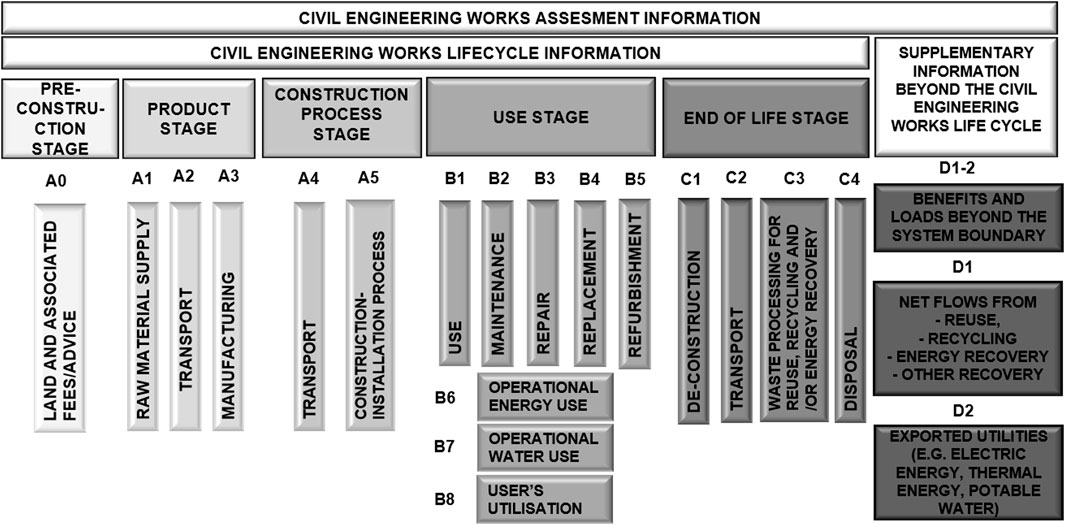
Figure 2. Stages of the life cycle of civil engineering works according to HRN EN 17472 (HRN EN 17472, 2022).
2.3 Life cycle stages of bridges
There are stages in the decision-making process that precede construction activities related to the building of new bridges. Bridges have a long lifespan and face the challenge of adapting to changing conditions on a global scale over the long term during their useful life. Challenges such as increased demands on the bridge in the form of a higher average number of vehicles on the road over a longer period of time during its service life and other challenges such as climate change.
Climate change may create new conditions in terms of different pressures on the bridge from the environment, more extreme weather conditions e.g., more frequent and intense exceptional, short-term pressures. The paper (Nasr et al., 2019) analyses the potential impact of climate change on bridges and identifies a total of 31 risks, which are divided into seven main categories: durability, serviceability, geotechnical risks, increased demand, accidental loads, extreme natural events and operational risks. It is also emphasised that the increase in precipitation and the rise in temperature each contribute to 18 potential risks and together can influence 25 of the 31 risks investigated (Nasr et al., 2019). The article (Orcesi et al., 2022) examines the effect of climate change on possible future extreme environmental actions on structures, such as wind, hurricanes, snow, sea level rise and flooding and discusses the same key topics in three different continental regions: Europe, North America and Asia. Due to the long service life, the deterioration of the mechanical properties and seismic performance of structural elements under the above-mentioned loads and environmental stresses, which are exacerbated by the climatic changes, must be analysed during the design process. Paper (Cui et al., 2022) proposed a time-dependent deterioration model and investigates seismic fragility of bridges RC columns subjected to the combined action of freeze–thaw cycles and chloride-induced corrosion and the results show that the time-dependent seismic fragility shows a non-linear increase with increasing lifetime under different damage conditions. Based on all these parameters, the long-term adaptability of bridges must be analysed in the preliminary design process on the basis of their specific location, function, traffic requirements, current and future climatic influences and their effects on the load-bearing structure.
Various rating systems for evaluating the sustainability of infrastructure projects, such as BREAAM infrastructure (BREEAM, 2022), Envision (Envision, 2018) and SuRe (SuRe, 2021) place the great emphasis on the strategic planning and project strategy development phases. Accordingly, in addition to the construction stages associated with the construction works, which are quantified through life cycle analyses, the preceding non-construction stages are also crucial to obtain the most optimal solution for a given design task. The non-construction stages of the life cycle can be divided into the planning stage, the study/analysis stage and the design stage. In the planning stage, the framework is set out and the project task defined (Figure 3). The functional and economic framework of the project task is defined, the purpose of the bridge (pedestrian, road or railway bridge) is stated, as well as the expected service life and the average annual number of vehicles if it is a road bridge. In this stage, the financial scope of the project itself and the estimated costs for annual maintenance during the useful life of the bridge are defined. When defining the project task, the necessary architectural and structural parameters for the design must also be established.
According to the criteria for the most sustainable bridge and the best solution for the given project task, the study/analysis stage should precede the selection of the most optimal solution. In line with the trend to promote sustainable bridges of the future, it is necessary to include a study/analysis stage prior to the design stage of a specific bridge according to the defined conditions of the project task and to carry out a life cycle sustainability assessment of the alternative variants of bridge solutions. In the study/analysis stage (Figure 4), preliminary solutions should be developed, different materials, structural systems and bridge types considered, possible long-term requirements for the bridge taken into account and several solution variants defined. This is followed by a life cycle sustainability assessment of the solution variants, which includes a combination of LCA, LCC, and S-LCA, and additional efforts on their optimisation in order to reach a consensus between all three components of the LCSA analysis and identify the optimal bridge variant.
In the design stage, the chosen solution variant is worked out in detail and the main projects of all technical professions involved are drawn up. After the building permit has been granted, the detailed design projects are drawn up. The initial criterion in decision-making for bridge projects is often the economic criterion, but we should aim to design sustainable bridges as a future goal by analysing their whole life cycle.
3 Overview of LCSA methodology
In the second generation Eurocode edition from 2023 (HRN EN 1990:2023) (HRN EN 1990, 2023), the “inclusion of provisions on sustainability” has been added compared to the previous version and is defined as: “the structure should be designed to limit its adverse impact on non-renewable environmental resources, on society, and on economy during its entire lifecycle.” There are numerous scientific studies in the literature that have developed various methods for assessing the life cycle of structures with regard to environmental, economic and social aspects. Reviewing the literature, it is clear that various researchers are conducting LCA, LCC and S-LCA for sustainability assessment (Figure 5).
3.1 Life cycle assessment - LCA
Sustainability issues began with questions about the environmental impacts of all human activities and operations. The first LCA analyses, at that time not yet under that name, were carried out around 1970 (van der Meer and Leal Filho, 2019). Life cycle assessment is a method for quantifying resource consumption, the release of harmful emissions and all other environmental impacts that occur during the life cycle of a product, process and/or project. The LCA methodology is a standardised ISO standard and the first ISO standard for LCA was published in 1996. The LCA methodology is standardised by ISO 14040 (HRN EN ISO 14040:2008) (HRN EN ISO 14040, 2008), which describes the principles and framework (Figure 6), and ISO 14044 (HRN EN ISO 14044:2008 + A1:2018 + A2:2020), which contains requirements and guidelines (HRN EN ISO 14044, 2008). Both standards must be followed if LCA is carried according to ISO standards. According to ISO 14040 (HRN EN ISO 14040, 2008) LCA: “addresses the environmental aspects and potential environmental impacts (e.g., use of resources and the environmental consequences of releases) throughout a product’s life cycle from raw material acquisition through production, use, end-of-life treatment, recycling and final disposal (i.e., cradle-to-grave).”
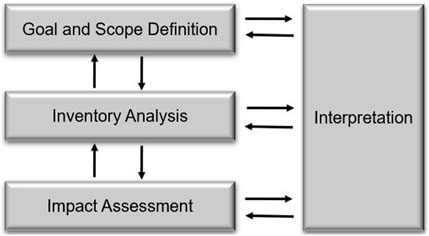
Figure 6. Life cycle assessment - LCA according to HRN EN ISO 14040 (HRN EN ISO 14040, 2008).
There are a number of papers that provide a general framework and discuss LCA analysis according to these standards. The article (Soukka et al., 2020) provides a brief overview of the LCA method in accordance with these ISO standards and discusses its general justification for use in practise.
According to the ISO standardisation, LCA comprises four phases: a) the goal and scope definition phase, b) the life cycle inventory analysis phase, c) the life cycle impact assessment phase, and d) the interpretation phase. The Life Cycle Inventory (LCI) phase is the second phase of the LCA analysis. It records the input/output data of the system to be analysed, collects data to calculate the emissions of harmful gases to air, soil and water, the energy and raw materials consumed and other related environmental impacts in each process within the boundaries of the system defined in the first phase. The third phase is the Life Cycle Impact Assessment (LCIA) phase, in which all environmental impacts collected from the LCI database are classified into impact categories. For example, all components that cause specific environmental impact are classified in one impact category in the third phase (Life Cycle Impact Assessment - LCIA), although different substances from different processes in the second phase (Life Cycle Inventory - LCI) may be responsible for the effect. The LCIA phase consists of several steps, the first three of which are mandatory under ISO 14040, while the other three are not. The first stage of the LCIA is the selection of impact categories, category indicators and characterisation models.
The second stage is classification, where LCI results are assigned to the selected impact categories, while the third stage is characterisation, where category indicator results are calculated. In characterisation, LCI results are converted into common units and classified into the same impact categories. The three optional stages of LCIA are normalisation, grouping and weighting. In the interpretation stage, the results obtained are evaluated.
The ILCD gives recommendations for the use of a specific LCIA method in the European context for a specific impact category (ILCD, 2011). Not all LCIA methods contain all of the listed impact categories. Some of the LCIA methods are: EDIP2003 (Hauschild and Potting, 2005), Eco-indicator 99 (Goedkoop and Spriensma, 2001), ReCiPe (Huijbregts et al., 2017), IMPACT World + (Bulle et al., 2019), USEtox (Huijbregts et al., 2015), EPS (Steen, 2015), TRACI 2.0 (Bare, 2011), Ecological Scarcity (Frischknecht et al., 2021). In the LCIA phase, often there are two approaches: midpoint and endpoint. The endpoint approach has an additional step where the midpoint impact categories are grouped and classified into endpoint areas that represent direct environmental and human impacts. Ecoinvent is one of the most representative certified eco-inventory databases in the world. Various software programs have been developed to carry out LCA analyses. Most of them have implemented databases, LCIA methods and all the necessary steps according to the general steps for carrying out the LCA methodology according to the ISO standards. The best known and most frequently used software programs for carrying out LCA analyses are SimaPRO (Goedkoop et al., 2016; SimaPro Tutorial, 2023), GaBi (Kupfer et al., 2021), OpenLCA (Tutorial, 2020) and Umberto (Umberto, 2018). The article (Aparecido Lopes et al., 2017) explains the differences between the four leading software tools as follows: GaBi, openLCA, SimaPro and Umberto NXT for performing LCAs and analyses their ease of use, modelling principles, hotspots and included databases, compares different LCA results in terms of performing analyses with different software tools and concludes that LCA results may change depending on the software used.
3.2 Life cycle cost – LCC
Life cycle costing is generally standardized with HRN ISO 15686-5:2023 (HRN ISO 15686-5, 2023). This standard aims to define a methodology and provide requirements for performing and quantifying life cycle cost analysis for buildings and constructed assets.
According to this standard Whole life cost (WLC) consist of externalities, non-construction cost, life cycle cost (LCC) and income. The life cycle costs are divided into construction, operation, maintenance and end of life. The operating phase is further subdivided into environmental costs. The difference between the WLC and LCC analysis is that the WLC may include in the analysis a broader range of externalities or non-construction costs for a given building, such as costs related to environmental impacts, social costs and benefits, contribution of the construction works to sustainability and sustainable development, intangibles – impact on business reputation, functional efficiency, future income streams, financing costs. Some other member states of the European Union have adopted the ISO 15686-5 standard, for example, Netherlands - NEN-ISO 15686-5:2017 (NEN-ISO 15686-5, 2017). United Kingdom has also adopted this ISO standard as BS ISO 15686-5:2017 (NEN-ISO 15686-5, 2017).
JRC Technical reports (Dodd et al., 2021) Level(s) Indicator 6.1: Life cycle costs, along the ISO 15686-6 standard for the calculation of LCC, refers to the standards EN 15459-1 (HRN EN 15459-1, 2017; HRI CEN/TR 15459-2, 2017) and EN 16627 (HRN EN 16627, 2015), but these standards are specified for buildings.
In the second generations Eurocode 0 (HRN EN 1990, 2023) costs can be taken into account when determining reliability requirements in the following way: “the service life cost optimal target reliability requirements depend on the expected failure consequences and on all cost associated with the design, operation, inspection, maintenance and renewal of structures over the time period for which they are needed.”
These standards are not specified for bridges, but can be a framework for all types of costs related to life cycle cost analysis. In addition, various road and bridge operators have their own manuals for conducting LCC (Soliman et al., 2019; Leiva Maldonado and Bowman, 2019; Leroy Hulsey et al., 2022; NCHRP REPORT 483, 2003). However, these manuals can only serve as a guide or are primarily intended for internal use.
3.2.1 Life cycle cost of bridges
Analysing the life cycle costs of bridges is becoming increasingly important in efforts to design sustainable bridges. The lowest initial cost of the project is not the right way to design sustainable bridges, because often the lowest cost of the project is also the one that requires the most maintenance, which in turn hinders the free movement of traffic on the bridge and thus has a negative impact on the environmental, economic and social aspects of sustainability.
In the use stage of the life cycle of bridges, we distinguish between the phase of free movement of vehicles and the maintenance phase, in which the bridges are mostly closed to traffic. The strategies for inspecting and maintaining bridges can vary from country to country and depend on climatic conditions, the type of bridge and the economic resources available. The main purpose of bridges is their functionality. Accordingly, functional restrictions due to maintenance or repair work should be minimised during the lifetime of the bridge (Maier et al., 2014). In life cycle cost analysis most authors consider the management costs and the user costs in the life cycle costs of bridges. Management costs consist of the bridge owner’s costs and include the following costs: initial costs, construction costs, maintenance, repair and rehabilitation costs, deck overlay replacement and deck replacement cost, and the cost of demolishing the bridge, while user costs include the cost of travel delays (TDC), vehicle operating costs (VOC) and accident costs (AC) (Mashhadia et al., 2021). Indeed, traffic congestion may occur due to maintenance work, and user costs are caused by congestion and higher fuel consumption or diversion journeys, which are often longer. The cost of traffic delays or user costs can be illustrated by the example of the I-35 W bridge collapse in Minneapolis, Minnesota, on 1 August 2007. The Minnesota Department of Transportation (DOT) estimated the user costs due to the bridge failure at $400,000 per day and the total costs for the 413-day period that the I-35 W bridge was out of service at $16,520,000 (Freyermuth, 2009).
Gervaiso emphasizes noise, esthetics and user costs as social problems and classifies user costs in S-LCA as driver delay costs, vehicle operating costs and accident costs (Gervasio, 2010). According to the paper (Zhao et al., 2010), life cycle costs include both agency and user costs as well as vulnerability costs, as the bridge is often exposed to exceptional circumstances and the resulting damage could be avoided if the bridge was designed, built or maintained to avoid the potential hazard. Damage can be caused by earthquakes, floods, collisions, washouts, i.e., exceptional events and intensities for which the bridge was not designed. According to the proposed methodology for a bridge in operation (Zhanli et al., 2021), the costs in the construction phase account for about 60% of the total life cycle costs, while the costs during use stage account for almost 40% of the total life cycle costs.
The question of durability is crucial when it comes to the longevity of bridges for which they were designed. Motorway bridges built in the 1960s and 1970s were designed to last 120 years. However, after 20–40 years, the first signs of deterioration began to appear, leading to many discussions on the issue of initial cost versus total life cycle cost (Long et al., 2008). It is therefore important to note that although a sustainable bridge may have a high initial cost compared to conventional bridges, the total life cycle cost of sustainable bridges is lower due to the low maintenance requirements and long service life. For example, the initial construction costs of a bridge reinforced with carbon fiber-reinforced polymer - CFRP are higher than those of steel-reinforced bridges. However, when the first major refurbishment of the bridge deck is due, the total cost of the steel reinforced concrete bridge then exceeds the cost of the CFRP-reinforced bridge (Grace et al., 2012). It is important to emphasize that due to the long life of bridges, it is necessary to take discount rate into account when comparing the costs for the different phases of the life cycle of bridges. The discount rate must be taken into account when analysing the total costs for the entire life cycle of bridges. The sensitivity analysis of the life cycle approach, which considers the economic and environmental impacts in the railroad sector when comparing bitumen-stabilized ballast and conventional ballast, shows that the discount rate is the most critical parameter due to its uncertainty and difficult predictability (Giunta et al., 2018).
3.3 Social - life cycle assessment– S-LCA
S-LCA is a methodology for assessing the social impact of products and services throughout their life cycle. The assessment of the social aspect of sustainability is the least represented in sustainability research in the infrastructure sector, which is due to the complexity of interpreting the impacts and the way they are quantified.
A study (Huertas-Valdivia et al., 2020), which analysed the literature on S-LCA published in the last 15 years (2003–2018) using bibliometric methods, shows that S-LCA research has increased significantly, especially after the publication of the UNEP/SETAC Guidelines/for Social Life Cycle Assessment of Products in 2009, and that 66% of all articles published for S-LCA were published in the period 2015–2018. The revision of the Guidance for the Social Life Cycle Assessment of Products (UNEP et al., 2020) and Organisations was published by United Nations Environment Programme (UNEP) in 2020, and revision of the Methodological Sheets for Subcategories in Social Life Cycle Assessment (S-LCA) (UNEP et al., 2021) in 2021. UNEP published Pilot projects on Guidelines for Social life cycle assessment (Traverso et al., 2022) of products and organizations in 2022. This document presents 40 methodological sheets categorised into six stakeholder categories: workers, local communities, value chain actors, consumers, society and children. Children is the new category introduced by the UNEP 2020.
In (Sierra et al., 2018), 94 works that include social aspects in the multi-criteria evaluation of infrastructures are reviewed and analysed. On this basis, 23 criteria for the social aspect of the sustainability of infrastructure projects are identified, of which the criteria economy and local development, mobility and accessibility, health and environmental safety are the most frequently considered.
When analysing life cycle of bridges, user costs are often examined from a social perspective. For example, when analysing the social impact, paper (Gervasio and Simões da Silva, 2012) considers the user costs during the use stage of the bridge, which may be caused by congestion, traffic accidents or renovation or maintenance work on the bridge, taking into account three types of user costs: vehicle operation costs, driver delay costs and accidents costs. In (Wu et al., 2021), on the other hand, models for calculating user costs are presented and demonstrated using two examples, namely, the construction of residential buildings and the maintenance of bridges, whereby the user costs for the construction of residential buildings amount to less than 7% of the project budget, while the user costs for the maintenance of bridges can amount to twice the direct costs. In addition to user costs (costs for traffic accidents, lost time and additional operating costs), the costs of construction workers (costs for medical assistance, loss of income due to death or disability and pain and suffering of injured workers), local communities (loss of income, loss of productivity due to noise, loss of property value due to noise) and public service providers (administrative costs for accidents, loss of parking revenue) are also taken into account (Wu et al., 2021). In addition, paper (Navarro et al., 2018a) assesses the social impacts of 15 different concrete bridge slabs with preventive maintenance strategies in the marine environment using social impact categories. The social aspects are assessed based on four impact groups: workers, society, users, local community, which can be assigned different weighting factors when assessing the social impacts of sustainability. It has been shown that the social impacts resulting from maintenance play an important role in the sustainability of bridges.
There are databases and methods that can be used for social impacts assessment in different software. Product Social Impact Life Cycle Assessment - PSILCA database (PSILCA et al., 2023) and Social Hotspot Database - SHDB (Benoit-Norris et al., 2012) are a comprehensive social impact assessment databases. SOCA database combines LCA, LCC and S-LCA allowing to carry out full LCSA.
3.4 Discussion of the LCSA methodology for bridges
LCSA is an approach that can be used as a method for sustainability assessment by considering environmental, economic and social aspects in different processes, products and industries. The paper (Isa Olalekan et al., 2023) gives a general, comprehensive framework for the LCSA approach, the challenges involved, gives an overview of software tools and databases, presents applications in different industries and sectors and emphasizes the purpose of LCSA analysis and sustainability challenges of processes or systems.
In the previous chapters, the LCSA methods and their application in the infrastructure sector were described using examples of methods that have been used in various scientific papers.
Although LCA analysis is the standardized method, the uncertainties and lack of well-specified methods that provide a basis for the infrastructure sector are perceived as a disadvantage. This is a particular problem when different LCA results are compared with each other. According to the paper (den Heede and De Belie, 2012), small differences in the way an LCA analysis is carried out can lead to large differences in the assessment of environmental impacts in the interpretation phase, such as: the choice of functional unit, the inventory data used and the choice of impact assessment method.
A Functional Unit (FU) in the international LCA standard ISO 14040 (HRN EN ISO 14040, 2008) is defined as “quantified performance of a product system for use as a reference unit.” According to (Furberg et al., 2022), three types of functional units were identified in the literature review on comparative LCAs of materials: the functional unit of reference flow, the functional unit of property and the functional unit of performance, with only the last strictly complying with the LCA guidelines. When carrying out LCA analyses, we find different functional units, e.g., 1 m2, 1 m3, 1 m length, 1 tone, 1 kg, whole structures or elements of structures. When defining the functional unit, one should be very careful and define it according to the objectives of the analysis. When researching and comparing the environmental impact of new materials, the functional unit is often expressed in m3. However, it should be taken into account that new materials used for structural purposes, in addition to the required properties of the material itself and the performance of the required tests, should also have adequate mechanical resistance and stability during the service life and fulfil the limit state of load-bearing capacity and serviceability as well as the expected service life. When comparing in the functional unit m3, the material that has a lower environmental impact per m3 may require more m3 to fulfil the same function of the structures due to the difference in strength, so it may end up being less environmentally friendly than a material that is less environmentally friendly per m3.
The service life is related to the durability, the different degradation processes of certain materials and the exposure conditions. When comparing the service life of different materials, it is important to emphasize that materials with a lower durability require more maintenance for the same expected service life and therefore additional emissions and costs during their service life. When carrying out a comparative LCA analysis of the environmental impact of different materials for structural purpose, it is therefore advisable to consider the entire load-bearing structure or be very careful when setting the inputs, outputs and objectives of research. The authors (Backes et al., 2023) discuss the functional unit of LCA analyses for comparative purposes in the construction sector, give a recommendation for FU of reinforced concrete and define it as: “1 m3 of concrete normalized by the 28-day compressive strength and the reference service life (RSL), which must include the exposure class, the specific concrete and the type and percentage of reinforcement.”
The use of different LCIA methods in the LCA analysis and the comparison of such results could potentially lead to different conclusions. The article (Dong et al., 2021) develops new conversion factors and conversion cards in order to make different life cycle impact assessment methods (LCIA) in the construction sector comparable and demonstrates this using case studies. Various authors have tried to point out the uncertainties associated with the LCA analyses carried out in the construction sector. The paper (Du and Karoumi, 2014) emphasize that uncertainties in the databases, methodology and scenario modelling can influence the LCA results and that biased results caused by normalization or weighting need to be carefully considered, especially for comparison purposes. Weighting is not mandatory in LCIA, but it is the element in which local data related to the production of building materials becomes important. The aim of study (Oztas and Tanacan, 2014) was to develop local weighting factors for building materials produced in Turkey that take into account the environmental impacts and emphasize that the most accurate results are obtained when the site-specific data on the processing and technology of the building materials are used. Differences in the LCA analysis due to the different LCA software used to perform the analysis has also been investigated by researchers. Paper (Aparecido Lopes Silva et al., 2019) analyses how different LCA results can be obtained due to using four different LCA software tools, namely, SimaPro, Gabi, Umberto and openLCA for the same product system. The study (Emami et al., 2019) compares the results of SimaPro and Gabi by applying the ReCiPe midpoint method and analysing the environmental impact for fifteen impact categories. The results confirm that the choice of software significantly influences the result in all impact categories and that extensive research is needed to improve the reliability of LCA software in the construction sector. The paper (Herrmann and Moltesen, 2014) also discusses whether there is a difference between SimaPro and GaBi that may influence the results and the decision-making process. Differences in the implementation of the impact assessment methods have been identified and it appears that these differences are primarily due to differences in the different databases used in these two software programs. All these uncertainties associated with the use of different methods, software, databases and steps in the LCA make the LCA analyses of different researchers difficult to compare.
Cost is a long-established aspect and the first of the three sustainability aspects analysed in the construction sector. Including costs in the analysis of the life cycle of a project ensures a reasonable balance between the variants offered and compared and aims to find the most cost-effective solution. When analysing the life cycle of costs, we find an imbalance in the methods described, i.e., in the classification of user costs. The user costs are divided into driver delay costs, vehicle operating costs and accident costs according to the methods described. It can be seen that user costs are considered as LCC, whereas in other analyses they are considered as part of the S-LCA. S-LCA is the least developed method in the infrastructure sector, but its impact is undeniable as it has a direct impact on society, both on the workers during construction, the people who will use the bridge, and on the wider social community. Studies that deal with all three analyses LCA, LCC and S-LCA of bridges simultaneously are not well represented in research. The article (Zhou et al., 2021) presents a comprehensive framework for the sustainability assessment of LCSA of six cable-stayed highway bridges in different regional economic zones in China, which shows that the analysis is under the influence of multifactorial change decisions and provides important insights for preliminary decisions in bridge construction. The study also determines an appropriate interval between gross domestic product (GDP) and sustainable development and discusses the correlation between regional economic development and the three pillars of sustainability.
Developed LCSA methods are in the application phase of sustainability research in the infrastructure sector and can be very useful for comparing different solution variants. However, caution is required when defining the methods and comparing the results obtained. Their application is of great importance for comparative purposes in defining solution options in order to derive the most sustainable solutions and discover the trade-offs that exist, for example, between the increased environmental impact of high-performance materials per unit and their benefits in terms of increased longevity and durability, reduced maintenance requirements or less material needed for the same function of the structure. These and similar themes are the kind of design decisions that LCSA analysis can highlight when designing new sustainable bridges.
4 Overview of the application of the LCSA methodology to bridges
The literature search for the LCSA research papers on bridges was carried out in the Scopus database. The search covered the period from 2008 to 2023, i.e., the last 15 years, for articles published in English in journals indexed in the Scopus database. The search area was limited to engineering and environmental sciences. The search was carried out using the following keywords: “bridge,” “LCA,” “life cycle assessment,” “environmental impact,” “carbon emission,” “life cycle cost,” “LCC,” “life cycle analysis,” “social-life cycle assessment,” “S-LCA,” “social impact assessment,” “LCSA,” “life cycle sustainability assessment.” Many of the studies found conduct only one of the LCSA analyses and refer to only one case, i.e., they have no comparable purpose. In this literature review, the research papers are selected in which the life cycle analysis of bridges was carried out at least with a LCA or in combination with one or both of the other LCSA assessment methods and in which different variants of the load-bearing structure of bridges were compared. The papers described were selected to cover the state of knowledge of the key parameters and uncertainties related to the input variables and their influence on the results when comparing LCSA of different bridge structures and to provide an insight into the possible development of sustainable bridges of the future. By analysing the work highlighted and the results obtained, possible directions for the development of more sustainable solutions for load-bearing bridge elements are identified and a critical review of all uncertainties, critical points and system limitations related to the results obtained is undertaken.
The following literature review lists papers that have performed a comparative analysis of different load-bearing element materials, different reinforcements or element types, different static systems or bridge types. It can be distinguished two cases of comparative analyses: the comparison of different variants of bridges, where whole bridges are considered and compared with LCSA, and the comparison of different alternative elements of bridges, e.g., different bridge superstructures while the substructures remain the same, with respect to different materials of the load-bearing elements (deck or girder) or different types of embedded reinforcement in the load-bearing elements. In both cases, analyses are often performed to compare new or optimized structural materials with the standard materials.
4.1 Comparison of various bridge solutions
Several LCA analyses were carried out to compare different bridge designs (Table 1). The analyses compared: three variants of the bridge (Bertola et al., 2021) designed to replace the existing dilapidated bridge with a span of 10 m: classical reinforced concrete, timber composite Ultra-high performance fiber-reinforced cementitious composite (UHPFRC) and the entire UHPFRC bridge, and three different variants (Hammervold et al., 2013) of a road bridge already built at different locations in Norway: a steel box girder bridge, a concrete box girder bridge and a timber arch bridge. LCA analyses were also carried out for two concrete bridges built with different types of concrete (Habert et al., 2012), one with standard concrete and the other with high performance concrete, as well as for five solution variants (Du et al., 2014) proposed for the construction of a new 320-m-long bridge with two lanes in both directions and for an average traffic volume of 10,000 vehicles per day.
In the first LCA analysis presented, a lifespan of 80 years is assumed and the environmental impact is compared using two indicators: Global Warming Potential and Ecological Scarcity. The results show that the composite timber-UHPFRC bridge and the full UHPFRC bridge have an advantage over the classic RC bridge. Although the UHPFRC emissions are higher compared to the emissions of the same volume of conventional concrete, a lower amount of UHPFRC is needed to build the same bridge and the UHPFRC has a higher durability, which reduces the need for maintenance during the service life.
In the second LCA analysis, which was conducted considering a 100-year service life, it is shown that the concrete box girder bridge is the most environmentally friendly in terms of overall impact, but in the category of potential global warming impact, the wooden bridge is the most favourable. Since the bridges were built at different locations and have different dimensions, lengths, widths and spans, 1 m2 of the bridge’s useable area was taken as the functional unit.
In the third LCA study, although the bridges are not the same size, as less concrete is needed for a bridge made of HPC concrete than for the same bridge made of standard concrete, authors define them as a functional unit, as they are two-lane bridges over a four-lane highway with a service life of 100 years. Although the environmental impact of HPC concrete is greater than that of standard concrete for the same material input, less HPC concrete is required for the same function. The results of the LCA analysis show that the HPC bridge has a 20% lower impact in the global warming potential category over the entire lifetime of the bridge cycle and a 50% lower impact if we consider only the concrete production phase. The paper shows that HPC concrete is more environmentally friendly than normal concrete and points out that the uncertainties related to the maintenance phase during the long lifetime of bridges are the biggest problem when assessing the environmental impact.
In the last case, the five variants analysed are: one-part bridge with two steel box girders, two-part bridge with two steel I-beams for each bridge section, two-part prestressed concrete box girder bridge with one prestressed concrete girder on each section, one-part concrete box girder bridge with two connected prestressed concrete box girders and concrete box girder bridge with one prestressed concrete box girder. Uncertainties related to key variables such as the percentage of recycled steel and the use of different concrete mixes affect the results of the analysis of the environmental impact of the life cycle of bridges, so the results of the comparison are not reliable, i.e., no single bridge proved to be the best, which contributed to the fact that a large number of load categories were analysed in addition to the factors mentioned above. In general, it was concluded that the composite steel bridges performed better in the construction phase and the single-span bridges in the maintenance phase, and that the most economical bridge is not necessarily the most environmentally friendly.
Study, which considered a simultaneous LCA and LCC analysis of different bridge designs during the life cycle of a bridge, compared a new FRP design for a new pedestrian bridge with a standard steel bridge design (Jena and Kaewunruen, 2021). The variants are compared for a service life of 120 years. If the FRP bridge does not reach this service life, it is replaced by a new identical bridge. Due to the uncertainties associated with the FRP material during its service life, the analysis was carried out considering five possible scenarios: a service life of 40 years, 60 years, 80 years, 100 years and 120 years. All scenarios show a cost reduction in the case of the FRP variant of the bridge. Although FRP has higher initial production costs, the construction costs are lower than the construction costs of a steel bridge. When analysing the environmental impact, the advantages of the FRP bridge are not obvious, as the FRP variant is only advantageous in the scenario where a service life of 120 years is considered. The results show that recycling of FRP at the end of its life cycle cannot provide an accurate analysis of this phase of the life cycle and that the sustainability of FRP materials can be questionable if the life cycle is short.
Another research conducts LCA and S-LCA analysis to compare three different bridges in each. In the first LCA and S-LCA were investigated to compare three optimised bridges (Penadés-Plà et al., 2020): two box-section post-tensioned concrete road bridges with different maintenance scenarios and a pre-stressed concrete precast bridge. The precast prestressed concrete bridge has the lowest impact on all environmental and social indicators and thus proves to be the most sustainable of the three options. The results also show that the production phase has the greatest impact on the environment and society, with concrete production being the process with the greatest environmental impact and steel production the process with the greatest social impact.
In the last study LCA, LCC and S-LCA are considered to compare three different bridges (Santos Gervasio and Silva, 2013): a steel–concrete composite bridge, a cast-in-place concrete bridge and a precast concrete bridge. As the bridges are not directly comparable, the functional equivalent was normalised by the area of the respective bridge deck to minimise this problem. The steel-concrete composite bridge performs better, although it has the highest initial costs, but is made of materials with a high recycled content and has short construction and maintenance times. The paper concludes that the construction phase and the traffic load in the working areas have a major influence on the service life of bridges and that a rapid construction time and low maintenance during the service life are the most important requirements for the bridge design.
4.2 Comparison of different individual elements of bridges
Several LCA analyses were carried out in which different variants of individual elements of bridges were compared (Table 2): four different designs of bridge concrete slab systems (prestressed concrete solid slab, prestressed concrete hollow slab, prestressed concrete box girder and composite steel-concrete box girder) for different spans (Martínez-Muñoz et al., 2021), two different solutions for the construction of a new bridge (O Born, 2018) glue-laminated timber superstructure and a classic reinforced concrete superstructure were designed, while the reinforced concrete foundations and steel pylons are the same for both versions of the bridge), 15 different variants of prestressed concrete slabs (Navarro et al., 2018b) in terms of corrosion resistance of RC slab reinforcement in an aggressive marine environment and two different bridges and their variants with different types of reinforcement (Khorgade et al., 2022).
In the first study, the analysis concludes that a prestressed concrete solid slab is the best solution for spans of less than 17 m and a prestressed concrete hollowed slab is the best solution for spans of 17–25 m, while for spans of 25–40 m the best solution depends on the percentage of recycled structural steel, with the best composite steel-concrete box girder bridge being chosen for a recycled content of more than 90% and a prestressed concrete box girder bridge for a lower recycled content. This article emphasizes the influence of the steel recycling rate as a key variable for the results of the LCA analysis.
In the second study, the timber superstructure is designed as a 70-m-long glulam prefabricated timber truss, while the second version is a reinforced concrete box girder with a span of 70 m. The results show that the timber superstructure has a lower environmental impact than the concrete bridge in all included impact categories. The main motivation for the design of the timber bridge superstructure in this case study was the utilisation of locally available industry. The results of the LCA analysis show that timber bridges have significantly lower emissions than concrete bridges and that timber should be considered as main material for bridges of that span.
In the third study, three categories of preventive anti-corrosion measures for concrete elements were considered: the properties of the protective layer by increasing the protective layer or increasing the density of the protective layer by reducing the water-cement ratio of the concrete mixtures and also includes cases where various additives are added to the concrete mixture to reduce the porosity of the concrete and the diffusion coefficient. In the second category, the reinforcement is modified by the use of corrosion-resistant, stainless or galvanized steel. The third category involves isolating the concrete from the environment and preventing the penetration of chlorides into the concrete through various coatings. In terms of environmental impact, concrete surface coatings have proven to be the best. They lead to a 70% lower environmental impact during the lifetime of the bridge than the classic design without preventive measures. Cases that focus on reducing the density of the protective layer have also proven to be competitive in reducing the environmental impact. This article emphasizes the importance of preventive design decisions to increase the durability of structures exposed to chloride and thus reduce the impact on the environment. By using improved designs instead of conventional designs, the environmental impact in the LCA can be reduced due to the lower maintenance requirements.
Since CFRP is a heterogeneous material, three environmental impact scenarios were analysed in the fourth study: an unfavourable, a realistic and a favourable scenario based on the most common results for GWP (Global Warming Potential) values of CFRP from the literature. For the first concrete bridge, three different types of reinforcement were tested: standard steel, CFRP and prestressed CFRP. The results show that CO2 emissions are reduced by 28% when standard steel is replaced by pre-stressed CFRP. For the second investigated bridge, which is in the planning phase, two variants were analysed: a post-tensioned bridge with steel and CFRP reinforcement. The CFRP reinforcement shows an 18.8% reduction in GWP, assuming the favourable scenario. Although the GWP values of CFRP per unit mass are almost 10 times higher than the GWP values of standard steel, construction with CFRP reinforcement leads to large savings in the material required. The article concludes that pre-stressed CFRP reinforcement is the most cost-effective solution for bridges without high traffic loads. By analysing the three different scenarios for the environmental impact of the production of CFRP materials, an attempt is also made to identify and overcome the uncertainties associated with the various production processes and input variables, especially when new materials are involved.
In studies where LCA and LCC analyses were performed to compare different variants of individual bridge elements, concrete and aluminium slabs on steel girders were compared for short span bridges (Pedneault et al., 2021), and a road bridge of aluminium slabs on timber girders was compared to a bridge of aluminium slabs on steel girders, while an aluminium-timber bridge was compared to a standard variant of a concrete slab on steel girders (Beudon et al., 2022).
The first study concludes that while the initial cost of an aluminium bridge is 2 times higher, the total life cycle cost of an aluminium bridge, analysed over a 75-year life span, is 4 times lower and that the traffic detour caused by maintenance contributes the most to the cost and environmental impact of both bridges. It follows that the aluminium bridge is viable if the traffic detour caused by the maintenance work is greater than or equal to the average daily traffic flow of 800 vehicles per year and a 5 km long bypass. The results also show that the aluminium bridge is a more environmentally friendly solution. This paper emphasises the impact of traffic flow design requirements on bridges, their specific microlocation and the user costs on the results of life cycle analysis.
In the second comparison, the bridge was considered as a functional unit, whereby only the superstructure of the three solution variants was compared over a service life of 75 years and an average daily car traffic of 2,500 vehicles. The aluminium-timber bridge is an economically and ecologically better solution than an aluminium bridge on steel girders. A comparison of an aluminium-timber bridge with a standard bridge with a concrete slab on steel girders shows a reduction in total costs of 86% and a reduction in environmental impact of 88%. The paper emphasises that timber initially reduces construction costs, while aluminium is initially more expensive but reduces maintenance costs.
In a study that analysed variants from an environmental, cost and social impact perspective, two rehabilitation proposals were compared: the replacement of the entire bridge and the replacement of the existing concrete deck with a FRP deck with a service life of 80 years for an existing dilapidated bridge made of steel girders with an RC slab with a span of 12 m (Mara et al., 2014). The new FRP deck would allow an increase in the traffic load that the steel girders can carry due to its low dead weight. Replacing the old concrete deck with a new one was not an option due to the high dead weight of the new RC deck, and the load-bearing capacity of the bridge would still be limited by the capacity of the existing steel I-beams. Although the manufacture of FRP materials creates a larger carbon footprint per unit value compared to concrete or steel, the savings are visible in the form of a reduction in the amount of material required for the substructure due to the lightweight FRP deck. The environmental impact was only analysed in the case of CO2 emissions. When comparing the entire life cycle, CO2 emissions were reduced by 20% for the variant with the FRP deck compared to the other variant where the entire bridge was replaced. The study shows that the variant with the replacement of the FRP deck has advantages in terms of costs and environmental impact when analysing the entire life cycle and that the impact on society is lower due to the shorter construction time, less traffic disruption and a safer construction site during the construction period.
4.3 Discussion on the application of the LCSA methodology to bridges
From studying the literature (Tables 1, 2), it can be concluded that there are the fewest impact analyses for the social aspect of sustainability and that research is mainly based on one or two pillars of sustainability. Thus, in most of the analysed works, only the environmental impacts are analysed without simultaneously combining the impacts of all three pillars of sustainability, i.e., both the costs and the social impacts in a specific LCSA method. However, when comparing different bridge solutions, the combination of environmental impact and life cycle costs is frequently analysed.
As the material of the load-bearing bridge structure has a major impact on the environmental performance of bridges, the investigation of alternative solutions involves analysing different alternative materials for the load-bearing structure of the bridge, e.g., steel, reinforced concrete, prestressed concrete, timber, FRP or various composite solutions such as steel-concrete, steel-wood, etc., by performing structural design and calculations for different alternative materials may require different types of bridges, taking into account the laws of mechanical resistance and stability of the structural elements made of different materials used for structural purposes. Sometimes a particular material requires a different structural system or bridge type due to its limitations and/or the cost-effectiveness of the application. The most commonly used materials for road bridges are reinforced concrete and steel. The review of the literature (Tables 3, 4) shows that the key variable that strongly influences the results of the life cycle analysis for bridges with standard construction materials is the percentage of recycled steel, for either structural steel or reinforcing steel. The above variables, which are often assigned assumptions in the analyses, influence the results, i.e., the uncertainties associated with the conclusions of the analysis. In (Martínez-Muñoz et al., 2021), different types of bridge decks made of concrete, steel or a concrete-steel composite material were analysed as load-bearing structures for different spans in order to determine the most environmentally sustainable one for each span. In this study, the steel recycling rate is the key variable that can determine which option is most sustainable for a given span. The study emphasises that in countries where less steel is recycled, concrete structures are the most environmentally friendly solution, but in countries with clean steel production processes, composite and steel bridges are the better choice. Paper (Du et al., 2014) also emphasise the steel recycling rate as a key variable.
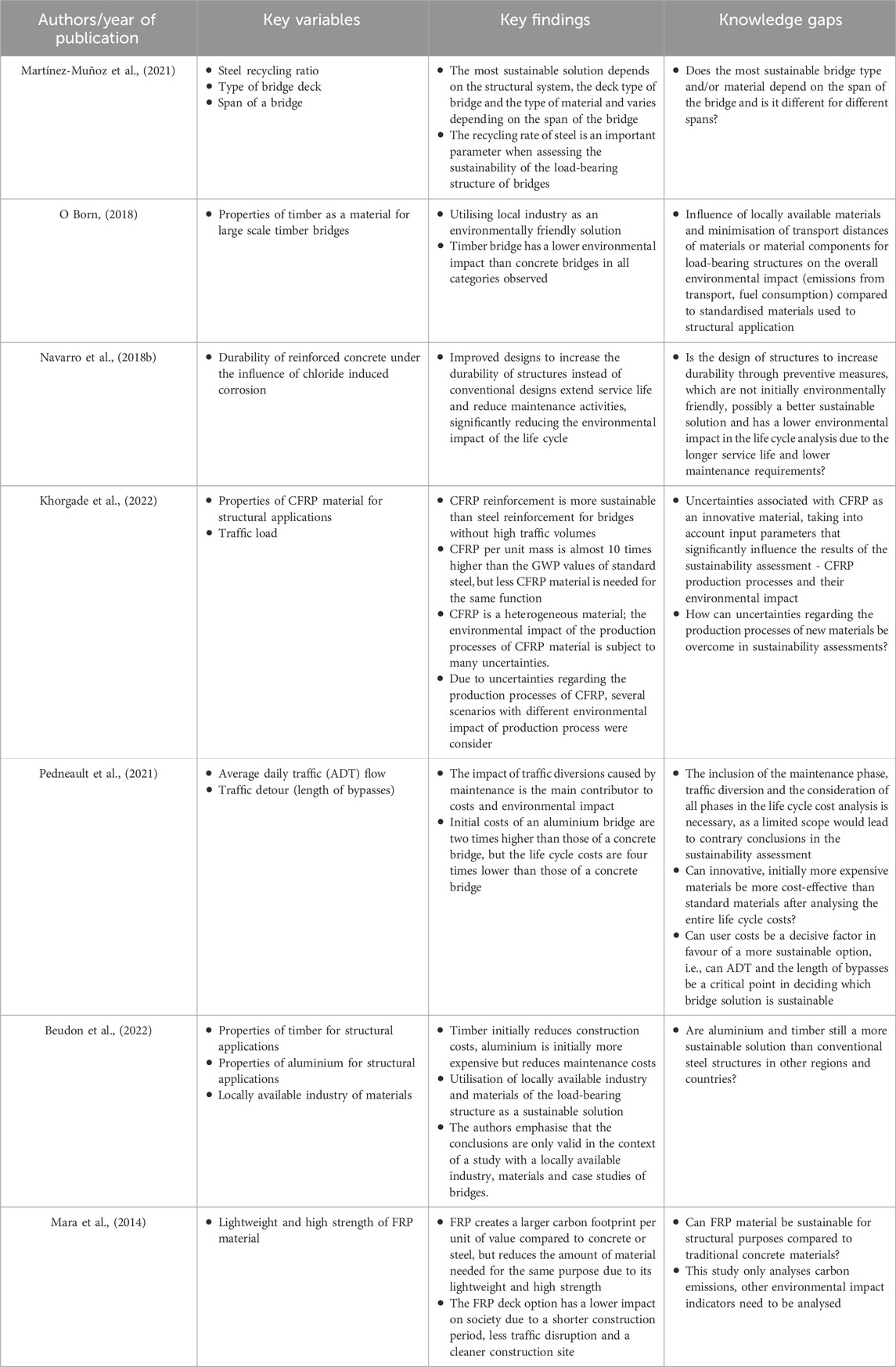
Table 4. Findings from the literature review for comparison of different individual elements of bridges.
In an article (Navarro et al., 2018b), the durability of reinforced concrete (RC) structures was identified as a key variable and different preventive measures to prevent chloride-induced corrosion throughout the life cycle of the bridge element were analysed, highlighting the maintenance phase of the RC as a key phase for calculating the environmental impact. The preventive measures analysed in this paper can be categorised into three groups: density of the protective layer, modified reinforcement and isolation of the concrete from the environment. In (Habert et al., 2012), the compressive strength of a concrete was analysed as a key variable and it was concluded that the environmental impact of HPC concrete is higher than that of conventional concrete for the same material input, but that less HPC concrete is required for the same bridge design at the case study level. The life cycle assessment carried out in this study shows that the HPC bridge is a more environmentally friendly solution than a bridge made of conventional concrete at the case study level.
The same trend can be observed in some other studies, especially for new innovative materials compared to commonly used materials. Innovative materials often have a greater environmental impact (Mara et al., 2014; Bertola et al., 2021; Khorgade et al., 2022) during material production than the same unit of the commonly used standard material to which they are often compared. However, due to their better mechanical properties and resistance, their quantity in the construction of the same bridge is lower, making them a more environmentally friendly solution when considering the case study of the bridge and analysing the entire life cycle. The articles (Mara et al., 2014; Bertola et al., 2021; Khorgade et al., 2022) highlight these gaps in knowledge by comparing such innovative materials with conventional materials. Paper (Khorgade et al., 2022) points out that CFRP per unit mass is almost 10 times higher than the GWP values of standard steel, but for the same case study less CFRP material is needed and CFRP reinforcement proves to be a more sustainable option than standard steel reinforcement. Paper (Mara et al., 2014) emphasise that FRP generates a larger carbon footprint per unit value compared to concrete or steel, but reduces the amount of material needed for the same purpose and is the better solution for replacement purposes in the analysed case study due to its light weight and ability to provide additional load-carrying capacity of the bridge.
But when it comes to new innovative materials for load-bearing elements of bridges, which are not yet widely used in bridge construction, there is uncertainty about durability, as there is not much practical data on such materials and the question of durability during service life is controversial. The article (Jena and Kaewunruen, 2021) addresses this issue and overcomes the problem of uncertainty regarding the durability of glass fibre reinforced plastics (GFRP) and the potential service life that such a bridge can achieve by analysing several service life scenarios in the case of a GFRP bridge and comparing each of these scenarios with a standard steel bridge scenario. Accordingly, uncertainties about maintenance requirements within the service life are often the subject of assumptions. For most new materials, the question of recycling at the end of their service life is also controversial, so that the conclusions of the analysis cannot be drawn with great certainty which was also emphasised in the paper (Jena and Kaewunruen, 2021) for the recyclability of GFRP in the end-of-life stage.
In addition, the results of LCC analyses show gaps in knowledge when analysing the life cycle costs of such innovative materials. Although these are higher initially, they can be much lower during their lifetime due to their better properties, especially their longer durability, which reduces the need for maintenance. Reducing the need for maintenance during the life cycle means that traffic on or under the bridge is less affected during the use stage. It reduces additional costs for users and emissions due to longer detours and crowds. It also reduces emissions and costs related to maintenance work. The feasibility of the application or the advantage of a particular solution option over another may depend on the average annual daily number of vehicles on the bridge or under the bridge and the length of the bypass, as shown in paper (Pedneault et al., 2021). This requires an analysis of the current and future demands on the bridge as part of the life cycle analysis. The articles (Santos Gervasio and Silva, 2013; Khorgade et al., 2022) emphasise the importance of traffic congestion in work zones and the influence of user costs on the overall results of the life cycle analysis. Paper (Pedneault et al., 2021) concludes that influence of traffic detours caused by maintenance contributes the most to the cost and environmental impacts. Due to their high initial costs, the authors in (Mara et al., 2014) highlight that the feasibility of FRP decks becomes clearer if the bridge is located on a high traffic road, where the traffic disruption and user costs caused by the construction works are higher. It can also be concluded that the uncertainties associated with the maintenance phase during the long lifetime of bridges are an important issue when assessing the environmental impact and costs, and that prefabricated elements have an advantage over cast in place elements in the construction phase due to their shorter construction time and lower impact on traffic during construction which is emphasised in the papers (Penadés-Plà et al., 2020; Santos Gervasio and Silva, 2013).
Studies prioritise the availability of materials at the bridge site and point out that locally available materials have a more favourable environmental footprint as they require less transport and therefore cause lower emissions during transport, lower fuel consumption and ultimately lower transport costs. The papers (O Born, 2018; Beudon et al., 2022), motivated by the locally available industries of certain materials, attempt to fill knowledge gaps in their application by showing that they have a lower environmental impact than conventional materials when analysing and comparing the environmental impact of their use in different bridge designs over the entire life cycle.
A paper (Du et al., 2014) shows a scattering of results due to excessive coverage, e.g., too many environmental impact categories in the LCA analysis, as the same variant is often not the most favourable solution in all impact categories. The paper also emphasises uncertainties in key variables, overly broad system boundaries and unclear objectives of the analysis as the main problems of comparative life cycle analysis. Some of the studies analysed compared bridges that are not in the same place and do not have the same dimensions. A “bridge that has the same function and purpose” was then taken as the functional unit or the results were reduced to 1 m2 bridge area. The article (Hammervold et al., 2013) compares three bridges already built at different locations, which do not have the same dimensions, spans, widths and number of lanes. Such a comparison raises questions about the functional unit for the comparison of different bridge variants, which must be discussed. In order to be compared transparently, the bridges must at least be functionally equivalent, i.e., have the same function, the same exposure classes, the same traffic loads and all other relevant loads on the bridge structures. Performing an analysis and interpreting the results when only one component (e.g., the main beam or the pier) is modified is not a sufficiently objective solution (if the system boundaries and analysis objectives are not adequately defined), as all components of the bridge interact with each other and act as a whole and should therefore also be analysed. Accordingly, one should not ignore the fact that the use of new, innovative and lighter materials has an advantage in terms of reserve capacity due to their lower weight. The advantage of using such materials for the bridge superstructure, for example, is that less material is needed for the substructure as it is less stressed, as shown in paper (Mara et al., 2014).
Another major problem is the fact that the standardised LCSA method for comparing bridges has not yet been developed. The paper (Penadés-Plà et al., 2020) emphasises that the comparability between studies performing a life cycle analysis and using different methodology leads to a loss of information on the sustainability assessment, as the results obtained cannot be compared. It should also be noted that due to a number of uncertainties related to the long life cycle of bridges and a number of parameters that may change during their service life, various assumptions are made in the analyses that are not objective and reliable enough to be comparable with other analyses. It is difficult to draw general conclusions about generally more sustainable solutions based on such assumptions and the comparison of results is complicated and unrealiable by the non-uniformity of definitions of functional units, boundary systems and methodology. The authors in (Beudon et al., 2022) highlight that the conclusions are only valid in the context of the study, as it was conducted with a locally available industry, materials and a specific case study of bridges.
As a contribution to sustainability research in the design of sustainable bridges in the future, this research shows how the application of life cycle sustainability assessment methods can be of interest to reduce the environmental impact, cost and impact on society during the life cycle of bridges. This paper identified knowledge gaps in the sustainability assessment of bridges by analysing the application of LCSA methods for comparative assessments of different bridge variants. One of the most common knowledge gaps whose potential can be explored by the LCSA method is the reduction of environmental emissions and life cycle costs of bridges using new or innovative high performance materials to determine their true potential or parametrically dependent trade-offs in comparison between different variants, even if their initial emissions and costs are not promising compared to standard variants. This may relate to the main material of the load-bearing structure, but also to the use of new types of reinforcement. It also includes investigation and development of improved standard types of materials, for example, concrete with optimized concrete mixes or high-performance concretes. The high performance materials often manifest in increased durability, a longer service life, but also in better mechanical strength and resistance properties. These findings can encourage the future research and development of high-performance materials with better mechanical strength and resistance as well as increased durability during service life in order to design more sustainable bridges in the future.
Further knowledge gaps were summarised regarding the importance of certain input parameters when analysing the life cycle of bridges and to identify case study dependent key variables such as analysed service life, local availability of materials or average daily traffic flow in relation to user costs (Pedneault et al., 2021). In the search for more sustainable approaches to bridge design, this overview addresses the need for a comparative analysis of a range of solution options in the design process using the LCSA method to explicitly identify the parametric dependent trade-off between different bridge options using a specific case study. This would minimise the assumptions regarding the input data, reduce the associated uncertainties regarding the results of the LCSA and allow a trade-off between the compared variants to be identified in a specific case study.
Some of the knowledge gaps or trade-offs in development of sustainable bridges that may be investigated through comparative LCSA are:
- Is there a real advantage of prefabricated elements due to their favourable impact on S-LCA, as they can be built quickly and have less impact on traffic, considering bridge’s potentially lower durability during their life cycle?
- Are improved materials with a higher initial cost a better option than standard materials, as they are needed less for the same function and may require less maintenance during their lifetime?
- Is there a real advantage in LCSA for high performance materials and their greater environmental impact per unit in the manufacturing processes as they potentially have longer durability and better structural performance during their life cycle?
- Do prefabricated elements have the advantage over monolithic construction because they can be better managed at the end of their life cycle in terms of waste minimisation, reuse or recycling potential?
- Does a locally available material have an advantage over a high-performance material, even if it has a lower durability during its life cycle?
These are examples of general research questions where LCSA analysis can be used as a basis for decision making in the early stages of planning and designing new bridges in order to design and build more sustainable bridges. A more precise definition of the research questions and recommendations will depend on the specific project task, site conditions and requirements of the bridge and should be developed by the project team in the early design phase in accordance with the guidelines and findings of the scientific community.
Although, the LCSA method needs to be harmonised so that it can be used effectively in the design of bridges and as a tool for the development of more sustainable bridges. It is up to the scientific community to define an LCSA method that is suitable for the comparison of bridge variants. The identification of knowledge gaps in the sustainability assessment of bridges and the development of directions and approaches for the design of more sustainable bridges is also a task for the scientific community. This would facilitate further steps for the professional community and enable and simplify the application of the LCSA method in the design process of bridges as well as the inclusion of life cycle sustainability assessments in the public procurement process for bridge projects. The paper (Du et al., 2014) points out the problem of incorporating the LCA method into the public procurement process and therefore emphasises that indicators and weighting systems must be clearly specified in order to be applicable in a procurement process. In order for LCSA analyses to be included in the criteria for public procurement, additional efforts are required from the scientific and professional communities. Currently, there are various rating tool systems for assessing the sustainability of infrastructure projects that can evaluate all types of infrastructure and obtain a certificate depending on the implementation of sustainable approaches in different phases of the life cycle of bridges, which are presented and described in more detail in the paper (Milić and Bleiziffer, 2024). The inclusion of the sustainability approaches, assessments and LCSA methodology is an objective that should be pursued towards global policy guidelines and strategies to establish sustainable development and prevent climate change.
5 Conclusion
Sustainable bridges for the new generations must fulfil holistic, integrated criteria in terms of economic, environmental and social aspects. The minimisation of environmental impact should be ensured by reducing embodied energy, emissions, waste production, consumption of natural resources and the recyclability of materials used in the production phase and at the end of life during dismantling. The bridge must also meet the needs of society now and during its long service life and minimise the negative impact on quality of life, the local environment and human health. Due to the long lifespan and potentially increasing demands, where operating costs can be higher than the initial construction costs, the bridge must be economically viable during its lifetime, taking into account both initial and operating costs. Given these requirements and in line with sustainable development approaches, considering the entire project life cycle is a new trend in the planning of major construction infrastructure projects. Conducting studies in the pre-design phase of new bridge projects to compare different conceptual design options and work on their optimization, taking into account the three fundamental pillars of sustainability throughout the project life cycle, is becoming increasingly important in terms of sustainable development.
By analysing the literature on LCSA of bridges, some recommendations for performing LCSA analyses for comparative purposes are given:
- Analysis of all stages of the life cycle of the bridge for a comparative purpose of different load-bearing structures: The life cycle analysis requires an analysis during the long service life of bridges. Comparisons of solution variants only for the production phase or the maintenance phase, i.e., the end-of-life phase, can lead to incorrect conclusions.
- Simultaneous analysis of environmental, economic and social aspects: Simultaneous analysis of all three aspects of sustainability and mutual ranking of the individual aspects and their criteria according to importance in the present case, i.e., according to the long-term plans and objectives of the project.
- The uncertainties associated with the input data should be reduced to a minimum: The long service life of bridges requires a multitude of information at every stage of the life cycle and a multitude of decision-making processes. The definition of the system boundaries is subject to various uncertainties during the life cycle, which should be taken into account with a certain margin and depending on the importance of the individual criteria. The differences can lie in the local characteristics of the industry and construction processes, the availability of construction materials, the needs of society and the way the bridge is managed during the utilisation phase, or in political decisions by the government. Such uncertainties should be minimized and should never be expressed in key variables and thus strongly influence the decision-making process and the selection of the optimal bridge solution. A sustainability analysis of a new bridge project on a case-by-case basis according to the respective project task would minimize this uncertainty.
- Functional unit for comparative purpose: For the comparison of variants to be objective, the comparison should be based on the bridge solution variants that have the same functional (purpose), structural (bearing capacity) and spatial parameters as well as input data. It is also desirable that the variants are in the same location so that they have the same geotechnical characteristics, the same level of seismic and other environmental stresses and the same exposure conditions.
If these recommendations do not apply, the research questions, and objectives of LCSA research need to be carefully defined when comparing different designs in order for the interpretation of the results to be valid.
By analysing the findings from the literature review, it is possible to provide an overview of the key development directions in the design of sustainable bridges of the future:
1. Longer durability of structural elements with less need for repair and replacement during the bridge’s service life: Reducing lifetime maintenance needs is key to lowering maintenance costs, traffic disruption and associated user costs, minimizing new resource consumption and waste production, and minimizing associated environmental impacts. The increased durability of bridges can lead not only to reduced maintenance requirements and associated benefits, but also to an extension of the service life for which bridges are designed, thereby extending the investment period and minimizing costs within that period. The extended lifespan therefore directly reduces the cost of demolishing the existing bridge and generating waste, the cost of building a new bridge, the emissions associated with the above works, the consumption of resources and the impact on traffic and the local population. Longer-lasting structures may initially be a more expensive or environmentally unfavourable solution, but in a life cycle analysis they may prove to be a better compromise solution for long-term goals.
2. Use of high-quality, high-performance materials: Development of high-performance materials for the load-bearing structure of new bridges with better structural and environmental life cycle performance. Better structural performance is desirable in terms of mechanical strength and stability, durability and capacity. Designing bridges that last longer and ultimately achieve a longer service life is a sustainable approach, and high-performance materials play an important role in this. From a review of the literature, it can be concluded that innovative materials are often initially more expensive per unit price compared to standard materials and may be less environmentally friendly. Due to their better mechanical properties, they have the advantage that they are often required in smaller quantities and in some cases their effectiveness is quickly apparent and even fully justified when the entire life cycle of the bridge is analysed. The development of high-performance materials and their optimization in terms of purchase price and environmental impact is crucial for the development of sustainable bridges of the future.
3. Faster construction with less traffic congestion: the development of new, advanced construction techniques that enable faster installation and thus less traffic disruption on the bridge or under the bridge, which in turn leads to less traffic congestion on the bypasses. From this point of view, prefabricated elements have great application potential in this development, and their advantage also lies in the reduced labour required on the construction site.
To improve the implementation of LCSA methods for bridges, further research is needed on the following terms:
- Functional unit: It is necessary to harmonise the functional unit for bridges when performing LCA analyses, especially for the analysis of new materials and for comparative purposes.
- Uncertainties: Investigation to minimise uncertainties or find a way to overcome them, especially for comparative purposes, is a key issue. Some of the uncertainties can be minimised by defining the correct system boundaries when performing LCSA. The discussion section of the literature review also presents some ways for overcoming certain uncertainties in comparative LCSA analyses. Uncertainties may also be associated with the use of different software, methods or databases.
- More research of S-LCA of bridges: The S-LCA of bridges is the least studied in the LCSA methodology. It is necessary to precisely define the methodology for S-LCA for bridges and the corresponding issues between LCC and S-LCA methods.
- Weighting between LCSA methods: Determine whether LCA, LCC and S-LCA aspects and their associated indicators have the same importance when weighting the results for the interpretation of the LCSA analysis. Determine which aspect should be weighted more heavily and does this depend on the micro-location of the bridge or can this be determined for bridges in general.
In addition, development of sustainable bridges requires further research and comparative LCSA analyses to find more sustainable approaches. The recently intensively researched LCSA methods for life cycle analysis should aim to standardise and simplify their application, which would contribute to their faster implementation in practise in the variant solution study phases in order to select the optimal variant when implementing new projects.
In terms of sustainable development, it is necessary to include LCSA analyses in the process of selecting the best proposed solution in public tenders for a new bridge project. The LCSA results of the proposed bridge solution options should be evaluated together with other tender criteria for the selection of the appropriate new sustainable bridge of the future.
Author contributions
IM: Conceptualization, Methodology, Visualization, Writing–original draft, Writing–review and editing. JB: Writing–review and editing, Methodology, Conceptualization, Supervision.
Funding
The author(s) declare that no financial support was received for the research, authorship, and/or publication of this article.
Acknowledgments
Parts of HRN EN 15804:2019, HRN EN ISO 14040:2008 and HRN EN 17472:2022 have been used with HZN’s permission. Croatian standards are issued by the Croatian Standards Institute, Ulica grada Vukovara 78, Zagreb. Copyright to and the Croatian Standards Institute reserves all rights of exploitation of standardization documents issued by it.
Conflict of interest
Author IM was employed by SMAGRA d.o.o.
The remaining author declares that the research was conducted in the absence of any commercial or financial relationships that could be construed as a potential conflict of interest.
Publisher’s note
All claims expressed in this article are solely those of the authors and do not necessarily represent those of their affiliated organizations, or those of the publisher, the editors and the reviewers. Any product that may be evaluated in this article, or claim that may be made by its manufacturer, is not guaranteed or endorsed by the publisher.
References
An EU-wide assessment (2020). An EU-wide assessment of National Energy and Climate Plans, Driving forward the green transition and promoting economic recovery through integrated energy and climate planning. China: COM, 564–final.
A new Circular Economy (2020). A new circular economy action plan for a cleaner and more competitive Europe, China COM.
Aparecido Lopes, S. D., Oliveira Nunes, A., Aparecida da Silva Moris, V., Moro, P. C., and Oliveira Rodrigues, T. (2017). How important is the LCA software tool you choose? Comparative results from GaBi. openLCA, SimaPro Umberto, CILCA, Medellín, 12–15.
Aparecido Lopes Silva, D. A., Nunes, A. O., Piekarski, C. M., da Silva Moris, V. A., de Souza, L. S. M., and Rodrigues, T. O. (2019). Why using different Life Cycle Assessment software tools can generate different results for the same product system? A cause–effect analysis of the problem. Sustain. Prod. Consum. 20, 304–315. doi:10.1016/j.spc.2019.07.005
Backes, J. G., Hinkle-Johnson, R., and Traverso, M. (2023). The influence of the Functional Unit on the comparability of life cycle assessments in the construction sector: a Systematic Literature Review and attempt at unification for Reinforced Concrete. Case Stud. Constr. Mater. 18, e01966. doi:10.1016/j.cscm.2023.e01966
Bare, J. (2011). TRACI 2.0: the tool for the reduction and assessment of chemical and other environmental impacts2.0. Clean Technol. Environ. Policy 13 (5), 687–696. doi:10.1007/s10098-010-0338-9
Benoit-Norris, C., Aulisio Cavan, D. A., and Norris, G. (2012). Identifying social impacts in product supply chains: overview and application of the social hotspot database. Sustainability 4, 1946–1965. doi:10.3390/su4091946
Bertola, N., Küpfer, C., Kälin, E., and Brühwiler, E. (2021). Assessment of the environmental impacts of bridge designs involving UHPFRC. Sustainability 13, 12399. doi:10.3390/su132212399
Beudon, C., Oudjene, M., Djedid, A., Annan, C.-D., and Fafard, M. (2022). Life cycle assessment of an innovative hybrid highway bridge made of an aluminum deck and glulam timber beams. Buildings 12, 1616. doi:10.3390/buildings12101616
BREEAM (2022). BREEAM infrastructure, projects - version 6, international, 2022, bregroup. China: com.
Bulle, C., Margni, M., Patouillard, L., Boulay, A. M., Bourgault, G., De Bruille, V., et al. (2019). IMPACT World+: a globally regionalized life cycle impact assessment method. Int. J. Life Cycle Assess. 24, 1653–1674. doi:10.1007/s11367-019-01583-0
Cui, F., Song, L., Wang, X., Li, M., Hu, P., Deng, S., et al. (2022). Seismic fragility analysis of the aging RC columns under the combined action of freeze–thaw cycles and chloride-induced corrosion. Buildings 12, 2223. doi:10.3390/buildings12122223
den Heede, P. V., and De Belie, N. (2012). Environmental impact and life cycle assessment (LCA) of traditional and ‘green’ concretes: literature review and theoretical calculations. Cem. Concr. Compos. 34, 431–442. doi:10.1016/j.cemconcomp.2012.01.004
Dodd, N., Donatello, S., and Cordella, M. (2021). Level(s) indicator 6.1: life cycle costs user manual: introductory briefing, instructions and guidance. China: JRC.
Dong, Y., Hossain, M. U., Li, H., and Liu, P. (2021). Developing conversion factors of LCIA methods for comparison of LCA results in the construction sector. Sustainability 13, 9016. doi:10.3390/su13169016
Du, G., and Karoumi, R. (2014). Life cycle assessment framework for railway bridges: literature survey and critical issues. Struct. Infrastructure Eng. Maintenance, Manag. Life-Cycle Des. Perform. 10 (3), 277–294. doi:10.1080/15732479.2012.749289
Du, G., Safi, M., Pettersson, L., and &Karoumi, R. (2014). Life cycle assessment as a decision support tool for bridge procurement: environmental impact comparison among five bridge designs. Int. J. Life Cycle Assess. 19, 1948–1964. doi:10.1007/s11367-014-0797-z
Emami, N., Heinonen, J., Marteinsson, B., Säynäjoki, A., Junnonen, J. M., Laine, J., et al. (2019). A life cycle assessment of two residential buildings using two different LCA database-software combinations: recognizing uniformities and inconsistencies. Uniformities Inconsistencies, Build. 9, 20. doi:10.3390/buildings9010020
Envision (2018). Sustainable infrastructure framework. Washington, DC, USA: Published by Institute for Sustainable Infrastructure.
Forging a climate-resilient Europe (2021). Forging a climate-resilient Europe - the new EU strategy on adaptation to climate change. China: COM. 82 final.
Freyermuth, C. (2009). Service life and sustainability of concrete bridges, perspective, aspire. Fall, 12–17.
Frischknecht, R., Krebs, L., et al. (2021). Swiss eco-factors 2021according to the ecological scarcity method, methodological fundamentals and their application in Switzerland, Fed. Office Environ. (FOEN), Bern, 2121, 252.
Furberg, A., Arvidsson, R., and Molander, S. (2022). A practice-based framework for defining functional units in comparative life cycle assessments of materials. J. Industrial Ecol. 26, 718–730. doi:10.1111/jiec.13218
Gervasio, H. (2010). Sustainable design and integral life-cycle analysis of bridges (Portugal: University of Coimbra). Thesis (PhD).
Gervasio, H., and Simões da Silva, L. (2012). Life-cycle social analysis of motorway bridges. Struct. Infrastructure Eng., 1–21. doi:10.1080/15732479.2011.654124
Giunta, M., Bressi, S., and D’Angelo, G. (2018). Life cycle cost assessment of bitumen stabilised ballast: a novel maintenance strategy for railway track-bed. Constr. Build. Mater. 172, 751–759. doi:10.1016/j.conbuildmat.2018.04.020
Goedkoop, M., Oele, M., Leijting, J., Ponsioen, T., and Meijer, E. (2016). Introduction to LCA with SimaPro.
Goedkoop, M., and Spriensma, R. (2001). The eco-indicator 99, A damage oriented method for life cycle impact assessment, methodology report. Third edition. PRé Consultants B.V.
Grace, N. F., Jensen, A. A., Eamon, C. D., and Shi, X. (2012). Life-cycle cost analysis of carbon fiber-reinforced polymer reinforced concrete bridges. ACI Struct. J., 697–704.
Habert, G., Arribe, D., Dehove, T., Espinasse, L., and Le Roy, R. (2012). Reducing environmental impact by increasing the strength of concrete: quantification of the improvement to concrete bridges. J. Clean. Prod. 35, 250–262. doi:10.1016/j.jclepro.2012.05.028
Hammervold, J., Reenaas, M., and Brattebø, H. (2013) “Environmental life cycle assessment of bridges,”J. bridge Eng., 18, 153–161. doi:10.1061/(asce)be.1943-5592.0000328
Hauschild, M., and Potting, J. (2005). Spatial differentiation in life cycle impact assessment - the EDIP2003 methodology, institute for product development. USA: Technical University of Denmark. Environmental news No. 80.
Herrmann, I. T., and Moltesen, A. (2014). Does it matter which Life Cycle Assessment (LCA) tool you choose? A comparative assessment of SimaPro and GaBi. J. Clean. Prod. 86, 163–169. doi:10.1016/j.jclepro.2014.08.004
HRI CEN/TR 15459-2 (2017). HRI CEN/TR 15459-2:2017 Energijska svojstva zgrada–Postupak ekonomske ocjene za Energijske sustave u zgradama -- 2. dio: objašnjenje i obrazloženje norme EN 15459-1, Modul M1-14 (CEN/TR 15459-2:2017); Energy performance of buildings -- Economic evaluation procedure for energy systems in buildings -- Part 2: explanation and justification of EN 15459-1.
HRN EN 16627 (2015). HRN EN 16627:2015 Održivost građevina–Ocjenjivanje ekonomskih svojstava zgrada– Metoda izračuna (EN 16627:2015); Sustainability of construction works -- Assessment of economic performance of buildings -- Calculation method (EN 16627:2015).
HRN EN 15459-1 (2017). HRN EN 15459-1:2017 Energijska svojstva zgrada–Postupak ekonomske ocjene za Energijske sustave u zgradama -- 1. dio: postupak proračuna, Modul M1-14 (EN 15459- 1:2017); Energy performance of buildings -- Economic evaluation procedure for energy systems in buildings – Part 1: calculation procedures. Module M1-14 (EN 15459-1:2017).
HRN EN 15804 (2019). HRN EN 15804:2019 Održivost građevina -- Izjave o utjecaju proizvoda na okoliš – osnovna pravila za kategorizaciju građevnih proizvoda (EN 15804:2012+A2:2019). Sustain. Constr. works – Environ. Prod. Declar. – Core rules Prod. Categ. Constr. Prod.
HRN EN 17472 (2022). HRN EN 17472:2022 Održivost građevina – ocjenjivanje održivosti inženjerskih građevina–Metode proračuna (EN 17472:2022); Sustainability of construction works – sustainability assessment of civil engineering works – calculation methods.
HRN EN 1990 (2023). HRN EN 1990:2023 Eurokod – osnove projektiranja konstrukcija i geotehničkog projektiranja (EN 1990:2023); Eurocode -- Basis of structural and geotechnical design (EN 1990:2023).
HRN EN ISO 14044 (2008). HRN EN ISO 14044:2008 + A1:2018+ A2:2020: upravljanje okolišem–procjena životnog ciklusa (LCA) – zahtjevi i smjernice (ISO 14044:2006+Amd 1:2017+Amd 2:2020; EN ISO 14044:2006+ A1:2018+a2:2020); environmental management -- life cycle assessment – requirements and guidelines (ISO 14044:2006 + amd 1:2017+Amd 2:2020;EN ISO 14044:2006+a1:2018+a2:2020).
HRN EN ISO 14040 (2008). Upravljanje okolišem – procjena životnog ciklusa (LCA) – načela i Okvir rada (ISO 14040:2006; EN ISO 14040:2006); Environmental management – life cycle assessment – principles and framework.
HRN ISO 15686-5 (2023). HRN ISO 15686-5:2023 Građevine–Planiranje uporabnog vijeka -- 5. dio: trošak životnog ciklusa (ISO 15686-5:2017); Buildings and constructed assets -- Service life planning -- Part 5: life-cycle costing.
Huertas-Valdivia, I., Ferrari, A. M., Settembre-Blundo, D., and García-Muiña, F. E. (2020). Social life- cycle assessment: a review by bibliometric analysis. Sustainability 12, 6211. doi:10.3390/su12156211
Huijbregts, M., Margni, M., Hauschild, M., Jolliet, O., McKone, T., Rosenbaum, R., et al. (2015). “USEtox® 2.0 user manual, version 2,” in UNEP/SETAC scientific consensus model for characterizing human toxicoloical and ecotoxicological impacts of chemical emissions in life cycle assessment. Editor P. Fantke (Germany: Springer).
Huijbregts, M. A. J., Steinmann, Z. J. N., Elshout, P. M. F., Stam, G., Verones, F., Vieira, M. D. M., et al. (2017). ReCiPe 2016 v1.1 A harmonized life cycle impact assessment method at, mid point and end point level, Report I: characterization, RIVM Report 2016-0104a. Netherlands: National Institute for Public Health and the Environment, 201.
ILCD (2011). Handbook- recommendations for life cycle impact assessment in the European context. First edition, EUR 24571 EN. Luxemburg: Publications Office of the European Union.
Isa Olalekan, E., Emily, I., and Toheeb, J. (2023). “Life cycle sustainability assessment,” in Springer nature Switzerland AG 2023 Encyclopedia of sustainable management. Editor S. O. Idowu, (Germany: Springer). doi:10.1007/978-3-030-02006-4_289-1
Jena, T., and Kaewunruen, S. (2021). Life cycle sustainability assessments of an innovative FRP Composite footbridge. Sustain. Switz. 13 (23), 13000. doi:10.3390/su132313000
Khorgade, P., Rettinger, M., Burghartz, A., and Schlaich, M. (2022). A comparative crade-to-gate life cycle assessment of carbon fiber-reinforced polymer and steel - reinforced bridges. Struct. Concr., 1–14. doi:10.1002/suco.202200229
Leiva Maldonado, S. L., and Bowman, M. D. (2019). Life-cycle cost analysis for short- and medium-span bridges (joint transportation research program publication No.FHWA/IN/JTRP-2019/09). West Lafayette: Purdue University. doi:10.5703/1288284316919
Leroy Hulsey, J., Connor, B., Metzger, A., and Pitts, D. J. (2022). Life cycle costs for Alaska bridges, january 2015, Alaska university transportation center. USA: University of Alaska Fairbanks.
Long, A. E., Basheer, P. A. M., Taylor, S. E., Rankin, B. G. I., and Kirkpatrick, J. (2008). Sustainable bridge construction through innovative advances. Proc. Institution Civ. Eng. Bridge Eng. 161 (BE4), 183–188. doi:10.1680/bren.2008.161.4.183
Maier, P., Kuhlmann, U., Pfaffinger, M., Mensinger, M., Schneider, S., Zinke, T., et al. (2014). Sustainability assessment of bridges – recent German research results. Conf. Pap. I. doi:10.13140/2.1.1656.4483
Mara, V., Haghani, R., and Harryson, P. (2014). Bridge decks of fibre reinforced polymer (FRP): a sustainable solution. Constr. Build. Mater. 50, 190–199. doi:10.1016/j.conbuildmat.2013.09.036
Martínez-Muñoz, D., Martí, J. V., and Yepes, V. (2021). Comparative life cycle analysis of concrete and composite bridges’ varying steel recycling ratio. Materials 14, 4218. doi:10.3390/ma14154218
Mashhadia, A. H., Reza Ghiami Azadb, A., and Tavakolan, M. (2021). Life cycle cost comparison of strengthening a steel bridge using post-installed shear connectors with bridge reconstruction. Int. J. Constr. Manag., 1–12. doi:10.1080/15623599.2021.1969323
Milić, I., and Bleiziffer, J. (2024). Rating systems for the sustainability assessment of infrastructure. GRAĐEVINAR 76 (4), 335–345. doi:10.14256/JCE.3858.2023
Nasr, A., Björnsson, I., Honfi, D., Larsson Ivanov, O., Kjellström, J. J. & E., and Kjellström, E. (2019). A review of the potential impacts of climate change on the safety and performance of bridges. Sustain. Resilient Infrastructure 6, 192–212. doi:10.1080/23789689.2019.1593003
Navarro, I. J., Yepes, V., and Martí, J. V. (2018a). Social life cycle assessment of concrete bridge decks exposed to aggressive environments. Environ. Impact Assess. Rev. 72, 50–63. doi:10.1016/j.eiar.2018.05.003
Navarro, I. J., Yepes, V., Martí, J. V., and Gonzalez-Vidosa, F. (2018b). Life cycle impact assessment of corrosion preventive designs applied to prestressed concrete bridge decks. J. Clean. Prod. 196, 698–713. doi:10.1016/j.jclepro.2018.06.110
NEN-ISO 15686-5 (2017). Buildings and constructed assets -- Service life planning -- Part 5: life-cycle costing. ISO 15686-5:2017).
Next steps for a sustainable European future (2016). Next steps for a sustainable European future, European action for sustainability. China: COM, 739.
O Born, R. (2018). Life cycle assessment of large scale timber bridges: a case study from the world’s longest timber bridge design in Norway. Transp. Res. Part D 59, 301–312. doi:10.1016/j.trd.2018.01.018
Orcesi, A., O’connor, A., Diamantidis, D., Sykora, M., Wu, T., Akiyama, M., et al. (2022). Investigating the effects of climate change on structural actions. Struct. Eng. Int. 32 (4), 563–576. doi:10.1080/10168664.2022.2098894
Oztas, S. K., and Tanacan, L. (2014). Development of local weighting factors in the context of LCIA. Adv. Mater. Res. 935, 293–296.
Pedneault, J., Desjardins, V., Margni, M., Conciatori, D., Fafard, M., and Sorelli, L. (2021). Economic and environmental life cycle assessment of a short-span aluminium composite bridge deck in Canada. J. Clean. Prod. 310, 127405. doi:10.1016/j.jclepro.2021.127405
Penadés-Plà, V., Martínez-Muñoz, D., García-Segura, T., Navarro, I. J., and Yepes, V. (2020). Environmental and social impact assessment of optimized post-tensioned concrete road bridges. Sustainability 12, 4265. doi:10.3390/su12104265
Political Guidelines (2019). Political Guidelines of President elect Ursula von der Leyen: Political guidelines for the next Commission (2019-2024) – ‘A Union that strives for more: My agenda for Europe.
Psilca, v., Loubert, M., Maister, K., Di Noi, C., Radwan, L., and Ciroth, A. (2023). 3.1 A product social impact life cycle assessment database documentation. SrockaM: GreenDelta.
Santos Gervasio, H. M., and Silva, L. A. P. (2013). A design approach for sustainable bridges – Part 2: case studies. Eng. Sustain. 166, 201–214. doi:10.1680/ensu.12.00003
Sierra, L. A., Yepes, V., and Pellicer, E.: “A review of multi-criteria assessment of the social sustainability of infrastructures”. J. Clean. Prod.,2018, 187, pp. 496–513. doi:10.1016/j.jclepro.2018.03.022
Soliman, M., Ahmed, S. A., and Shen, L. (2019). A comprehensive framework for life-cycle cost assessment of reinforced concrete bridge decks.
Soukka, R., Väisänen, S., Grönman, K., Uusitalo, V., and Kasurinen, H. (2020).“Life cycle assessment,” in Springer nature Switzerland AG 2020 Encyclopedia of sustainable management. Editor S. O. Idowu, (Switzerland: Springer). doi:10.1007/978-3-030-02006-4_623-1
Steen, B. (2015). The EPS 2015d impact assessment method – an overview, department of energy and environment, division of environmental systems analysis, chalmers university of technology, göteborg, sverige 2015, Swedish life cycle center. Report number.
SuRe (2021). SuRe – the standard for sustainable and resilient infrastructure, ST01 ENG version 2.0. Switzerland: Global Infrastructure Basel Foundation.
Transforming our world (2022). Transforming our world: the 2030 agenda for sustainable development, A/RES/70/1. United Nations: General Assembly.
M. Traverso, M. N. Mankaa, S. Valdivia, L. Roche, A. Luthin, S. R. Garridoet al. (2022). Pilot projects on guidelines for social life cycle assessment of products and organizations (China: Life Cycle Initiative).
Tutorial, openL. C. A. (2020). Basic modelling in openLCA. Softw. Version:1.10, Man. Version 1.3. GreenDelta.
Umberto (2018). Umberto®, tutorial 1 – basic functions, DocVersion: 4.1. Publisher: ifu Hamburg GmbH. Available at: http://www.umberto.de.
UNEP (2020). in Guidelines for social life cycle assessment of products and organizations 2020. Editors C. Benoît Norris, M. Traverso, S. Neugebauer, E. Ekener, T. Schaubroeck, S. Russo Garridoet al. United Nations: United Nations Environment Programme UNEP.
UNEP (2021). in Methodological sheets for Subcategories in social life cycle assessment (S- LCA) 2021. Editors M. Traverso, S. Valdivia, A. Luthin, L. Roche, G. Arcese, S. Neugebaueret al. United Nations: United Nations Environment Programme UNEP.
van der Meer, Y. (2019). “Life cycle sustainability assessment,” in Decent work and economic growth. Editor W. Leal Filho, (Nature Switzerland AG: Springer). doi:10.1007/978-3-319-71058-7_18-1
WCED (2022). Report of the WCED: our common future. Available at: http://www.un-documents.net/wced-ocf.htm.
Wu, K., Yang, D. Y., Frangopol, D. M., and Jin, W. (2021). Multi-stakeholder framework for assessing the life-cycle social cost of construction projects. Struct. Infrastructure Eng. 18, 129–144. doi:10.1080/15732479.2021.1924795
Zhanli, C., Jintao, S., and Baiyong, F. (2021). Technical and economic analysis of the life cycle of huangmaohai cross-sea bridge. IOP Conf. Ser. Earth Environ. Sci. 787, 012161. doi:10.1088/1755-1315/787/1/012161
Zhao, X., Liang, D., Wu, M., and Kong, D. (2010). Parameter analysis of bridge life-cycle costs and its application in bridge design, ICCTP 2010: integrated transportation systems green· intelligen· reliable. China: ASCE, 3471–3479.
Keywords: bridges, life cycle assessment, life cycle cost, social-life cycle analysis, LCSA methods, functional unit, climate change, sustainability
Citation: Milić I and Bleiziffer J (2024) Life cycle assessment of the sustainability of bridges: methodology, literature review and knowledge gaps. Front. Built Environ. 10:1410798. doi: 10.3389/fbuil.2024.1410798
Received: 01 April 2024; Accepted: 26 June 2024;
Published: 13 August 2024.
Edited by:
Shehata E. Abdel Raheem, Assiut University, EgyptCopyright © 2024 Milić and Bleiziffer. This is an open-access article distributed under the terms of the Creative Commons Attribution License (CC BY). The use, distribution or reproduction in other forums is permitted, provided the original author(s) and the copyright owner(s) are credited and that the original publication in this journal is cited, in accordance with accepted academic practice. No use, distribution or reproduction is permitted which does not comply with these terms.
*Correspondence: Ivana Milić, aXZhbmEubWlsaWMyMTFAZ21haWwuY29t